DOI:
10.1039/D4NR03969F
(Review Article)
Nanoscale, 2025, Advance Article
Latest developments in the synthesis of metal–organic frameworks and their hybrids for hydrogen storage†
Received
27th September 2024
, Accepted 1st February 2025
First published on 7th February 2025
Abstract
Metal–organic frameworks (MOFs) are promising materials for hydrogen (H2) storage due to their versatile structures, high surface areas and substantial pore volumes. This paper provides a comprehensive review of MOF synthesis and characterization, as well as their practical applications for H2 storage. We explore various MOF synthesis techniques, highlighting their impact on the nanopore structure and functionality. Special emphasis is placed on strategies for enhancing H2 storage capacities by increasing specific surface areas, optimizing pore size distributions, and facilitating H2 release by improving thermal conductivity. Key advances in MOF-based hybrids, such as MOFs combined with carbonaceous materials, metals or other inorganic materials, are discussed. This review also addresses the effectiveness of linker functionalization and the introduction of unsaturated metal centers to optimize H2 storage under ambient conditions. We conclude that the development of competitive MOF-based hybrids, particularly those that incorporate carbons, offers significant potential for improving H2 storage and recovery, enhancing thermal stability and increasing thermal conductivity. These advancements are in line with the US Department of Energy (DOE) specifications and pave the way for future research into the optimization of MOFs for practical H2 storage applications.
1. Introduction
Hydrogen (H2) is a promising alternative energy carrier due to its potential to reduce greenhouse gas emissions when produced from renewable energy sources. However, efficient and safe storage of H2 remains a major challenge.1 Although H2 has the highest specific energy density, 33.6 kW h kg−1, it also has the lowest volumetric energy density, 0.0108 MJ L−1, measured under standard conditions,2 which is 3000 times less than that of gasoline.3 Hydrogen appears to be one of the most promising alternatives to achieve carbon neutrality, offering a clean energy source to replace fossil fuels in industries, transportation and power systems.4 Produced from renewables, it enables deep decarbonization and ensures a stable energy supply, driving the transition to a sustainable, low-carbon future.5
Storing H2 at pressures above 70 MPa or at temperatures below −253 °C is a possible alternative for increasing H2 density during transport and storage, but it requires harsh conditions and raises public safety concerns. Adsorption of H2 at −196 °C, the boiling point of liquid nitrogen, enables H2 to be stored at moderate pressures, of the order of 5 MPa, but requires high-surface area materials with a suitable pore size distribution (PSD) and high bulk density.
A wide range of carbon-based materials has been thoroughly studied for H2 storage, showing that excess H2 adsorption capacities at room temperature are lower, typically less than 2 wt%, compared with capacities observed at −196 °C, which remain generally below 7 wt%.6 For this reason, metal–organic frameworks (MOFs) have emerged as promising candidates for H2 storage due to their tunable porosity, high surface area and the possibility of tailoring their properties to specific applications.7 Excess H2 storage capacities in the range of 2.4 to 9.1 wt% have been obtained in MOFs with Brunauer–Emmett–Teller (BET) areas (ABET) of up to 6000 m2 g−1. The highest H2 storage capacity reported to date is 9.05 wt% at −196 °C and 7 MPa for NU100.8
Due to the limitations of the BET method, these very high ABET values are more indicative of pore volume than the actual surface area. Indeed, when excess H2 storage capacities at −196 °C are plotted as a function of ABET for a large number of MOFs, the observed trend does not follow Chahine's heuristic rule of 1 wt% H2 per 500 m2 g−1 of surface area. Moreover, increasing ABET leads to an increase in average pore volume and thus to a decrease in adsorbent–sorbate interactions and isosteric enthalpy of adsorption. The increase in pore volume also results in a reduction of particle density. Indeed, while absolute gravimetric H2 capacities reach values as high as 120 mg H2 per g (12 wt%), volumetric H2 capacities peak at values of around 40 kg H2 per m3. Thus, MOFs with high ABET and high absolute H2 uptake on a gravimetric basis are not necessarily those with the highest H2 uptake on a volumetric basis.
In this field, MOF hybrids, i.e., MOFs combined with other materials, such as other MOFs, carbon-based materials or metallic particles, have shown promise as a viable choice for H2 adsorption by increasing their adsorption capacity through an increased specific surface area.9,10 The addition of carbon-based materials to produce MOF hybrids can improve thermal conductivity and allow tuning of the chemical composition to provide greater selectivity11 for H2 adsorption compared with other gases. A bibliometric analysis (conducted in English in the Scopus database, followed by an analysis using the Bibliometrix package in R language) was carried out to give an overview of the use of MOFs for H2 adsorption. A clear upward trend in the use of MOFs for H2 storage is observed over time, with a peak of 111 publications in 2024 (last access 15 August 2024) (Fig. S1†). Another bibliometric analysis has been performed, now considering the use of MOF-based hybrids for H2 adsorption. A clear upward trend in the use of MOF-based hybrid materials for H2 storage is observed over time, especially after 2016, peaking in 2024 (last access 15 August 2024) with 27 publications, followed by 2019 and 2022 with 24 publications each (Fig. S2†).
This review aims to provide a comprehensive overview of the current state of research in H2 storage, using MOFs as materials of interest, focusing on the enhancement of the properties of MOF hybrids, the challenges faced and future prospects, including their potential applications in the energy industry.
2. Basic aspects of MOF synthesis and characterization
MOFs or porous coordination polymers (PCPs) are materials containing metal ions connected by organic linkers to form a 3D network.14 The metal ions form primary building units (PBUs) with precise geometric structures, linked by secondary building units (SBUs).15 Commonly used metal ions include Cr3+, Fe3+, Co2+ and Zn2+,16 with coordination numbers from 2 to 7, yielding various geometries such as linear, T-shaped, tetrahedral, square planar, octahedral, etc.17 Organic linkers, such as carboxylates, phosphates, sulfonates, etc., can form coordination bonds, with fumaric, succinic, and terephthalic acids being low-cost options. Other ligands such as peptides, carbohydrates, amino acids and cyclodextrins are also used.18 Different classes of MOFs exist, of which “MOFs” is the abbreviation and general name,19 and their discovery is the result of the collective efforts of many research groups and laboratories around the world. Several major laboratories have made significant contributions to the field of MOFs, including materials from Universitetet i Oslo (UIO), Institut Lavoiser (MIL) and Northwestern University (NU), among others (Fig. S3a†).
MOFs have high surface area, tunable pore size and diverse functionalities, making them attractive for various applications such as gas storage, separation, catalysis and sensing.20 In Fig. 1a, (–CO2)x represents the organic linker, with x being the number of linkers associated with the cluster, giving rise to the crystalline geometry of the MOF. MOFs are also highly customizable, with the ability to vary not only the metal ion (Fig. 1b) and the organic linker (Fig. 1c), but also the synthesis conditions to tailor the material's properties and provide different varieties of MOFs with varied characteristics of interest.
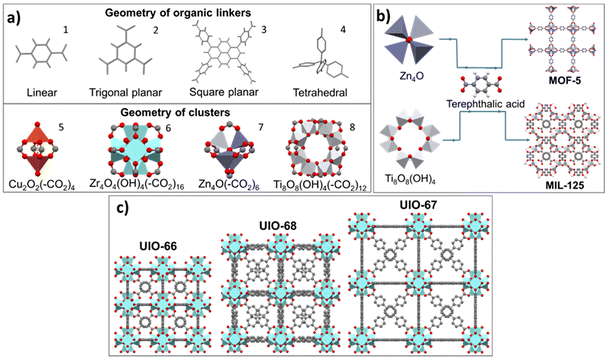 |
| Fig. 1 (a) Geometry of some organic linkers (1: BDC; 2: BTC; 3: H4TBAPy and 4: tetraphenylmethane) (adapted from ref. 12 and 13), and geometry of some clusters; (b) assembly of two different clusters with the same organic linker to obtain two MOFs; (c) assembly of the same cluster (Zr4O4(OH)4(–CO2)16) with 3 linkers to obtain three different MOFs. | |
2.1. MOF synthesis
The energy required for MOF synthesis, to allow linkage between PBUs and SBUs, can be provided by a variety of synthesis approaches, including solvothermal/hydrothermal, sonochemical, electrochemical, microwave-assisted and mechanochemical, which can be applied to produce MOFs with contrasting structures and features. Table 1 shows some advantages and disadvantages of each of these approaches, which are briefly reviewed, from the classical solvothermal/hydrothermal route to the Resonant Acoustic Mixing (RAM) route:
- Solvothermal (with organic solvents) or hydrothermal synthesis (with water) takes place in sealed vessels above the boiling point of the liquid medium under standard pressure.21 Conventional solvothermal/hydrothermal synthesis involves heating metal ions, linkers and solvents together (80–200 °C)22 in a polytetrafluoroethylene (PTFE)-lined autoclave (for high temperatures) or in scintillation vials (for lower temperatures).16 The synergy of pressure, temperature and solvent produces single crystals due to the material's solubility in a hot and pressurized solvent, whether organic or aqueous,22 which favors reaction and crystal growth. Although solvothermal/hydrothermal synthesis produces well-formed MOFs with controlled size, it requires longer synthesis times and often organic solvents. Zheng et al.23 compared hydrothermal, reflux, vessel, and microwave methods to synthesize MOF-303 for water harvesting from desert air. The hydrothermal method took 24 h, while the reflux and vessel methods needed 4 to 8 h. Microwave synthesis was the fastest at 5 min.
- Non-solvothermal synthesis takes place at temperatures below the boiling point of the solvent under standard pressure, usually at room temperature.16 For instance, some widely used MOFs such as MOF-5, MOF-74, MOF-177, HKUST-1, IRMOF-0, MIL-53(Al), MOF-2 and ZIF-7 have been successfully synthesized under ambient conditions.24–28 The shape of the crystals obtained and the percentage of purity are strongly influenced by the reaction temperature.29 Yields are also affected by temperature, as shown by Lestari et al.,30 who reported a 24% yield for HKUST-1 at room temperature versus 99% at 120 °C. However, by using ambient temperature, other synthetic routes emerge as alternatives, broadening the horizons for future research.
- Sonochemical synthesis uses ultrasound to create nanomaterials with the help of sound-wave-induced bubbles. The small bubbles promote interactions between particles up to 13 eV, leading to significant reactions. The bubble stages – formation, growth and collapse – induce chemical reactions in the synthesis of MOFs.31,32 Reaction time has a significant influence on crystal size in sonochemical synthesis. Qiu et al.33 found that short runs (5–10 min) produced spherical nanocrystals of 50–100 nm, while longer runs (30–90 min) produced needle-shaped crystals of 700–900 nm. Vaitsis et al. concluded that ultrasound outperforms electrical and microwave heating due to its simplicity, faster reactions and improved energy efficiency, but with the use of organic solvents.34
- Electrochemical synthesis was first used to synthesize HKUST-1 in 2005, paving the way for various electrochemical methods and materials design for MOFs, including MOF-5,35 MIL-53,36 MIL-100(Fe)37 and ZIF-8.38 Electrosynthesis is practical and cost-effective, as it operates under milder conditions and within shorter timescales. It offers advantages such as mild reactions, easy operation and efficient charge transfer, which promotes rapid MOF crystal growth.35,39 Antonio et al.40 used electrosynthesis to prepare titanium(III)-based MOFs, namely TiIII-MIL-101 and TiIII-MIL-102, and extended it to other TiIII-MIL structures using TiCl4. The materials produced by electrosynthesis showed similar characteristics to the more expensive TiCl3, demonstrating its cost-effectiveness. However, electrochemical synthesis often involves organic solvents, which can potentially impact the surface area, pore volume and thermal stability.41
- Microwave-assisted synthesis is an effective method for high-throughput syntheses, as it heats the material uniformly due to molecular dipolarity and ionic conduction.42 Rapid microwave heating can generate hot spots that give rise to metastable materials.43 However, after careful control of the synthesis conditions, this approach increases efficiency as crystals disperse throughout the solution, leading to faster and higher yields due to rapid consumption of reagents.44 Although crystals are smaller than with traditional methods, microwave crystallization reduces synthesis time and material use while maintaining the surface area.45–47 Wu et al.48 synthesized conductive, partially carbonized metal–organic frameworks with ruthenium nanoparticles using household microwave ovens. Among them, Ru@p-Co3HHTP2-3.2% exhibited excellent performance in the electrocatalytic H2 evolution reaction. These studies highlight the potential of microwave-assisted methods for the efficient synthesis of advanced materials with enhanced H2-related properties, but they are generally associated with the use of organic solvents.
- Ionothermal synthesis uses ionic liquids (ILs) as solvents or templates, resulting in MOFs with a negatively charged skeleton structure. The IL cations are firmly integrated into the MOF framework.49 ILs have properties that can be advantageous over traditional solvents, such as their extremely low vapor pressures. They can advantageously replace organic solvents such as N,N-dimethylformamide (DMF).50 This synthesis can operate at lower temperatures such as 30 °C, consuming less energy than the solvothermal approach. Peng et al. synthesized mesoporous Cu-MOF nanoplates using ILs.51 The resulting material had high crystallinity and combined the properties of a mesoporous material with small particle sizes. In 2014, Liu et al. introduced an innovative approach for immobilizing ILs into ZIF-8 membranes. ZIF-8's inherent hydrophobicity and ability to act as a template with an ionic liquid effectively confined the IL within the membranes and reduced leaching when exposed to water.52
- Mechanochemical synthesis uses mechanical energy, such as compression, shear or friction, to facilitate chemical transformations. This technique is particularly relevant to green chemistry, as it eliminates or reduces the need for solvents.53 Mechanochemical synthesis is a promising method for the large-scale production of MOFs due to its advantages, such as solvent-free reactions at room temperature and relatively short reaction times of 10 to 60 min. Ball milling is the primary process used to crush precursors between balls, resulting in bond breakage, defects and increased reactivity.54,55 Over the past decade, mechanochemical synthesis of MOFs has performed well compared with conventional techniques, as shown in reviews56 or book chapters.57 Although ball milling is widely used, it has limitations such as sample damage and non-uniform mixing. Other methods, such as RAM, can overcome these problems. RAM uses low-frequency, high-intensity acoustic energy to gently mix materials without physical contact with balls.58 Titi et al. demonstrated that the RAM technique is more advantageous than ball milling for synthesizing ZIF-L, ZIF-8 and HKUST-1.59 RAM enables larger batches to be synthesized while retaining control over product composition and particle size.
Table 1 Advantages and disadvantages of different MOF synthesis methodologies/strategies
Synthesis method |
Advantages |
Disadvantages |
Solvothermal/hydrothermal |
○ High crystallinity63 |
○ Long synthesis times30 |
 |
○ Control of morphology64,65 |
○ Use of organic solvents67 |
○ Control of particle size66 |
Non-solvothermal |
○ Energy saving by using room temperature68,69 |
○ Low yield70 |
 |
○ Use of organic solvents26 |
Sonochemical/ultrasound |
○ Control of crystal growth33 |
○ Use of organic solvents74 |
 |
○ High yield71,72 |
○ Short synthesis times73 |
Electrochemical |
○ Easy to scale75 |
○ Reduction in ABET39,41 |
 |
○ Short synthesis times76,77 |
○ Use of organic solvents78 |
Microwave-assisted |
○ Short synthesis times79,80 |
○ Small crystal size44 |
 |
○ Use of organic solvents21 |
Ionothermal |
○ Ionic liquids replace organic solvents81,82 |
○ Reduction in ABET83 |
 |
Mechanochemical |
○ Solvent-free57,84 |
○ Difficult to control morphology and particle size86 |
 |
○ Short synthesis times85 |
MOF formation, layout and shape are affected not only by the building blocks, but also by factors such as solvent, pH, temperature, reagent concentration, reaction time, molar ratios, counter ions and pressure. These factors fall into two categories (Fig. S3b†): (1) compositional parameters – solvent, pH, molar ratios, counter ions, and concentration; and (2) process parameters – pressure, time, and temperature.60 In assembly processes, solvents coordinate with metal ions or integrate into the lattice.61 Although they do not integrate directly into the MOF, solvents play a role in structure orientation and crystal growth. The choice of solvent influences ligand deprotonation, which in turn has an impact on the MOF structure.60 For instance, using different solvents, Banerjee et al. revealed various crystal structures in MOFs synthesized with magnesium and 3,5-pyridine dicarboxylic acid (PDC).62 Water coordinated best with magnesium, but the maximum ABET value achieved was 52 m2 g−1. Acidity/basicity has a significant influence on the crystallization and growth of inorganic–organic composite materials. pH has an impact on ligand deprotonation, strengthening the bond between ligands and metal ions through pH adjustments.60 Temperature also has a significant impact on MOF characteristics, as demonstrated by Biemmi et al., who found that temperature variations (from 75 to 180 °C) altered the morphology and purity of HKUST-1.29
Synthesis time has a significant effect on MOFs’ crystal size, as shown by Xu et al. who synthesized MOF-808 at 120 °C using ZrCl4/BTC (3
:
1 ratio) for 36–96 h.87 During the first 36–72 h, ABET and micropore volume increased from 1052 to 2177 m2 g−1 and from 0.30 to 0.72 cm3 g−1, respectively. After 72–96 h, ABET and micropore volume decreased to 968 m2 g−1 and 0.28 cm3 g−1, respectively. Suresh et al.88 studied various process parameters to optimize MOF synthesis for H2 storage. The researchers investigated the effect of reagent concentration, temperature and reaction time on crystal size distribution. Shorter reactions (12–18 h) at higher temperatures (110–150 °C) produced cubic crystals with a wide size distribution (200–1300 μm), whereas longer reactions (24–72 h) at lower temperatures (60–90 °C) produced crystals with a narrower size distribution and with a 15% reduction in ABET.
