DOI:
10.1039/D4QI02449D
(Review Article)
Inorg. Chem. Front., 2025,
12, 85-117
The use of single-metal atom-based photocatalysts for the production of ammonia through photocatalytic nitrogen fixation
Received
27th September 2024
, Accepted 12th November 2024
First published on 12th November 2024
Abstract
The conventional synthetic ammonia industry is characterized by its high energy consumption, necessitating the exploration of a new environmentally sustainable method for NH3 synthesis. The photocatalytic nitrogen reduction reaction (pNRR) allows NH3 production under room conditions. The optimization of photocatalysts, particularly through the use of single-metal atom catalysts (SMACs), plays a significant role in enhancing the performance of pNRR. SMACs have garnered growing attention in photocatalysis for their exceptional catalytic activity, selectivity, stability, and complete atom utilization. These catalysts involve isolated atoms supported on substrates without aggregating into nanoparticles. In this review, we elucidate the mechanisms and pathways of pNRR, focusing on the latest advances in carbon-based and non-carbon-based SMACs, and conclude with an overview of the existing challenges and prospects of pNRR for sustainable ammonia production.
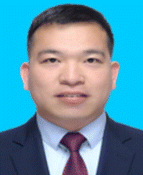 Ping Zhang | Ping Zhang is a professor at the School of Chemical Engineering, at Northwest Minzu University. In December 2014, he majored in organic chemistry at Northwest Normal University and obtained a doctor of science degree. Main research fields: photocatalysis and environmental treatment; photocatalytic CO2 reduction; study of photocatalytic nitrogen reduction. He has presided over more than 10 scientific research projects and published more than 50 papers. He has won the 9th Gansu Youth Science and Technology Award, the Young Teachers’ Talent Award in Gansu Province, and the Young Innovative and Entrepreneurial Talents in Longyuan. |
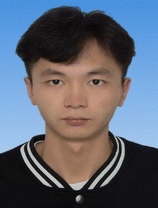 Yongchong Yu | Yongchong Yu received a bachelor's degree from Guizhou Normal University in June 2022. He is currently a master's student of Professor Ping Zhang's at the School of Chemical Engineering of Northwest Minzu University. The main research directions are the design and synthesis of nanomaterials, photocatalytic N2 reduction, photocatalytic pollutant degradation, and photocatalytic CO2 reduction. |
1. Introduction
NH3 is a pivotal chemical compound globally, crucial in producing nitrogen fertilizers essential for ensuring an ample food supply for human societies worldwide.1–3 Apart from its widespread application in agriculture, ammonia finds utility across various domains like military operations and chemical engineering, while also being recognized as an efficient renewable fuel source.4–6 The significance of ammonia is underscored by its extensive large-scale production over the past century. Notably, the industrial manufacturing of ammonia predominantly hinges on the Haber–Bosch process, which was first introduced in the early 1900s. However, this process operates at elevated reaction temperatures (673.15–773.15 K) and pressures (100–200 atm), necessitating substantial reliance on fossil fuels.7–12 Furthermore, the substantial hydrogen (H2) demand in the Haber–Bosch process is met through steam-reforming natural gas (CH4 + 2H2 → 4H2 + CO2), contributing to an annual release of approximately 1.6% of CO2 emissions into the atmosphere.13,14 Such carbon dioxide discharges have the potential to heighten the prevailing greenhouse effect and pose environmental challenges, diverging from the pursuit of sustainable development goals such as carbon neutrality and peak carbon emissions. Hence, the quest for an environment-friendly, economical, and sustainable way to achieve green synthesis is of paramount importance.
A great number of methods have been explored for ammonia synthesis, such as photocatalysis, biocatalysis assisted, photoelectrocatalysis combined, photothermal catalysis combined, and electrocatalysis. Since 1977, Schrauzer and Guth15 have been at the forefront of converting N2 to NH3 on TiO2 surfaces under ultraviolet light irradiation, contributing significantly to understanding TiO2-based nitrogen-fixing catalysts. In the 21st century, extensive efforts have been made towards advancing photocatalytic nitrogen fixation. The process relies on the clean, cost-effective, readily available light, water, and air components, as demonstrated by the following equation.
In theory, photocatalytic nitrogen fixation (PNF) is regarded as an energetically favorable process involving water splitting and N2 fixation, driven by solar energy. This approach presents a promising avenue for sustainable ammonia production without energy losses during power generation.16,17 By avoiding greenhouse gas by-products, PNF serves as a green alternative to conventional ammonia synthesis, offering a solution to environmental concerns and energy scarcity. Consequently, the employment of PNF emerges as a clean, energy-efficient, and environmentally benign strategy for NH3 production and environmental preservation.18,19 In recent years, the burgeoning interest in diverse semiconductor designs has propelled photocatalysis to the forefront of research in the field of energy and chemical fuel production, particularly for harnessing the boundless and eco-friendly solar energy derived from sunlight.20,21 This encompasses a wide array of applications such as the photocatalytic treatment of wastewater,22 which includes the breakdown of dyes, antibiotics like tetracycline,23,24 organic and inorganic pollutants, and pesticides.25–27 Additionally, photocatalysis has been instrumental in processes like hydrogen generation,28,29 CO2 reduction,30,31 and so on. The current landscape boasts a plethora of semiconductor materials, ranging from transition metal compounds,32,33 non-metallic polymers,34,35 metal–organic frameworks,36,37 layered double hydroxides,38,39 and single-metal atom catalysts,40–44 all of which have demonstrated considerable efficacy in facilitating photonitrogen fixation.
In 2011, Zhang et al. introduced a groundbreaking catalyst system by developing a metal platinum single-atom Pt/FeOx catalyst, thereby pioneering the concept of a single-metal atom catalyst (SMAC).45 Following this seminal work, scholars have extensively explored the realm of metal single atoms. A distinguishing characteristic of SMAC is the homogeneous dispersion of the co-catalyst (comprising precious metal/non-precious metal) as individual atoms across the surface of the semiconductor carrier. These isolated single atoms serve as active centers, thereby maximizing atomic efficiency. Distinct from conventional metal–metal bonding, single atoms primarily form bonds with heteroatoms present on the carrier.46 Recently, various SMACs have been successfully synthesized, prompting thorough investigations into the active center characteristics and photocatalytic reaction mechanisms.47 SMACs exhibit distinctive unsaturated coordination surroundings and electronic configurations, setting them apart from nanoparticles, clusters, and bulk catalysts. These particular features afford SMACs notable benefits in reactant adsorption/activation, carrier separation, and catalytic performance.48 For example, He et al.49 used Au single-atom isomorphic porphyrin-based COFs (COFX Au, X = 1–5) for pNRR. The COFs anchored with Au atoms exhibited high NH3 generation activity (427 μmol g−1 h−1), Similarly, Hu and colleagues42 fabricated single-atom Fe-porous g-C3N4 (FPx) samples through a one-step annealing method employing bubble template and direct metal atomization, eliminating the demand for sacrificial agents or cocatalysts. The FPx material significantly improved photocatalytic performance, achieving a rate of 62.42 μmol g−1 h−1, which was more than five times higher than the photocatalytic activity of bulk g-C3N4. In summary, SMACs present a range of compelling strengths. (1) The unsaturated coordination conditions and distinct electronic structure can influence reactants, intermediates, and products’ adsorption/desorption behavior and intensity, thus enhancing catalytic efficiency and selectivity. (2) Theoretically, isolated SMACs have the highest atomic utilization efficiency, rapidly expanding operational locations and mitigating metallic usage. (3) SMACs can be regarded as a versatile stage for exploring the structure–activity relationship in heterogeneous catalysis at the atomic and molecular levels. (4) SMACs can ensure an even distribution of active sites while maintaining the stereostructural characteristic of heterogeneous catalysts, positioning this as an optimal approach for the sustainable advancement of catalytic reaction technologies.46,48,50–53
The utilization of SMACs as photocatalysts in the pNRR process has been extensively studied due to their numerous benefits. For example, Li and colleagues successfully developed a well-defined Pt–N3 single-atom catalyst (Pt SACs/CTF) by incorporating Pt into the N3 position within two-dimensional ultra-thin covalent triazine framework (CTF) nanosheets. This catalyst exhibited a notable photocatalytic efficiency of 170.4 μmol g−1 h−1 for ammonia production without sacrificial agents.54 A critical aspect of the pNRR process lies in developing effective photocatalyst materials. SMACs, known for their exceptional activity and controlled charge transfer pathways, were shown to enhance the effectiveness of photocatalytic nitrogen fixation for ammonia synthesis. The introduction of single-metal atom attachment on metal–organic frameworks via defects was demonstrated to significantly improve the efficiency of the pNRR process. Additionally, the interaction between single-metal atoms and carriers was highlighted as equally crucial for pNRR. Ren et al.55 illustrated this by creating defects within the UIO-66 (Zr) framework and introducing Ru single-atoms on the framework, establishing electron metal–support interactions (EMSI) through covalent bonds that enhanced charge transfer efficiency and substantially boosted photocatalytic yield. Researchers addressed the challenge of low reaction activity in the pNRR process through the selective design of catalytic materials, the construction of suitable active sites to lower the reaction activation energy, and the coupling of semiconductor band structures to enable efficient transfer of photogenerated charge carriers. This approach effectively improved the efficiency of pNRR for ammonia synthesis.
In this paper, we summarize the recent progress in research on applying single-metal atoms in pNRR. The basic principle of pNRR is introduced, and the mechanism of metal single atoms anchoring on carbon-based and non-carbon-based photocatalysts to improve nitrogen fixation performance is also discussed. Finally, the shortcomings and prospects of single-metal atom photocatalysts in pNRR are discussed.
2 The reaction process and mechanism of pNRR
2.1. Basic process of the pNRR
Before photocatalytic reactions occur, it is essential to transport N2 molecules from the external conditions to the catalyst's surface, where N2 interacts with the catalyst and adsorbs. By reducing the reaction energy barrier using a catalyst, ammonia synthesis can be catalyzed under the energy provided by light. The reaction process of pNRR to synthesize ammonia mainly involves the following steps: (1) when sunlight irradiates the photocatalyst, the energy of visible light (hν) is greater than the catalyst's band gap width (Eg), which excites electrons (e−) from the valence band to the conduction band (CB), creating positive holes (h+) in the catalyst, forming electron–hole pairs inside the catalyst;56–59 (2) some of the electron–hole pairs recombine inside or on the surface of the catalyst, while the rest diffuse to the catalyst surface under chemical potential and react with substances adsorbed on the surface in redox reactions. In the oxidation reaction, due to the valence band (VB) holes being strong oxidants (1.0–3.0 V vs. NHE), water combines with the holes and gets oxidized, producing protons and oxygen; the CB electrons act as strong reducing agents (0.5 to −1.5 V vs. NHE), which combine with N2 molecules and protons to be reduced by the photogenerated electrons, resulting in the generation of ammonia molecules.2,60–63 The produced ammonia eventually desorbs from the surface of the catalyst, diffusing externally into liquid or gas phase systems.
The occurrence of photocatalytic redox reactions is contingent on the reduction potential of the adsorbate and the positioning of the adsorbent, specifically, the band position within the semiconductors, as illustrated in Fig. 1.64–67 The conduction band of a semiconductor must possess a greater energy level than the reduction potential of N2, whereas the valence band should be lower than the oxygen evolution potential. The critical energy transition states were identified in the initial electron transfer (N2 + e− → N2 −4.16 V vs. NHE) and proton-coupled electron transfer (N2 + H+ + e− → N2H −3.2 V vs. NHE) processes, which significantly influence the overall kinetic reaction.16,68,69 These factors present primary obstacles that must be addressed to activate the N2 molecules for NH3 formation. It is imperative to maintain a small bandgap in semiconductors, ideally in the visible light range, as it can also meet the thermodynamic reduction potential of N2 to NH3.70–72 Additionally, it is imperative to inhibit the recombination of charge carriers within semiconductor photocatalysts to optimize the reaction's solar conversion efficiency and apparent quantum yield (AQY) of the response.
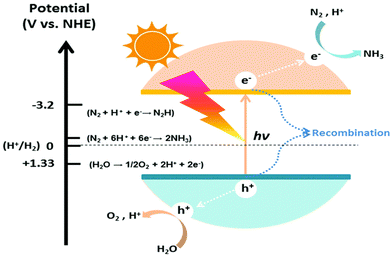 |
| Fig. 1 The schematic diagram of the photocatalyst for converting N2 to NH3 and its oxidation–reduction potential (V vs. NHE, pH = 0) is marked on the left side.16 | |
2.2 The mechanism of pNRR
This process could have followed either a N2 reduction reaction (NRR) or a N2 oxidation reaction (NOR). The pNRR process involves several steps. When exposed to light, the conduction band (CB) energizes electrons, creating holes in the valence band (VB). Some of these electrons and protons are released into the system. Meanwhile, the additional photogenerated holes (h+) facilitate the oxidation of water to produce H+ and O2 (eqn (1)). Simultaneously, the reduction of hot electrons leads to the production of NH3 from N2 (eqn (2)). Consequently, NH3 is formed under controlled conditions by utilizing sunlight as the energy source (eqn (3)). | Water splitting: 2H2O + 4h+ → O2 + 4H+ | (1) |
| Ammonia synthesis: N2 + 6H+ + 6e− → 2NH3 | (2) |
| Overall reaction: 2N2 + 6H2O → NH3 + 3O2 | (3) |
Fig. 2a illustrates the various mechanisms of N2 photofixation observed under alkaline conditions (pH 14). The primary emphasis of nitrogen fixation lies in converting N2 to NH3, which involves dissociative and associative pathways, and interaction of reaction intermediates.73–76 The dissociative way (Fig. 2a, path 1) involves the initial activation of the triple bond in the N2 molecule. This activation leads to the generation of two separate atoms on the surface of the catalyst that undergo individual protonation to yield NH3, mirroring the process used in conventional NH3 production methodologies. Given that the breaking of the N
N triple bond requires an energy of 9.79 eV (945 kJ mol−1), this specific pathway under consideration manifests a significant energy barrier for reactions, posing a formidable challenge in maintaining its presence on the surface of a catalyst under moderate environmental conditions.76,77
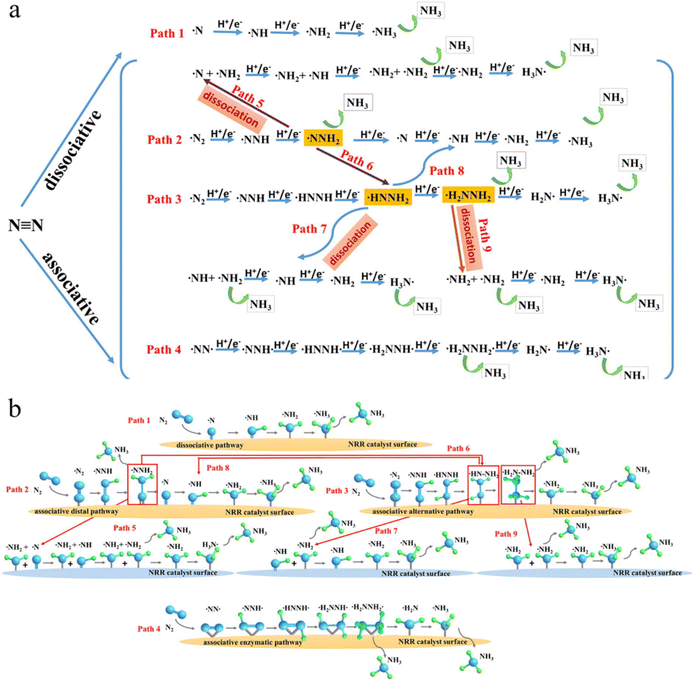 |
| Fig. 2 (a) Different NH3 evolution routes and their intermediates (b) their spherical and rod-shaped representations.84,85 | |
Implementing this procedure becomes particularly intricate when dealing with materials characterized by layered structures. In contrast to the dissociative system, the associative system operates akin to a chemical mechanism such as the enzymes involved in N2 fixation. This process involves gradually preparing adsorbed N2 molecules that link two atoms before forming NH3.78 The hydrogenation step in the associative approach can be broken down into associative distal pathways and other associative paths 2–8, as illustrated in Fig. 2, confirming the nitrogen-reduction reaction ways need an initial nitrogen adsorption configuration. Generally, there are two kinds of N2 adsorption patterns: side-mounted and end-mounted. The associative distal (Fig. 2a, path 2) and alternative associative pathways (Fig. 2a, path 3) are accessed through the side-mounted configuration. In contrast, the associative enzymatic route is achieved via the end-mounted configuration (Fig. 2a, path 4). The formation of N2 configurations proved to be intricate without the application of advanced in situ FTIR and first-principle computations that provided further insights into the adsorption configurations and mechanisms. The surface atoms of the catalytic adsorbent were found to predominantly participate in the hydrogenation process, leading to the formation of a distant NH3 molecule. Subsequently, a second NH3 molecule was generated as another atom that participated in the hydrogenation process (path 2). Alternatively, two atoms undergo hydrogenation through proton-coupled electron transfer resulting in the sequential liberation of two NH3 molecules in the final step (path 3). Remarkably, the hydrogenation of the intermediate *NNH2 (where * represents the N2 adsorption site) can occur through either the distal way or an alternative pathway leading to the formation of *HN–NH2 (path 6). Following this, in (path 3), the intermediate *HN–NH2 may proceed along the distal pathway, resulting in the formation of *NH with the release of an NH3 molecule (path 8). At potentials below 0.71 V, it is anticipated that the *NNH2, *NHNH2, and intermediate hydrazine (*NH2NH2) intermediates will dissociate, giving rise to several reaction paths (paths 5, 7, and 9). Back and Jung's calculations (Fig. 2a) have shown that the Watt and Chrisp technique was previously utilized for the identification of hydrazine synthesis or the comparison of Gibbs values of *HN–NH and *NH2NH2, potentially originating from the protonation of *N2H.78,79 Moreover, the enzymatic method (path 4) operates on a hydrogenation mechanism akin to the alternate pathway, where both atoms adhere to a catalyst surface in an end-mounted coordinating configuration, which is a rarity among heterogeneous catalysts. Li et al.79 explored an additional route known as the Langmuir–Hinshelwood (LH) mechanism. In brief, H2 was initially adsorbed onto the surface of a nitrogen fragment. For specific transition metal nitride (TMN) catalysts, the Mars–van Krevelen mechanism is advantageous for the electrolytic synthesis of ammonia. Furthermore, the atoms within the TMN lattice continually combine with proton–electron pairs (PEPs) to produce NH3 molecules, consequently producing vacancies on the surface.80–83 The vacancies were effectively absorbed chemically and stimulated N2 molecules to facilitate the ensuing nitrogen reduction reaction (NRR). Generally, the prediction of multiple electrons and the process of hydrogenation are deemed unfavorable because of the complexities of different bonding mechanisms and reaction pathways, which continue to pose challenges. Consequently, as illustrated in Fig. 2, nine alternative reaction pathways were investigated based on innovative mechanistic investigations conducted through first-principles calculations.
The dissociative process depicted in Fig. 2b, involving N
N triple-bond cleavage, requires a substantial amount of energy input as the initial step in the Haber–Bosch process. Two distinct associative mechanisms for N2 reduction have been proposed: distal and alternating pathways, each of which suggests a unique intermediate formation. In the alternating pathway, two hydrogen atoms are added sequentially to N2, resulting in the release of the first ammonia and the formation of a hydrazine-bound intermediate, as shown in Fig. 2b, after 4 hydrogenation steps. In contrast, within the distal route, a sole nitrogen atom experiences a series of three hydrogenation reactions to form the initial NH3 molecule, while the remaining nitride –N atom undergoes three hydrogenation processes to generate a second NH3 molecule. The examination of thermodynamics in pNRR can be classified into 2 distinct types: whole-reaction and half-reaction thermodynamics, both of which remain unaltered by the presence of a catalyst. However, photon-induction mechanisms rely on specific photocatalysts. Comer and colleagues86 undertook a comprehensive thermodynamic study of prevalent nitrogenous substances through the utilization of explicit entropy and enthalpy to evaluate the viability of diverse N2 fixation techniques. It is possible to estimate the free energy and anticipate the initial potential of the reaction by determining the adsorption energy and vibrational energy of the potential intermediates formed during N2 reduction. In the photocatalytic process, various constraints are imposed by photocatalysts. Achieving a reduction potential of 4.2 V vs. NHE is essential to disrupt N2 bond breakage on the photocatalyst surface, which is a task that proves challenging for most semiconductors. Conversely, many electrical strategies offer more practical alternatives.87,88
Additionally, the conversion of N2 into a singular nitrogen molecule, such as ammonia or nitrate, is relatively straightforward in terms of thermodynamics. Conversely, converting diazo compounds into single nitrogen compounds presents specific challenges. Nitrogen fixation, classified as a reduction or oxidation reaction, may produce nitrogen reduction and nitrate oxidation products when titanium-based photocatalysts are used under ambient conditions.89 Consequently, discerning the predominant oxidation or reduction processes becomes complex. The transformation of redox products involves a temporal dimension, leading to complex pathways between oxidation and reduction reactions. Several studies have documented the oxidation of NH3 under room conditions. Photocatalysis in such settings may aid in the oxidation of reduced products, as the shift from reduced to oxidized forms requires a driving force. Nonetheless, this process requires lower voltage and thermodynamic energy than traditional nitrogen fixation techniques.90,91
In proton environments, the hydrogen evolution reaction (HER) constantly challenges the nitrogen reduction reaction (NRR). Certain intriguing materials exhibiting significant nitrogen catalytic capabilities are posited to possess hydrogen binding energies approaching the optimal threshold for facilitating hydrogen evolution, thus potentially aiding the facilitation of competitive HER processes. Consequently, in the pursuit of metal co-catalysts for nitrogen reduction Norskov92 group investigated the correlation between the catalytic efficacy of N2 reduction and intermediate binding energies, employing density functional theory (DFT) to scrutinize FCC metals. Notably, the HER displays a higher positive limiting potential than the NRR.93,94
The limitation potential discrepancy between the HER and NRR is less pronounced on the (111) facet than on the (211) facet. A significant potential difference exceeding 0.4 V was observed between these two reactions, indicating a level of selectivity in the HER process (Fig. 3). It is crucial to impede the HER while advancing N2 reduction catalysts. The critical step is the weakening of the metal-to-nitrogen bond during the reductive adsorption of N2 to *N2H. The reaction rate is determined by either the hydrogenation of *NH to *NH2 or the transformation of *NH2 to NH3, contingent on the strength of the metal-to-nitrogen bond. An effective heterogeneous nitrogen reduction catalyst should exhibit strong nitrogen affinity, facilitating rapid nitrogen reduction while maintaining a low affinity for intermediate species.
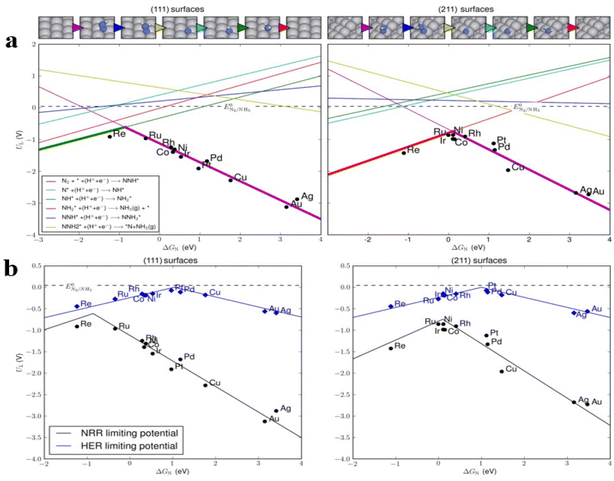 |
| Fig. 3 (a) The restriction of the achievable NRR is examined about the binding energy of *N on (111) and (211) crystallographic planes of different transition metal substrates; (b) a scholarly comparison was conducted analyzing the potential volcano plots’ limiting potential of HER (depicted in blue) and NRR (depicted in black) on the (111) and (211) surfaces across diverse transition metals.92 | |
3 Preparation process and formation mechanism of SMACs
This study focuses on preparing SMACs based on semiconductive supports, given detailed coverage of the various synthetic approaches for SMACs in the existing literature.95–97 The vulnerability of active sites in SMACs to relocating and forming clusters or nanoparticles due to high surface energy poses challenges in stabilizing them at high loading levels. To address this, innovative methods for creating SMACs have been briefly outlined.
3.1 Wet chemical method
Wet chemical methods employing uncomplicated processes hold potential for the large-scale synthesis of SMACs. Typically, these methods involve two processes to attach SMAs to the catalyst support. In the liquid phase, the hydrothermal method is employed to affix metal atoms to the support or reduce metal ions to individual atoms. The fabrication of SMACs involves utilizing freeze-drying techniques and gas-phase reduction methods. For example, Li et al.98 devised an N-coordination approach for creating Fe–N5 sites uniformly dispersed on N-doped porous nanofibers (Fe SA/PNCNFs-0.1) as a high-performance catalyst through a wet chemical synthesis method (Fig. 4a). In detail, porous N-doped carbon nanofibers (PNCNFs) were meticulously crafted through a series of electrospinning, oxidation, and pyrolysis procedures. Subsequently, integrating the Fe source onto PNCNFs facilitated thorough contact between the fiber matrix and Fe species. This was achieved by preabsorbing PNCNFs onto Fe species through a wet impregnation method. The decomposition of Fe acetylacetone at elevated temperatures resulted in the localization of Fe on nitrogen-doped carbon, with certain Fe species being reduced to a zero valence in the presence of carbon. To eliminate the Fe particles, HCl etching was performed meticulously. However, the nanoparticles that developed within Fe SA/PNCNFS-0.4 at an elevated impregnation level underwent increased etching, resulting in a reduced presence of single atoms.
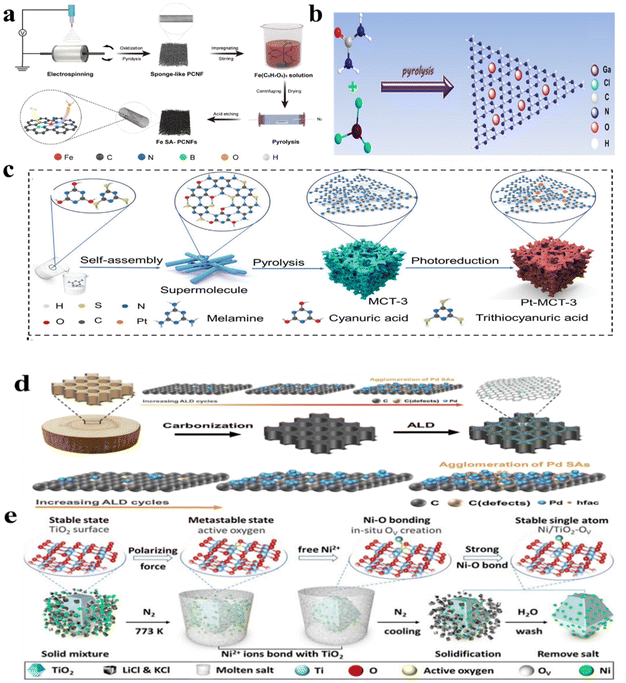 |
| Fig. 4 (a) Schematic of the structure of Fe SA/PNCNFs-0.1;98 (b) schematic of Ga-doped C3N4 single-atom photocatalyst;99 (c) schematic of the preparation process of Pt-MCT-3;100 (d) schematic of preparation CW-Pd;101 (e) schematic of Ni atoms modified TiO2.102 | |
3.2 Pyrolysis method
The pyrolysis method served as a crucial approach for producing single-atom photocatalysts by heating a combination of metal and organic semiconductor precursors at elevated temperatures. Establishing a robust coordination bond between the metal centers and the organic precursor is typically necessary to prevent the aggregation of isolated metal sites during the pyrolysis process. For instance, Jiang et al.99 successfully incorporated Ga (0.014–0.045 wt%) into g-C3N4 through the direct pyrolysis of a mixture of GaCl3 and urea at 550 °C (Fig. 4b).
3.3 Photodeposition method
The advantage of photodeposition lies in its ability to produce high-quality thin films with complex structures, while controlling various parameters such as thickness, composition, and microstructure. It demonstrates unique advantages in the synthesis of single-atom catalysts. Liu et al.100 developed a hybrid system by combining nitrogen defects with single Pt atoms, achieved through a straightforward self-assembly and photodeposition approach, named Pt-MCT-3. The MCT precursors were produced through the in situ self-assembly process involving trithiocyanuric acid, melamine, and cyanuric acid. A specific volume of chloroplatinic acid solution was then added dropwise into the solution of MCT-3. After light deposition for 30 minutes, an efficient single-metal atom photocatalyst Pt-MCT-3 was obtained (Fig. 4c).
3.4 Other methods
In addition to the methods outlined above, alternative strategies were devised for the production of SMACs. Atomic layer deposition (ALD) presented a precise technique for synthesizing such photocatalysts by sequentially depositing single metal sites onto semiconductor surfaces in a controlled manner. Zhang et al.101 deposited Pd single atoms onto carbonized wood (CD) using ALD. By heating the Pd(hfac)2 precursor to approximately 85 °C, the desired vapor pressure was achieved. Deposition was conducted at 200 °C, with the ALD delivery lines maintained at 100 °C. Nitrogen was used as a purging and carrier gas throughout the deposition process, resulting in the creation of an efficient SMAC photocatalyst (Fig. 4d). Xiao et al.102 successfully achieved the precise incorporation of single Ni atoms onto the TiO2 photocatalyst using a controllable molten-salt method (MSM). This method provided ample ionized anions and cations with a strong polarizing force to disrupt covalent, ionic, or metallic bonds within the molten salt environment (Fig. 4e). The molten salts, comprising LiCl and KCl, created a liquid medium and spatial constraint that enabled the isolated dispersion of Ni species and facilitated the formation of robust Ni–O bonds on TiO2. Moreover, chemical etching was employed as a method to fabricate single-atom photocatalysts with a substantial metal content.
4 SMACs for pNRR
The physical and chemical characteristics of a catalyst undergo a significant transformation when the dispersion of the doping metal is heightened to an individual atomic level, leading to the emergence of a distinct class of catalysts known as single-metal atom catalysts (SMACs).103–106 Recently, SMACs have demonstrated remarkable stability, targeting activity, selectivity, and reactivity, making them prospective candidates for applications in the pNRR. Recent studies have illustrated the superior photocatalytic performance of SMACs in the pNRR, achieving a notable atomic efficiency.107–117 Notably, SMACs containing a singular metal atom readily integrate into the matrix carrier, facilitating interactions with specific external species at the unimpregnated coordination sites. Strong covalent or ionic bonds between the matrix and metal atoms prevent the agglomeration or dissolution of SMACs. The remarkable catalytic efficiency of SMACs can be ascribed to their low coordination number, a characteristic that promotes the adsorption and activation of dinitrogen on the catalytic sites. Furthermore, the adsorption characteristics of the substrate can be regulated by changing the electronic states caused by charge transfer. Varied coordination structures of metal atoms yield distinct free energies during reactions, enhancing selectivity and reaction efficiency. Currently, SMACs exhibit promising prospects for advancement in the domain of pNRR. Here, we discuss the NRR activity of diverse SMACs dispersed on various matrices.
4.1 Single-metal atoms supported on a carbon-based substrate for pNRR
Carbon-based materials exhibit appealing band structures, robust chemical stability, high abundance on the Earth, and ease of production, rendering them well suited for photocatalytic nitrogen fixation. Diverse carbon-based materials, such as graphene oxide (GO) featuring oxidative functional groups, graphene and its derivatives such as graphene quantum dots (GQD), GO, reduced graphene oxide (rGO), graphitic nitride carbon, N-doped carbon, reduced graphene oxide, cellulose, graphene diyne, carbon nanotubes (CNTs), and ultra-thin carbon nanosheets,34,118–123 among others, have demonstrated exceptional performance, establishing them as ideal nanocomposite materials. When dispersed in the matrix and carbon nanotubes, they exhibit outstanding electron transfer efficiency, cost-effectiveness, stability, and environmental friendliness, even in acidic and alkaline environments. Augmentation of SMAC active sites with a larger surface area can enhance ammonia production by boosting light absorption for effective fixation.124–128 Moreover, carbon substrates facilitate electron guidance to metal atoms by improving the contact of surface-active sites, thereby improving N2 adsorption sites and subsequently increasing NH3 production.129
4.1.1 Single atoms of noble metals on a carbon substrate for pNRR.
Recently, metals like Ru,108,130–132 Pd,133,134 and Ag
135,136 have been employed in contemporary endeavors aimed at facilitating environmentally friendly ammonia synthesis processes. Li et al.131 successfully anchored Ru atoms onto nitrogen-doped carbon substrates containing cyanide defects. Through a one-pot calcination process, Ru single atoms were loaded onto a g-C3N4 catalyst, demonstrating remarkable performance in the NRR with a value of 125.5 μmol gcat−1 h−1. The experimental results indicated that incorporating single-atom Ru reduces the band gap of carbon nitride, expands the photoresponse range of catalysts, and enhances its absorption capacity.
Similarly, Ding et al.132 synthesized Ru/MOF/C3N4 bifunctional catalysts in situ, which not only demonstrated success in the NRR but also in the CO2RR. Initially, C3N4 sheets were introduced into a Zn(NO3)2·6H2O solution containing ligands such as 2,5-dihydroxyterephthalic acid, DMF, and H2O to effectively attach Zn ions onto the C3N4 surface, promoting the homogeneous separation of MOFs during the in situ synthesis procedure. Subsequently, RuCl3 with unsaturated coordination sites was incorporated into the metal–organic framework (MOF) possessing unsaturated coordination sites. This allowed Ru ions to coordinate with the unsaturated sites on the MOF, immobilizing the dispersed Ru to produce a single Ru atom (Fig. 5a). Theoretical calculations using DFT demonstrated that a singular Ru atom generates energy levels within the bandgap of the MOFs, thus hindering electron–hole complex formation and augmenting the abundance of photocarriers. Furthermore, the researchers conducted Gibbs free energy calculations for each intermediate product in various models of distinct reaction pathways, showing a decrease in the Gibbs free energy of the NRR in both the distal and alternating mechanisms (Fig. 5b and c).