2.2. MOF characterization
Characterizing MOFs is a crucial step in understanding their structure and properties. The main techniques to characterize a MOF are as follows: X-ray diffraction (XRD), to identify the crystal structure and unit cell dimensions; scanning electron microscopy (SEM), which provides images of surface morphology and particle size distribution (Fig. 2a); thermogravimetric analysis (TGA), to determine thermal stability and weight loss behavior upon heating (Fig. 2c); gas sorption analysis, to determine pore volume, pore size distribution and the surface area (Fig. 2b); Fourier-transform infrared spectroscopy (FTIR), to identify functional groups present; and elemental analysis to find out the composition of the MOF, usually in terms of carbon, nitrogen and oxygen contents. These analyses provide valuable information on the physical and chemical properties of MOF materials. This information is crucial for understanding their potential applications.
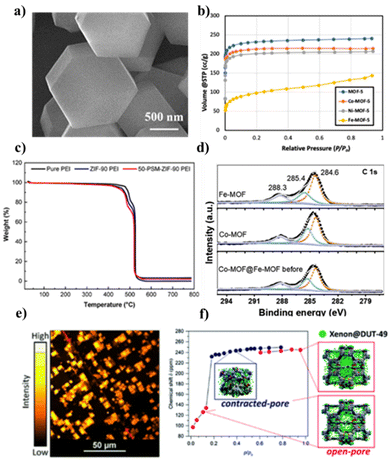 |
| Fig. 2 Main MOF characterization techniques: (a) SEM images of ZIF-8 (reproduced from ref. 89 © 1996–2024 MDPI); (b) nitrogen adsorption isotherms at −196 °C of MOF-5 and doped MOF-5 (reproduced from ref. 90 © 1996–2024 MDPI); (c) TGA of pure PEI, ZIF-90 PEI and 50-PSM-ZIF-90 PEI membranes (reproduced from ref. 91 © 1996–2024 MDPI); (d) XPS spectra of Fe-MOF, Co-MOF, Co-MOF@Fe-MOF, and the hybrid MOF (reprinted with permission from ref. 92; Copyright © 2024 with permission from Elsevier. Also Lancet special credit, reprinted from The Lancet from ref. 92; Copyright © 2024 with permission from Elsevier); (e) confocal fluorescence imaging of defects in a MOF-5 single-crystal (reproduced from ref. 93 © 1999–2024 John Wiley & Sons, Inc. or related companies); (f) chemical shift of xenon adsorbed in DUT-49 at −73 °C measured during the adsorption experiment (red symbols indicate DUT-49op signals; blue symbols indicate DUT-49cp signals) (reprinted with permission from ref. 94; Copyright(2017) American Chemical Society). | |
Other promising but less explored techniques are described in Fig. 2. Confocal fluorescence microscopy has the unique ability to capture three-dimensional images at the level of individual crystals (Fig. 2e), while offering the sensitivity needed to investigate the initiation of defect formation. Raman spectroscopy provides detailed information on the molecular vibrations and vibrational modes present in a material, which can be useful for studying the structure, composition and properties of MOFs. Confocal laser scanning microscopy can be used to observe the stability of MOF hybrids if they contain a fluorescent component such as carbon dots (CDs). XPS spectra are used to analyze the elemental composition and chemical state of a material's surface, to confirm the doping of a MOF (Fig. 2d), and nuclear magnetic resonance (NMR) can be used to study the structure of organic components in MOFs and the interaction between organic molecules and metal ions (Fig. 2f).
3. Fundamental properties and performance of MOFs for hydrogen storage
The characteristics required to design the ideal MOF for H2 sorption have been reported in several studies.15,95,96 In general, MOFs must have a high surface area and a large number of relatively small, interconnected pores to maximize H2 adsorption. Low-density MOFs are disadvantageous as they can lead to weak H2–H2 interactions in the channels, reducing adsorption capacity. Additionally, volumetric H2 storage capacities are also reduced. When used near room temperature, the inner surface of MOFs must feature local polar groups to enhance interactions between MOFs and H2 molecules, and this requirement relates specifically to the properties of the organic linker used in MOF synthesis.
3.1. Specific surface area
H2 is adsorbed on the surface of the material and stored in the pores (physisorption). In general, micropore volume is directly related to the surface area of a material. Consequently, to enhance H2 adsorption capacity at −196 °C, it is necessary to increase both specific surface area (SSA) and micropore volume within the MOF. Fig. 3a shows a limitation of the BET method in representing excess H2 uptake in wt% (at −196 °C) of MOFs as a function of their ABET. It demonstrates that for very high ABET, Chahine's heuristic rule is not valid, as the BET method accounts for pore volume more than SSA. The isosteric enthalpy of adsorption ((–ΔH)ads) exhibits a linear decrease with increasing average pore diameter (Fig. 3b). This phenomenon is attributed to stronger adsorption forces in narrow pores, due to overlapping van der Waals forces. The presence of 1.5 to 2.5 nm pores has been shown to significantly enhance H2 storage capacity at high pressures. However, nanopores smaller than 1.5 nm are found to be the most efficient for H2 storage at all pressure levels.97 H2 interactions with MOFs can be enhanced by reducing pore size, with the optimum size corresponding to the kinetic diameter of the H2 molecule (0.289 nm),98 although porous materials with a unimodal PSD of around 0.3 nm and high surface areas are difficult to obtain.
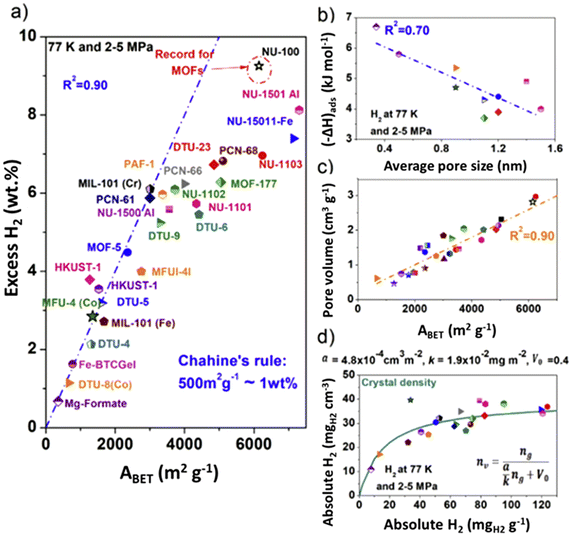 |
| Fig. 3 (a) Excess H2 uptake at −196 °C and 2–7 MPa of metal–organic frameworks (MOFs) reported in recent studies ( ) ref. 111, ( ) ref. 104, ( ) ref. 112, ( ) ref. 113, ( ) ref. 8, ( ) ref. 114, ( ) ref. 115, ( ) ref. 116. (a) Chahine's rule (dotted line) applied to MOFs; (b) isosteric heat of adsorption (−ΔH)ads as a function of average pore size; (c) evolution of pore volume with ABET; (d) volumetric versus gravimetric absolute hydrogen uptake of porous materials using the density of the single crystal for its calculation for MOFs: ( ) ref. 111, ( ) ref. 104, ( ) ref. 112, ( ) ref. 113, ( ) ref. 8, ( ) ref. 114. The green line in (d) corresponds to the model proposed by Balderas-Xicohténcatl et al. in ref. 101. The absolute uptake from the excess reported data was calculated using REPROF software to calculate the density of H2 gas and assuming that the volume of the adsorbed phase volume is equal to Vpore. (reprinted with permission from ref. 117; Copyright © 2024 with permission from Elsevier. Also Lancet special credit, reprinted from The Lancet from ref. 117; Copyright © 2024, with permission from Elsevier). | |
Fig. 3c illustrates a linear relationship between SSA and pore volume for different MOF families. This behavior was previously reported by Gómez-Gualdrón et al., who calculated the ABET of microporous and mesoporous MOFs.99 In Fig. 3d, absolute volumetric H2 adsorption is plotted against absolute gravimetric H2, showing that for MOFs with high BET areas, a plateau in H2 uptake on a volumetric basis is reached. The latter was determined using the following equation, deduced from H2 uptake on several MOFs, at −196 °C and in a pressure range of 2 to 2.5 MPa:100,101
In this equation, nv and ng are the volumetric and gravimetric absolute uptakes, respectively; α represents the slope of the relationship between volumetric absolute hydrogen uptake (measured at −196 °C and 2.0–2.5 MPa) and the volumetric surface area, which is derived from single-crystal densities; κ is the H2 surface density, and V0 is the density of the MOF single-crystal.
The ability of any adsorbent to store H2 (wt%) is closely linked to its SSA. Moreover, there is a linear correlation between the wt% of H2 stored and the BET area.102 The shapes of N2 isotherms observed for physisorption on MOFs, which are often type I with minimal or no hysteresis, indicate the presence of well-defined microporous structures. The pronounced rise at very low p/p0 is attributed to adsorbent–adsorbate interactions in these narrow micropores.20,103
3.2. Isosteric enthalpy of hydrogen adsorption
In gas storage, especially in H2 storage on MOFs, van der Waals forces are considered the main gas–adsorbent interaction and contribute to the decrease in H2 storage pressure in the system.19 For practical storage applications, a reversible mechanism is required for the adsorption and release of H2 from its storage material. The low physisorption of H2 on MOFs offers an advantage in this regard, as it allows H2 to be adsorbed on pore surfaces within the MOF and readily released on demand. However, the challenge lies in the low (–ΔH)ads of H2 on most known MOFs. For optimum hydrogen storage and release cycles at room temperature, an adsorbent should have a (–ΔH)ads between 15 and 25 kJ mol−1. This range is crucial when considering the system's operating pressures. The release pressure corresponds to the pressure after most of the hydrogen has been released from the adsorbent. If (–ΔH)ads is too high, desorption will require higher temperatures or lower pressures, which may not be practical under ambient conditions. Conversely, the storage pressure is the pressure at which the adsorbent is fully loaded with hydrogen. If (–ΔH)ads is too low, the adsorbent may not retain hydrogen effectively at this pressure, leading to inefficient storage.104–106 Unfortunately, the majority of MOFs exhibit (–ΔH)ads values between 5 and 12 kJ mol−1.107
Recent studies have provided H2 adsorption isotherms for more than 30 MOFs, covering a pressure range of up to 1 bar.108 Among the challenges posed by these porous materials for H2 adsorption are: achieving high H2 uptake under ambient conditions, improving the kinetics of H2 adsorption and desorption, and increasing material stability and durability. While MOFs exhibit significant H2 uptake at cryogenic temperatures, reaching up to 99.5 mg g−1 (approximately 9 wt%) at 56 bar and −196 °C,8 their storage capacities at room temperature are generally less than 1 wt%.95 This limitation is due to the weak interaction, typically ranging from −12 to −5 kJ mol−1, between H2 molecules and MOFs.109 However, as temperature increases, H2 storage capacity decreases significantly, and none of the existing MOFs currently meet the target set by the US DOE at room temperature.107 According to the US DOE, materials must have a minimum H2 uptake capacity of 5.5 wt% under moderate temperature and pressure conditions.
The amount of energy released by interactions between the MOF surface and H2 molecules can be determined by analyzing H2 adsorption isotherms obtained at least at two distinct temperatures, typically −196 and −186 °C. This is achieved by fitting the experimental data to either the virial equation or the Langmuir–Freundlich equation.107 Bae and Snurr110 conducted a study of H2 storage and release with a MOF at pressures between 1.5 and 120 bar using Grand Canonical Monte Carlo simulations. The aim was to determine the ideal value of (–ΔH)ads to maximize H2 delivery. The simulations revealed that the optimum (–ΔH)ads value for this purpose is approximately 20 kJ mol−1, in agreement with other works.104–106
Linker functionalization seems to be an effective approach for enhancing the H2 adsorption enthalpy of MOFs and improve hydrogen storage capacity, either through direct modification or post-synthetic modification of the linker.118 In this context, Han et al. enhanced UiO-66 by incorporating dihydroxy and dialkoxy groups into its linker. This modification resulted in UiO-66-(OCH2CH3)2, which exhibited a 98.3% improvement in performance compared to the unmodified UiO-66.119
The incorporation of different functional groups such as –CH3, –NH2, –OH, and –Br into the organic linker results in a series of isoreticular MOFs whose fundamental framework remains unchanged and which can effectively increase the (–ΔH)ads.120 The potential of linker functionalization can be seen with tetrazolate-based ligands, which generate a series of robust, microporous materials. Dinca et al.121 successfully synthesized nitrogen-rich MOFs using 1,4-benzeneditetrazolate as an organic linker, achieving H2 storage densities of up to 1.46 wt% at ambient pressure. Wang et al. covalently modified MOFs (IRMOF-3, UMCM-1-NH2 and DMOF-1-NH2) with a series of anhydrides or isocyanates: storage adsorption capacities (gravimetric and volumetric gas uptakes) and (–ΔH)ads demonstrated that post-synthetic covalent modifications can greatly enhance the sorption affinity of MOFs for H2.122
Another way to strengthen the interaction between hydrogen and MOFs is through the introduction of unsaturated metal sites. Developing these sites effectively enhances the enthalpy, thereby improving hydrogen storage performance. This is commonly achieved by removing coordinated solvents using methods such as heating, vacuum heating, or supercritical drying.123 Sengupta et al. synthesized a robust Cu(I)-based MOF, called NU-2100, which contains open metal sites. To access these open metal sites, the MOF underwent an activation process. The study concluded that the activated MOF exhibited a high enthalpy of adsorption of 30 kJ mol−1.124
3.3. Hydrophobicity
To achieve optimal performance and recyclability in H2 storage, one of the most important objectives is to improve the water stability of MOFs, which are sensitive to moisture.125,126 When MOFs are exposed to moisture from the air, the organic ligands in the framework are replaced by water molecules. This substitution disrupts the metal–linker bonds, which are generally the weakest points of the MOF structure. As a result, the MOF structure partially decomposes, leading to a reduction in SSA.127,128 There are two approaches for addressing this challenge. The first is the direct synthesis of water-stable MOFs, which can be achieved by incorporating non-polar functional groups into the organic linkers.129,130 The second method involves post-synthetic modifications, which include encapsulation of hydrophobic guest molecules in the MOF's pores and channels,131 as well as functionalization of the MOF's external surface.132 Qian et al. have synthesized a hydrophobic DUT-4 by a facile solution-immersion approach. After exposure to aqueous solutions, DUT-4 treated with a hydrophobic coating successfully preserved its crystal structure, morphology, surface area and H2 uptake capacity.133
3.4. Thermal conductivity
Thermal conductivity within MOFs is a crucial but often overlooked issue in numerous adsorption-based applications, including gas storage and separation. In adsorptive gas storage applications, the heat generated by exothermic gas adsorption can potentially lead to a considerable increase in temperature (hot spots) and decrease the MOF's adsorption capacity if not promptly dissipated.134 Effective thermal conductivity is a crucial parameter for assessing the rate at which adsorption heat dissipates and for devising strategies to maintain a lower temperature in a fuel storage tank while it is being filled with hydrogen.135 Islamov et al. reported that MOFs exhibit enhanced thermal conductivity when they have high densities (>1.0 g cm−3),136 small pores (<1 nm)137 and four-connected metal nodes.138 The highly porous nature and low density of MOFs prevent efficient phonon transport139 and result in low thermal conductivities (<2 W m−1 K−1).140
3.5. Tap density
The use of MOF powders in large industrial reactors can lead to pressure drops, clogs and complex handling. To mitigate these issues, MOF powders are shaped into defined structures using various methods such as pelletization, extrusion, granulation, spray-drying or 3D printing.141 This shaping process usually involves the incorporation of binders to improve the mechanical stability of the pellets, which is highly advantageous. Binders generally fall into two categories: organic binders, which include starch, cellulose and polyvinyl alcohol, and inorganic binders, such as clay, silica and graphite.142,143 Factors such as pore collapse, pore blocking and amorphization of the crystalline structure can lead to a reduction in the storage capacity of compressed MOFs.144 Packing efficiency is a key factor in determining the H2 storage capacity of MOFs. Research on the packing density on real MOF samples with different crystal shapes and sizes is the most effective method for gaining insight into packing efficiency. The choice of the synthesis method and the precise adjustment of various parameters (such as temperature, synthesis time, pH, concentration, etc.) are essential in determining the size and shape of the resulting crystal.145 Suresh et al. designed a strategy to improve packing efficiency and significantly increase the volumetric storage density of H2 gas by controlling the crystal morphologies and crystal size distributions for MOF-5.88 This strategy has the potential to minimize the structural damage when the MOF is compacted, thereby maximizing the H2 storage capacity compared with compacted commercial MOF-5. The synthesis method chosen can also alter the crystal size. For example, Leng et al. synthesized MIL-101(Cr) by mechanosynthesis, obtaining crystals between 40 and 200 nm, in contrast to the 300 to 500 nm crystals achieved by solvothermal methods.146 The choice of the appropriate synthesis route thus plays an important role in MOF crystal size, which can result in better packing.88
3.6. Thermal stability
The thermal stability of a MOF refers to its ability to avoid irreversible changes to its structure when heated to a specific temperature.147 Yuan et al. highlight that the stability of MOFs is strongly influenced by various factors, such as the framework structure, particle size, crystal defects, and operational conditions,126 but overall, two factors have been identified to contribute the most to the stability of MOFs: the properties of the linkers and the metal–linker bonds.148
Recognizing the key influence of linkers on the thermal stability of MOFs, Mohamed et al. studied the impact of using functionalized linkers in MOF-5. They concluded that non-functionalized linkers exhibit greater thermal stability.149
The thermal stability of amorphous regions of MOFs is generally much lower than that of crystalline regions of similar composition.150 During the heat treatment process, degradation of MOF structures can lead to amorphization, melting, cluster dehydration or dehydrogenation of the organic ligand.151–153 Increased thermal stability results in more stable and durable materials.
4. Hydrogen adsorption on MOFs and their hybrids
MOF hybrids are materials that combine MOFs with other substances to create new materials with improved properties or functionalities.163 The aim of combining MOFs with alternative materials is to overcome the limitations of MOFs, such as low stability, poor mechanical properties or high production costs. In addition, the hybrids can be tailored to specific applications such as H2 capture, with improved efficiency and performance.164–167 Table 2 presents the advantages and disadvantages of certain types of MOF hybrids. The following sections provide a detailed description of each selected hybrid.
Table 2 Advantages and disadvantages of different MOF hybrids
Hybrids |
Advantages |
Disadvantages |
Interpenetrated MOFs (IMOFs) |
○ Improved framework stability154 |
○ Surface area potentially decreased156 |
 |
○ Larger adsorption selectivity155 |
MOF-on-MOF |
○ Properties of different MOFs combined157 |
○ Difficult synthesis11 |
 |
MOF/carbon-based materials |
○ Increased ABET158 |
○ Decreased crystallinity159 |
 |
○ Increased H2 adsorption (−196 °C)159 |
○ Increased thermal conductivity143 |
MOF/metal particles and ions |
○ Increased H2 adsorption (25 °C)160 |
○ Decreased surface area162 |
 |
○ Enhanced adsorption enthalpy161 |
4.1. MOF@MOF hybrids for hydrogen adsorption
MOF@MOF hybrids refer to materials composed of two MOFs combined into a single system. By combining two MOFs, it is possible to achieve various advantages aimed at increasing H2 adsorption capacity, such as reducing pore size, increasing gas selectivity and improving MOF stability.168,169 Fig. 5 shows the influence of combining two MOFs on H2 adsorption at −196 °C and 25 °C. Combining MOFs by interpenetration can have a reducing effect on the SSA of the resultant hybrids. However, all samples show an increase in H2 adsorption.
4.1.1. Interpenetrated MOFs (IMOFs). The presence of large pore spaces in the MOF system can be associated with instability and subsequent collapse of the structure. To solve this problem, interpenetration (also called catenation or interweaving) can be used, as it allows the entanglement of two identical MOFs (homo IMOFs) or two different MOFs (hetero IMOFs) that are not directly connected but cannot be separated without breaking bonds154,170–172 (Fig. 4). Interpenetration minimizes vacant zones and has the potential to significantly improve framework stability. It allows empty spaces to be occupied and generates repulsive forces that prevent individual networks from collapsing. Consequently, the synthesis of MOFs with adjustable pore sizes using elongated organic linkers poses considerable challenges, as the formation of interwoven frameworks should be preferred to improve stability.173 Catenation can be used to produce materials with fine pores and enhance adsorption heat by increasing the overlap of attractive potential between opposing pore walls. As a result, the interpenetrated framework shows a significant reduction in pore size, resulting in stronger interaction with H2 molecules.100
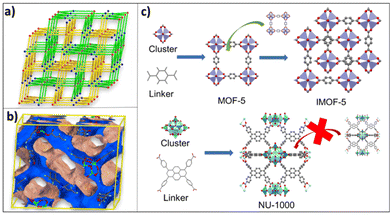 |
| Fig. 4 (a) Topological representations of interpenetration along the a-axis; (b) the 1D channel viewed along the a-axis (a) and (b) reproduced from ref. 174, with these figures having been published in CCS Chem. [2022]; [Ultrahigh hydrogen uptake in an interpenetrated Zn4O-based metal–organic framework] is available online at https://10.31635/ccschem.021.202000738; (c) simplified topology of an interpenetrated MOF-5 and the impossibility of NU-1000 interpenetration. | |
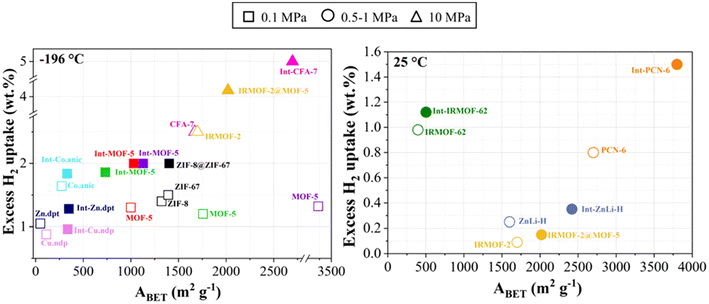 |
| Fig. 5 Excess H2 storage capacities of MOFs and their MOF@MOF hybrids at −196 °C (left) and 25 °C (right). ( ref. 175); ( ref. 176); ( ref. 177); ( ref. 178); ( ref. 174); ( ref. 100); ( , ref. 179); ( ref. 180); ( ref. 181); ( ref. 182); ( ref. 183); ( ref. 184). | |
Ma et al. (2007) investigated the effect of interpenetration on H2 adsorption in PCN-6, a porous coordination network. The N2 adsorption isotherm at −196 °C shows that the non-interpenetrated material has a Langmuir surface area of 2700 m2 g−1, while the interpenetrated material has a Langmuir surface area of 3800 m2 g−1, representing a 41% increase. Interpenetration resulted in a 133% increase in volumetric H2 uptake (3.94 kg m−3 for non-interpenetrated frameworks vs. 9.19 kg m−3 for interpenetrated frameworks) and a 29% increase in gravimetric H2 uptake (1.35 wt% for non-interpenetrated frameworks and 1.9 wt% for interpenetrated frameworks).185
Jiang et al. also used a MOFMC for H2 storage, an interpenetrated MOF-5@Multi-Walled Carbon Nanotubes (MWCNTs), called Int-MOFMC-meso.175 The latter was able to store 2.02 wt% H2 at −196 °C under 1 bar. This value is higher than those obtained for the aforementioned MOFMC materials and indicates better thermal and moisture stability. However, the ABET was significantly lower than that of previous MOFMCs: 805 m2 g−1. According to the authors, the pores were not filled with zinc species or solvent, but it was the presence of an interpenetrated structure and the unsaturated metal site present on the mesopore surface that improved H2 storage performance, even with a lower ABET.175
4.1.2. MOF-on-MOF. Extensive studies on MOFs have led to the development of a promising class of hybrid materials known as MOF-on-MOF, as shown in Fig. 6a. The left-hand side of the figure shows the original MOF seed, while the right-hand side displays the MOF grown as a secondary layer. This demonstrates how two or more MOF units are combined to create a new hybrid material.186 This approach results in unique properties that can be customized for specific applications. This method enables the distinct properties of different MOFs to be combined, such as high porosity, specific functionality or catalytic activity, and can also improve the stability and mechanical properties of the resulting material.187
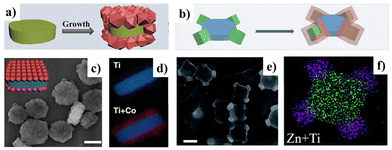 |
| Fig. 6 (a) Scheme of a binary MOF-on-MOF heterostructure (reproduced from ref. 157; Copyright © 1999–2024 John Wiley & Sons, Inc. or related companies); (b) scheme of a ternary MOF-on-MOF heterostructure (ref. 188, reprinted with permission as indicated in the Terms and Conditions of the license); (c) SEM image of MOF MIL-125@ZIF-67 (ref. 188, reprinted with permission as indicated in the Terms and Conditions of the license); (d) element mapping image of MIL-125@ZIF-67 (reproduced from ref. 188, reprinted with permission as indicated in the Terms and Conditions of the license); (e) SEM image of MIL-125@ZIF-8 (reproduced from ref. 189 with permission from the Royal Society of Chemistry); (f) elemental mapping image of MIL-125@ZIF-8 (reproduced from ref. 189 with permission from the Royal Society of Chemistry). | |
In general, there are two main approaches for synthesizing MOF-on-MOF structures. The first approach involves a two-step process, with pre-synthesized host MOFs used as seeds in the first step, and guest MOFs are grown on them in the second step to form MOF-on-MOF structures. The second approach involves a one-pot process that controls the nucleation and growth kinetics of both host and guest MOFs.11
The growth of guest MOFs can occur via five mechanisms, namely: epitaxial, heteroepitaxial, surfactant-assisted, nucleation kinetic-guided, and ligand/metal ion-exchange growth.11,190 However, current MOF-on-MOF systems mainly focus on compositions consisting of two or more components188 (Fig. 6a and b). For example, Ikigaki et al. reported a ternary MOF-on-MOF copper structure,191 composed of an oriented MOF-on-MOF film, with lower and upper MOF layers. The films were created by epitaxially matching the interface and “one-pot” and liquid-phase epitaxy methods for the layers with copper-based reactants.
To assess the interaction between MOFs involved in MOF-on-MOF hybrids, their structures can be studied using SEM images, and element mapping enables their structures to be investigated through morphology analysis of two or more MOF varieties (see Fig. 6c–f). By integrating the aforementioned properties of various MOFs into MOF-on-MOF systems, it is possible to control gas adsorption capacity and selectivity while improving adsorbent stability.11
Li et al. developed a MOF-on-MOF structure specifically designed for gas adsorption. The structure comprised a core composed of a mixture of bio-MOF-11 and bio-MOF-14, while a bio-MOF-14 shell encapsulated the core. Water stability testing confirmed that the hydrophobic bio-MOF-14 shell protected the water-sensitive core from degradation. The resulting MOF-on-MOF hybrids displayed selective gas storage properties and improved water stability.192
Panchariya et al. synthesized two variants of zeolitic imidazolate frameworks (ZIFs), the core–shell ZIF-8@ZIF-67 and the ZIF-67@ZIF-8. Both exhibited high H2 storage values, reaching 2.03 wt% for ZIF-8@ZIF-67 and 1.69 wt% for ZIF-67@ZIF-8. Under −196 °C and 1 bar conditions, the H2 storage capacity of the hybrids improved by 41% and 18% compared with their ZIF-67 and ZIF-8 parents, respectively. According to the authors, these values of H2 uptake indicate that the unique structural features of the core–shell materials, such as the confinement of a porous structure within another one and their elemental heterogeneity, were the main reasons. The best material in terms of H2 storage, the core–shell ZIF-8@ZIF-67, had an ABET of 1402 m2 g−1, higher than that of ZIF-8 with 1324 m2 g−1 and ZIF-67 with 1392 m2 g−1. The authors suggested that this increase was due to the well-developed ZIF-67 shell over the ZIF-8 core, which did not block the ZIF-8 pores. Nevertheless, the core–shell ZIF-67@ZIF-8 was the second-best performing and had a lower ABET than its ZIF parents, 1272 m2 g−1, indicating that the most important variables were the distinct surface and porosity characteristics of the core–shell topologies.180 The results underscore the potential of MOF-on-MOF systems for efficient gas storage applications.
4.2. MOF hybrids with carbon-based materials or metals
The exceptional porosity and versatility of MOFs enable the synthesis of hybrids with a variety of materials, including polymers, graphene oxide, carbon nanotubes, metal nanoparticles, etc.170,193 This can be achieved by growing MOFs on the surface or in the pores of other substrates or by incorporating additional materials into MOF cavities. As a result, hybrids can exhibit a combination of the properties of both components or even acquire new functionalities, such as water stability, or even improved mechanical properties.194,195
Fig. 7 shows the various possible synthesis approaches for obtaining a MOF hybrid, including covalent modification at metal nodes (Fig. 7a) or organic ligands (Fig. 7b), encapsulation (Fig. 7c), layer-by-layer deposition (Fig. 7d), or nucleation in the presence of other materials (Fig. 7e).196
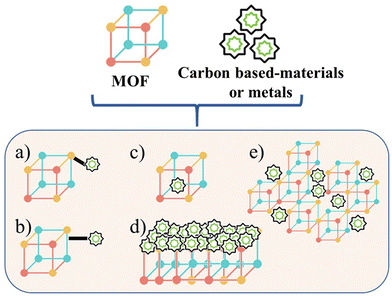 |
| Fig. 7 Scheme of hybrid materials based on MOFs and carbons or metals: (a) covalent modification at metal nodes; (b) covalent modification at organic ligands; (c) encapsulation; (d) layer-by-layer deposition; and (e) nucleation in the presence of other materials (adapted from ref. 196). | |
MOF hybrids can be synthesized by various methods, such as in situ growth, post-synthetic modification and physical mixing.180,197,198
- In situ growth: In this approach, carbon-based materials or metals are incorporated into the MOF structure during MOF synthesis. These materials can therefore serve as nucleation sites for MOF crystal growth, and their presence can influence the morphology, structure and properties of the resulting MOF hybrid. The designation for this approach is MOF@A, with “A” being another material that forms a non-covalent interaction between the two components. Alfe et al. used graphene-like (GL) materials to prepare HKUST-1@GL, MIL-96(Al)@GL and MIL-101(Fe)@GL by in situ growth. The materials obtained achieved good selectivity for CO2 over CH4 using MOF@GL hybrids.199 Pt and Au nanoparticles were used as nucleation sites during ZIF-8 synthesis, enabling Pt and Au nanoparticles to be encapsulated.200 He et al. synthesized MOF-5@Au201 and Liu et al. synthesized UIO-66@Pt using a one-pot method in which the nanoparticles and MOFs were synthesized simultaneously.202
- Post-synthetic modification: In this approach, pre-synthesized MOFs are modified by introducing carbon-based materials or metals onto the MOF surface or into its pores once MOF synthesis has been completed. Post-synthetic modification methods allow other materials with specific properties or functionalities to be introduced into MOFs without altering the MOF structure.203
Covalent modifications encompass both coordinated covalent modifications of metal clusters and covalent modifications of ligands. By employing covalent modification techniques, it is possible to synthesize MOF hybrids with small molecules, metals/metal clusters, covalent organic frameworks (COFs), polymers and graphene.196,203 Rao et al. developed a composite membrane combining graphene oxide (GO) and UiO-66-NH2 by tethering UiO-66-NH2 to GO surfaces. The covalent attachment of UiO-66-NH2 with GO enabled the creation of a continuous proton transfer channel, contributing to the high performance of the composite membrane.215
Post-synthetic modification also includes techniques such as coating, layering and other methods that do not rely on the formation of covalent bonds. For instance, Chen et al. developed a material comprising perovskite quantum dots (QDs) encapsulated in HKUST-1 by immersing HKUST-1 thin films in solutions containing QD precursors. QDs of 1.5–2 nm matched the pore size of HKUST-1 and the resulting material improved its stability under 70% relative humidity, whereas QDs alone decomposed under the same conditions.216 Villajos et al. obtained a ZIF-8@Pd hybrid by synthesizing Pd nanoparticles in the presence of commercial ZIF-8212 and Hu et al. followed a similar route to obtain HKUST-1@Pd.209
- Physical mixing: This approach involves simply mixing MOFs and carbon-based or inorganic materials without any covalent or coordination bonds between them. This approach is relatively simple and straightforward but may result in phase separation or weak interactions between MOFs and other materials. Otal et al. stated that the inherent characteristics of MOFs, including crystallinity, structure and porosity, were maintained even after the modification of pre-synthesized MOFs.217 Some studies confirm a significant improvement in H2 sorption capacities when MOFs are mixed with carbon-based materials.163,218 Prasanth et al. clearly demonstrated a substantial improvement in the H2 sorption capacities of MIL-101 samples through the incorporation of Single-Walled Carbon Nanotubes (SWCNTs). H2 sorption capacities increased from 6.4 to 9.2 wt% at −196 °C and 6 MPa and from 0.2 to 0.6 wt% at 25 °C and 6 MPa.218 In their feature article, Szczęśniak et al. studied the incorporation of GO into different MOF matrices such as HKUST-1 and MIL-101(Cr). They observed that the presence of GO can significantly increase their surface area and influence their morphology and structure. The dense atomic structure of GO contributes to stronger dispersion interactions and a greater number of unsaturated coordination bonds when combined with MOFs, which is crucial for improving adsorption properties.163