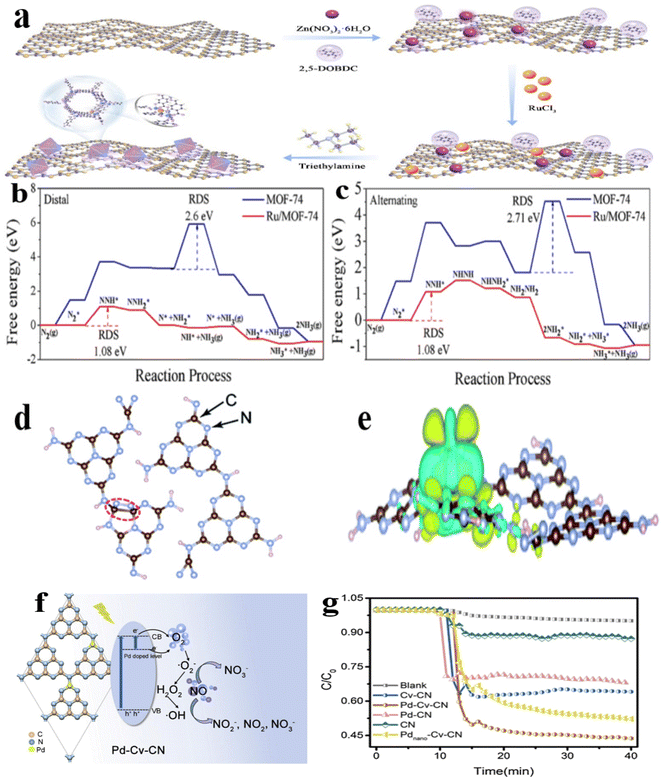 |
| Fig. 5 (a) Diagrams of the synthesis of Ru/MOF/C3N4; (b and c) free energy diagrams were calculated for the photocatalytic NRR processes, specifically for pNRR (Distal) and pNRR (Alternating) pathways;132 (d) adsorption configuration and (e) the charge density contrast observed in an N2 molecule adhered to an N2C NV site on a PCN surface is depicted by yellow and light blue isosurfaces, denoting areas of charge accumulation and depletion in three-dimensional space. The specific charge density value measured is 0.0025 elementary charges per cubic angstrom.137 (f and g) A photocatalyst comprising g-C3N4 adorned with single Pd sites was investigated for its efficacy in eliminating NOx pollutants.138 | |
Gold's distinctive electronic configuration, which facilitates effective charge transfer and exhibits low reactivity towards hydrogen, which inhibits the hydrogen evolution reaction, has established it as a promising catalyst contender in the pNRR. Studies have demonstrated that gold nanoparticles or clusters exhibit remarkable pNRR performance. In line with this, Guo et al.137 used an etching method to uniformly embed Au nanoparticles into the mesopores of nitrogen-deficient hollow nitrogen-doped carbon spheres to form a plasma hybrid catalyst. Incorporating Au nanoparticles within the mesoporous shell of nitrogen-doped carbon spheres significantly improved the absorption of visible light, leading to the generation of hot electrons for N2 reduction while effectively suppressing carrier recombination. This design promoted concurrent oxidation and reduction reactions within the system. 7.4 wt% of gold embedded in nitrogen-doped carbon spheres achieved a NH3 yield rate of 783.4 mmol h−1 gcat−1 under visible light. Theoretical investigations have indicated that N2 molecules exhibit favorable capture and adsorption properties when interacting with NVs at the N2C site of PCN in a dinuclear end-on coordination configuration, resembling the coordination of N2 bound to metal complexes (Fig. 5d). The discrepancy in charge density was computed to assess the electron transfer dynamics between the nitrogen vacancies (NVs) and the N2 molecules adhered to them. The electrons residing at the carbon atoms neighboring the NV locations are shifted to the adsorbed nitrogen molecule, thereby triggering the activation of the N
N triple bond (Fig. 5e). Scholars have extended their investigations from Ru- and Au-based systems to Pd-based systems, thereby broadening the horizons of catalyst research. Liu et al.138 utilized urea as a precursor to immobilize Pd atoms on g-C3N4 carriers embellished with adjustable carbon defects, resulting in the formation of Pd–N3 active sites (Fig. 5f). These sites demonstrated superior and consistent performance in the photocatalytic removal of NO, surpassing the original g-C3N4 by 4.4 times (Fig. 5g).
Steady-state.
Silver (Ag), a noble metal, is extensively used in pNRR. Wang et al.136 conducted a study using first-principles research to elucidate the mechanisms underlying the improvement of pNRR performance. In their study, silver single atoms were evenly distributed on N-doped graphene carbon (Ag/g-C3N4) synthesized via a straightforward hydrothermal process employing block-like cyanamide (CN). Subsequently, using reduction deposition, a one-pot technique was employed to make uniformly dispersed Ag/g-C3N4 as a highly effective N2 photofixation catalyst at extremely low temperatures (Fig. 6a). When the loading amount of Ag was 4%, the catalytic activity of ammonia was the highest (1.45 mmol L−1 gcat−1 h−1), which was nearly 15 times higher than the original CN value (Fig. 6b). Theoretical investigations have indicated that the increase in photocatalytic activity can be attributed as follows: (1) the small silver particles exhibit a reduced recombination rate of the photogenerated electron–hole pairs in comparison with their larger counterparts, owing to the abbreviated transfer distance within their structure to the Ag–CN interface. This proximity facilitates enhanced contact between the small Ag particles, improving charge-separation efficiency. (2) Small Ag particles offer the advantage of exposing supplementary active sites. Furthermore, incorporating Ag as a cocatalyst enhances the disjunction of photogenerated electron–hole pairs, thereby diminishing the potential for NH2 production and bolstering its catalytic generation (Fig. 6c).
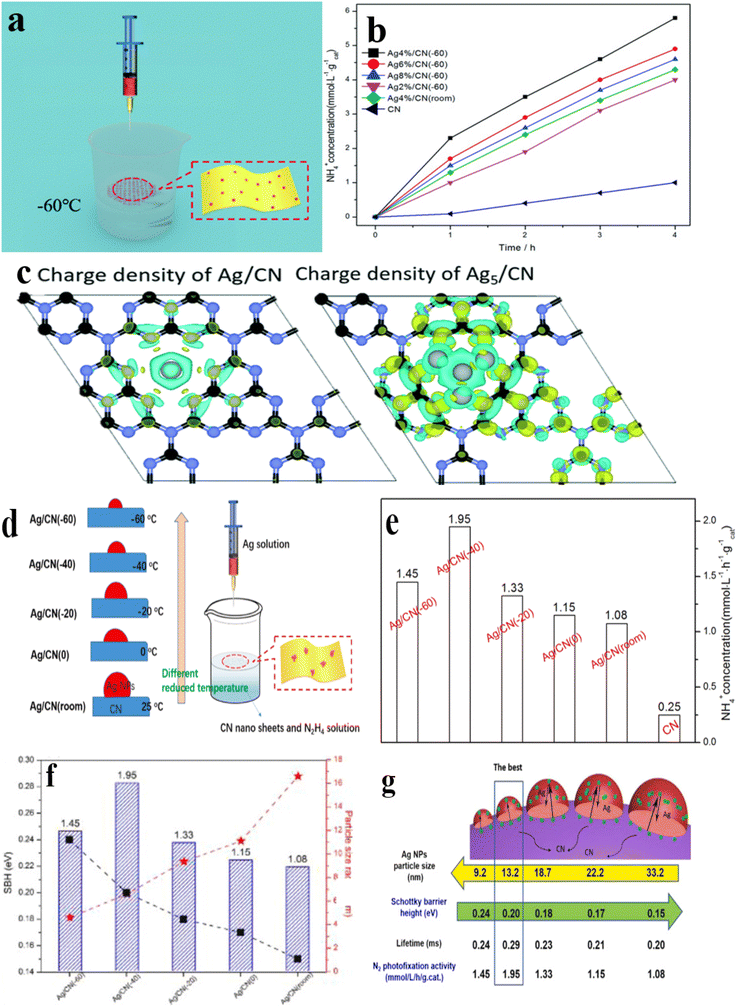 |
| Fig. 6 (a) Illustration of the synthesis of Ag/g-C3N4. (b) NH3 production rate graph with different Ag/CN; (c) the yellow denotes charge accumulation and cyan denotes charge depletion;136 (d) illustration of the synthesis of Ag/g-C3N4 at different temperatures; (e) NH3 production rate at various temperatures; (f) the impact of particle size on both the catalytic SBH value and the photocatalytic performance is under examination; (g) the size of Ag nanoparticles can be controlled, leading to the establishment of an inherent electric field between silver and C3N4 material.139 | |
Similarly, in Li et al.139 the deposition of silver particles with an average diameter of 9.3–33.2 nm on N2 photofixed catalyst g-C3N4 was studied using the ultra-low temperature reduction deposition method (Fig. 6d). The Ag/CN (−40) exhibited the best NH3 precipitation rate (1.95 mmol L−1 gcat−1 h−1) (Fig. 6e). The researchers delved into the mechanism of this process and observed that as the preparation temperature increased, the size of the silver particles on g-C3N4 increased gradually, while the work function and Schottky barrier height (SBH) value decreased. Larger silver nanoparticles led to a smaller SBH, facilitating interactions between silver and g-C3N4 at the interface. As the dimensions of the silver particles enlarged, the electrons and (or) holes found themselves confronted with a lengthier journey to interact with the photocatalyst's surface. This discrepancy in the propulsive force for extended-range movement heightened the likelihood of charge recombination, thus leading to a decline in the effectiveness of the photocatalytic process (Fig. 6f and g).
4.1.2 Single atoms of non-noble metals on a carbon substrate for pNRR.
Apart from noble metal SMACs, non-noble metals like Fe, Mo, Co, and Cu have recently gained attention as photocatalysts for pNRR under environmental conditions. The shift from precious to non-precious metal catalysts presents a compelling opportunity to broaden the realm of catalyst exploration. Notably, Fe stands out for its positioning at the apex of the volcano plot based on DFT, highlighting its potential as a promising transition metal species. Building on this premise and drawing insights from nitrogenase, scientists42 have successfully fabricated Fe porous g-C3N4 (FPx) by dispersing a solitary iron atom onto nitrogen-doped carbon with a porous structure that exposes more active sites. This unique design not only alters the adsorption mechanism of N2 from physical to chemical but also switches the pNRR pathway from the associative distal pathway to the associative alternating pathway (Fig. 7a). The synthesis of the single-atom iron porous g-C3N4 (FPx) specimen involved a one-step annealing process using NH4Cl to generate gaseous NH3 and HCl as bubble templates, facilitating the direct atomization of Fe particles to form isolated Fe sites (Fig. 7b).
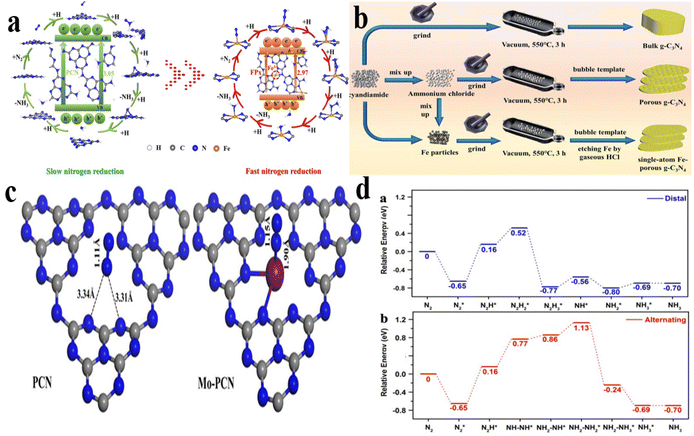 |
| Fig. 7 (a) Demonstration of the amalgamation process of FeSA/NC; (b) comparison of accelerated nitrogen reduction with decelerated nitrogen reduction;42 (c) optimized N2 adsorption model on pure PCN and Mo-PCN; (d) N2 reduction catalyzed by Mo-PCN through distal and alternating mechanisms.140 | |
Scholars have recently explored deeply the possibilities of molybdenum-based systems in catalysis following the advancements in iron-based SMAC. In light of the coexistence of Fe and Mo metal centers within nitrogenase, numerous studies have strived to integrate molybdenum atoms into porous carbon frameworks as a means to enhance the development of NRR catalysts. Guo and colleagues140 synthesized a collection of Mo single-atom catalysts through the calcination of economically feasible urea as the primary precursor, in conjunction with different quantities of Na2MoO4·2H2O. The individual Mo center exhibited coordination with two nitrogen donors, resulting in the formation of a two-coordinated MoN2 species, which was immobilized on the poly-nitride carbon structure formed in situ. The low coordination Mo centers can be used as active sites for N2 chemical adsorption and activation, realizing high photocatalytic activity for NH3 release in pure water. Experimental studies and calculations using density functional theory have shown that the coordinatively unsaturated metal site within single-atom catalysts exhibits a high affinity for N2 molecules, facilitating strong adsorption through an end-on configuration. This interaction leads to the elongation of the N
N bond from 1.11 to 1.15 Å (Fig. 7c). Consequently, photoexcited electrons can transfer to the weakened N
N bond, enhancing the efficacy of nitrogen fixation in natural settings. The analysis presented in Fig. 7d indicates that in both distal and alternating mechanisms, the crucial step governing the rate of the process is the conversion of N2 to N2H*, characterized by an endothermic nature with an energy barrier of 0.81 eV. These findings suggest the coexistence of two distinct reaction pathways during the photocatalytic N2 fixation process.
Liu and colleagues conducted an extensive DFT investigation, employing the N
N dipole within the adsorbed N2 as a metric to assess the efficiency of catalytic sites in the catalyst for NRR.141 The study revealed that employing 2D phthalocyanine (2D Mo–Pc) to support Mo single atoms led to superior performance compared with other transition metals. The enhanced performance observed can be ascribed to the dominant dipole moment produced within the N
N triple bond due to the interaction between Mo and N atoms. This dipole moment effectively promotes the migration of d electrons from individual Mo atoms to the π* anti-bonding orbitals of the N2 molecule, thereby enhancing the reaction. Additionally, the reduced affinity of Mo with hydrogen minimizes the competing HER, consequently improving the selectivity of the 2D Mo–Pc catalyst towards the NRR.
Theoretical studies have underscored the importance of employing single atoms of transition metals belonging to the 3d, 4d, and 5d series on two-dimensional rectangular monolayers of tetracyanoquinodimethane (TM-rTCNQ) for NRR. Among these transition metals, Mo, Tc, and W have been identified as promising SMACs due to their remarkable structural stability and high selectivity, rendering them favorable for NRR applications.142 Significant adsorption and activation of N2 were discovered for all three SMACs. The observed phenomenon can be ascribed to the augmented orbital hybridization and charge transfer mechanisms, which are fostered by the interplay between the catalyst and nitrogen species. Through a wide evaluation process, the Mo-rTCNQ monolayer was determined to be the most effective SMAC for catalyzing NRR, showcasing a distal mechanism. Tetracyanoquinodimethane has the potential to be fluorinated to produce tetrafluorotetracyanoquinodimethane (F4TCNQ), which serves as a promising organic charge transfer material and robust π electron acceptor. Researchers conducted a detailed investigation on the viability of a single transition metal atom (TM = 3d–5d series transition metal atoms) incorporated into a rectangular F4TCNQ (M-rF4TCNQ) monolayer for the pNRR using advanced first-principles high-throughput screening calculations.143 Among the identified four SMACs capable of catalyzing the NRR (M-rF4TCNQ, where M = Ti, Mo, Nb, or Tc), their efficacy was considered to be the robust adsorption and activation of N2, primarily arising from the “acceptance–donation” interaction between the transition metal and the adsorbed nitrogen molecule. The research demonstrated that Ti-rF4TCNQ could efficiently promote the NRR process through an enzyme-like mechanism. Moreover, Mo-rF4TCNQ was also recognized as a feasible SMAC for NRR, facilitating the reaction through a distal pathway.144
Loading cobalt single atom has occurred as a viable method to enhance the catalytic performance of semiconductors, thereby facilitating the efficient separation of electron–hole pairs generated during photoinduced reactions and providing essential sites for nitrogen reduction. This approach is crucial for achieving optimal energy synergy in catalytic processes.145,146 Recently, Wang et al.147 employed H3PW12O40 (PW12) incorporated with ZIF-67 as a starting material to synthesize nitrogen-doped graphitic carbon (NGC) nanocages hybridized with tungsten carbide and cobalt nanoparticles (WC–Co/NGC) through a calcination process (Fig. 8a). The NH3 production rate (142 μmol g−1 h−1) of WC/Co–NGC-2 under visible light irradiation was approximately six times higher than that of Co–NGC with WC unloaded (Fig. 8b). The advancement observed in the pNRR process can be attributed to the inclusion of WC particles and the cooperative influence arising from the interaction between WC and Co. The 3D nanocages exhibited a high specific surface area and an appropriate band structure location, contributing to efficient N2 fixation (Fig. 8c).
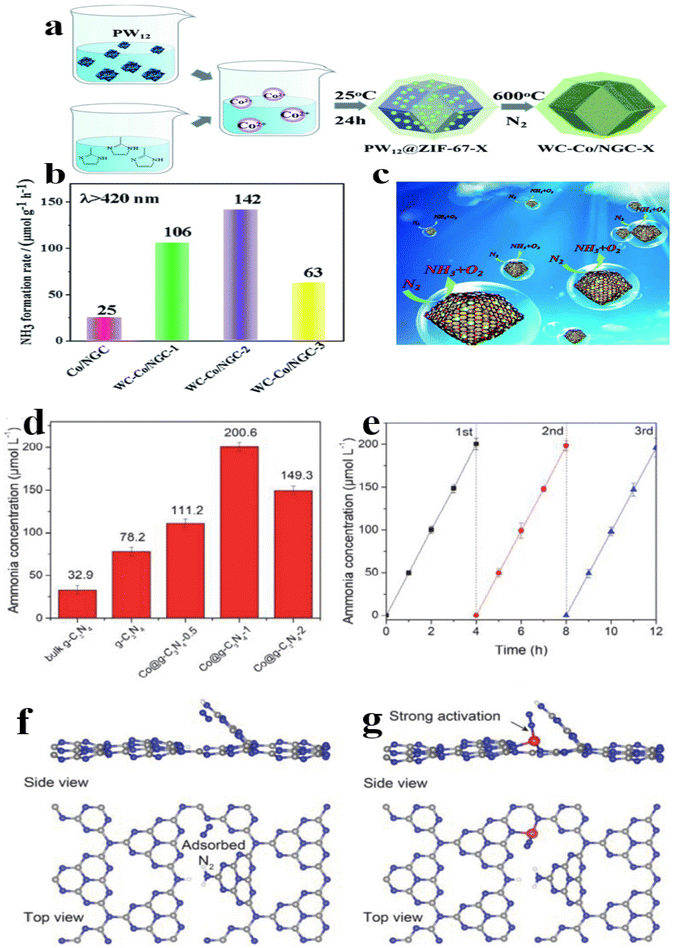 |
| Fig. 8 (a) The synthetic procedure of WC–Co/NGC; (b) the rate of ammonia formation through photocatalysis, and (c) a schematic illustration depicting the process of photocatalytic nitrogen fixation utilizing WC–Co/NGC is presented;147 (d) ammonia yield across various samples; (e) cycling measurements were conducted to assess the pNRR performance of Co@g-C3N4-1 nanosheets;(f–g) The adsorption geometry of nitrogen molecules on pristine g-C3N4 and cobalt-decorated g-C3N4, denoted as Co@g-C3N4-1 was investigated.148 | |
In another work by Fu et al.,148 a molecular scaffolding approach was utilized to immobilize and support single-site Co on porous crimped g-C3N4 (Co@g-C3N4), proposing these as highly efficient photocatalysts for solar-photon-driven N2 fixation under ambient circumstances. As revealed in Fig. 7d, it was observed that the bulk g-C3N4 showed a significantly low N2 fixation activity of 8.2 μmol h−1, while the supramolecular synthesized g-C3N4 showed a higher activity of 19.5 μmol h−1. Upon integrating single cobalt sites, the optimal activity of Co@g-C3N4-1 reached 50.2 μmol h−1. Notably, the catalyst displayed good stability over 3 cycles under severe nitrogen fixation circumstances with visible light irradiation (Fig. 8e). DFT calculations were performed to identify the theoretically catalytically active sites. Intriguingly, the coordinated cobalt site emerged as the active center with a robust adsorption energy of 0.99 eV compared with bare g-C3N4 (Fig. 8f and g).
The utilization of copper (Cu) catalyst in the photocatalytic reduction of nitrogen gas for producing green ammonia under mild conditions and atmospheric pressure has been extensively investigated. However, the application of single-atom copper in the NRR process remains relatively limited in current research. Huang et al.149 successfully synthesized a copper single-atom catalyst by modifying copper on g-C3N4. The resulting Cu–CN material demonstrated a notable concentration of active Cu–N2 sites (Fig. 9a). X-ray absorption fine structure (srXAFS) provided additional evidence supporting the presence of a solitary Cu atom configuration within Cu–CN (Fig. 9b). Notably, the ammonia production rate of copper-altered carbon nitride (Cu–CN) when subjected to visible light in pure water was recorded at 186 μmol g−1 h−1 (Fig. 9c); DFT simulations indicated that an individual copper atom can effectively extract paired electrons from the extended π electron system of p-CN, with the majority of these limited copper atoms likely to form associations with the T-defect vacancies within the polymerized carbon nitride structure (Fig. 9d).
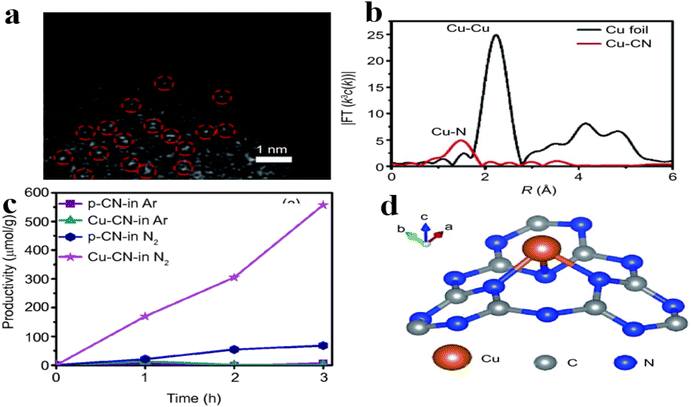 |
| Fig. 9 (a) HAADF-STEM image of Cu–CN; (b) Cu foil and Cu–CN FT-EXAFS spectra were analyzed; (c) quantitative determination of NH3 generated under visible light; (d) the suggested arrangement of copper cyanide within a T-shaped defect (illustrated in color online) is being proposed.149 | |
Alkaline-earth metal elements located in the s-block of the periodic table have received limited attention as potential active sites for nitrogen (N2) photofixation. A recent study by Pan et al.150 focused on the synthesis of alkaline earth metal single atom-modified mesoporous g-C3N4 materials (including Ca, Mg, Sr, Ba) via calcination and continuous stirring techniques (Fig. 10a). Notably, the research found that the 0.5 Ca/m-g-C3N4 catalyst exhibited superior performance, generating 42.23 μg gcat−1 h−1 of NH3 (Fig. 10b). Furthermore, nitrogen-programmed temperature desorption experiments (N2-TPD) revealed that atomically dispersed Ca acted as the active site on Ca/m-g-C3N4, demonstrating efficient N2 molecule adsorption. The isolated Ca atoms not only functioned as the primary center for N2 activation but also optimized the band structure of m-g-C3N4, thereby enhancing the photocatalytic production of NH3 (Fig. 10c). The reduction mechanism of N2 molecules predominantly involves binding via remote pathways, where N2 adsorption during the photoinduced nitrogen reduction reaction occurs with concurrent chemical transformations. Specifically, N2 molecules along the distant pathway are adsorbed in a terminal configuration on Ca single-atom sites, leading to a weakening of the N
N bond (Fig. 10d).
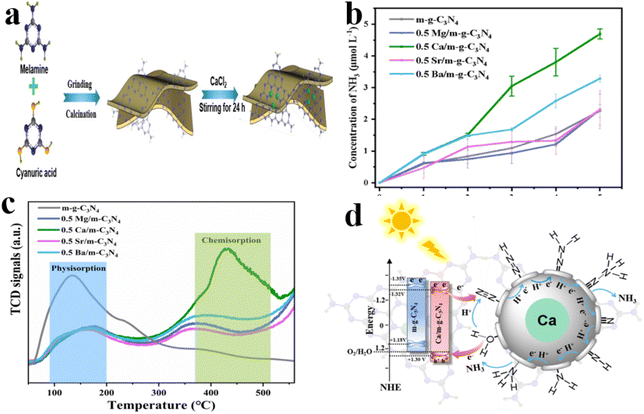 |
| Fig. 10 (a) Schematic illustration for the preparation of Ca/m-g-C3N4; (b) different alkaline earth metal NH3 production; (c) N2-TPD spectra of the as-prepared different photocatalysts; (d) the band structure and pNRR pathway of continuous hydrogenation process on Ca active sites of 0.5 Ca/m-g-C3N4.150 | |
4.2 Single atoms of non-noble metals on a non-carbon substrate for pNRR
The exploration of single-atom catalysts on non-carbon substrates for NRR has been a prominent area of research. While doped carbon-based substrates are typically utilized for dispersing single-metal atoms, non-carbon substrates are essential for controlling the dispersion of metal atoms and influencing the NRR process. This section delves into the theoretical and practical aspects of NRR photocatalysts that employ single-metal atom catalysts supported on non-carbon materials, elucidating their significance and mechanisms in detail.
4.2.1 Metal oxides as supports.
Transition metals such as Fe, Co, Ti, and Ni and their respective oxides in the Earth's crust represent cost-effective substrates suitable for implementation as catalysts across various catalytic applications. These include, but are not limited to, the facilitation of the oxygen reduction reaction, nitrogen reduction reaction, hydrogen evolution reaction, oxygen evolution reaction, and hydrogenation processes.144 Notably, nanostructured mesoporous TiO2 exhibits favorable characteristics such as a substantial surface area and porous structure, which can significantly enhance various catalytic processes as well as improve the efficacy of adsorption and separation mechanisms.
By employing the molten salt technique for the incorporation of Ru single atoms into TiO2, the electronic metal–support interactions (EMSI) established between Ru single atoms and TiO2 play a crucial role in stabilizing oxygen vacancies. This stabilization facilitates the activation and adsorption of N2 molecules for NRR151 (Fig. 11a). Remarkably, a notable NH3 production of 18.9 μmol g−1 h−1 is attainable under gentle conditions without the need for sacrificial agents. The collective findings from empirical observations and theoretical computations indicate that the doping of Ru exhibits the optimal synergistic effect between OVs, thereby facilitating the concurrent adsorption, N2 activation, and electron transfer to N2. In the associative distal pathway context, the initial hydrogenation of proton and electron pairs occurs on the N atom that is distant from the active sites, yielding ammonia. Conversely, in the associative alternating mechanism, these pairs interact with N atoms on opposing sides in an alternating fashion. Within the Ti–VO–Ti site, the transition from *N2 to *N-NH presents the most substantial energy barrier at 0.69 eV among all involved steps, establishing the transformation of *N–N to *N-NH as the pivotal rate-limiting stage. The hydrogenation of *N-NH encompasses two distinct procedures, namely the formation of *NNH2via the distal pathway and *NH–NH through the alternating pathway. The energy changes (ΔG) associated with NH–NH* and NH2* generation are recorded at −0.37 and −0.08 eV, respectively. Hence, the preference for N2 molecule hydrogenation via the associative alternate pathway at the Ti–VO–Ti site is evident. Following the introduction of Ru, the transition from *N–N to *N–NH emerges as the controlling step with an energy barrier of approximately 0.42 eV (Fig. 11b and c). Furthermore, EMSI plays a role in modulating the local electronic structure by facilitating electron transfer from the Ru atom to TiO2, consequently diminishing the energy barriers of rate-limiting steps and promoting the activation of N2 and subsequent hydrogenation reactions.151
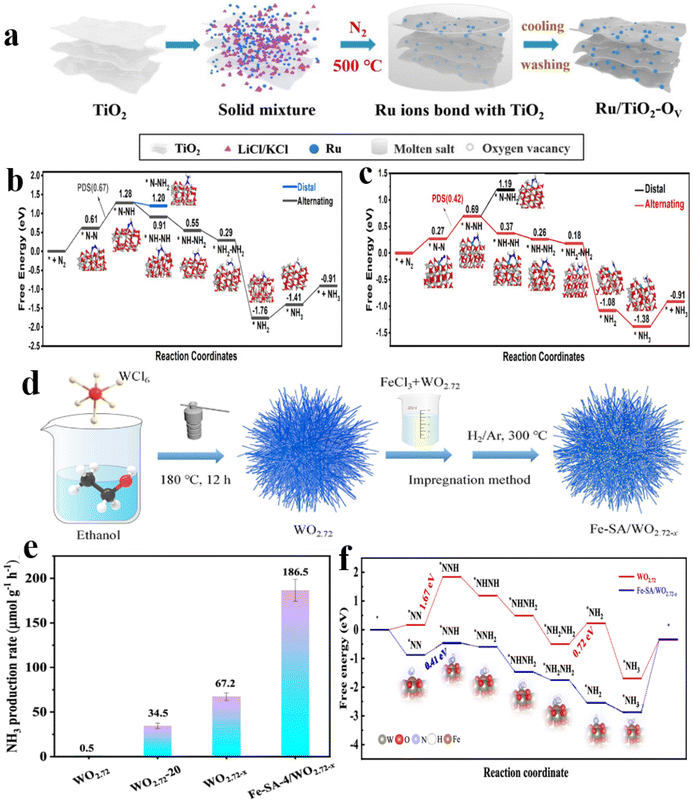 |
| Fig. 11 (a) Schematic illustration for the preparation of Ru/TiO2-VO; (b) the free energy diagram depicting the NRR on a TiO2-VO catalyst, along with the corresponding atom configurations; (c) the free energy diagram depicting the NRR occurring on a Ru1/TiO2-VO catalyst, accompanied by the corresponding atomic configurations denoted by distinct colors: Ti in gray, Ru in green, O in red, N in blue, and H in white;151 (d) diagram illustration for the preparation of Fe-SA/WO2.72−x; (e) photocatalytic ammonia production rates over different catalysts; (f) Gibbs free energy diagrams of different paths on WO2.72 and Fe-SA/WO2.72−x.41 | |
In addition, Hu et al.41 fabricated Fe-SA/WO2.72−x photocatalysts through a series of methods including solvent heat treatment, impregnation, and annealing under an H2/Ar atmosphere at a controlled temperature (Fig. 11d), Notably, the Fe-SA-4/WO2.72−x catalyst demonstrated the highest NH3 production rate at 186.5 μmol g−1 h−1 among all samples (Fig. 10e). The study employed DFT calculations to investigate the hydrogenation process of N2* to NNH* on pristine WO2.72−x, revealing a rate-limiting energy of 1.67 eV. However, the presence of Fe–O–W sites on Fe-SA/WO2.72−x resulted in a notable weakening of the N
N bond of adsorbed N2 (*N2), resulting in a substantial reduction in the energy barrier for hydrogenation and transformation to *N2H. Consequently, the incorporation of Fe single atoms on WO2.72−x and the presence of Ov were identified as key factors contributing to the improved nitrogen fixation (Fig. 11f).
4.2.2 Transition metal dichalcogenide as supports.
Transition metal dichalcogenides (MoS2 WS2, etc.) exhibit great potential as catalysts in photocatalysis, presenting a viable alternative option to noble metals and showing the capability to surpass their performance. The exceptional catalytic performance of transition metal dichalcogenides in their metallic phase can be attributed to their remarkable characteristics. The materials exhibit notable conductivity (Fig. 12a), facilitating the creation of trapping sites for photoinduced charges and facilitating the processes of charge separation, migration, and collection, thereby enhancing quantum efficiency (Fig. 12b). With abundant active sites present on both the edge and basal planes (Fig. 12c), these materials are capable of serving as crucial locations for adsorption, activation, and reaction of reactant molecules, effectively reducing activation energies and accelerating reaction kinetics (Fig. 12d). Importantly, their atomically thin structure allows for the formation of a dense interface structure of light-harvesting semiconductor, ensuring quick transfer of photoinduced charges. In addition, these materials also possess other ideal interface surface properties and behaviors, such as active site self-optimization, abundant sulfur vacancies as induced photothermal effects, and more. These distinct properties and behaviors enhance the potential of these materials as efficient cocatalysts in photocatalysis.91,152,153
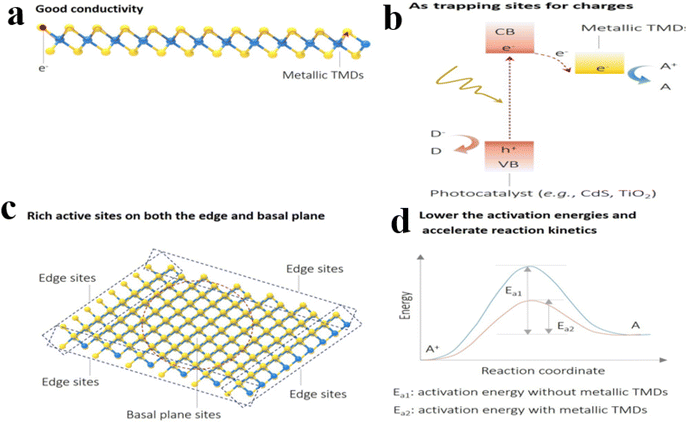 |
| Fig. 12 (a) Good conductivity; (b) facilitating the creation of trapping locations for charges generated by light exposure, and enhancing the processes of charge separation, movement, and gathering; (c) abundant active sites are present on both the edge and basal plane surfaces; (d) engaging actively in the adsorption, activation, and reaction sites of reactant molecules is crucial for reducing activation energies and enhancing the kinetics of chemical reactions.155 | |
Based on this, Hu et al.154 conducted a study where Mn single-atom doped MoS2−x nanoflowers were synthesized utilizing manganese chloride as the precursor for manganese atoms and thiourea as the sulfur source through a hydrothermal method (Fig. 13a). The research employed DFT to investigate the pNRR process on Mn-MoS2−x. The outcomes indicated a remarkable decrease in the adsorption of N2 and intermediate post-single-atom Mn doping. The energy barrier of Mn-MoS2−x-Sv was found to be higher than Mo edge sites yet lower than pure MoS2-based Mo sites. Notably, the energy barrier of pNRR on Mn-MoS2−x-Sv was significantly reduced to 0.87 eV, representing the lowest potential among MoS2 structures. This reduction was attributed to the fixation of N2 on exposed Mo sites facilitated by the presence of double S vacancies (Fig. 13b). Furthermore, under light irradiation without sacrificial agents in a pure water medium, Mn-MoS2−x-4 exhibited a visible light NH3 generation rate of 148.3 μmol g−1 h−1, a marked improvement compared with the original MoS2 (30.5 μmol g−1 h−1), demonstrating a 4.9 times increase in efficiency (Fig. 13c).
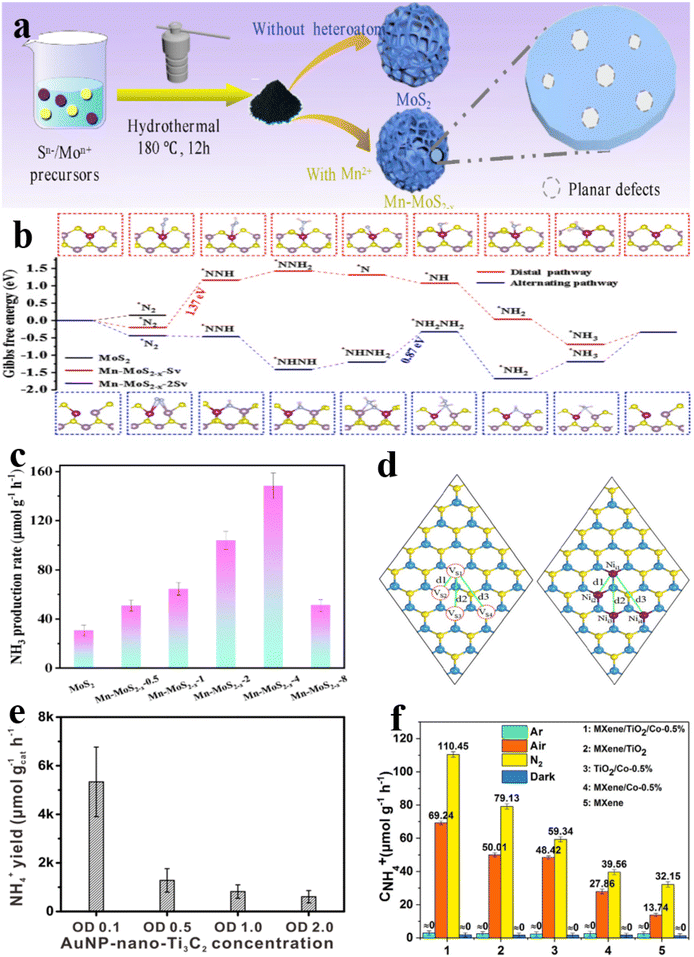 |
| Fig. 13 (a) Schematic illustration for the preparation of Mn-MoS2−x; (b) Gibbs free energy diagram of N2 reduction of Mo-MoS2−x; (c) photocatalytic NH3 generation rate of Mn-MoS2−x-x in N2 saturated water under visible light irradiation;154 (d) the top view of the Ni dopant configuration represented by the black solid line and the S vacancy represented by the red dashed line W as blue and element S as yellow.156 (e) Photocatalytic performance for visible-light-induced ammonia production reaction depending on AuNP-nano-Ti3C2 MXene heterozygotes weight.157 (f) The NH4+ yield rate of the control experiments under Ar, Air, N2, and dark ambient conditions.158 | |
The monolayer of WS2 stands out as a highly promising and economical catalyst across various electrocatalysis disciplines. Despite its inherent inertness, the WS2 basal plane boasts a notable surface-to-volume ratio, rendering it a prime candidate for enhancement through heteroatom doping methods. These strategies encompass the directed placement of dopant atoms into interstitial sites or the substitution of primitive lattice atoms within the WS2 framework. Ma et al.156 conducted a comprehensive examination of the pNRR of transition metal (TM) atoms, including Nb through Cd, Sc through Zn, W, Au, and Pt, attached to WS2 nanosheets featuring vacancies in their sulfur atoms. Through spin-polarized DFT calculation, it was revealed that a singular Ni atom positioned within a sulfur vacancy (Ni-WS2) exhibited heightened pNRR activity (Fig. 13d). Additionally, the NRR energy diagram of the Ni–WS2 surface indicated its inclination to proceed along the alternating associative pathway for NH3 preparation autonomously, devoid of external energy input.