4.2.1. MOF hybrids with metal particles and ions for hydrogen storage. One of the most attractive structural features of some MOFs is coordinative unsaturation, which can be achieved by introducing additional terminal ligands bound to metal clusters.219 Fig. 8 shows the influence of ion and metal particle doping on H2 adsorption. The addition of ions or nanoparticles often reduces the SSA of the hybrid, calling into question the reliability of using the BET area as an indicator of H2 storage capacities in MOFs and MOF-derived materials. However, all samples show an increase in H2 adsorption, especially at room temperature (25 °C). To improve H2 storage, it is thus crucial to design novel MOF materials that not only have customized pore sizes and substantial void volumes, but also contain highly efficient adsorption sites. Among these sites are exposed metal ions, whose binding enthalpy lies between −20 and −30 kJ mol−1.161
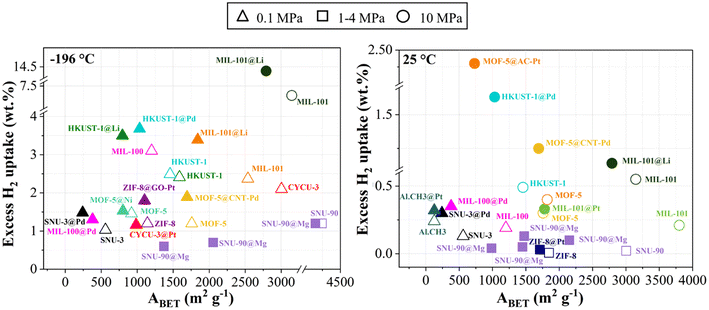 |
| Fig. 8 Influence of adding metal particles into MOFs on the H2 storage capacities of the resultant hybrid materials ( ref. 204); ( , ref. 205); ( ref. 206); ( , ref. 207); ( , ref. 90); ( ref. 208); ( , ref. 209); ( ref. 210); ( ref. 162); ( ref. 211); ( ref. 212); ( ref. 213); ( ref. 214). | |
- Metal ion doping of MOFs: Metal ions can be introduced into the MOF matrix by ion exchange procedures as well as by chemical reduction methods, with the aim of increasing the adsorption enthalpy. Peedikakkal et al. showed that metal-exchanged MOF-5@Ni and MOF-5@Co exhibit slightly higher H2 uptake (about 5 wt%) compared with the parent MOF-5.90 Adsorption results by Prabhakaran and Deschamps revealed a substantial increase in the H2 uptake capacity of MIL-101 thanks to the synergistic modification involving activated carbon and lithium doping (at both −196 °C and 25 °C). The H2 storage capacity (−196 °C, 1 bar) of the doped MOF (MIL-101@AC-Li) showed an H2 uptake of 1.60 wt% compared with 1.20 wt% in undoped MIL-101.204 Prabhakaran et al. demonstrated that Li+ doping (830 ppm) of MIL-101@SWCNTs improved H2 uptake by more than twofold (4.96–10.43 mg g−1) at 25 °C and 90 bar.160
- Metal nanoparticles on MOFs: The synthesis of MOF hybrids with metal nanoparticles involves incorporating the latter into the MOF structure using template methods or pre-synthesis strategies. This introduction effectively increases the number of adsorption sites, boosts adsorption enthalpy and enables synergistic physical–chemical H2 adsorption. In particular, MOF@Pd hybrids have received considerable attention because Pd can absorb large amounts of H2 at ambient temperature and pressure. Cheon et al. reported that H2 sorption capacity was increased to 1.48 wt% at −196 °C and 1 atm, compared to 1.03 wt% for pure SNU-3 MOF (despite a 43% decrease in the surface area).162 Zlotea et al. showed that the MIL-100(Al)@Pd hybrid doubled H2 uptake compared with the original MOF at room temperature.206 Yang et al. loaded Pt into MOF-5@CNT hybrids to further increase H2 storage capacity. The hybrid was prepared by in situ incorporation of the as-prepared Pt-loaded MWCNTs into MOF-5 crystals. Four materials were evaluated in terms of H2 uptake: MWCNTs (ABET = 147 m2 g−1), MWCNTs-Pt (ABET = 124 m2 g−1), MOF-5 (ABET = 1758 m2 g−1) and MOF@MWCNT-Pt (ABET = 1692 m2 g−1). The authors studied H2 uptake at −196 °C and 1 bar, as well as 25 °C and 100 bar. The highest H2 uptake (−196 °C and 1 bar) was observed for MOF@MWCNT-Pt with 1.89 wt%, followed by MOF-5 with 1.20 wt%. At 25 °C and 100 bar, MOF@MWCNT-Pt showed much higher H2 uptake values compared to the other materials, reaching 1.25 wt%.207
Prabhakaran and Deschamps synthesized a hybrid MOF by incorporating an AC, namely NORIT-RB3, during the synthesis of MIL-101, as well as lithium at different concentrations. H2 adsorption capacities were evaluated at −196 °C and 25 °C, using pressures up to 100 bar. The highest H2 uptakes were obtained for the MIL-101-B@AC-Li material, with 14.4 wt% at −196 °C and 1.1 wt% at 25 °C, both at 100 bars. Although the ABET of the MIL-101-B@AC-Li material was much lower, 2791 m2 g−1 than those of MIL-101, 3148 m2 g−1, and MIL-101@AC, 3458 m2 g−1, and slightly lower than that of MIL-101-A@AC-Li, 2958 m2 g−1, all of these hybrids provided H2 uptake capacities >100 mg g−1 (10 wt%) at −196 °C. However, the significantly lower ABET value of MIL-101-C@AC-Li (1868 m2 g−1) compromised higher H2 uptakes, reaching a maximum of 87.70 mg g−1 at −196 °C.204
4.2.2. MOF hybrids with carbon materials for hydrogen storage. In this section, we examine how the addition of carbon materials improves the properties relevant to H2 storage described in section 3.- Increase in surface area: The addition of carbon-based materials to the MOF matrix often increases the SSA of the resulting MOF hybrid, enhancing its adsorption capacity at cryogenic and near-ambient temperatures.199,204,220–222,226,227 Fig. 9 shows the effect of the BET area on H2 adsorption for pure MOFs and for MOF hybrids with carbon-based materials at different storage pressures.
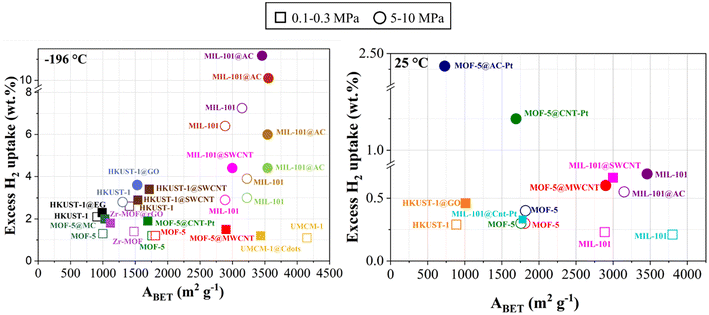 |
| Fig. 9 Excess H2 uptake of MOF hybrids reported in recent studies at −196 °C (left) and 25 °C (right) ( ref. 220); ( ref. 221); ( ref. 222);( ref. 223); ( , ref. 131); ( , ref. 207); ( ref. 175); ( ref. 224); ( ref. 214); ( , ref. 158); ( , ref. 218); ( , ref. 159); ( ref. 204); ( ref. 225). | |
Yang et al. prepared MOF-5@MWCNT hybrids using acid-treated MWCNTs and MOF-5. The resultant materials exhibited a higher Langmuir specific surface area, from 2160 to 3550 m2 g−1, resulting in an approximately 50% increase in H2 storage capacity at 25 °C and 95 bar (from 0.3 to 0.6 wt%) and a 25% increase in H2 storage capacity at −196 °C and 1 bar (from 1.2 to 1.5 wt%). Furthermore, these hybrids demonstrated significantly improved stability in the presence of ambient moisture.131 Petit et al. synthesized copper-based MOF@graphite oxide hybrids, and four materials were evaluated for H2 adsorption: HKUST-1, HKUST-1@GO (5 wt%), HKUST-1@GO (9 wt%), and HKUST-1@GO (18 wt%). ABET and H2 uptake were 990 m2 g−1 and 2.2 wt% for HKUST-1, 989 m2 g−1 and 2.4 wt% for HKUST-1@GO (5 wt%), 1002 m2 g−1 and 2.2 wt% for HKUST-1@GO (9 wt%), and 996 m2 g−1 and 2.0 wt% for HKUST-1@GO (18 wt%), respectively. The authors attributed the improved uptake to the formation of new small pores for the hybrids.221
Prasanth et al. incorporated SWCNTs into MIL-101, and the best-performing material, incorporating 8 wt% of SWCNTs, presented H2 sorption capacities of 9.2 wt% (at −196 °C and 6 MPa) and 0.6 wt% (at 25 °C and 6 MPa) compared with 6.4 wt% and 0.2 wt%, respectively, for the pristine MIL-101. Although this material has a slightly lower SSA, the authors ascribed these higher H2 sorption capacities to the reduction in pore size and the increase in micropore volume in the hybrid due to the incorporation of SWCNTs.218 Liu et al. hybridized Cu-MOFs with graphene oxide (GO) and achieved about 30% higher H2 capacities at −196 °C and 4.2 MPa due to the increased SSA.220 Rallapalli et al. introduced activated carbon (AC) during the synthesis of MIL-101(Cr), and the best-performing material reached an ABET of 3556 m2 g−1 and an H2 uptake of 10.1 wt% at −196 °C and 6 MPa, outperforming pristine MIL-101 (ABET = 2887 m2 g−1 and 6.4 wt% H2 uptake under the same conditions159). Li et al. used carbon nanodots (CDs) to prepare UMCM-1@CD materials and the best H2 uptake was 1.2 wt% at −196 °C and 0.1 MPa, slightly higher than that of UMCM-1 with 1.1 wt%. The authors suggested that the improvement was due to specific interactions between polar functional groups, e.g. –COOH and –OH, on the CD surface and H2 molecules.225 Yu et al. investigated MIL-101(Cr) and a MIL-101(Cr)@AC hybrid that displayed a maximum excess H2 uptake (at −196 °C and 10 MPa) of 8.2 and 13.5 wt%, respectively, due to an increase in ABET from 3407 for the pristine MOF to 3542 m2 g−1 for the hybrid.158
The morphology, not just the texture, of the MOF hybrid often changes with the percentage of carbon-based material added. Fig. 10a and b show the HKUST-1@GO hybrid modified by increasing concentrations of GO from 1 to 2.5 g L−1. The morphology of both samples changed significantly on reaching the highest GO concentration tested. In addition to the typical polyhedral crystals, distinctive rod- and flower-like structures appeared. Fig. 10c and d show the characteristic morphology of the Co-bpdc and Co-bpdc@MWCNT hybrids. In Fig. 10c, the Co-bpdc crystal exhibits a rectangular structure and Fig. 10d clearly shows the successful synthesis of a hybrid of MWCNTs with Co-bpdc.
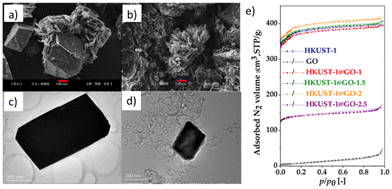 |
| Fig. 10 (a) SEM images of HKUST-1@1g L−1 GO; (b) SEM images of HKUST-1@2.5 g L−1 GO. Scale bar is 10 μm (reproduced from ref. 228 © 1996–2024 MDPI); (c) TEM images of Co-bpdc (cobalt benzene-1,4-dicarboxylate) as a pure MOF; (d) TEM images of Co-bpdc@MWCNTs as a hybrid (reproduced from ref. 229 © 1996–2024 MDPI); (e) N2 adsorption–desorption isotherms (−196 °C) of pristine GO, HKUST-1 and hybrid materials with different GO contents (reproduced from ref. 228 © 1996–2024 MDPI). | |
Although GO had no measurable SSA by N2 adsorption at −196 °C, the total SSA of the HKUST-1@GO hybrid remained constant or even increased with the addition of GO up to a GO concentration in solution of 2.5 g L−1 (see Fig. 10e).228
- Increase in thermal stability: The presence of a carbon-based material can also increase the thermal stability of the hybrid.175,222 Policicchio et al. provided an overview of the thermal stability of hybrids obtained after the incorporation of nanographite (nGr) during the synthesis of UiO-66. In addition to a notable increase in material porosity (∼30%), linker decomposition in all hybrids occurred at a temperature of around 80 °C higher than that of the pure MOF, probably due to changes in heat capacity caused by the introduction of nGr.9
Jiang et al. studied the influence of introducing MWCNTs into the MOF-5 matrix, obtaining a hybrid that showed a 6% increase in thermal stability.175 Rojas-Garcia et al. showed that HKUST-1@SWCNT materials exhibit improved thermal stability (320 °C) compared to pristine HKUST-1 (220 °C) using an InVia Renishaw Raman instrument equipped with a Linkam cell.222
- Increase in hydrophobicity: Kim et al. used carbon black to fabricate a hybrid with MOF-5 and demonstrated that it did not affect the integrity of the MOF-5 and effectively protected the framework from moisture.230 For the same MOF, Yang and Park reported that an amorphous carbon-coated MOF-5 could be prepared by a simple thermal modification process carried out under an N2 atmosphere in a temperature range of 480–530 °C. Amorphous carbon-coated MOF-5 samples were found to have reduced BET areas (1740 vs. 3450 m2 g−1), but displayed better water stability compared with pristine MOF-5.231 Jayaramulu et al. obtained a hybrid material using a highly fluorinated graphene oxide (HFG) and ZIF-8 (ZIF-8@HFG). The material exhibited superhydrophobic behavior with an exceptionally high water contact angle of 162°.232
- Increase in thermal conductivity: The addition of carbon-based materials to a MOF boosts its thermal conductivity, making it more efficient at conducting and dissipating heat. The degree of improvement depends on factors such as the filler type, concentration and dispersion. Nandasiri et al. reported an ∼23% improvement in thermal conductivity by adding GO to the MOF matrix MIL-101(Cr).233 The addition of 10 wt% expanded natural graphite to MOF-5 pellets with a density of 0.5 g cm−3 was previously found to improve thermal conduction near room temperature by a factor of 5 (compared with undoped MOF-5 pellets).234 A similar behavior was observed by Farrando-Pérez et al. for the HKUST-1@graphite flake hybrid, showing that the thermal conductivity of the hybrid monolith (50 wt% graphite flakes) was 3.15 W m−1 K−1 vs. 0.49 W m−1 K−1 for the pure HKUST-1 monolith. In terms of H2 uptake, the hybrid monolith with 10 wt% graphite flakes showed the best result with 1.7 wt% vs. 1.25 wt% for the pure HKUST-1 monolith.143
4.2.3. MOF hybrids with other inorganic materials. Hybrids with MOFs have been used for various applications, such as CO2 capture, and can include alternative materials such as zeolites, cellulose and clays.235 MOFs@clay hybrids include feedstock materials such as attapulgite, amino-clay, cordierite, kaolin and bentonite. Wang et al. studied the influence of introducing diatomite (Da) into the MIL-101 (Cr) matrix. Their study evaluated the H2 storage capacity of the Cr-MOF@Da hybrid at 25 °C and 1 bar. The H2 adsorption isotherms showed a linear increase as the wt% of diatomite increased, obtaining 0.022 wt% for Cr-MIL-101@Da-4. The hybrids also showed improved thermal stability.236MOF@clays have been the subject of research interest, especially for the development of complex geometries of MOFs using 3D printing.237 MOFs@zeolite hybrids combine the high SSA of MOFs with the high mechanical strength of zeolites. By selecting the type of synthesis, a core–shell structure can be obtained that may offer certain advantages, such as gas separation, hydrophobicity, etc.238 For instance, Musyoka et al. fabricated a MIL-101(Cr)@zeolite-templated carbon (ZTC) hybrid for H2 storage applications. H2 adsorption measurements were carried out at −196 °C up to 1 bar. The hybrid material was compared with the individual materials and showed an increased surface area and H2 uptake capacity of 2957 m2 g−1 and 2.55 wt%, respectively. These results are significantly better than those obtained with MIL-101 (2552 m g−1, 2.39 wt%) and ZTC (2577 m2 g−1, 2.39 wt%).239
5. Conclusions
The development of MOF-based hybrid adsorbents, combining MOFs with a variety of other materials and nanostructures, has attracted considerable interest in recent times. This approach aims to improve the performance and scalability of MOFs, opening up possibilities for future materials.
MOFs alone, due to their versatile structures, high surface areas and substantial pore volumes, are already considered promising materials for H2 storage, as evidenced by NU100, which reaches 9.05 wt% at 7 MPa and −196 °C. Moreover, a variety of synthesis methods enables the nanopore structure of MOFs to be controlled, which is essential for their applicability in adsorption. Our review covered different synthesis techniques, delineating their respective advantages and drawbacks in controlling MOF parameters, as well as characterizing MOFs and their hybrids. By recognizing the potential of MOFs, increasing the SSA and optimizing pore size distribution should make it possible to increase H2 storage capacities in line with the DOE specifications.
To optimize hydrogen storage under ambient conditions, strategies such as linker functionalization and the introduction of unsaturated metal centers are highly effective. A notable example is that of exposed metal ions, which often have binding enthalpies ranging from −20 to −30 kJ mol−1. Other advances concern the creation of competitive MOF hybrids, especially through the incorporation of carbon materials, which show promise in increasing adsorption capacity, improving thermal stability and enhancing thermal conductivity. To improve the selectivity and stability of MOFs, MOF@MOF hybrids offer a promising approach. By integrating the characteristics of two distinct MOFs into a single, unified material, this method effectively combines their advantages. Combinations such as MOF@carbon-based materials preserve the advantages of MOFs while improving thermal conductivity, surface area and ease of shaping.
In conclusion, MOF-based hybrids represent a promising approach for improving H2 storage capabilities, offering significant potential to meet the demanding standards set by the US DOE. Key areas of future research should focus on increasing the volumetric capacity of these materials, as their gravimetric performance is already outstanding, but volumetric improvements are essential for practical applications such as transportation and portable energy systems. These developments will play a decisive role in optimizing the performance of MOF hybrids and advancing their viability for commercial hydrogen storage applications.
Abbreviation
(–ΔH)ads | Adsorption enthalpy |
ABET | Brunauer–Emmett–Teller area |
BDC | 1,4-Benzenedicarboxylic acid |
BET | Brunauer–Emmett–Teller |
BPDC | Biphenyldicarboxylate |
BTC | Benzene-1,3,5-Tricarboxylic acid |
CDs | Carbon dots |
Co-bpdc | Cobalt benzene-1,4-dicarboxylate |
COFs | Covalent organic frameworks |
DMF | N,N-Dimethylformamide |
DOE | U.S. Department of Energy |
FTIR | Fourier transform infrared spectroscopy |
GL | Graphene-like |
GO | Graphene oxide |
H2 | Hydrogen |
ILs | Ionic liquids |
IMOFs | Interpenetrated MOFs |
MIL | Materials of Institut Lavoiser |
MOFs | Metal organic frameworks |
MWCNTs | Multi-walled carbon nanotubes |
nGr | Nano graphite |
NMR | Nuclear magnetic resonance |
NU | Northewestern University |
PBUs | Primary building units |
PCPs | Porous coordination polymers |
PDC | 3,5-Pyridine dicarboxylic acid |
PSD | Pore size distribution |
PTFE | Polytetrafluoroethylene |
QDs | Quantum dots |
RAM | Resonant acoustic mixing |
SBUs | Secondary building units |
SEM | Scanning electron microscopy |
SSA | Specific surface area |
SWCNT | Single-walled carbon nanotubes |
TGA | Thermogravimetric analysis |
UIO | Universitetet i Oslo |
XRD | X-ray diffraction analysis |
ZTC | Zeolite-templated carbon |
Author contributions
L.J.-L.: writing – original draft and formal analysis; R.M.-O.: review & editing, formal analysis, and supervision; L.G. A.: writing – original draft and formal analysis; A.C.: review & editing and supervision; V.F. review & editing, supervision, project administration, and formal analysis.
Data availability
The data supporting this article have been included as part of the ESI.†
No primary research results, software or code have been included and no new data were generated or analysed as part of this review.
Conflicts of interest
There are no conflicts to declare.
Acknowledgements
This work was made possible thanks to (i) the SOLHYD project (ANR-22-PEHY-0007) funded by the Agence Nationale de la Recherche, (ii) the FRCR HyPE project funded by Région Grand-Est, and (iii) TALiSMAN and TALiSMAN2 projects funded by FEDER. De Araujo, L. G. acknowledges the MOPGA program, funded by the French Ministry of Europe and Foreign Affairs, in collaboration with the French Ministry of Higher Education and Research.
References
- E. Ilisca, Microporous Materials for Hydrogen Liquefiers and Storage Vessels, J. Mater. Sci. Lett., 2022, 3, 1–10 Search PubMed.
- M. Ball and M. Wietschel, The Hydrogen Economy: Opportunities and Challenges, Cambridge University Press, 2009, pp. 671 Search PubMed.
- D. J. Durbin and C. Malardier-Jugroot, Review of hydrogen storage techniques for on board vehicle applications, Int. J. Hydrogen Energy, 2013, 38(34), 14595–14617 CrossRef CAS.
- S. Evro, B. A. Oni and O. S. Tomomewo, Carbon neutrality and hydrogen energy systems, Int. J. Hydrogen Energy, 2024, 78, 1449–1467 CrossRef CAS.
- G. Squadrito, G. Maggio and A. Nicita, The green hydrogen revolution, Renewable Energy, 2023, 216, 119041 CrossRef CAS.
- R. Morales-Ospino, L. Jiménez-López, A. Celzard and V. Fierro, Hydrogen – Storage | Physical storage, in Reference Module in Chemistry, Molecular Sciences and Chemical Engineering [Internet], Elsevier, 2024. Available from: https://www.sciencedirect.com/science/article/pii/B9780323960229002905 [cited 2024 Jun 27] Search PubMed.
- S. Bordiga, L. Regli, F. Bonino, E. Groppo, C. Lamberti and B. Xiao, et al., Adsorption properties of HKUST-1 toward hydrogen and other small molecules monitored by IR, Phys. Chem. Chem. Phys., 2007, 9(21), 2676–2685 RSC.
- O. K. Farha, A. Özgür Yazaydın, I. Eryazici, C. D. Malliakas, B. G. Hauser and M. G. Kanatzidis, et al., De novo synthesis of a metal–organic framework material featuring ultrahigh surface area and gas storage capacities, Nat. Chem., 2010, 2(11), 944–948 CrossRef CAS PubMed.
- A. Policicchio, M. Florent, A. Celzard, V. Fierro, J. Jagiello and T. J. Bandosz, Enhancing the gas adsorption capacities of UiO-66 by nanographite addition, Microporous Mesoporous Mater., 2020, 309, 110571 CrossRef CAS.
- M. Hasanzadeh, A. Simchi and H. Shahriyari Far, Nanoporous composites of activated carbon-metal organic frameworks for organic dye adsorption: Synthesis, adsorption mechanism and kinetics studies, J. Ind. Eng. Chem., 2020, 81, 405–414 CrossRef CAS.
- C. Liu, J. Wang, J. Wan and C. Yu, MOF-on-MOF hybrids: Synthesis and applications, Coord. Chem. Rev., 2021, 432, 213743 CrossRef CAS.
- M. Jouyandeh, F. Tikhani, M. Shabanian, F. Movahedi, S. Moghari and V. Akbari, et al. Synthesis, characterization, and high potential of 3D metal–organic framework (MOF) nanoparticles for curing with epoxy, J. Alloys Compd., 2020, 829, 154547 CrossRef CAS.
- A. Schoedel and S. Rajeh, Why Design Matters: From Decorated Metal Oxide Clusters to Functional Metal–Organic Frameworks, Top. Curr. Chem., 2020, 378(1), 19 CrossRef CAS PubMed.
- X. W. Liu, T. J. Sun, J. L. Hu and S. D. Wang, Composites of metal–organic frameworks and carbon-based materials: preparations, functionalities and applications, J. Mater. Chem. A, 2016, 4(10), 3584–3616 RSC.
- F. A. A. Paz, J. Klinowski, S. M. F. Vilela, J. P. C. Tomé, J. A. S. Cavaleiro and J. Rocha, Ligand design for functional metal–organic frameworks, Chem. Soc. Rev., 2012, 41(3), 1088–1110 RSC.
- S. Soni, P. Bajpai and C. Arora, A review on metal-organic framework: synthesis, properties and application, Charact. Appl. Nanomater., 2018, 2, 87–106 Search PubMed.
- S. Kitagawa, R. Kitaura and S. I. Noro, Functional Porous Coordination Polymers, Angew. Chem., Int. Ed., 2004, 43(18), 2334–2375 CrossRef CAS PubMed.
- P. Rocío-Bautista, I. Taima-Mancera, J. Pasán and V. Pino, Metal-Organic Frameworks in Green Analytical Chemistry, Separations, 2019, 6(3), 33 CrossRef.
- S. P. Shet, S. Shanmuga Priya, K. Sudhakar and M. Tahir, A review on current trends
in potential use of metal-organic framework for hydrogen storage, Int. J. Hydrogen Energy, 2021, 46(21), 11782–11803 CrossRef CAS.
- J. L. C. Rowsell and O. M. Yaghi, Metal–organic frameworks: a new class of porous materials, Microporous Mesoporous Mater., 2004, 73(1), 3–14 CrossRef CAS.
- N. Stock and S. Biswas, Synthesis of Metal-Organic Frameworks (MOFs): Routes to Various MOF Topologies, Morphologies, and Composites, Chem. Rev., 2012, 112(2), 933–969 CrossRef CAS PubMed.
- D. Sud and G. Kaur, A comprehensive review on synthetic approaches for metal-organic frameworks: From traditional solvothermal to greener protocols, Polyhedron, 2021, 193, 114897 CrossRef CAS.
- Z. Zheng, H. L. Nguyen, N. Hanikel, K. K. Y. Li, Z. Zhou and T. Ma, et al., High-yield, green and scalable methods for producing MOF-303 for water harvesting from desert air, Nat. Protoc., 2023, 18(1), 136–156 CrossRef CAS PubMed.
- D. J. Tranchemontagne, J. R. Hunt and O. M. Yaghi, Room temperature synthesis of metal-organic frameworks: MOF-5, MOF-74, MOF-177, MOF-199, and IRMOF-0, Tetrahedron, 2008, 64(36), 8553–8557 CrossRef CAS.
- M. Sánchez-Sánchez, N. Getachew, K. Díaz, M. Díaz-García and Y. Chebude, Díaz I. Synthesis of metal–organic frameworks in water at room temperature: salts as linker sources, Green Chem., 2015, 17(3), 1500–1509 RSC.
- N. Getachew, Y. Chebude, I. Diaz and M. Sanchez-Sanchez, Room temperature synthesis of metal organic framework MOF-2, J. Porous Mater., 2014, 21(5), 769–773 CrossRef CAS.
- S. Proch, J. Herrmannsdörfer, R. Kempe, C. Kern, A. Jess and L. Seyfarth, et al., Pt@MOF-177: Synthesis, Room-Temperature Hydrogen Storage and Oxidation Catalysis, Chem. – Eur. J., 2008, 14(27), 8204–8212 CrossRef CAS PubMed.
- M. Ebrahimi and M. Mansournia, Rapid room temperature synthesis of zeolitic imidazolate framework-7 (ZIF-7) microcrystals, Mater. Lett., 2017, 189, 243–247 CrossRef CAS.
- E. Biemmi, S. Christian, N. Stock and T. Bein, High-throughput screening of synthesis parameters in the formation of the metal-organic frameworks MOF-5 and HKUST-1, Microporous Mesoporous Mater., 2009, 117(1), 111–117 CrossRef CAS.
- W. W. Lestari, M. Adreane, C. Purnawan, H. Fansuri, N. Widiastuti and S. B. Rahardjo, Solvothermal and electrochemical synthetic method of HKUST-1 and its methane storage capacity, IOP Conf. Ser.:Mater. Sci. Eng., 2016, 107(1), 012030 Search PubMed.
- N. Pokhrel, P. K. Vabbina and N. Pala, Sonochemistry: Science and Engineering, Ultrason. Sonochem., 2016, 29, 104–128 CrossRef CAS PubMed.
- S. Głowniak, B. Szczęśniak, J. Choma and M. Jaroniec, Recent Developments in Sonochemical Synthesis of Nanoporous Materials, Molecules, 2023, 28(6), 2639 CrossRef PubMed.
- L. G. Qiu, Z. Q. Li, Y. Wu, W. Wang, T. Xu and X. Jiang, Facile synthesis of nanocrystals of a microporous metal–organic framework by an ultrasonic method and selective sensing of organoamines, Chem. Commun., 2008,(31), 3642–3644 RSC.
- C. Vaitsis, G. Sourkouni and C. Argirusis, Metal Organic Frameworks (MOFs) and ultrasound: A review, Ultrason. Sonochem., 2019, 52, 106–119 CrossRef CAS PubMed.
- H. M. Yang, X. Liu, X. L. Song, T. L. Yang, Z. H. Liang and C. M. Fan, In situ electrochemical synthesis of MOF-5 and its application in improving photocatalytic activity of BiOBr, Trans. Nonferrous Met. Soc. China, 2015, 25(12), 3987–3994 CrossRef CAS.
- S. Kalhor, M. Zarei, M. A. Zolfigol, H. Sepehrmansourie, D. Nematollahi and S. Alizadeh, et al. Anodic electrosynthesis of MIL-53(Al)-N(CH2PO3H2)2 as a mesoporous catalyst for synthesis of novel (N-methyl-pyrrol)-pyrazolo[3,4-b]pyridines via a cooperative vinylogous anomeric based oxidation, Sci. Rep., 2021, 11(1), 19370 CrossRef CAS PubMed.
- A. Martinez Joaristi, J. Juan-Alcañiz, P. Serra-Crespo, F. Kapteijn and J. Gascon, Electrochemical Synthesis of Some Archetypical Zn2+, Cu2+, and Al3+ Metal Organic Frameworks, Cryst. Growth Des., 2012, 12(7), 3489–3498 CrossRef CAS.
- S. Yadnum, J. Roche, E. Lebraud, P. Négrier, P. Garrigue and D. Bradshaw, et al., Site-Selective Synthesis of Janus-type Metal-Organic Framework Composites, Angew. Chem., Int. Ed., 2014, 53(15), 4001–4005 CrossRef CAS PubMed.
- V. Vm and G. Nageswaran, Review—Direct Electrochemical Synthesis of Metal Organic Frameworks, J. Electrochem. Soc., 2020, 167(15), 155527 CrossRef.
- A. M. Antonio, J. Rosenthal and E. D. Bloch, Electrochemically Mediated Syntheses of Titanium(III)-Based Metal–Organic Frameworks, J. Am. Chem. Soc., 2019, 141(29), 11383–11387 CrossRef CAS PubMed.
- M. Hartmann, S. Kunz, D. Himsl, O. Tangermann, S. Ernst and A. Wagener, Adsorptive Separation of Isobutene and Isobutane on Cu3(BTC)2, Langmuir, 2008, 24(16), 8634–8642 CrossRef CAS PubMed.
- P. Kunal and T. J. Toops, A Review of Microwave-Assisted Synthesis-Based Approaches to Reduce Pd-Content in Catalysts, Catalysts, 2020, 10(9), 991 CrossRef CAS.
- G. A. Tompsett, W. C. Conner and K. S. Yngvesson, Microwave Synthesis of Nanoporous Materials, ChemPhysChem, 2006, 7(2), 296–319 CrossRef CAS PubMed.
- Z. Ni and R. I. Masel, Rapid Production of Metal–Organic Frameworks via Microwave-Assisted Solvothermal Synthesis, J. Am. Chem. Soc., 2006, 128(38), 12394–12395 CrossRef CAS PubMed.
- J. Klinowski, F. A. A. Paz, P. Silva and J. Rocha, Microwave-Assisted Synthesis of Metal–Organic Frameworks, Dalton Trans., 2010, 40(2), 321–330 RSC.
- Z. Ni and R. I. Masel, Rapid Production of Metal–Organic Frameworks via Microwave-Assisted Solvothermal Synthesis, J. Am. Chem. Soc., 2006, 128(38), 12394–12395 CrossRef CAS PubMed.
- M. Taddei, P. V. Dau, S. M. Cohen, M. Ranocchiari, J. A. van Bokhoven and F. Costantino, et al., Efficient microwave assisted synthesis of metal–organic framework UiO-66: optimization and scale up, Dalton Trans., 2015, 44(31), 14019–14026 RSC.
- X. Wu, W. Xu, Z. Wang, H. Li, M. Wang and D. Zhang, et al., Rapid microwave synthesis of Ru-supported partially carbonized conductive metal–organic framework for efficient hydrogen evolution, Chem. Eng. J., 2022, 431, 133247 CrossRef CAS.
- S. Wan, O. Xu and X. Zhu, Synthesis of ionic liquid modified metal-organic framework composites and its application in solid-phase extraction: a review, Ionics, 2021, 27(2), 445–456 CrossRef CAS.
- T. P. Vaid, S. P. Kelley and R. D. Rogers, Structure-directing effects of ionic liquids in the ionothermal synthesis of metal–organic frameworks, IUCrJ, 2017, 4(4), 380–392 CrossRef CAS PubMed.
- L. Peng, J. Zhang, J. Li, B. Han, Z. Xue and G. Yang, Surfactant-directed assembly of mesoporous metal–organic framework nanoplates in ionic liquids, Chem. Commun., 2012, 48(69), 8688–8690 RSC.
- C. Liu, G. Zhang, C. Zhao, X. Li, M. Li and H. Na, MOFs synthesized by the ionothermal method addressing the leaching problem of IL–polymer composite membranes, Chem. Commun., 2014, 50(91), 14121–14124 RSC.
- M. Leonardi, M. Villacampa and J. Carlos Menéndez, Multicomponent mechanochemical synthesis, Chem. Sci., 2018, 9(8), 2042–2064 RSC.
- B. Szczęśniak, S. Borysiuk, J. Choma and M. Jaroniec, Mechanochemical synthesis of highly porous materials, Mater. Horiz., 2020, 7(6), 1457–1473 RSC.