4.2.3 MXenes as supports.
Recently, MXene-derived materials have exhibited notable effectiveness in a diverse range of catalytic uses. The distinctive two-dimensional structure of these materials enables proficient anchoring of individual metal atoms due to the abundant cation vacancies present in MXenes, which possess highly reactive and reductive attributes. Researchers have investigated the enhanced performance of gold-based single-atom catalysts in conjunction with two-dimensional materials known as MXenes, owing to their expansive specific surface area, abundant functional groups (–OH, –O, and –F), high conductivity, and capability to adsorb N2 chemically. Consequently, MXenes are recognized as promising candidates for the conversion of N2 to NH3.159–162 The relatively low light absorption coefficient of MXenes within the visible light spectrum can be enhanced through the incorporation of plasma nanomaterials such as Au.163,164 Shin et al.157 demonstrated the synthesis of ammonia under visible-light conditions by utilizing nanoscale Ti3C2 MXene in tandem with plasma Au. At a wavelength of 523 nm, the optical density (OD 0.1) reached 5334 μmol gcat−1 h−1. The enhanced photocatalytic efficiency was attributed to the increased light absorption of Au and efficient N2 fixation facilitated by chemically reduced nanoscale Ti3C2 MXene (Fig. 13e). Gao et al.158 effectively produced MXene-derived in situ TiO2 incorporated with cobalt for the pNRR through a dual-stage calcination technique. Notably, the production of NH3 reached 110 μmol g−1 h−1 in pure water under UV-vis light irradiation without the need for a sacrificial agent (Fig. 13f). The remarkable achievement observed can be ascribed to the integration of Cobalt, which serves to modulate the adsorption energy levels of nitrogen and ammonia, thereby facilitating the attainment of a state of chemical adsorption equilibrium.
4.2.4. Covalent/metal–organic framework (COF/MOF) as supports.
COFs and MOFs are structured porous materials formed by the establishment of covalent linkages between organic monomers and coordination interactions involving metal clusters and organic ligands. The amalgamation of organic frameworks and single atoms in the context of pNRR presents an opportunity to synergistically leverage their unique strengths while circumventing inherent drawbacks. Specifically, the unique catalytic sites of single atoms compensate for the inherent limited activity of framework supports in N2 reduction. Simultaneously, the ability to redesign and adjust the structures of frameworks ensures an optimal setting for anchoring single atoms and preventing their aggregation. Moreover, by controlling the chemical compositions of frameworks, atoms’ physical and chemical characteristics can be precisely manipulated, resulting in improved catalytic efficiency. Additionally, the customizable pore structures within frameworks facilitate mass transfer pathways during photocatalysis and modulate the interfaces for reactions between reactants and single atomic catalytic sites. Notably, the properties such as band gap, band structure, highest occupied molecular orbital, and lowest unoccupied molecular orbital levels of single-atom catalysts upheld by frameworks can be finely tuned at the molecular scale, thereby enhancing light absorption, photoinduced charge separation and migration and, ultimately, the photocatalytic performance in N2 reduction.165–168
He et al.49 synthesized Au single-atom modified covalent organic frameworks (COFs) following a specific procedure. Initially, COFs were synthesized using acetic acid, benzaldehyde, and diamine tetraacetic acid. Subsequently, KAuCl4 was employed as the source of Au atoms, which were then stirred at 85 °C for 24 hours. The resulting precipitate was isolated through centrifugation at 8000 rpm for 5 minutes and washed thrice with tetrahydrofuran (THF). The final product, denoted as COFX Au, was subjected to vacuum drying at ambient conditions overnight to ensure the complete removal of solvent residues (Fig. 14a). After being functionalized with Au atoms, COFX-Au exhibited preserved crystal structures and porosity. Analysis using aberration-corrected high-angle annular dark-field scanning transmission electron microscopy (HAADF-STEM) displayed an even distribution of single Au atoms as luminous points on the COF frameworks (Fig. 14b). The characteristics of Au atoms were further scrutinized through synchrotron-radiation-based X-ray absorption fine-structure spectroscopy (Fig. 14c). The coordination environment of Au atoms was determined to be 3.0 Au–N (±0.9) and 1.9 Au–Cl (±0.5) based on extended X-ray absorption fine-structure (EXAFS) fitting analyses. These results revealed that the N atoms from porphyrin subunits functioned as anchoring sites for single Au atoms, facilitating the formation of square-planar coordination structures. The most noteworthy compound was COF5-Au featuring a robust electron-deficient pentafluorophenyl group attached to the terminal position of the porphyrin Au center. This configuration exhibited superior NH3 release capabilities, demonstrating a heightened NH3 generation rate of 427.9 μmol g−1 h−1 (Fig. 14d). These findings reinforce the notion that electron-withdrawing entities play a pivotal role in improving ammonia production.
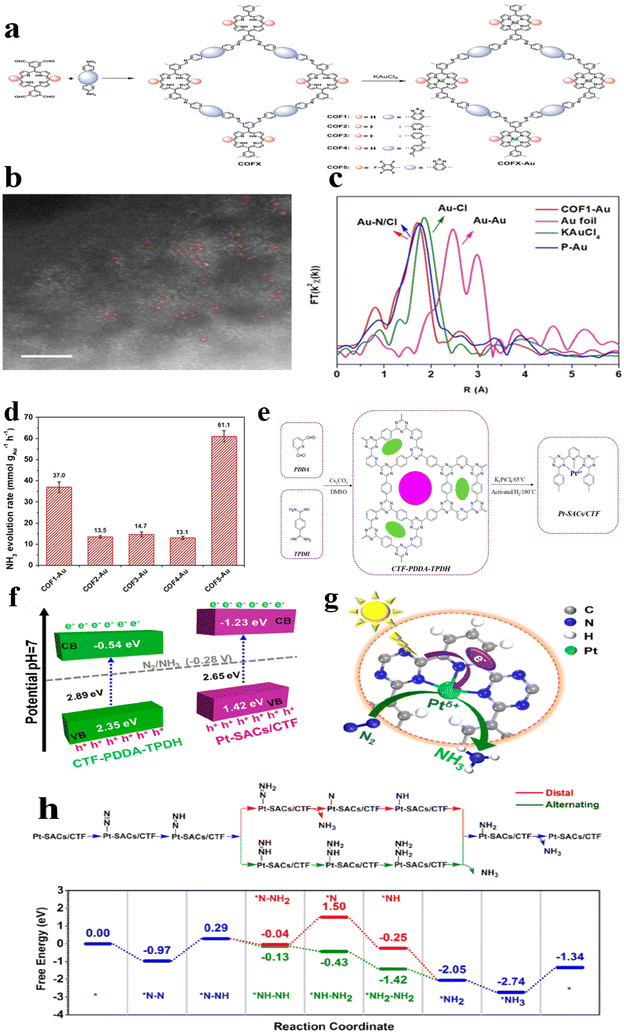 |
| Fig. 14 (a) Schematic illustration of COFX-Au; (b) HAADF-STEM of COFX-Au; (c) XAFS of COFX-Au; (d) mass production ammonia rate with different Au loadings.49 (e) Schematic illustration of PtSACs/CTF; (f) the band structure and (g) the N2 fixation mechanism of Pt-SACs/CTF; (h) the diagram illustrating the distal and alternating mechanisms, along with the corresponding free energy profiles, during the nitrogen reduction process on the Pt-SACs/CTF catalyst is presented.54 | |
Similarly, Li et al.54 successfully prepared single-atom Pt anchored at the –N3 sites of stable and ultrathin CTF nanosheets, denoted as Pt-SACs/CTF, using photodeposition techniques (Fig. 14e). The synthesis method led to the production of NH3 from an N2-saturated aqueous solution at a rate of 171.40 μmol g−1 h−1, without the need for a sacrificial agent, with an AQE of 1.4% at 420 nm. The CB potential of Pt-SACs/CTF (Fig. 14f and g) met the requirements for the ammonia synthesis reaction (N2/NH3 = −0.28 V vs. NHE). When exposed to light, the photogenerated electrons on the CB of the stable ultrathin CTF-PDDA-TPDH nanosheets were transferred to Pt-SACs, enabling the conversion of N2 molecules adsorbed on Pt-SACs to ammonia, which then dissolved in water to form NH4+. DFT analysis revealed that the fixation of N2 in the Pt-SAC/CTF catalyst occurred through an alternating mechanism. In this mechanism, the initial protonation step of forming NNH* from adsorbed N2 is electron withdrawing, whereas all subsequent fundamental steps are exergonic. The alternating mechanism's onset potential was 1.26 eV (Fig. 14h).
Shang et al.169 also explored the pNRR performance of Fe–N–C catalysts derived from a porphyrin-based metal–organic framework (PMOF). A notable feature of PMOF is the utilization of Al as a stable metal node. Fe is uniformly distributed at the atomic level within the porphyrin rings, facilitating N2 adsorption and activation. The Fe–N site emerges as the pivotal center for photocatalytic activity. The integrated findings from experiments and theoretical analyses indicate that the Fe–N site within Al-PMOF(Fe) serves as the primary photocatalytic site, alleviating the challenges associated with the rate-controlling way in photocatalytic nitrogen reduction reactions. The optimal configuration of N2 within Al PMOF (Fe) involves the end-to-end adsorption of the N–N bond onto the Fe single-atom positioned between two PMOF layers, with the reaction proceeding through a series of alternating pathways (Fig. 15a and b).
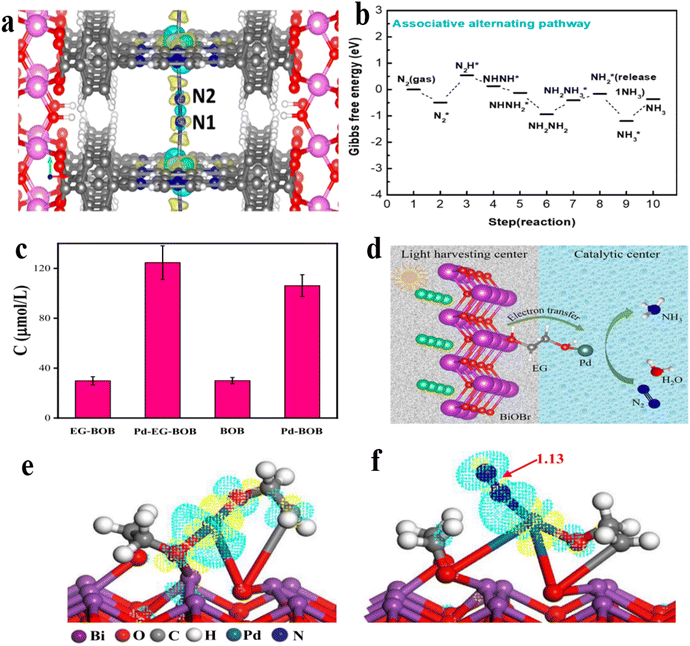 |
| Fig. 15 (a) The illustration presents the charge disparity map, depicting regions of positive density difference in yellow and negative density difference in cyan when nitrogen gas is adsorbed onto the aluminum-based porous metal–organic framework containing iron. Herein, C in gray, N in blue, H in white, O in red, Al in pink, and Fe in purple; (b) the free energy diagram of the associative alternating pathways in Al-PMOF(Fe);169 (c) quantitative determination of NH3 on Pd EG BiOBr; (d) photocatalytic mechanism of Pd EG BiOBr; (e and f) the illustration presents the charge disparity map, depicting regions of positive density difference in yellow and negative density difference in cyan when nitrogen gas is adsorbed onto the aluminum-based porous metal–organic framework containing iron.61 | |
4.2.5 Bismuth-based material as support.
Bismuth (Bi)-based layered materials have emerged as prospective and innovative semiconductors for different applications, particularly in photocatalysis.170–172 The preferred hybridization between Bi 6s and O 2p orbitals imparts the majority of Bi-based materials with the ability to harness visible light. Furthermore, the exceptional stability of Bi3+ and cost-effectiveness of Bi metal position Bi-based layered materials as a favorable catalyst.173 Advancements in pNRR have been noted in recent years; however, the outcomes achieved thus far have yet to meet the desired levels of success. Notably, Bi, a main group metal with semiconductor characteristics, is being increasingly recognized as a potentially effective catalyst for N2 fixation. Metals exhibiting diminished reactivity, exemplified by bismuth, possess the capacity to preferentially augment the reductive adsorption of nitrogen to produce N2H*, without succumbing to the impact of the binding energy of ensuing intermediate species. This property also limits surface electron accessibility,92 suppressing the Hydrogen Evolution Reaction (HER). Currently, Bi-based materials demonstrate remarkable activity and selectivity in synthetic NRR processes. Since 2015, the group led by Zhang174 has successfully fabricated BiOBr nanosheets featuring exposed (001) surfaces and OVs (BOB-001-OV) for N2 reduction under visible light irradiation, sans the need for sacrificial agents. Notably, the catalyst exhibited a commendable NH3 production of 104.2 μmol gcat−1 h−1. Subsequently, materials based on bismuth have garnered significant interest among academic researchers.
Recently, Liu et al.61 developed a catalytic system consisting of atomically dispersed palladium (Pd) anchored on bismuth oxybromide (BiOBr) through ethylene glycol (EG) as a molecular connector. The incorporation of 0.20 wt% of Pd EG BiOBr led to a notable enhancement in the pNRR efficiency, resulting in a NH3 production rate of 124.63 μmol h−1 (Fig. 15c). The adsorption of N2 through chemisorption and the activation of the chemically inert N
N triple bond on the surface of BiOBr, facilitated by atomically dispersed Pd, were investigated through DFT. The extensibility of the N
N triple bond length to 1.139 Å signifies an intensified activation of the N
N bond over the Pd atom when contrasted with the N2 molecule in isolation (1.093 Å). Analysis of the charge density difference (Fig. 15e and f) illustrates the electron distribution and interactions between the Pd atom and adsorbed nitrogen. The observed reduction in electron density on the Pd atom and corresponding increase in electron density on the adsorbed N2 molecule indicates a possible initiation of N
N triple bond cleavage (Fig. 14f). Moreover, the electron redistribution on the C and O atoms of EG (Fig. 15e and f) implies that EG may modulate the electron density of the Pd atom to improve the adsorption capacity of N2. These findings suggest that atomically dispersed Pd is more effective at adsorbingand activating the inert N
N triple bond. In the illustrated mechanism denoted as Fig. 14d, the EG molecule acts as a bridge, promoting the generation of atomically dispersed Pd species. Additionally, the EG molecule facilitates electron transfer from BiOBr to both Pd and activated N2 molecules. Subsequently, the electrons combine with H+ to yield the formation of NH3. Table 1 presents an overview of the photocatalytic efficiency of SMACs in the context of pNRR as documented in the existing literature.