- M. Afkhami-Ardekani, M. R. Naimi-Jamal, S. Doaee and S. Rostamnia, Solvent-Free Mechanochemical Preparation of Metal-Organic Framework ZIF-67 Impregnated by Pt Nanoparticles for Water Purification, Catalysts, 2023, 13(1), 9 CrossRef CAS.
- D. Chen, J. Zhao, P. Zhang and S. Dai, Mechanochemical synthesis of metal–organic frameworks, Polyhedron, 2019, 162, 59–64 CrossRef CAS.
- S. Tanaka, Chapter 10 – Mechanochemical synthesis of MOFs, in Metal-Organic Frameworks for Biomedical Applications [Internet], ed. M. Mozafari, Woodhead Publishing, 2020. pp. 197–222. Available from: https://www.sciencedirect.com/science/article/pii/B9780128169841000123 [cited 2023 Aug 29] Search PubMed.
- C. A. Tao and J. F. Wang, Synthesis of Metal Organic Frameworks by Ball-Milling, Crystals, 2021, 11(1), 15 CrossRef CAS.
- H. M. Titi, J. L. Do, A. J. Howarth, K. Nagapudi and T. Friščić, Simple, scalable mechanosynthesis of metal–organic frameworks using liquid-assisted resonant acoustic mixing (LA-RAM), Chem. Sci., 2020, 11(29), 7578–7584 RSC.
- R. Seetharaj, P. V. Vandana, P. Arya and S. Mathew, Dependence of solvents, pH, molar ratio and temperature in tuning metal organic framework architecture, Arabian J. Chem., 2019, 12(3), 295–315 CrossRef CAS.
- A. A. Yakovenko, Z. Wei, M. Wriedt, J. R. Li, G. J. Halder and H. C. Zhou, Study of Guest Molecules in Metal–Organic Frameworks by Powder X-ray Diffraction: Analysis of Difference Envelope Density, Cryst. Growth Des., 2014, 14(11), 5397–5407 CrossRef CAS.
- D. Banerjee, J. Finkelstein, A. Smirnov, P. M. Forster, L. A. Borkowski and S. J. Teat, et al., Synthesis and Structural Characterization of Magnesium Based Coordination Networks in Different Solvents, Cryst. Growth Des., 2011, 11(6), 2572–2579 CrossRef CAS.
- T. A. Mulyati, R. Ediati and A. Rosyidah, Influence of Solvothermal Temperatures and Times on Crystallinity and Morphology of MOF-5, Indones. J. Chem., 2015, 15(2), 101–107 CrossRef CAS.
- B. Li, Y. Zhang, D. Ma, L. Li, G. Li and G. Li, et al., A strategy toward constructing a bifunctionalized MOF catalyst: post-synthetic modification of MOFs on organic ligands and coordinatively unsaturated metal sites, Chem. Commun., 2012, 48(49), 6151–6153 RSC.
- S. Ko, F. Gao, X. Yao, H. Yi, X. Tang and C. Wang, et al., Synthesis of metal–organic frameworks (MOFs) and their application in the selective catalytic reduction of NOx with NH3, New J. Chem., 2022, 46(33), 15758–15775 CAS.
- C. Mottillo and T. Friščić, Advances in Solid-State Transformations of Coordination Bonds: From the Ball Mill to the Aging Chamber, Molecules, 2017, 22(1), 144 Search PubMed.
- H. Luo, F. Cheng, L. Huelsenbeck and N. Smith, Comparison between conventional solvothermal and aqueous solution-based production of UiO-66-NH2: Life cycle assessment, techno-economic assessment, and implications for CO2 capture and storage, J. Environ. Chem. Eng., 2021, 9(2), 105159 CrossRef CAS.
- S. Dai, C. Simms, I. Dovgaliuk, G. Patriarche, A. Tissot and T. N. Parac-Vogt, et al., Monodispersed MOF-808 Nanocrystals Synthesized via a Scalable Room-Temperature Approach for Efficient Heterogeneous Peptide Bond Hydrolysis, Chem. Mater., 2021, 33(17), 7057–7066 CrossRef CAS.
- M. Díaz-García, Á. Mayoral, I. Díaz and M. Sánchez-Sánchez, Nanoscaled M-MOF-74 Materials Prepared at Room Temperature, Cryst. Growth Des., 2014, 14(5), 2479–2487 CrossRef.
- S. He, L. Wu, X. Li, H. Sun, T. Xiong and J. Liu, et al., Metal-organic frameworks for advanced drug delivery, Acta Pharm. Sin. B, 2021, 11(8), 2362–2395 CrossRef CAS PubMed.
- D. W. Jung, D. A. Yang, J. Kim, J. Kim and W. S. Ahn, Facile synthesis of MOF-177 by a sonochemical method using 1-methyl-2-pyrrolidinone as a solvent, Dalton Trans., 2010, 39(11), 2883–2887 RSC.
- W. J. Son, J. Kim, J. Kim and W. S. Ahn, Sonochemical synthesis of MOF-5, Chem. Commun., 2008,(47), 6336–6338 RSC.
- W. Xu, G. Li, W. Li and H. Zhang, Facile room temperature synthesis of metal–organic frameworks from newly synthesized copper/zinc hydroxide and their application in adsorptive desulfurization, RSC Adv., 2016, 6(44), 37530–37534 RSC.
- J. H. Lee, Y. Ahn and S. Y. Kwak, Facile Sonochemical Synthesis of Flexible Fe-Based Metal–Organic Frameworks and Their Efficient Removal of Organic Contaminants from Aqueous Solutions, ACS Omega, 2022, 7(27), 23213–23222 CrossRef CAS PubMed.
- H. Ren and T. Wei, Electrochemical Synthesis Methods of Metal-Organic Frameworks and Their Environmental Analysis Applications: A Review, ChemElectroChem, 2022, 9(13), e202200196 CrossRef CAS.
- H. Al-Kutubi, J. Gascon, E. J. R. Sudhölter and L. Rassaei, Electrosynthesis of Metal–Organic Frameworks: Challenges and Opportunities, ChemElectroChem, 2015, 2(4), 462–474 CrossRef CAS.
- W. Wu, G. E. Decker, A. E. Weaver, A. I. Arnoff, E. D. Bloch and J. Rosenthal, Facile and Rapid Room-Temperature Electrosynthesis and Controlled Surface Growth of Fe-MIL-101 and Fe-MIL-101-NH2, ACS Cent. Sci., 2021, 7(8), 1427–1433 CrossRef CAS PubMed.
- Y. R. Lee, J. Kim and W. S. Ahn, Synthesis of metal-organic frameworks: A mini review, Korean J. Chem. Eng., 2013, 30(9), 1667–1680 CrossRef CAS.
- S. H. Jhung, J. H. Lee, P. M. Forster, G. Férey, A. K. Cheetham and J. S. Chang, Microwave Synthesis of Hybrid Inorganic–Organic Porous Materials: Phase-Selective and Rapid Crystallization, Chem. – Eur. J., 2006, 12(30), 7899–7905 CrossRef PubMed.
- H. K. Liu, T. H. Tsao, Y. T. Zhang and C. H. Lin, Microwave synthesis and single-crystal-to-single-crystal transformation of magnesium coordination polymers exhibiting selective gas adsorption and luminescence properties, CrystEngComm, 2009, 11(7), 1462–1468 RSC.
- T. J. Azbell, T. A. Pitt, M. M. Bollmeyer, C. Cong, K. M. Lancaster and P. J. Milner, Ionothermal Synthesis of Metal-Organic Frameworks Using Low-Melting Metal Salt Precursors, Angew. Chem., Int. Ed., 2023, 62(17), e202218252 CrossRef CAS PubMed.
- P. Li, F. F. Cheng, W. W. Xiong and Q. Zhang, New synthetic strategies to prepare metal–organic frameworks, Inorg. Chem. Front., 2018, 5(11), 2693–2708 RSC.
- R. E. Morris, Ionothermal synthesis—ionic liquids as functional solvents in the preparation of crystalline materials, Chem. Commun., 2009,(21), 2990–2998 RSC.
- S. Tanaka, K. Kida, T. Nagaoka, T. Ota and Y. Miyake, Mechanochemical dry conversion of zinc oxide to zeolitic imidazolate framework, Chem. Commun., 2013, 49(72), 7884–7886 RSC.
- S. Głowniak, B. Szczęśniak, J. Choma and M. Jaroniec, Mechanochemistry: Toward green synthesis of metal–organic frameworks, Mater. Today, 2021, 46, 109–124 CrossRef.
- A. Pichon, A. Lazuen-Garay and S. L. James, Solvent-free synthesis of a microporous metal–organic framework, CrystEngComm, 2006, 8(3), 211–214 RSC.
- J. Xu, J. Liu, Z. Li, X. Wang, Y. Xu and S. Chen, et al., Optimized synthesis of Zr(IV) metal organic frameworks (MOFs-808) for efficient hydrogen storage, New J. Chem., 2019, 43(10), 4092–4099 RSC.
- K. Suresh, D. Aulakh, J. Purewal, D. J. Siegel, M. Veenstra and A. J. Matzger, Optimizing Hydrogen Storage in MOFs
through Engineering of Crystal Morphology and Control of Crystal Size, J. Am. Chem. Soc., 2021, 143(28), 10727–10734 CrossRef CAS PubMed.
- X. Yang, T. Song, T. Su, J. Hu and S. Wu, Exploring the Influence of the Reused Methanol Solution for the Structure and Properties of the Synthesized ZIF-8, Processes, 2022, 10(9), 1705 CrossRef CAS.
- A. M. P. Peedikakkal and I. H. Aljundi, Upgrading the Hydrogen Storage of MOF-5 by Post-Synthetic Exchange with Divalent Metal Ions, Appl. Sci., 2021, 11(24), 11687 CrossRef CAS.
- M. Usman, M. Y. Khan, T. Anjum, A. L. Khan, B. Hoque and A. Helal, et al., Controlled Covalent Functionalization of ZIF-90 for Selective CO2 Capture & Separation, Membranes, 2022, 12(11), 1055 CrossRef CAS PubMed.
- Y. Liu, C. Wang, S. Ju, M. Li, A. Yuan and G. Zhu, FeCo-based hybrid MOF derived active species for effective oxygen evolution, Prog. Nat. Sci.:Mater. Int., 2020, 30(2), 185–191 CrossRef CAS.
- R. Ameloot, F. Vermoortele, J. Hofkens, F. C. De Schryver, D. E. De Vos and M. B. J. Roeffaers, Three-Dimensional Visualization of Defects Formed during the Synthesis of Metal–Organic Frameworks: A Fluorescence Microscopy Study, Angew. Chem., Int. Ed., 2013, 52(1), 401–405 CrossRef CAS PubMed.
- J. Schaber, S. Krause, S. Paasch, I. Senkovska, V. Bon and D. M. Többens, et al., In Situ Monitoring of Unique Switching Transitions in the Pressure-Amplifying Flexible Framework Material DUT-49 by High-Pressure 129Xe NMR Spectroscopy, J. Phys. Chem. C, 2017, 121(9), 5195–5200 CrossRef CAS.
- B. Chen, X. Zhao, A. Putkham, K. Hong, E. B. Lobkovsky and E. J. Hurtado, et al., Surface Interactions and Quantum Kinetic Molecular Sieving for H2 and D2 Adsorption on a Mixed Metal–Organic Framework Material, J. Am. Chem. Soc., 2008, 130(20), 6411–6423 CrossRef CAS PubMed.
- J. L. Belof, A. C. Stern, M. Eddaoudi and B. Space, On the Mechanism of Hydrogen Storage in a Metal–Organic Framework Material, J. Am. Chem. Soc., 2007, 129(49), 15202–15210 CrossRef CAS PubMed.
- G. Sdanghi, R. L. S. Canevesi, A. Celzard, M. Thommes and V. Fierro, Characterization of Carbon Materials for Hydrogen Storage and Compression, C, 2020, 6(3), 46 CAS.
- T. He, P. Pachfule, H. Wu, Q. Xu and P. Chen, Hydrogen carriers, Nat. Rev. Mater., 2016, 1(12), 1–17 Search PubMed.
- D. A. Gómez-Gualdrón, P. Z. Moghadam, J. T. Hupp, O. K. Farha and R. Q. Snurr, Application of Consistency Criteria To Calculate BET Areas of Micro- And Mesoporous Metal–Organic Frameworks, J. Am. Chem. Soc., 2016, 138(1), 215–224 CrossRef PubMed.
- R. Balderas-Xicohténcatl, P. Schmieder, D. Denysenko, D. Volkmer and M. Hirscher, High Volumetric Hydrogen Storage Capacity using Interpenetrated Metal–Organic Frameworks, Energy Technol., 2018, 6(3), 510–512 CrossRef.
- R. Balderas-Xicohténcatl, M. Schlichtenmayer and M. Hirscher, Volumetric Hydrogen Storage Capacity in Metal–Organic Frameworks, Energy Technol., 2018, 6(3), 578–582 CrossRef.
- M. Hirscher, Hydrogen Storage by Cryoadsorption in Ultrahigh-Porosity Metal–Organic Frameworks, Angew. Chem., Int. Ed., 2011, 50(3), 581–582 CrossRef CAS PubMed.
- M. Thommes, K. Kaneko, A. V. Neimark, J. P. Olivier, F. Rodriguez-Reinoso and J. Rouquerol, et al., Physisorption of gases, with special reference to the evaluation of surface area and pore size distribution (IUPAC Technical Report), Pure Appl. Chem., 2015, 87(9–10), 1051–1069 CrossRef CAS.
- M. Schlichtenmayer and M. Hirscher, The usable capacity of porous materials for hydrogen storage, Appl. Phys. A, 2016, 122(4), 379 CrossRef.
- S. K. Bhatia and A. L. Myers, Optimum Conditions for Adsorptive Storage, Langmuir, 2006, 22(4), 1688–1700 CrossRef CAS PubMed.
- D. E. Jaramillo, H. Z. H. Jiang, H. A. Evans, R. Chakraborty, H. Furukawa and C. M. Brown, et al., Ambient-Temperature Hydrogen Storage via Vanadium(II)-Dihydrogen Complexation in a Metal–Organic Framework, J. Am. Chem. Soc., 2021, 143(16), 6248–6256 CrossRef CAS PubMed.
- M. P. Suh, H. J. Park, T. K. Prasad and D. W. Lim, Hydrogen Storage in Metal–Organic Frameworks, Chem. Rev., 2012, 112(2), 782–835 CrossRef CAS PubMed.
- K. M. Thomas, Hydrogen adsorption and storage on porous materials, Catal. Today, 2007, 120(3), 389–398 CrossRef CAS.
- H. W. Langmi, J. Ren, B. North, M. Mathe and D. Bessarabov, Hydrogen Storage in Metal-Organic Frameworks: A Review, Electrochim. Acta, 2014, 128, 368–392 CrossRef CAS.
- Y. S. Bae and R. Q. Snurr, Optimal isosteric heat of adsorption for hydrogen storage and delivery using metal–organic frameworks, Microporous Mesoporous Mater., 2010, 132(1), 300–303 CrossRef CAS.
- M. Schlichtenmayer and M. Hirscher, Nanosponges for hydrogen storage, J. Mater. Chem., 2012, 22(20), 10134–10143 RSC.
- D. A. Gómez-Gualdrón, T. C. Wang, P. García-Holley, R. M. Sawelewa, E. Argueta and R. Q. Snurr, et al., Understanding Volumetric and Gravimetric Hydrogen Adsorption Trade-off in Metal–Organic Frameworks, ACS Appl. Mater. Interfaces, 2017, 9(39), 33419–33428 CrossRef PubMed.
- Z. Chen, P. Li, R. Anderson, X. Wang, X. Zhang and L. Robison, et al., Balancing volumetric and gravimetric uptake in highly porous materials for clean energy, Science, 2020, 368(6488), 297–303 CrossRef CAS PubMed.
- D. Yuan, D. Zhao, D. Sun and H. C. Zhou, An Isoreticular Series of Metal–Organic Frameworks with Dendritic Hexacarboxylate Ligands and Exceptionally High Gas-Uptake Capacity, Angew. Chem., Int. Ed., 2010, 49(31), 5357–5361 CrossRef CAS PubMed.
- P. García-Holley, B. Schweitzer, T. Islamoglu, Y. Liu, L. Lin and S. Rodriguez, et al., Benchmark Study of Hydrogen Storage in Metal–Organic Frameworks under Temperature and Pressure Swing Conditions, ACS Energy Lett., 2018, 3(3), 748–754 CrossRef.
- A. Ahmed, S. Seth, J. Purewal, A. G. Wong-Foy, M. Veenstra and A. J. Matzger, et al., Exceptional hydrogen storage achieved by screening nearly half a million metal-organic frameworks, Nat. Commun., 2019, 10(1), 1568 CrossRef PubMed.
- P. Ramirez-Vidal, G. Sdanghi, A. Celzard and V. Fierro, High hydrogen release by cryo-adsorption and compression on porous materials, Int. J. Hydrogen Energy, 2022, 47(14), 8892–8915 CrossRef CAS.
- W. Shi, X. Jin, C. Zhang, X. Zhang, X. Liu and Y. Gao, et al., Recent advancement in metal-organic frameworks for hydrogen storage: Mechanisms, influencing factors and enhancement strategies, Int. J. Hydrogen Energy, 2024, 83, 432–449 CrossRef CAS.
- X. Han, X. Yang, C. Yu, S. Lu, E. S. Pouya and P. Bai, et al., Fine-tuning the pore structure of metal–organic frameworks by linker substitution for enhanced hydrogen storage and gas separation, CrystEngComm, 2021, 23(16), 3026–3032 RSC.
- R. M. Kumar, J. V. Sundar and V. Subramanian, Improving the hydrogen storage capacity of metal organic framework by chemical functionalization, Int. J. Hydrogen Energy, 2012, 37(21), 16070–16077 CrossRef CAS.
- M. Dincǎ, A. F. Yu and J. R. Long, Microporous Metal–Organic Frameworks Incorporating 1,4-Benzeneditetrazolate: Syntheses, Structures, and Hydrogen Storage Properties, J. Am. Chem. Soc., 2006, 128(27), 8904–8913 CrossRef PubMed.
- Z. Wang, K. K. Tanabe and S. M. Cohen, Tuning Hydrogen Sorption Properties of Metal–Organic Frameworks by Postsynthetic Covalent Modification, Chem. – Eur. J., 2010, 16(1), 212–217 CrossRef CAS PubMed.
- A. L. Sutton, J. I. Mardel and M. R. Hill, Metal-Organic Frameworks (MOFs) As Hydrogen Storage Materials At Near-Ambient Temperature, Chem. – Eur. J., 2024, 30(44), e202400717 CrossRef CAS PubMed.
- D. Sengupta, P. Melix, S. Bose, J. Duncan, X. Wang and M. R. Mian, et al., Air-Stable Cu(I) Metal–Organic Framework for Hydrogen Storage, J. Am. Chem. Soc., 2023, 145(37), 20492–20502 CrossRef CAS PubMed.
- N. C. Burtch, H. Jasuja and K. S. Walton, Water Stability and Adsorption in Metal–Organic Frameworks, Chem. Rev., 2014, 114(20), 10575–10612 CrossRef CAS PubMed.
- S. Yuan, L. Feng, K. Wang, J. Pang, M. Bosch and C. Lollar, et al., Stable Metal–Organic Frameworks: Design, Synthesis, and Applications, Adv. Mater., 2018, 30(37), 1704303 CrossRef PubMed.
- J. A. Greathouse and M. D. Allendorf, The Interaction of Water with MOF-5 Simulated by Molecular Dynamics, J. Am. Chem. Soc., 2006, 128(33), 10678–10679 CrossRef CAS PubMed.
- G. G. Terrones, S. P. Huang, M. P. Rivera, S. Yue, A. Hernandez and H. J. Kulik, Metal–Organic Framework Stability in Water and Harsh Environments from Data-Driven Models Trained on the Diverse WS24 Data Set, J. Am. Chem. Soc., 2024, 146(29), 20333–20348 CrossRef CAS PubMed.
- P. Deria, D. A. Gómez-Gualdrón, W. Bury, H. T. Schaef, T. C. Wang and P. K. Thallapally, et al., Ultraporous, Water Stable, and Breathing Zirconium-Based Metal–Organic Frameworks with ftw Topology, J. Am. Chem. Soc., 2015, 137(40), 13183–13190 CrossRef CAS PubMed.
- H. R. Fu, Z. X. Xu and J. Zhang, Water-Stable Metal–Organic Frameworks for Fast and High Dichromate Trapping via Single-Crystal-to-Single-Crystal Ion Exchange, Chem. Mater., 2015, 27(1), 205–210 CrossRef CAS.
- S. J. Yang, J. Y. Choi, H. K. Chae, J. H. Cho, K. S. Nahm and C. R. Park, Preparation and Enhanced Hydrostability and Hydrogen Storage Capacity of CNT@MOF-5 Hybrid Composite, Chem. Mater., 2009, 21(9), 1893–1897 CrossRef CAS.
- W. Zhang, Y. Hu, J. Ge, H. L. Jiang and S. H. Yu, A Facile and General Coating Approach to Moisture/Water-Resistant Metal–Organic Frameworks with Intact Porosity, J. Am. Chem. Soc., 2014, 136(49), 16978–16981 CrossRef CAS PubMed.
- X. Qian, R. Zhang, L. Chen, Y. Lei and A. Xu, Surface Hydrophobic Treatment of Water-Sensitive DUT-4 Metal–Organic Framework To Enhance Water Stability for Hydrogen Storage, ACS Sustainable Chem. Eng., 2019, 7(19), 16007–16012 CrossRef CAS.
- C. Schlemminger, E. Næss and U. Bünger, Adsorption hydrogen storage at cryogenic temperature – Material properties and hydrogen ortho-para conversion matters, Int. J. Hydrogen Energy, 2015, 40(20), 6606–6625 CrossRef CAS.
- H. Wang, Z. Qu, W. Zhang and W. Tao, Effective Thermal Conductivity of MOF-5 Powder under a Hydrogen Atmosphere, Computation, 2015, 3(4), 558–573 CrossRef CAS.
- L. Han, M. Budge and P. Alex Greaney, Relationship between thermal conductivity and framework architecture in MOF-5, Comput. Mater. Sci., 2014, 94, 292–297 CrossRef CAS.
- H. Babaei, A. J. H. McGaughey and C. E. Wilmer, Effect of pore size and shape on the thermal conductivity of metal-organic frameworks, Chem. Sci., 2016, 8(1), 583–589 RSC.
- M. Islamov, H. Babaei, R. Anderson, K. B. Sezginel, J. R. Long and A. J. H. McGaughey, et al., High-throughput screening of hypothetical metal-organic frameworks for thermal conductivity, npj Comput. Mater., 2023, 9(1), 1–12 CrossRef.
- H. Babaei, M. E. DeCoster, M. Jeong, Z. M. Hassan, T. Islamoglu and H. Baumgart, et al., Observation of reduced thermal conductivity in a metal-organic framework due to the presence of adsorbates, Nat. Commun., 2020, 11(1), 4010 CrossRef CAS PubMed.
- M. Islamov, H. Babaei and C. E. Wilmer, Influence of Missing Linker Defects on the Thermal Conductivity of Metal–Organic Framework HKUST-1, ACS Appl. Mater. Interfaces, 2020, 12(50), 56172–56177 CrossRef CAS PubMed.
- B. Yeskendir, J. P. Dacquin, Y. Lorgouilloux, C. Courtois, S. Royer and J. Dhainaut, From metal–organic framework powders to shaped solids: recent developments and challenges, Mater. Adv., 2021, 2(22), 7139–7186 RSC.
- G. T. Whiting, A. D. Chowdhury, R. Oord, P. Paalanen and B. M. Weckhuysen, The curious case of zeolite–clay/binder interactions and their consequences for catalyst preparation, Faraday Discuss., 2016, 188(0), 369–386 RSC.
- J. Farrando-Pérez, M. Rodríguez-Castillo, M. Martínez-Escandell, M. Monge and J. Silvestre-Albero, Improved thermal management in HKUST-1 composites upon graphite flakes incorporation: Hydrogen adsorption properties, Int. J. Hydrogen Energy, 2023, 48(93), 36474–36484 CrossRef.
- R. Sule, A. K. Mishra and T. T. Nkambule, Recent advancement in consolidation of MOFs as absorbents for hydrogen storage, Int. J. Energy Res., 2021, 45(9), 12481–12499 CrossRef CAS.
- J. Łuczak, M. Kroczewska, M. Baluk, J. Sowik, P. Mazierski and A. Zaleska-Medynska, Morphology control through the synthesis of metal-organic frameworks, Adv. Colloid Interface Sci., 2023, 314, 102864 CrossRef PubMed.
- K. Leng, Y. Sun, X. Li, S. Sun and W. Xu, Rapid Synthesis of Metal–Organic Frameworks MIL-101(Cr) Without the Addition of Solvent and Hydrofluoric Acid, Cryst. Growth Des., 2016, 16(3), 1168–1171 CrossRef CAS.
- G. Mouchaham, S. Wang and C. Serre, The Stability of Metal–Organic Frameworks, in Metal–Organic Frameworks [Internet], ed. H. García and S. Navalón, Wiley, 1st edn, 2018, pp. 1–28. Available from: https://onlinelibrary.wiley.com/doi/10.1002/9783527809097.ch1 [cited 2024 Feb 16] Search PubMed.
- H. U. Escobar-Hernandez, L. M. Pérez, P. Hu, F. A. Soto, M. I. Papadaki and H. C. Zhou, et al., Thermal Stability of Metal–Organic Frameworks (MOFs): Concept, Determination, and Model Prediction Using Computational Chemistry and Machine Learning, Ind. Eng. Chem. Res., 2022, 61(17), 5853–5862 CrossRef CAS.
- S. A. Mohamed, S. Chong and J. Kim, Thermal Stability of Methyl-Functionalized MOF-5, J. Phys. Chem. C, 2019, 123(49), 29686–29692 CrossRef CAS.
- M. Balcerzak, J. Ternieden and M. Felderhoff, Synthesis, thermal stability, and hydrogen storage properties of poorly crystalline TiVFeCuNb multi-principal element alloy: Dedicated to the memory of Michel Latroche and his great contribution to the field of metal hydrides, J. Alloys Compd., 2023, 943, 169142 CrossRef CAS.
- T. D. Bennett, T. K. Todorova, E. F. Baxter, D. G. Reid, C. Gervais and B. Bueken, et al., Connecting defects and amorphization in UiO-66 and MIL-140 metal–organic frameworks: a combined experimental and computational study, Phys. Chem. Chem. Phys., 2016, 18(3), 2192–2201 RSC.
- L. Valenzano, B. Civalleri, S. Chavan, S. Bordiga, M. H. Nilsen and S. Jakobsen, et al., Disclosing the Complex Structure of UiO-66 Metal Organic Framework: A Synergic Combination of Experiment and Theory, Chem. Mater., 2011, 23(7), 1700–1718 CrossRef CAS.
- H. Tao, T. D. Bennett and Y. Yue, Melt-Quenched Hybrid Glasses from Metal–Organic Frameworks, Adv. Mater., 2017, 29(20), 1601705 CrossRef PubMed.
- Y. N. Gong, D. C. Zhong and T. B. Lu, Interpenetrating metal–organic frameworks, CrystEngComm, 2016, 18(15), 2596–2606 RSC.
- F. D. Lahoz-Martín, S. Calero, J. J. Gutiérrez-Sevillano and A. Martin-Calvo, Adsorptive separation of ethane and ethylene using IsoReticular Metal-Organic Frameworks, Microporous Mesoporous Mater., 2017, 248, 40–45 CrossRef.
- G. Verma, S. Butikofer, S. Kumar and S. Ma, Regulation of the Degree of Interpenetration in Metal–Organic Frameworks, Top. Curr. Chem., 2020, 378(1), 4 CrossRef CAS PubMed.
- L. Yuan, C. Zhang, Y. Zou, T. Bao, J. Wang and C. Tang, et al., A S-Scheme MOF-on-MOF Heterostructure, Adv. Funct. Mater., 2023, 33(20), 2214627 CrossRef CAS.
- Z. Yu, J. Deschamps, L. Hamon, P. Karikkethu Prabhakaran and P. Pré, Hydrogen adsorption and kinetics in MIL-101(Cr) and hybrid activated carbon-MIL-101(Cr) materials, Int. J. Hydrogen Energy, 2017, 42(12), 8021–8031 CrossRef CAS.
- P. B. Somayajulu Rallapalli, M. C. Raj, D. V. Patil, K. P. Prasanth, R. S. Somani and H. C. Bajaj, Activated carbon @ MIL-101(Cr): a potential metal-organic framework composite material for hydrogen storage, Int. J. Energy Res., 2013, 37(7), 746–753 CrossRef CAS.
- P. K. Prabhakaran and J. Deschamps, Room temperature hydrogen uptake in single walled carbon nanotubes incorporated MIL-101 doped with lithium: effect of lithium doping, J. Porous Mater., 2015, 22(6), 1635–1642 CrossRef CAS.
- J. G. Vitillo, L. Regli, S. Chavan, G. Ricchiardi, G. Spoto and P. D. C. Dietzel, et al., Role of Exposed Metal Sites in Hydrogen Storage in MOFs, J. Am. Chem. Soc., 2008, 130(26), 8386–8396 CrossRef CAS PubMed.
- Y. E. Cheon and M. P. Suh, Enhanced Hydrogen Storage by Palladium Nanoparticles Fabricated in a Redox-Active Metal–Organic Framework, Angew. Chem., Int. Ed., 2009, 48(16), 2899–2903 CrossRef CAS PubMed.
- B. Szczęśniak, J. Choma and M. Jaroniec, Gas adsorption properties of hybrid graphene-MOF materials, J. Colloid Interface Sci., 2018, 514, 801–813 CrossRef PubMed.
- X. Zhang, P. Liu and Y. Zhang, The application of MOFs for hydrogen storage, Inorg. Chim. Acta, 2023, 557, 121683 CrossRef CAS.
- K. K. Gangu, S. Maddila, S. B. Mukkamala and S. B. Jonnalagadda, Characteristics of MOF, MWCNT and graphene containing materials for hydrogen storage: A review, J. Energy Chem., 2019, 30, 132–144 CrossRef.
- M. C. Kreider, M. Sefa, J. A. Fedchak, J. Scherschligt, M. Bible and B. Natarajan, et al., Toward 3D printed hydrogen storage materials made with ABS-MOF composites, Polym. Adv. Technol., 2018, 29(2), 867–873 CrossRef CAS PubMed.
- Z. Ma, J. Zou, D. Khan, W. Zhu, C. Hu and X. Zeng, et al., Preparation and hydrogen storage properties of MgH2-trimesic acid-TM MOF (TM=Co, Fe) composites, J. Mater. Sci. Technol., 2019, 35(10), 2132–2143 CrossRef CAS.
- D. H. Hong, H. S. Shim, J. Ha and H. R. Moon, MOF-on-MOF Architectures: Applications in Separation, Catalysis, and Sensing, Bull. Korean Chem. Soc., 2021, 42(7), 956–969 CrossRef CAS.
- J. Sánchez-Laínez, A. Veiga, B. Zornoza, S. R. G. Balestra, S. Hamad and A. R. Ruiz-Salvador, et al., Tuning the separation properties of zeolitic imidazolate framework core–shell structures via post-synthetic modification, J. Mater. Chem. A, 2017, 5(48), 25601–25608 RSC.
- M. Ding, X. Cai and H. L. Jiang, Improving MOF stability: approaches and applications, Chem. Sci., 2019, 10(44), 10209–10230 RSC.
- M. J. Manos, M. S. Markoulides, C. D. Malliakas, G. S. Papaefstathiou, N. Chronakis and M. G. Kanatzidis, et al., A Highly Porous Interpenetrated Metal–Organic Framework from the Use of a Novel Nanosized Organic Linker, Inorg. Chem., 2011, 50(22), 11297–11299 CrossRef CAS PubMed.
- M. Gupta and J. J. Vittal, Control of interpenetration and structural transformations in the interpenetrated MOFs, Coord. Chem. Rev., 2021, 435, 213789 CrossRef CAS.
- H. L. Jiang, T. A. Makal and H. C. Zhou, Interpenetration control in metal–organic frameworks for functional applications, Coord. Chem. Rev., 2013, 257(15), 2232–2249 CrossRef CAS.
- F. G. Li, C. Liu, D. Yuan, F. Dai, R. Wang and Z. Wang, et al., Ultrahigh Hydrogen Uptake in an Interpenetrated Zn4O-Based Metal–Organic Framework, CCS Chem., 2021, 4(3), 832–837 CrossRef.
- H. Jiang, Y. Feng, M. Chen and Y. Wang, Synthesis and hydrogen-storage performance of interpenetrated MOF-5/MWCNTs hybrid composite with high mesoporosity, Int. J. Hydrogen Energy, 2013, 38(25), 10950–10955 CrossRef CAS.
- M. Xue, S. Ma, J. Z. Schaffino, R. M. Zhu, G. S. Lobkovsky and E. B, et al., Robust Metal–Organic Framework Enforced by Triple-Framework Interpenetration Exhibiting High H2 Storage Density, Inorg. Chem., 2008, 47(15), 6825–6828 CrossRef CAS PubMed.
- P. Kanoo, R. Matsuda, M. Higuchi, S. Kitagawa and T. K. Maji, New Interpenetrated Copper Coordination Polymer Frameworks having Porous Properties, Chem. Mater., 2009, 21(24), 5860–5866 CrossRef CAS.
- Y. Feng, H. Jiang, M. Chen and Y. Wang, Construction of an interpenetrated MOF-5 with high mesoporosity for hydrogen storage at low pressure, Powder Technol., 2013, 249, 38–42 CrossRef CAS.
- P.Á Szilágyi, M. Lutz, J. Gascon, J. Juan-Alcañiz, J. van Esch and F. Kapteijn, et al., MOF@MOF core–shell vs. Janus particles and the effect of strain: potential for guest sorption, separation and sequestration, CrystEngComm, 2013, 15(30), 6003–6008 RSC.