Table 1 Summary of the photocatalytic efficiency of SMACs for pNRR
Photocatalysts |
Amount [mg] |
Ammonia production rate |
Light source |
Efficiency of pNRR |
Sacrificial agent |
Ref. |
Ru/g-C3N4 |
50 |
125.5 μmol gcat−1 h−1 |
300 W Xe lamp (λ = 420) |
— |
— |
131
|
Ru/MOF/C3N4 |
10 |
8 mmol g−1 |
300 W Xe lamp (λ = 420) |
AQY = 7.7% |
Methanol |
132
|
Au/HCNS-NV |
50 |
783.4 mmol gcat−1 h−1 |
300 W Xe lamp (λ = 425) |
AQE = 0.64 |
Methanol |
137
|
Pd/g-C3N4 |
20 |
2200 μmol gcat−1 h−1 |
300 W Xe lamp (λ > 425) |
NO conversion 56.3% |
— |
138
|
Ag/g-C3N4 |
100 |
1.45 mmol gcat−1 h−1 |
300 W Xe lamp (λ > 400) |
— |
Alcohol |
136
|
Ag/CN |
100 |
1.95 mmol L−1 gcat−1 h−1 |
300 W Xe lamp (λ > 400) |
— |
Alcohol |
139
|
Fe-g-C3N4 |
30 |
62.42 μmol gcat−1 h−1 |
300 W Xe lamp (λ = 420) |
AQE = 0.1% |
— |
42
|
Mo-PCN |
3 |
50.9 mmol gcat−1 h−1 |
300 W Xe lamp (λ = 400) |
AQE = 0.7% |
— |
140
|
WC–Co/NGC |
50 |
142 μmol g−1 h−1 |
300 W Xe lamp (λ > 420) |
— |
1 mmol L−1 Na2SO3 |
147
|
Co@g-C3N4 |
20 |
50.2 μmol h−1 |
500 W Xe lamp (λ > 420) |
AQE = 5.4% |
Methanol |
148
|
Cu–CN |
50 |
186 μmol g−1 h−1 |
300 W Xe lamp (λ > 420) |
AQE = 1.01% |
— |
149
|
Ca/m-g-C3N4 |
20 |
42.23 μg gcat−1 h−1 |
300 W Xe lamp (full-spectrum) |
— |
— |
150
|
Ru/TiO2-VO |
30 |
18.9 μmol g−1 h−1 |
300 W Xe lamp (full-spectrum) |
— |
— |
151
|
Fe-SA/WO2.72−x |
5 |
186.5 μmol g−1 h−1 |
300 W Xe lamp (full-spectrum) |
AQE = 0.41% |
— |
41
|
Mn-MoS2−x-4 |
20 |
148.3 μmol g−1 h−1 |
300 W Xe lamp (λ = 400) |
AQE = 0.26% |
— |
154
|
Co-Mxene-TiO2 |
30 |
110 μmol g−1 h−1 |
300 W Xe lamp (λ = 420) |
— |
— |
158
|
Au-nano-Ti3C2 |
10 |
5334 μmol gcat−1 h−1 |
300 W Xe lamp (400 < λ < 780) |
— |
— |
157
|
COFX-Au |
100 |
427.9μmol gcat−1 h−1 |
300 W Xe lamp (full-spectrum) |
AQE = 0.29% |
K2SO3 |
49
|
Pt-SACs/CTF |
50 |
171.40 μmol g−1 h−1 |
300 W Xe lamp (420 < λ < 780) |
AQE = 1.4% |
— |
54
|
Al-PMOF(Fe) |
10 |
127 μg gcat−1 h−1 |
100 mW cm−2 Xe lamp (λ = 420) |
AQE = 0.05% |
Methanol |
169
|
Pd EG BiOBr |
50 |
124.63 μmol h−1 |
300 W Xe lamp (λ = 420) |
— |
Methanol |
61
|
5 Theoretical perspective
The design of efficient photocatalysts for pNRR has largely relied on the interplay between N2 molecules and the elemental components of the catalyst. This interplay plays a crucial role in influencing the adsorption and activation mechanisms of N2. The distinctive characteristics of N2 generally result in several interactions, including σ-donation, π-back donation, and their combination. A molecule of N2 is characterized by a strong triple bond between two N atoms, each possessing electrons in the s and p orbitals. One pair of electrons resides in the 2s orbital, while three lone-pair electrons are in the p orbitals. The hybridization of orbitals leads to the formation of four bonding and four antibonding orbitals, consisting of two σ and two π orbitals. This hybridization results in a robust N
N bond (Fig. 16a). The short bond length of 109.8 pm contributes significantly to the stability of the compound. This heightened stability poses a challenge in activating N2 because of the notable energy gap between the Highest Occupied Molecular Orbital (HOMO) and the Lowest Unoccupied Molecular Orbital (LUMO) of N2. The influence of N2 activation on the catalytic surface is crucial for pNRR, emphasizing the need to develop tailor-made catalysts. A thorough comprehensive understanding of N2 interactions with different elements is essential for designing such catalysts. Extensive theoretical and experimental investigations have explored various transition metals (TMs), demonstrating that TMs with several free d-electrons exhibit a more advantageous π-back donation effect (Fig. 16b and c). The vacant d-orbitals of TMs are capable of accepting lone-pair electrons from N2, resulting in the formation of a σ bond. Simultaneously, the occupied d-electrons are back-donated to the empty π* orbital of N2. Consequently, Ru, Fe, Mn, and Mo have been recognized as high-performing catalysts for pNRR within this specific group.175,176
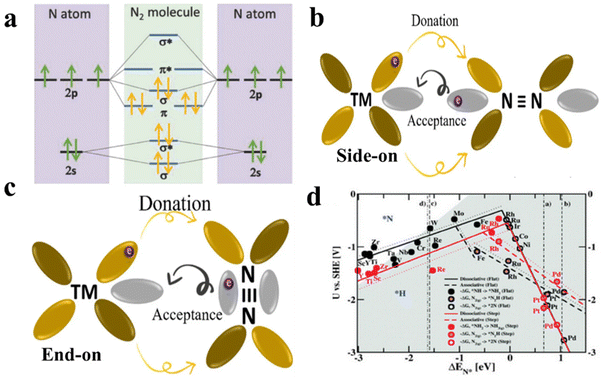 |
| Fig. 16 (a) Diagram of nitrogen molecular orbital;175 (b and c) interaction between TM and N2 molecules occurs through side-on and end-on adsorption in orbital interactions;176 (d) volcano plot for TMs (flat and stepped) surfaces versus absorption energy of *N.180 | |
5.1 Density functional theory (DFT)
First-principles calculations have been instrumental in narrowing the vast chemical space for effective photocatalyst discovery and providing a detailed atomic-level understanding of chemical reaction tasks often challenging for experimental approaches. Several modeling studies using DFT have demonstrated the promise of theoretical frameworks in elucidating and designing novel catalysts for pNRR. DFT has been extensively studied and has proved capable of accurately predicting the adsorption energies of reaction intermediates across numerous benchmark investigations.177–179
Skúlason and colleagues180 conducted a comprehensive study on various tightly packed and stepped TM surfaces, laying a foundational basis for the development of non-noble metal catalysts in NRR. The Ru single-atom and other transition metals positioned at the peak of a volcano plot (Fig. 16d) demonstrated elevated rates of NRR activity. However, HER posed a notable challenge to NRR by reducing selectivity and covering the surface with *H adsorbates during primary reactions. The surfaces of early transition metals, such as Y, Sc, Ti, and Zr, were shown to be predominantly coated with *N, effectively suppressing HER and enhancing selectivity. Alternative forms of transition metals, including oxides, carbides, and nitrides, have also been proposed to enhance NRR efficiency and deter competitive HER pathways. Similarly, Li et al.181 systematically studied the NRR catalytic activity of transition metals (TM = Sc–Cu, Y–Ag, and Hf–Au) anchored on monolayer graphyne (GY) using DFT calculations. The study highlighted TM@GY (TM = Sc, V, Mn, Y, Tc, and Os) as exhibiting remarkable NRR capabilities, with Mn, Tc, and Os@GY demonstrating particularly high selectivity toward NRR. This study provided a method for identifying highly effective catalysts for NRR under standard environmental conditions. Abghoui et al.182 proposed several TM nitrides (including VN, ZrN, NbN, and CrN) for NRR through the Mars–van Krevelen mechanism, demonstrating notably low limiting potentials (η = 0.5 V for VN). SMACs have been employed to enhance the catalytic activity of transition metals by overcoming surface limitations posed by NRR. This approach aimed to optimize the electronic characteristics of TMs and improve atom efficiency.183,184 SMACs doped on graphene exhibited great potential for the Nitrogen Reduction Reaction (NRR), demonstrating elevated catalytic efficiency while inhibiting the competing HER. Choi et al.184 identified Ti@N4 and V@N4 catalysts as having the most favorable energy barriers of 0.69 and 0.87 eV, respectively, through an evaluation of various TM-doped graphene variants with different coordination numbers (Fig. 17a). Ling et al.185 identified W-doped graphene with C3 coordinated atoms as the most effective catalyst, displaying the lowest potential-determining step (PDS) of 0.25 eV compared with other catalysts (Fig. 14b). NRR involves numerous intermediate stages within each mechanistic pathway, and SMACs may struggle to activate highly stable dinitrogen compounds. Biatom catalysts (BACs) leverage two TMs as active sites capable of effectively adsorbing N2 and enhancing NRR. Gu et al.186 studied various homonuclear and heteronuclear BACs on a phthalocyanine (Pc) material. Among these, V–V doped on Pc exhibited the most significant activity (Fig. 17c).
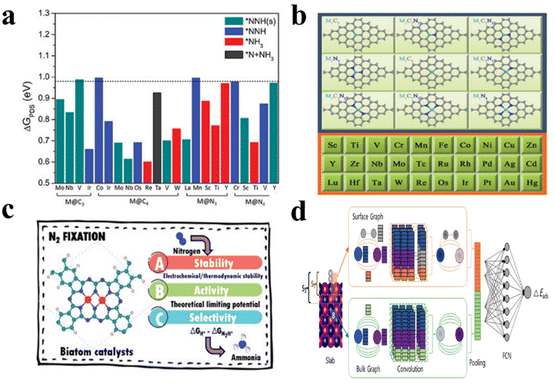 |
| Fig. 17 (a) PDS of SACs for N-doped graphene loaded with different TMs;184 (b) different materials of TMs supported N-doped graphene;185 (c) structures of BACs supported phthalocyanine;186 (d) schematic of describing the SGCNN model.192 | |
5.2 Machine learning
Recently, there has been a significant rise in the utilization of Machine Learning (ML) within pNRR research, leveraging advancements in artificial intelligence, computational capacities, big data, and ML methodologies.187,188 ML methodologies have greatly enhanced the development of efficient catalysts by playing a crucial role in evaluating potential catalysts and optimizing catalyst structures.189 This innovative approach involves the application of various ML models specifically tailored for the advancement of non-precious metal catalysts. Generally, the ML process is influenced by three critical factors: the database utilized, the choice of algorithms, and the creation of distinctive descriptors.190 Multiple elements affect ML learning and prediction processes. The algorithm selected is vital for managing data extraction, organization, and distribution. Additionally, descriptors tailored to different materials and properties are instrumental in capturing the fundamental physical and chemical attributes essential for catalytic activity.190 ML applications have focused extensively on nanomaterials, revealing complex correlations between catalyst structure and key performance indicators, such as stability, selectivity, and activity. Nonetheless, high-throughput DFT techniques have proved beneficial for evaluating potential catalysts, particularly when managing extensive datasets with numerous systems, thereby underscoring the crucial role of ML in such scenarios.191 Advances in ML methodologies involved the creation of ML interatomic potentials based on first-principles data, utilizing ab initio datasets. These potentials have been employed to perform dynamic simulations of intricate substance systems. Given the high computational cost associated with DFT and ab initio molecular dynamics (AIMD) simulations, ML-driven AIMD simulations can be utilized to assess the long-term stability of catalysts during operation, offering reduced simulation costs. Recently, Yeo et al.192 introduced a novel method known as the slab-graph convolutional neural network (SGCNN), designed for surface–adsorbate systems involved in catalytic reactions. This innovative model enhanced the ability of the graph-based convolutional neural network (GCNN), which was effective at predicting bulk characteristics but less effective in surface reaction catalysis (Fig. 17d).
5.3 Big data collection
Machine Learning (ML) utilizes big data generated from experiments or simulations, with particular emphasis on DFT-based calculations. These calculations provide a precise dataset that can be used to effectively understand and enhance catalyst performance. As part of a standard procedure, a dataset is first created using DFT calculations, including significant information on catalyst surface electronics (e.g., electronegativity, neighboring atoms, bond length, electron affinity, bond angle, atomic number, etc.) and computational metrics (e.g., adsorption energies of intermediates, theoretical overpotential, energy barriers, etc.). Diverse open databases, such as the Materials Project, Novel Materials Discovery (NOMAD),193 and the International Crystal Structure Database (ICSD),194,195 are available to access the necessary data pool. Subsequently, a machine learning algorithm is used to establish a mapping relationship between input and output matrices, enhancing fitting results during the training phase. Although machine learning provides valuable insights, challenges such as computational limitations and the potential incompleteness of databases persist due to differences in characterization technologies and inadequate data for various catalysts.
5.4 Artificial intelligence (AI)
The incorporation of artificial intelligence (AI) holds the potential to accelerate the identification of substances with specific desired attributes. However, the limitations of current ML algorithms highlight the necessity for more sophisticated models capable of containing a wide range of properties without overfitting, even when tested under realistic, non-ideal circumstances.196 Foundational insights are further enhanced through AI, particularly ML, offering an opportunity to expedite the discovery of new materials. Customized descriptors for NRR catalysts play a crucial role, and enhancing ML models by integrating core principles of condensed matter physics has been shown to improve forecasts and broaden datasets for more precise results.197,198 Essentially, the journey toward developing highly effective photocatalysts for NRR requires a comprehensive approach. This effort encompasses leveraging various materials, addressing catalyst development challenges, enhancing the integration of AI and ML, emphasizing synthesis uniformity, deepening the understanding of mechanisms, and exploring innovative methods to improve catalysis. This collaborative endeavor aims to bring the field closer to achieving efficient and environmentally friendly pNRR processes.191
6 Summary and perspectives
This article briefly reviews the SMACs used in the production of green ammonia through the pNRR process. The review encompasses a comprehensive analysis of different SMACs supported on various substrates, categorized as carbon and non-carbon materials, detailing their synthesis techniques, physicochemical characteristics, and performances in pNRR reactions. The superior pNRR activity exhibited by SMACs on carbon-based substrates is substantiated by theoretical investigations and elucidated with precision. Moreover, the review delves into theoretical projections regarding diverse SMACs supported on non-carbon substrates. While SMACs have made notable advancements in the pNRR sector in recent decades, there persist challenges within this promising domain that necessitate resolution to enhance efficacy:
(I) Recent research has demonstrated effective mitigation of nanoparticle and cluster formation through meticulous regulation of precursor ion concentrations at the substrate surface. While achieving optimal atomic dispersion, this approach often sacrifices loading capacity. Hence, to develop a single-atom catalyst boasting exceptional atomic dispersion, high loading efficiency, and scalability for industrial applications, a comprehensive interdisciplinary investigation is imperative.
(II) The selection and preparation of a suitable support system play pivotal roles in the efficacy of single-metal atom catalysts. Before application, careful consideration and treatment of the support material are imperative. Furthermore, enhancing the stability of singular metal atoms can be achieved by facilitating metal–support interactions (MSIs) or forming strong bonds with adjacent coordination atoms. The objective is to advance the quantity of active sites and improve the reactivity of said sites concurrently. Currently, a limited number of semiconductors serve as supports; therefore, it is imperative to expand the development of other semiconductor systems for this purpose.
(III) HAADF-STEM and XAS techniques are employed in the analysis of metal single-atom photocatalysts to ascertain the precise atomic distribution of individual atoms while simultaneously negating the existence of metal nanoclusters or nanoparticles. These characterization methods, however, are limited to assessing the catalyst's state before and after a reaction. The direct observation of processes such as migration, aggregation, and dissociation of active sites on single-atom photocatalysts during reactions remains unexplored. The dynamic observation of metal single-atom sites during preparation and reaction stages is crucial for comprehending their characteristics and operational mechanisms, which is pivotal for developing robust and highly active single-atom photocatalysts. Although various studies have employed in situ or operando techniques to monitor the evolution of metal single-atom photocatalysts, the outcomes are susceptible to environmental conditions, and definitive mechanisms are yet to be elucidated. Enhancing the efficacy of characterization techniques is instrumental in advancing catalyst development. Despite progress, there exist hurdles in effectively characterizing single-metal atom photocatalytic systems for N2 fixation, necessitating the utilization of straightforward, effective, and dependable methodologies.
(IV) Currently, significant advancements are being made in the field of pNRR. Nonetheless, the challenge persists in transitioning this technology from laboratory settings to commercial applications due to the limited absorption and conversion efficiency of actual solar energy. To address this critical issue, it is imperative to enhance catalyst efficiency and devise photocatalytic reduction nitrogen reaction engineering strategies. By optimizing the effective irradiation surface area of the reactor, and adjusting sunlight incidence angles and reaction device configurations, solar energy conversion efficiency can be elevated at a fundamental level, consequently enhancing photocatalytic reaction rates. We encourage researchers to delve into this realm which may unveil a novel era in photocatalytic nitrogen fixation.
Author contributions
Ping Zhang: funding acquisition, investigation, supervision. Yongchong Yu: writing – original draft, writing – review & editing. Reyila Tuerhong: software. Xinyu Du: validation. Keyi Chai: investigation. Su Xiaoping: supervision, validation. Shujuan Meng: resources, supervision. Qing Su: resources, supervision. Han Lijuan: funding acquisition, resources. The manuscript was written through the contributions of all authors. All authors have approved the final version of the manuscript.
Data availability
Data availability does not apply to this article as no new data were created or analyzed in this study.
Conflicts of interest
There are no conflicts to declare
Acknowledgements
The authors are grateful for financial aid from the National Natural Science Foundation of China (22378326), Supported by the Fundamental Research Funds for the Central Universities (331920240059), the Science and Technology Project of Lanzhou (2024-3-42), the Scientific Research Project of Introducing Talents of Northwest Minzu University (xbmuyjrc202215).
References
- V. Smil, Detonator of the population explosion, Nature, 1999, 400, 415–415 CrossRef CAS
.
- M. Cheng, C. Xiao and Y. Xie, Photocatalytic nitrogen fixation: the role of defects in photocatalysts, J. Mater. Chem. A, 2019, 7, 19616–19633 RSC
.
- R. Shi, Y. Zhao, G. I. N. Waterhouse, S. Zhang and T. Zhang, Defect engineering in photocatalytic nitrogen fixation, ACS Catal., 2019, 9, 9739–9750 CrossRef CAS
.
- L. Li, P. Zhang, N. Li, T. Reyila, Y. Yu, X. Su, C. Peng and L. Han, Application of g-C3N4-based photocatalysts for N2 photofixation, J. Environ. Chem. Eng., 2024, 12, 112142 CrossRef CAS
.
- W. Liu, K. Yin, K. Yuan, S. Zuo, S. Yang, C. Yao and M. Chen, In situ synthesis of Bi2MoO6@C@attapulgite photocatalyst for enhanced photocatalytic nitrogen fixation ability under simulated solar irradiation, Colloids Surf., A, 2020, 591, 124488 CrossRef CAS
.
- C. MacLaughlin, L. Liu, L. Greenlee and D. MacFarlane, Role for Standardization in electrocatalytic ammonia synthesis, ACS Energy Lett., 2019, 4, 1432–1436 CrossRef CAS
.
- J. Li, X. Guo, L. Gan, Z.-F. Huang, L. Pan, C. Shi, X. Zhang, G. Yang and J.-J. Zou, Fundamentals and advances in emerging crystalline porous materials for photocatalytic and electrocatalytic nitrogen fixation, ACS Appl. Energy Mater., 2022, 5, 9241–9265 CrossRef CAS
.
- R. Shi, X. Zhang, G. I. N. Waterhouse, Y. Zhao and T. Zhang, The Journey toward low Temperature, low pressure catalytic nitrogen fixation, Adv. Energy Mater., 2020, 10, 2000659 CrossRef CAS
.
- F. Jiao and B. Xu, Electrochemical ammonia synthesis and ammonia fuel cells, Adv. Mater., 2019, 31, 1805173 CrossRef
.
- T. Kandemir, M. E. Schuster, A. Senyshyn, M. Behrens and R. Schlögl, The haber–bosch process revisited: On the real structure and stability of “ammonia iron” under working conditions, Angew. Chem., Int. Ed., 2013, 52, 12723–12726 CrossRef CAS PubMed
.
- H. Li, C. Mao, H. Shang, Z. Yang, Z. Ai and L. Zhang, New opportunities for efficient N2 fixation by nanosheet photocatalysts, Nanoscale, 2018, 10, 15429–15435 RSC
.