- D. K. Panchariya, R. K. Rai, A. Kumar, E. Singh and S. K, Core–Shell Zeolitic Imidazolate Frameworks for Enhanced Hydrogen Storage, ACS Omega, 2018, 3(1), 167–175 CrossRef CAS PubMed.
- P. Pachfule, Y. Chen, J. Jiang and R. Banerjee, Experimental and computational approach of understanding the gas adsorption in amino functionalized interpenetrated metal organic frameworks (MOFs), J. Mater. Chem., 2011, 21(44), 17737–17745 RSC.
- D. J. Tranchemontagne, K. S. Park, H. Furukawa, J. Eckert, C. B. Knobler and O. M. Yaghi, Hydrogen Storage in New Metal–Organic Frameworks, J. Phys. Chem. C, 2012, 116(24), 13143–13151 CrossRef CAS.
- B. Kesanli, Y. Cui, M. R. Smith, E. W. Bittner, B. C. Bockrath and W. Lin, Highly Interpenetrated Metal–Organic Frameworks for Hydrogen Storage, Angew. Chem., 2005, 117(1), 74–77 CrossRef.
- V. Zeleňák and I. Saldan, Factors Affecting Hydrogen Adsorption in Metal–Organic Frameworks: A Short Review, Nanomaterials, 2021, 11(7), 1638 CrossRef PubMed.
- S. Ma, D. Sun, M. Ambrogio, J. A. Fillinger, S. Parkin and H. C. Zhou, Framework-Catenation Isomerism in Metal–Organic Frameworks and Its Impact on Hydrogen Uptake, J. Am. Chem. Soc., 2007, 129(7), 1858–1859 CrossRef CAS PubMed.
- L. Chai, J. Pan, Y. Hu, J. Qian and M. Hong, Rational Design and Growth of MOF-on-MOF Heterostructures, Small, 2021, 17(36), 2100607 CrossRef CAS PubMed.
- Y. Gu, Y. N. Wu, L. Li, W. Chen, F. Li and S. Kitagawa, Controllable Modular Growth of Hierarchical MOF-on-MOF Architectures, Angew. Chem., 2017, 129(49), 15864–15868 CrossRef.
- C. Liu, Q. Sun, L. Lin, J. Wang, C. Zhang and C. Xia, et al., Ternary MOF-on-MOF heterostructures with controllable architectural and compositional complexity via multiple selective assembly, Nat. Commun., 2020, 11(1), 4971 CrossRef CAS PubMed.
- C. Liu, L. Lin, Q. Sun, J. Wang, R. Huang and W. Chen, et al., Site-specific growth of MOF-on-MOF heterostructures with controllable nano-architectures: beyond the combination of MOF analogues, Chem. Sci., 2020, 11(14), 3680–3686 RSC.
- P. Sanati-Tirgan, H. Eshghi and A. Mohammadinezhad, Designing a new method for growing metal–organic framework (MOF) on MOF: synthesis, characterization and catalytic applications, Nanoscale, 2023, 15(10), 4917–4931 RSC.
- K. Ikigaki, K. Okada, Y. Tokudome, T. Toyao, P. Falcaro and C. J. Doonan, et al., MOF-on-MOF: Oriented Growth of Multiple Layered Thin Films of Metal–Organic Frameworks, Angew. Chem., Int. Ed., 2019, 58(21), 6886–6890 CrossRef CAS PubMed.
- T. Li, J. E. Sullivan and N. L. Rosi, Design and Preparation of a Core–Shell Metal–Organic Framework for Selective CO2 Capture, J. Am. Chem. Soc., 2013, 135(27), 9984–9987 CrossRef CAS PubMed.
- C. Verma, T. Rasheed, M. T. Anwar and M. A. Quraishi, From metal-organic frameworks (MOFs) to metal-doped MOFs (MDMOFs): Current and future scenarios in environmental catalysis and remediation applications, Microchem. J., 2023, 192, 108954 CrossRef CAS.
- C. M. Wu, M. Rathi, S. P. Ahrenkiel, R. T. Koodali and Z. Wang, Facile synthesis of MOF-5 confined in SBA-15 hybrid material with enhanced hydrostability, Chem. Commun., 2013, 49(12), 1223–1225 RSC.
- Z. Jaffar, N. M. Yunus, M. S. Shaharun, M. F. Allim and A. H. A. Rahim, Incorporated Metal–Organic Framework Hybrid Materials for Gas Separation, Catalysis and Wastewater Treatment, Processes, 2022, 10(11), 2368 CrossRef CAS.
- J. D. Sosa, T. F. Bennett, K. J. Nelms, B. M. Liu, R. C. Tovar and Y. Liu, Metal–Organic Framework Hybrid Materials and Their Applications, Crystals, 2018, 8(8), 325 CrossRef.
- M. Muschi, S. Devautour-Vinot, D. Aureau, N. Heymans, S. Sene and R. Emmerich, et al., Metal–organic framework/graphene oxide composites for CO 2 capture by microwave swing adsorption, J. Mater. Chem. A, 2021, 9(22), 13135–13142 RSC.
- A. Dutta, Y. Pan, J. Q. Liu and A. Kumar, Multicomponent isoreticular metal-organic frameworks: Principles, current status and challenges, Coord. Chem. Rev., 2021, 445, 214074 CrossRef CAS.
- M. Alfe, A. Policicchio, L. Lisi and V. Gargiulo, Solid sorbents for CO2 and CH4 adsorption: The effect of metal organic framework hybridization with graphene-like layers on the gas sorption capacities at high pressure, Renewable Sustainable Energy Rev., 2021, 141, 110816 CrossRef CAS.
- W. Zhang, Y. Liu, G. Lu, Y. Wang, S. Li and C. Cui, et al., Mesoporous Metal–Organic Frameworks with Size-, Shape-, and Space-Distribution-Controlled Pore Structure, Adv. Mater., 2015, 27(18), 2923–2929 CrossRef CAS PubMed.
- L. He, Y. Liu, J. Liu, Y. Xiong, J. Zheng and Y. Liu, et al., Core–Shell Noble-Metal@Metal-Organic-Framework Nanoparticles with Highly Selective Sensing Property, Angew. Chem., 2013, 125(13), 3829–3833 CrossRef.
- H. Liu, L. Chang, C. Bai, L. Chen, R. Luque and Y. Li, Controllable Encapsulation of “Clean” Metal Clusters within MOFs through Kinetic Modulation: Towards Advanced Heterogeneous Nanocatalysts, Angew. Chem., 2016, 128(16), 5103–5107 CrossRef.
- Z. Wang and S. M. Cohen, Postsynthetic modification of metal–organic frameworks, Chem. Soc. Rev., 2009, 38(5), 1315–1329 RSC.
- P. K. Prabhakaran and J. Deschamps, Doping activated carbon
incorporated composite MIL-101 using lithium: impact on hydrogen uptake, J. Mater. Chem. A, 2015, 3(13), 7014–7021 RSC.
- Z. Xiang, Z. Hu, W. Yang and D. Cao, Lithium doping on metal-organic frameworks for enhancing H2 Storage, Int. J. Hydrogen Energy, 2012, 37(1), 946–950 CrossRef CAS.
- C. Zlotea, R. Campesi, F. Cuevas, E. Leroy, P. Dibandjo and C. Volkringer, et al., Pd Nanoparticles Embedded into a Metal-Organic Framework: Synthesis, Structural Characteristics, and Hydrogen Sorption Properties, J. Am. Chem. Soc., 2010, 132(9), 2991–2997 CrossRef CAS PubMed.
- S. J. Yang, J. H. Cho, K. S. Nahm and C. R. Park, Enhanced hydrogen storage capacity of Pt-loaded CNT@MOF-5 hybrid composites, Int. J. Hydrogen Energy, 2010, 35(23), 13062–13067 CrossRef CAS.
- D. W. Lim, J. W. Yoon, K. Y. Ryu and M. P. Suh, Magnesium Nanocrystals Embedded in a Metal–Organic Framework: Hybrid Hydrogen Storage with Synergistic Effect on Physi- and Chemisorption, Angew. Chem., Int. Ed., 2012, 51(39), 9814–9817 CrossRef CAS PubMed.
- X. Hu, J. Wang, S. Li, X. Hu, R. Ye and L. Zhou, et al., Pd-doped HKUST-1 MOFs for enhanced hydrogen storage: effect of hydrogen spillover, RSC Adv., 2023, 13(22), 14980–14990 RSC.
- J. R. Varghese, C. Wendt, F. B. Dix, D. Aulakh, U. Sazama and A. A. Yakovenko, et al., Design and Characterization of Metal Nanoparticle Infiltrated Mesoporous Metal–Organic Frameworks, Inorg. Chem., 2021, 60(17), 13000–13010 CrossRef CAS PubMed.
- S. N. Klyamkin, S. V. Chuvikov, N. V. Maletskaya, E. V. Kogan, V. P. Fedin and K. A. Kovalenko, et al., High-pressure hydrogen storage on modified MIL-101 metal–organic framework, Int. J. Energy Res., 2014, 38(12), 1562–1570 CrossRef CAS.
- J. A. Villajos, G. Orcajo, G. Calleja, J. A. Botas and C. Martos, Beneficial cooperative effect between Pd nanoparticles and ZIF-8 material for hydrogen storage, Int. J. Hydrogen Energy, 2016, 41(42), 19439–19446 CrossRef CAS.
- J. Jin, J. Ouyang and H. Yang, Pd Nanoparticles and MOFs Synergistically Hybridized Halloysite Nanotubes for Hydrogen Storage, Nanoscale Res. Lett., 2017, 12(1), 240 CrossRef PubMed.
- N. M. Musyoka, J. Ren, H. W. Langmi, B. C. North, M. Mathe and D. Bessarabov, Synthesis of rGO/Zr-MOF composite for hydrogen storage application, J. Alloys Compd., 2017, 724, 450–455 CrossRef CAS.
- Z. Rao, K. Feng, B. Tang and P. Wu, Construction of well interconnected metal-organic framework structure for effectively promoting proton conductivity of proton exchange membrane, J. Membr. Sci., 2017, 533, 160–170 CrossRef CAS.
- Z. Chen, Z. G. Gu, W. Q. Fu, F. Wang and J. Zhang, A Confined Fabrication of Perovskite Quantum Dots in Oriented MOF Thin Film, ACS Appl. Mater. Interfaces, 2016, 8(42), 28737–28742 CrossRef CAS PubMed.
- E. H. Otal, M. L. Kim, Y. Hattori, Y. Kitazawa, J. P. Hinestroza and M. Kimura, Versatile Covalent Postsynthetic Modification of Metal Organic Frameworks via Thermal Condensation for Fluoride Sensing in Waters, Bioengineering, 2021, 8(12), 196 CrossRef CAS PubMed.
- K. P. Prasanth, P. Rallapalli, M. C. Raj, H. C. Bajaj and R. V. Jasra, Enhanced hydrogen sorption in single walled carbon nanotube incorporated MIL-101 composite metal–organic framework, Int. J. Hydrogen Energy, 2011, 36(13), 7594–7601 CrossRef CAS.
- J. L. C. Rowsell and O. M. Yaghi, Strategies for Hydrogen Storage in Metal–Organic Frameworks, Angew. Chem., Int. Ed., 2005, 44(30), 4670–4679 CrossRef CAS PubMed.
- S. Liu, L. Sun, F. Xu, J. Zhang, C. Jiao and F. Li, et al., Nanosized Cu-MOFs induced
by graphene oxide and enhanced gas storage capacity, Energy Environ. Sci., 2013, 6, 818–823 RSC.
- C. Petit, J. Burress and T. J. Bandosz, The synthesis and characterization of copper-based metal–organic framework/graphite oxide composites, Carbon, 2011, 49(2), 563–572 CrossRef CAS.
- E. Rojas-Garcia, A. A. Castañeda-Ramírez, D. Angeles-Beltrán, R. López-Medina and A. M. Maubert-Franco, Enhancing in the hydrogen storage by SWCNT/HKUST-1 composites: Effect of SWCNT amount, Catal. Today, 2022, 394–396, 357–364 CrossRef CAS.
- A. M. Varghese, K. S. K. Reddy and G. N. Karanikolos, An In situ-Grown Cu-BTC Metal–Organic Framework/Graphene Oxide Hybrid Adsorbent for Selective Hydrogen Storage at Ambient Temperature, Ind. Eng. Chem. Res., 2022, 61(18), 6200–6213 CrossRef CAS.
- S. Y. Lee and S. J. Park, Effect of platinum doping of activated carbon on hydrogen storage behaviors of metal-organic frameworks-5, Int. J. Hydrogen Energy, 2011, 36(14), 8381–8387 CrossRef CAS.
- J. S. Li, Y. J. Tang, S. L. Li, S. R. Zhang, Z. H. Dai and L. Si, et al., Carbon nanodots functional MOFs composites by a stepwise synthetic approach: enhanced H2 storage and fluorescent sensing, CrystEngComm, 2015, 17(5), 1080–1085 RSC.
- K. Kamal, D. I. Grekov, A. M. Shariff, M. A. Bustam and P. Pré, Improving textural properties of magnesium-based metal-organic framework for gas adsorption by carbon doping, Microporous Mesoporous Mater., 2021, 323, 111246 CrossRef CAS.
- Y. Liu, P. Ghimire and M. Jaroniec, Copper benzene-1,3,5-tricarboxylate (Cu-BTC) metal-organic framework (MOF) and porous carbon composites as efficient carbon dioxide adsorbents, J. Colloid Interface Sci., 2019, 535, 122–132 CrossRef CAS PubMed.
- A. Domán, S. Klébert, J. Madarász, G. Sáfrán, Y. Wang and K. László, Graphene Oxide Protected Copper Benzene-1,3,5-Tricarboxylate for Clean Energy Gas Adsorption, Nanomaterials, 2020, 10(6), 1182 CrossRef PubMed.
- H. Zhu, K. Li, M. Chen, H. Cao and F. Wang, A Novel Metal–Organic Framework Route to Embed Co Nanoparticles into Multi-Walled Carbon Nanotubes for Effective Oxygen Reduction in Alkaline Media, Catalysts, 2017, 7(12), 364 CrossRef.
- J. Kim, S. Yeo, J. D. Jeon and S. Y. Kwak, Enhancement of hydrogen storage capacity and hydrostability of metal–organic frameworks (MOFs) with surface-loaded platinum nanoparticles and carbon black, Microporous Mesoporous Mater., 2015, 202, 8–15 CrossRef CAS.
- S. J. Yang and C. R. Park, Preparation of Highly Moisture-Resistant Black-Colored Metal Organic Frameworks, Adv. Mater., 2012, 24(29), 4010–4013 CrossRef CAS PubMed.
- K. Jayaramulu, K. K. R. Datta, C. Rösler, M. Petr, M. Otyepka and R. Zboril, et al., Biomimetic Superhydrophobic/Superoleophilic Highly Fluorinated Graphene Oxide and ZIF-8 Composites for Oil-Water Separation, Angew. Chem., Int. Ed., 2016, 55(3), 1178–1182 CrossRef CAS PubMed.
- M. I. Nandasiri, J. Liu, B. P. McGrail, J. Jenks, H. T. Schaef and V. Shutthanandan, et al., Increased Thermal Conductivity in Metal-Organic Heat Carrier Nanofluids, Sci. Rep., 2016, 6(1), 27805 CrossRef CAS PubMed.
- J. Purewal, D. Liu, A. Sudik, M. Veenstra, J. Yang and S. Maurer, et al., Improved Hydrogen Storage and Thermal Conductivity in High-Density MOF-5 Composites, J. Phys. Chem. C, 2012, 116(38), 20199–20212 CrossRef CAS.
- S. K. Gebremariam, L. F. Dumée, P. L. Llewellyn, Y. F. AlWahedi and G. N. Karanikolos, Metal-organic framework hybrid adsorbents for carbon capture – A review, J. Environ. Chem. Eng., 2023, 11(2), 109291 CrossRef CAS.
- G. Wang, E. Graham, S. Zheng, J. Zhu, R. Zhu and H. He, et al., Diatomite-Metal-Organic Framework Composite with Hierarchical Pore Structures for Adsorption/Desorption of Hydrogen, Carbon Dioxide and Water Vapor, Materials, 2020, 13(21), 4700 CrossRef CAS PubMed.
- H. S. Far, M. Najafi, M. Hasanzadeh and R. Rahimi, A 3D-printed hierarchical porous architecture of MOF@clay composite for rapid and highly efficient dye scavenging, New J. Chem., 2022, 46(48), 23351–23360 RSC.
- F. Gao, Y. Li, Z. Bian, J. Hu and H. Liu, Dynamic hydrophobic hindrance effect of zeolite@zeolitic imidazolate framework composites for CO2 capture in the presence of water, J. Mater. Chem. A, 2015, 3(15), 8091–8097 RSC.
- N. M. Musyoka, J. Ren, P. Annamalai, H. W. Langmi, B. C. North and M. Mathe, et al., Synthesis of a hybrid MIL-101(Cr)/ZTC composite for hydrogen storage applications, Res. Chem. Intermed., 2016, 42(6), 5299–5307 CrossRef CAS.
|
This journal is © The Royal Society of Chemistry 2025 |
Click here to see how this site uses Cookies. View our privacy policy here.