- S. Licht, B. Cui, B. Wang, F.-F. Li, J. Lau and S. Liu, : Ammonia synthesis by N2 and steam electrolysis in molten hydroxide suspensions of nanoscale Fe2O3, Science, 2014, 345, 637–640 CrossRef CAS PubMed
.
- Y. Liu, X. Ye, R. Li, Y. Tao, C. Zhang, Z. Lian, D. Zhang and G. Li, Boosting the photocatalytic nitrogen reduction to ammonia through adsorption-plasmonic synergistic effects, Chin. Chem. Lett., 2022, 33, 5162–5168 CrossRef CAS
.
- H. Shen, M. Yang, L. Hao, J. Wang, J. Strunk and Z. Sun, Photocatalytic nitrogen reduction to ammonia: Insights into the role of defect engineering in photocatalysts, Nano Res., 2022, 15, 2773–2809 CrossRef CAS
.
- G. N. Schrauzer and T. D. Guth, Photolysis of water and photoreduction of nitrogen on titanium dioxide, J. Am. Chem. Soc., 1977, 99, 7189–7193 CrossRef CAS
.
- X. Chen, N. Li, Z. Kong, W.-J. Ong and X. Zhao, Photocatalytic fixation of nitrogen to ammonia: state-of-the-art advancements and future prospects, Mater. Horiz., 2018, 5, 9–27 RSC
.
- Y. Sun, Y. Ahmadi, K.-H. Kim and J. Lee, The use of bismuth-based photocatalysts for the production of ammonia through photocatalytic nitrogen fixation, Renewable Sustainable Energy Rev., 2022, 170, 112967 CrossRef CAS
.
- R. Guan, X. Cheng, Y. Chen, Z. Wu, Z. Zhao, Q. Shang, Y. Sun and Z. Sun, Wettability control of defective TiO2 with alkyl acid for highly efficient photocatalytic ammonia synthesis, Nano Res., 2023, 16, 10770–10778 CrossRef CAS
.
- T. Hou, H. Peng, Y. Xin, S. Wang, W. Zhu, L. Chen, Y. Yao, W. Zhang, S. Liang and L. Wang, Fe single-atom catalyst for visible-light-driven photofixation of nitrogen Sensitized by triphenylphosphine and sodium iodide, ACS Catal., 2020, 10, 5502–5510 CrossRef CAS
.
- J. Yu, T. Zhang and N. Wu, Solar photocatalysis, Sol. RRL, 2021, 5, 2100037 CrossRef
.
- C. Mao, H. Li, H. Gu, J. Wang, Y. Zou, G. Qi, J. Xu, F. Deng, W. Shen, J. Li, S. Liu, J. Zhao and L. Zhang, Beyond the thermal equilibrium limit of ammonia synthesis with dual temperature zone catalyst powered by solar light, Chem, 2019, 5, 2702–2717 CAS
.
- W. Zhang, R. Liu, Z. Fan, H. Wen, Y. Chen, R. Lin, Y. Zhu, X. Yang and Z. Chen, Synergistic copper nanoparticles and adjacent single atoms on biomass-derived N-doped carbon toward overall water splitting, Inorg. Chem. Front., 2023, 10, 443–453 RSC
.
- W. Shao, M. Yu, X. Xu, X. Han, Y. Chen, J. Han, G. Wu and W. Xing, Design of a single-atom In–N3−S site to modulate exciton behavior in carbon nitride for enhanced photocatalytic performance, Small, 2024, 20, 2306567 CrossRef CAS
.
- X. Xu, W. Shao, G. Tai, M. Yu, X. Han, J. Han, G. Wu and W. Xing, Single-atomic Co-N site modulated exciton dissociation and charge transfer on covalent organic frameworks for efficient antibiotics degradation via peroxymonosulfate activation, Sep. Purif. Technol., 2024, 333, 125890 CrossRef CAS
.
- P. Zhang, Z. Wang, L. Zhao, L. Li, N. Li, X. Su and Q. Su, Construction of a novel 0D-3D boron nitride quantum dots/NH2-MIL-125(Ti) composite for photodegradation of Rhodamine B, Mater. Sci. Semicond. Process., 2023, 167, 107793 CrossRef CAS
.
- X. Wang, P. Zhang, L. Li, N. Li, X. Su, X. Wei and L. Han, Preparation of a high-performance N-defect ZnO@g-C3N4 nanocomposite and its photocatalytic degradation of tetracycline, Mater. Today Commun., 2023, 36, 106732 CrossRef CAS
.
- P. Zhang, L. Zhao, Z. Wang, L. Li, N. Li, X. Su, Q. Su and L. Han, Efficient visible light performance of MoS2QDs/TiO2 hollow sphere photocatalysts for the degradation of dyes and antibiotics, Vacuum, 2024, 219, 112695 CrossRef CAS
.
- W. Zhan, Y. Yuan, L. Sun, Y. Yuan, X. Han and Y. Zhao, Hierarchical NiO@N-doped carbon microspheres with ultrathin nanosheet subunits as excellent photocatalysts for hydrogen evolution, Small, 2019, 15, 1901024 CrossRef PubMed
.
- H. Wang, X. Gao, Z. Lv, T. Abdelilah and A. Lei, Recent advances in oxidative R1-H/R2-H cross-coupling with hydrogen evolution via photo-/electrochemistry, Chem. Rev., 2019, 119, 6769–6787 CrossRef CAS PubMed
.
- L. F. Garay-Rodríguez, L. M. Torres-Martínez and E. Moctezuma, Photocatalytic performance of K2Ti6O13 whiskers to H2 evolution and CO2 photo-reduction, J. Energy Chem., 2019, 37, 18–28 CrossRef
.
- X. Li, N. Li, Y. Gao and L. Ge, Design and applications of hollow-structured nanomaterials for photocatalytic H2 evolution and CO2 reduction, Chin. J. Catal., 2022, 43, 679–707 CrossRef CAS
.
- H. Bai, S. H. Lam, J. Yang, X. Cheng, S. Li, R. Jiang, L. Shao and J. Wang, A schottky-barrier-free plasmonic semiconductor photocatalyst for nitrogen fixation in a “one-stone-two-birds” Manner, Adv. Mater., 2022, 34, 2104226 CrossRef CAS
.
- Z. Liu, M. Luo, S. Yuan, L. Meng, W. Ding, S. Su, Y. Cao, Y. Wang and X. Li, Boron-doped graphene quantum dot/bismuth molybdate composite photocatalysts for efficient photocatalytic nitrogen fixation reactions, J. Colloid Interface Sci., 2023, 650, 1301–1311 CrossRef CAS PubMed
.
- D. Zhang, W. He, J. Ye, X. Gao, D. Wang and J. Song, Polymeric carbon nitride-derived photocatalysts for water splitting and nitrogen fixation, Small, 2021, 17, 2005149 CrossRef CAS PubMed
.
- F. Dong, Z. Zhao, T. Xiong, Z. Ni, W. Zhang, Y. Sun and W.-K. Ho, In situ construction of g-C3N4/g-C3N4 metal-free heterojunction for enhanced visible-light photocatalysis, ACS Appl. Mater. Interfaces, 2013, 5, 11392–11401 CrossRef CAS PubMed
.
- K. Hu, Z. Huang, L. Zeng, Z. Zhang, L. Mei, Z. Chai and W. Shi, Recent advances in MOF-based materials for photocatalytic nitrogen fixation, Eur. J. Inorg. Chem., 2022, 2022, e202100748 CrossRef CAS
.
- R. Bariki, S. K. Pradhan, S. Panda, S. K. Nayak, A. R. Pati and B. G. Mishra, Hierarchical UiO-66(−NH2)/CuInS2 S-scheme photocatalyst with controlled topology for enhanced photocatalytic N2 fixation and H2O2 production, Langmuir, 2023, 39, 7707–7722 CrossRef CAS
.
- Y. Zhao, Y. Zhao, G. I. N. Waterhouse, L. Zheng, X. Cao, F. Teng, L.-Z. Wu, C.-H. Tung, D. O'Hare and T. Zhang, Layered-Double-Hydroxide nanosheets as efficient visible-light-driven photocatalysts for dinitrogen fixation, Adv. Mater., 2017, 29, 1703828 CrossRef
.
- G. Zhang, Y. Li, C. He, X. Ren, P. Zhang and H. Mi, Recent progress in 2D catalysts for photocatalytic and electrocatalytic artificial nitrogen reduction to ammonia, Adv. Energy Mater., 2021, 11, 2003294 CrossRef CAS
.
- P. Liu, Z. Huang, S. Yang, J. Du, Y. Zhang, R. Cao, C. Chen, L. Li, T. Chen, G. Wang, D. Rao, X. Zheng and X. Hong, Support amorphization engineering regulates single-atom Ru as an electron pump for nitrogen photofixation, ACS Catal., 2022, 12, 8139–8146 CrossRef CAS
.
- B. Hu, B.-H. Wang, L. Chen, Z.-J. Bai, W. Zhou, J.-K. Guo, S. Shen, T.-L. Xie, C.-T. Au, L.-L. Jiang and S.-F. Yin, Electronic modulation of the interaction between Fe single atoms and WO2.72−x for photocatalytic N2 reduction, ACS Catal., 2022, 12, 11860–11869 CrossRef CAS
.
- T. Hu, G. Jiang, Y. Yan, S. Lan, J. Xie, Q. Zhang and Y. Li, Facile synthesis of Fe single-atom porous photocatalysts via direct metal atomization achieving efficient photocatalytic nitrogen fixation, J. Mater. Sci. Technol., 2023, 167, 248–257 CrossRef CAS
.
- S. Liu, Y. Wang, S. Wang, M. You, S. Hong, T.-S. Wu, Y.-L. Soo, Z. Zhao, G. Jiang, Q. Jieshan, B. Wang and Z. Sun, Photocatalytic fixation of nitrogen to ammonia by single Ru atom decorated TiO2 nanosheets, ACS Sustainable Chem. Eng., 2019, 7, 6813–6820 CrossRef CAS
.
- J. Zhang, W. Yang and C. Sun, Synergetic effect between non-metals and dual metal catalysts for nitrogen reduction reaction, Inorg. Chem. Front., 2023, 10, 4746–4753 RSC
.
- B. Qiao, A. Wang, X. Yang, L. F. Allard, Z. Jiang, Y. Cui, J. Liu, J. Li and T. Zhang, Single-atom catalysis of CO oxidation using Pt1/FeOx, Nat. Chem., 2011, 3, 634–641 CrossRef CAS PubMed
.
- G. Huang, G. Lin, Q. Niu, J. Bi and L. Wu, Covalent triazine-based frameworks confining cobalt single atoms for photocatalytic CO2 reduction and hydrogen production, J. Mater. Sci. Technol., 2022, 116, 41–49 CrossRef CAS
.
- A. Chipojola Mtukula, X.-D. Zhang, S.-Z. Hou, J.-M. Huang, M. Xu and Z.-Y. Gu, Metal-organic frameworks for efficient electrochemical reduction of carbon dioxide, Eur. J. Inorg. Chem., 2023, 26, e202300170 CrossRef CAS
.
- S. Si, H. Shou, Y. Mao, X. Bao, G. Zhai, K. Song, Z. Wang, P. Wang, Y. Liu, Z. Zheng, Y. Dai, L. Song, B. Huang and H. Cheng, Low-coordination single Au atoms on ultrathin ZnIn2S4 nanosheets for selective photocatalytic CO2 reduction towards CH4, Angew. Chem., Int. Ed., 2022, 61, e202209446 CrossRef CAS PubMed
.
- T. He, Z. Zhao, R. Liu, X. Liu, B. Ni, Y. Wei, Y. Wu, W. Yuan, H. Peng, Z. Jiang and Y. Zhao, Porphyrin-based covalent organic frameworks anchoring Au single atoms for photocatalytic nitrogen fixation, J. Am. Chem. Soc., 2023, 145, 6057–6066 CrossRef CAS PubMed
.
- C.-F. Li, W.-G. Pan, Z.-R. Zhang, T. Wu and R.-T. Guo, Recent progress of single-atom photocatalysts applied in energy conversion and environmental protection, Small, 2023, 19, 2300460 CrossRef CAS PubMed
.
- H. Zhang, J. Wei, J. Dong, G. Liu, L. Shi, P. An, G. Zhao, J. Kong, X. Wang, X. Meng, J. Zhang and J. Ye, Efficient visible-light-driven carbon dioxide reduction by a single-atom implanted metal–organic framework, Angew. Chem., Int. Ed., 2016, 55, 14310–14314 CrossRef CAS PubMed
.
- C. Gao, S. Chen, Y. Wang, J. Wang, X. Zheng, J. Zhu, L. Song, W. Zhang and Y. Xiong, Heterogeneous single-atom catalyst for visible-light-driven high-turnover CO2 reduction: the role of electron transfer, Adv. Mater., 2018, 30, 1704624 CrossRef
.
- L. Cheng, X. Yue, L. Wang, D. Zhang, P. Zhang, J. Fan and Q. Xiang, Dual-single-atom tailoring with bifunctional integration for high-performance CO2 photoreduction, Adv. Mater., 2021, 33, 2105135 CrossRef CAS PubMed
.
- J. Li, P. Liu, Y. Tang, H. Huang, H. Cui, D. Mei and C. Zhong, Single-atom Pt–N3 sites on the stable covalent triazine framework nanosheets for photocatalytic N2 fixation, ACS Catal., 2020, 10, 2431–2442 CrossRef CAS
.
- G. Ren, J. Zhao, Z. Zhao, Z. Li, L. Wang, Z. Zhang, C. Li and X. Meng, Defects-induced single-atom anchoring on metal–organic frameworks for high-efficiency photocatalytic nitrogen reduction, Angew. Chem., Int. Ed., 2024, 63, e202314408 CrossRef CAS PubMed
.
-
B. G. Seo, J. Park, B. J. Kim, G. D. Han, K. H. Park, H. Park and J. H. Shim, Hetero-structured palladium-coated zinc oxide photocatalysts for sustainable water treatment, ECS Meeting Abstracts, 2023, vol. MA2023-01, p. 2407.
- L. Wang, Y. Xia and J. Yu, Hydrogen-bond activation of N2 molecules
and photocatalytic nitrogen fixation, Chem, 2021, 7, 1983–1985 CAS
.
- M. He, Z. Fang, P. Wang, Y. You and Z. Li, Recent progress in photocatalytic chemical fixation of carbon dioxide, ACS Sustainable Chem. Eng., 2023, 11, 12194–12217 CrossRef CAS
.
- G. Ren, S. Liu, M. Shi, Z. Zhang, Z. Li and X. Meng, Ultraviolet light-modulated defects on BiOBr to improve the photocatalytic fixation of nitrogen to ammonia, Sol. RRL, 2022, 6, 2200653 CrossRef CAS
.
- K. Li, W. Cai, Z. Zhang, H. Xie, Q. Zhong and H. Qu, Boron doped C3N5 for photocatalytic nitrogen fixation to ammonia: The key role of boron in nitrogen activation and mechanism, Chem. Eng. J., 2022, 435, 135017 CrossRef CAS
.
- J. Liu, F. Li, J. Lu, R. Li, Y. Wang, Y. Wang, X. Zhang, C. Fan and R. Zhang, Atomically dispersed palladium-ethylene glycol- bismuth oxybromide for photocatalytic nitrogen fixation: Insight of molecular bridge mechanism, J. Colloid Interface Sci., 2021, 603, 17–24 CrossRef CAS PubMed
.
- H. Li, J. Wang, Z. Ruan, P. Nan, B. Ge, M. Cheng, L. Yang, X. Li, Q. Liu, B. Pan, Q. Zhang, C. Xiao and Y. Xie, Electron transfer bridge inducing polarization of nitrogen molecules for enhanced photocatalytic nitrogen fixation, Mater. Horiz., 2023, 10, 5053–5059 RSC
.
- C.-L. Chen, H.-Y. Wang, J.-P. Li, L.-S. Long, X.-J. Kong and L.-S. Zheng, Assembling lanthanide–transition metal clusters on TiO2 for photocatalytic nitrogen fixation, Inorg. Chem. Front., 2022, 9, 2862–2868 RSC
.
- M. A. Khan, M. A. Nadeem and H. Idriss, Ferroelectric polarization effect on surface chemistry and photo-catalytic activity: A review, Surf. Sci. Rep., 2016, 71, 1–31 CrossRef CAS
.
- M. Shen, T. Ding, W. H. Rackers, C. Tan, K. Mahmood, M. D. Lew and B. Sadtler, Single-molecule colocalization of redox reactions on semiconductor photocatalysts connects surface heterogeneity and charge-carrier separation in bismuth oxybromide, J. Am. Chem. Soc., 2021, 143, 11393–11403 CrossRef CAS PubMed
.
- X. Zhang, Z. Li, T. Liu, M. Li, C. Zeng, H. Matsumoto and H. Han, Water oxidation sites located at the interface of Pt/SrTiO3 for photocatalytic overall water splitting, Chin. J. Catal., 2022, 43, 2223–2230 CrossRef CAS
.
- L. Chang, S. T. Yong, S. P. Chai, L. K. Putri, L. L. Tan and A. R. Mohamed, A review of methanol photoreforming: elucidating the mechanisms, photocatalysts and recent advancement strategies, Mater. Today Chem., 2023, 27, 101334 CrossRef CAS
.
- M. Li, Y. Cui, X. Zhang, Y. Luo, Y. Dai and Y. Huang, Screening a suitable Mo form supported on graphdiyne for effectively electrocatalytic N2 reduction reaction: From atomic catalyst to cluster catalyst, J. Phys. Chem. Lett., 2020, 11, 8128–8137 CrossRef CAS PubMed
.
- T. Nakao, K. Ogasawara, M. Kitano, S. Matsuishi, P. V. Sushko and H. Hosono, Ship-in-a-bottle synthesis of high concentration of N2 molecules in a cage-structured electride, J. Phys. Chem. Lett., 2021, 12, 1295–1299 CrossRef CAS PubMed
.
- X. Niu, A. Shi, D. Sun, S. Xiao, T. Zhang, Z. Zhou, X. A. Li and J. Wang, Photocatalytic ammonia synthesis: mechanistic insights into N2 activation at oxygen vacancies under visible light excitation, ACS Catal., 2021, 11, 14058–14066 CrossRef CAS
.
- A. R. Singh, B. A. Rohr, J. A. Schwalbe, M. Cargnello, K. Chan, T. F. Jaramillo, I. Chorkendorff and J. K. Nørskov, Electrochemical ammonia synthesis—The selectivity challenge, ACS Catal., 2017, 7, 706–709 CrossRef CAS
.
- J. Wong, S. T. Omelchenko and H. A. Atwater, Impact of semiconductor band tails and band filling on photovoltaic efficiency limits, ACS Energy Lett., 2021, 6, 52–57 CrossRef CAS
.
- K. Sridharan, S. Shenoy, S. G. Kumar, C. Terashima, A. Fujishima and S. Pitchaimuthu, Advanced two-dimensional heterojunction photocatalysts of stoichiometric and non-stoichiometric bismuth oxyhalides with graphitic carbon nitride for sustainable energy and environmental applications, Catalysts, 2021, 11(4), 426 CrossRef CAS
.
- X. Li, X. Sun, L. Zhang, S. Sun and W. Wang, Efficient photocatalytic fixation of N2 by KOH-treated g-C3N4, J. Mater. Chem. A, 2018, 6, 3005–3011 RSC
.
- X. Chen, X. Zhang, Y.-H. Li, M.-Y. Qi, J.-Y. Li, Z.-R. Tang, Z. Zhou and Y.-J. Xu, Transition metal doping BiOBr nanosheets with oxygen vacancy and exposed {102} facets for visible light nitrogen fixation, Appl. Catal., B, 2021, 281, 119516 CrossRef CAS
.
- S. Sun, Q. An, W. Wang, L. Zhang, J. Liu and W. A. Goddard III, Efficient photocatalytic reduction of dinitrogen to ammonia on bismuth monoxide quantum dots, J. Mater. Chem. A, 2017, 5, 201–209 RSC
.
- H. Ali, M. Masar, A. C. Guler, M. Urbanek, M. Machovsky and I. Kuritka, Heterojunction-based photocatalytic nitrogen fixation: principles and current progress, Nanoscale Adv., 2021, 3, 6358–6372 RSC
.
- P. Li, Z. Zhou, Q. Wang, M. Guo, S. Chen, J. Low, R. Long, W. Liu, P. Ding, Y. Wu and Y. Xiong, Visible-light-driven nitrogen fixation catalyzed by Bi5O7Br nanostructures: enhanced performance by oxygen vacancies, J. Am. Chem. Soc., 2020, 142, 12430–12439 CrossRef CAS PubMed
.
- H. Li, J. Shang, Z. Ai and L. Zhang, Correction to “Efficient visible light nitrogen fixation with BiOBr nanosheets of oxygen vacancies on the exposed {001} Facets”, J. Am. Chem. Soc., 2018, 140, 526–526 CrossRef CAS PubMed
.
- X. Kong, K. Chang, X. Tao and L. Huang, Oxygen vacancy and Z-scheme heterojunction cooperate to promote visible light nitrogen photofixation, Chem. Eng. J., 2023, 475, 146159 CrossRef CAS
.
- L. Zhang, F. Xie, J. Liu, Z. Sun, X. Zhang, Y. Wang, Y. Wang, R. Li and C. Fan, Light-switchable oxygen vacancies enhanced nitrogen fixation performance on BiOBr: mechanism of formation, reconversion and function, Chem. Eng. J., 2022, 450, 138066 CrossRef CAS
.
- J. Li, M. Zheng, F. Wei, C. Dong, Z. Xiu, W. Mu, X. Zhou, Y. Ding and X. Han, Fe doped InVO4 nanosheets with rich surface oxygen vacancies for enhanced electrochemical nitrogen fixation, Chem. Eng. J., 2022, 431, 133383 CrossRef CAS
.
- W. Xie, J. Huang, L. Huang, S. Geng, S. Song, P. Tsiakaras and Y. Wang, Novel fluorine-doped cobalt molybdate nanosheets with enriched oxygen-vacancies for improved oxygen evolution reaction activity, Appl. Catal., B, 2022, 303, 120871 CrossRef CAS
.
-
M. H. Urgesa, D. F. A. Putra, A. Qadir, U. A. Khan, T.-C. Huang, Y. X. Chiu, J. H. Lin and R. T. Ginting, in Photocatalytic activities for environmental remediation and energy conversion, ed. H. Abdullah, Springer Nature Singapore, Singapore, 2023 Search PubMed
.
- Y. Fu, Y. Liao, P. Li, H. Li, S. Jiang, H. Huang, W. Sun, T. Li, H. Yu, K. Li, H. Li, B. Jia and T. Ma, Layer structured materials for ambient nitrogen fixation, Coord. Chem. Rev., 2022, 460, 214468 CrossRef CAS
.
- B. M. Comer and A. J. Medford, Analysis of photocatalytic nitrogen fixation on rutile TiO2(110), ACS Sustainable Chem. Eng., 2018, 6, 4648–4660 CrossRef CAS
.
- Q. Liu, J. Yuan, Z. Gan, C. Liu, J. Li, Y. Liang and R. Chen, Photocatalytic N2 reduction: uncertainties in the determination of ammonia production, ACS Sustainable Chem. Eng., 2021, 9, 560–568 CrossRef CAS
.
- Q. Hao, C. Liu, G. Jia, Y. Wang, H. Arandiyan, W. Wei and B.-J. Ni, Catalytic reduction of nitrogen to produce ammonia by bismuth-based catalysts: state of the art and future prospects, Mater. Horiz., 2020, 7, 1014–1029 RSC
.
- M.-H. Vu, C.-C. Nguyen and T.-O. Do, Synergistic effect of Fe doping and plasmonic Au nanoparticles on W18O49 nanorods for enhancing photoelectrochemical nitrogen reduction, ACS Sustainable Chem. Eng., 2020, 8, 12321–12330 CrossRef CAS PubMed
.
- X. Yao, W. Zhang, J. Huang, Z. Du, X. Hong, X. Chen, X. Hu and X. Wang, Enhanced photocatalytic nitrogen fixation of Ag/B-doped g-C3N4 nanosheets by one-step in-situ decomposition-thermal polymerization method, Appl. Catal., A, 2020, 601, 117647 CrossRef CAS
.
- B. Sun, Z. Liang, Y. Qian, X. Xu, Y. Han and J. Tian, Sulfur vacancy-rich O-doped 1T-MoS2 nanosheets for exceptional photocatalytic nitrogen fixation over CdS, ACS Appl. Mater. Interfaces, 2020, 12, 7257–7269 CrossRef CAS PubMed
.
- J. H. Montoya, C. Tsai, A. Vojvodic and J. K. Norskov, The Challenge of electrochemical ammonia synthesis: A new perspective on the role of nitrogen scaling relations, ChemSusChem, 2015, 8, 2180–2186 CrossRef CAS PubMed
.
- M. T. Tang, X. Liu, Y. Ji, J. K. Norskov and K. Chan, Modeling hydrogen evolution reaction kinetics through explicit water–metal interfaces, J. Phys. Chem. C, 2020, 124, 28083–28092 CrossRef CAS
.
- X. Yu, H. Qiu, Z. Wang, B. Wang, Q. Meng, S. Sun, Y. Tang and K. Zhao, Constructing the Z-scheme TiO2/Au/BiOI nanocomposite for enhanced photocatalytic nitrogen fixation, Appl. Surf. Sci., 2021, 556, 149785 CrossRef CAS
.
- Y. Huang, J. Xiong, Z. Zou and Z. Chen, Emerging strategies for the synthesis of correlated single atom catalysts, Adv. Mater., 2024, 2312182 CrossRef PubMed
.
- J. Xi, H. S. Jung, Y. Xu, F. Xiao, J. W. Bae and S. Wang, Synthesis strategies, catalytic applications, and performance regulation of single-atom catalysts, Adv. Funct. Mater., 2021, 31, 2008318 CrossRef CAS
.
- Z.-H. Xue, D. Luan, H. Zhang and X. W. Lou, Single-atom catalysts for photocatalytic energy conversion, Joule, 2022, 6, 92–133 CrossRef CAS
.
- M. Li, J. Yu, Q. Liu, J. Liu, R. Chen, J. Zhu, R. Li and J. Wang, Atomically dispersed Fe–N5 sites anchored in porous N-doped carbon nanofibers for effective hydrogen Evolution Reaction, ACS Sustainable Chem. Eng., 2022, 10, 13505–13513 CrossRef CAS
.
- W. Jiang, Y. Zhao, X. Zong, H. Nie, L. Niu, L. An, D. Qu, X. Wang, Z. Kang and Z. Sun, Photocatalyst for high-performance H2 production: Ga-doped polymeric carbon nitride, Angew. Chem., Int. Ed., 2021, 60, 6124–6129 CrossRef CAS PubMed
.
- D. Liu, C. Zhang, J. Shi, Y. Shi, T. T. T. Nga, M. Liu, S. Shen and C.-L. Dong, Defect engineering
simultaneously regulating exciton dissociation in carbon nitride and local electron density in Pt single atoms toward highly efficient photocatalytic hydrogen production, Small, 2024, 20, 2310289 CrossRef CAS PubMed
.
- B. Zhang, X. Song, Z. Wang, B. Xu, W. Ye, L. Zhang, H. Lin, T. Ji, X. Lu and J. Zhu, Atomic layer deposition of Pd nanoparticles and single atoms on self-supported carbon monolithic catalysts synergistically boosts the hydrogen evolution reaction, J. Mater. Chem. A, 2024, 12, 28503–28511 RSC
.
- M. Xiao, L. Zhang, B. Luo, M. Lyu, Z. Wang, H. Huang, S. Wang, A. Du and L. Wang, Molten-salt-mediated synthesis of an atomic nickel co-catalyst on TiO2 for improved photocatalytic H2 evolution, Angew. Chem., Int. Ed., 2020, 59, 7230–7234 CrossRef CAS PubMed
.
- A. Wang, J. Li and T. Zhang, Heterogeneous single-atom catalysis, Nat. Rev. Chem., 2018, 2, 65–81 CrossRef CAS
.
- M. B. Gawande, P. Fornasiero and R. Zboril, Carbon-based single-atom catalysts for advanced applications, ACS Catal., 2020, 10, 2231–2259 CrossRef CAS
.
- C. Gao, J. Low, R. Long, T. Kong, J. Zhu and Y. Xiong, Heterogeneous single-atom photocatalysts: fundamentals and applications, Chem. Rev., 2020, 120, 12175–12216 CrossRef CAS PubMed
.
- F. Kraushofer and G. S. Parkinson, Single-atom catalysis: insights from model systems, Chem. Rev., 2022, 122, 14911–14939 CrossRef CAS PubMed
.
- Z. Geng, Y. Liu, X. Kong, P. Li, K. Li, Z. Liu, J. Du, M. Shu, R. Si and J. Zeng, Achieving a record-high yield rate of 120.9 for N2 electrochemical reduction over Ru single-atom catalysts, Adv. Mater., 2018, 30, 1803498 CrossRef PubMed
.
- B. Yu, H. Li, J. White, S. Donne, J. Yi, S. Xi, Y. Fu, G. Henkelman, H. Yu, Z. Chen and T. Ma, Tuning the catalytic preference of ruthenium catalysts for nitrogen reduction by atomic dispersion, Adv. Funct. Mater., 2020, 30, 1905665 CrossRef CAS
.
- X. Wang, W. Wang, M. Qiao, G. Wu, W. Chen, T. Yuan, Q. Xu, M. Chen, Y. Zhang, X. Wang, J. Wang, J. Ge, X. Hong, Y. Li, Y. Wu and Y. Li, Atomically dispersed Au1 catalyst towards efficient electrochemical synthesis of ammonia, Sci. Bull., 2018, 63, 1246–1253 CrossRef CAS PubMed
.
- H. Zou, W. Rong, S. Wei, Y. Ji and L. Duan, Regulating kinetics and thermodynamics of electrochemical nitrogen reduction with metal single-atom catalysts in a pressurized electrolyser, Proc. Natl. Acad. Sci. U. S. A., 2020, 117, 29462–29468 CrossRef CAS PubMed
.
- Y. Chen, R. Guo, X. Peng, X. Wang, X. Liu, J. Ren, J. He, L. Zhuo, J. Sun, Y. Liu, Y. Wu and J. Luo, Highly productive electrosynthesis of ammonia by molecule-targeting single Ag sites, ACS Nano, 2020, 14, 6938–6946 CrossRef CAS PubMed
.
- H. Yang, Y. Liu, Y. Luo, S. Lu, B. Su and J. Ma, Achieving high activity and selectivity of nitrogen reduction via Fe–N3 coordination on iron single-atom electrocatalysts at ambient conditions, ACS Sustainable Chem. Eng., 2020, 8, 12809–12816 CrossRef CAS
.
- S. Zhang, M. Jin, T. Shi, M. Han, Q. Sun, Y. Lin, Z. Ding, L. R. Zheng, G. Wang, Y. Zhang, H. Zhang and H. Zhao, Electrocatalytically active Fe-(O-C2)4 single-atom sites for efficient reduction of nitrogen to ammonia, Angew. Chem., Int. Ed., 2020, 59, 13423–13429 CrossRef CAS PubMed
.
- P. Kumar, T. A. Al-Attas, J. Hu and M. G. Kibria, Single atom catalysts for selective methane oxidation to oxygenates, ACS Nano, 2022, 16, 8557–8618 CrossRef CAS PubMed
.
- L. Wang, J. Wang, X. Gao, C. Chen, Y. Da, S. Wang, J. Yang, Z. Wang, J. Song, T. Yao, W. Zhou, H. Zhou and Y. Wu, Periodic one-dimensional single-atom arrays, J. Am. Chem. Soc., 2022, 144, 15999–16005 CrossRef CAS PubMed
.
- B.-B. Xu, X.-B. Fu, X.-M. You, E. Zhao, F.-F. Li, Z. Chen, Y.-X. Li, X. L. Wang and Y.-F. Yao, Synergistic promotion of single-atom Co surrounding a PtCo alloy based on a g-C3N4 nanosheet for overall water splitting, ACS Catal., 2022, 12, 6958–6967 CrossRef CAS
.
- J. Yu, C. Chen, Q. Zhang, J. Lin, X. Yang, L. Gu, H. Zhang, Z. Liu, Y. Wang, S. Zhang, X. Wang and L. Guo, Au Atoms anchored on amorphous C3N4 for single-site raman enhancement, J. Am. Chem. Soc., 2022, 144, 21908–21915 CrossRef CAS PubMed
.
- O. Pinto-Burgos, J. Castro-Gutiérrez, P. S. Poon, M. T. Izquierdo, A. Celzard, V. Fierro and J. Matos, Enhancing electrochemical capacitor performance of N-doped tannin-derived carbons by hydrothermal treatment in ammonia, J. Power Sources, 2024, 602, 234332 CrossRef CAS
.
- J. Wang, H. Huang, P. Wang, S. Wang and J. Li, N, S synergistic effect in hierarchical porous carbon for enhanced NRR performance, Carbon, 2021, 179, 358–364 CrossRef CAS
.
- S. Zhao, X. Lu, L. Wang, J. Gale and R. Amal, Carbon-based metal-free catalysts for electrocatalytic reduction of nitrogen for synthesis of ammonia at ambient conditions, Adv. Mater., 2019, 31, 1805367 CrossRef PubMed
.
- S. S. Sekhon and J.-S. Park, Biomass-derived N-doped porous carbon nanosheets for energy technologies, Chem. Eng. J., 2021, 425, 129017 CrossRef CAS
.
- W. Zhang, S. Zhan, Q. Qin, T. Heil, X. Liu, J. Hwang, T. H. Ferber, J. P. Hofmann and M. Oschatz, Electrochemical generation of catalytically active edge sites in C2N-type carbon materials for artificial nitrogen fixation, Small, 2022, 18, 2204116 CrossRef CAS PubMed
.
- P. Sun, J. Huang, F. Xu, J. Xu, T. Lin, W. Zhao, W. Dong and F. Huang, Boron-induced nitrogen fixation in 3D carbon materials for supercapacitors, ACS Appl. Mater. Interfaces, 2020, 12, 28075–28082 CrossRef CAS PubMed
.
- X. Han, C. S. Gerke, S. Banerjee, M. Zubair, J. Jiang, N. M. Bedford, E. M. Miller and V. S. Thoi, Strategic design of MoO2 nanoparticles supported by carbon nanowires for enhanced electrocatalytic nitrogen reduction, ACS Energy Lett., 2020, 5, 3237–3243 CrossRef CAS
.
- L. Prati, C. E. Chan-Thaw, S. Campisi and A. Villa, N-modified carbon-based materials: nanoscience for catalysis, Chem. Rec., 2016, 16, 2187–2197 CrossRef CAS PubMed
.
- S. Cao and J. Yu, Carbon-based H2-production photocatalytic materials, J. Photochem. Photobiol., C, 2016, 27, 72–99 CrossRef CAS
.
- Z. Li, K. Li, P. Du, M. Mehmandoust, F. Karimi and N. Erk, Carbon-based photocatalysts for hydrogen production: A review, Chemosphere, 2022, 308, 135998 CrossRef CAS PubMed
.
- C. Feng, Z.-P. Wu, K.-W. Huang, J. Ye and H. Zhang, Surface Modification of 2D photocatalysts for solar energy conversion, Adv. Mater., 2022, 34, 2200180 CrossRef CAS PubMed
.
- M. A. Shipman and M. D. Symes, Recent progress towards the electrosynthesis of ammonia from sustainable resources, Catal. Today, 2017, 286, 57–68 CrossRef CAS
.
- S. Wang, D. Guo, M. Zong, C. Fan, X. Jun and D.-H. Wang, Unravelling the strong metal-support interaction between Ru quantum dots and g-C3N4 for visible-light photocatalytic nitrogen fixation, Appl. Catal., A, 2021, 617, 118112 CrossRef CAS
.
- L. Li, Y. Yu, S. Lin, W. Chu, D. Sun, Q. Su, S. Ma, G. Du and B. Xu, Single ruthenium atom supported on g-C3N4 as an efficient photocatalyst for nitrogen fixation in ultra-pure water, Catal. Commun., 2021, 153, 106294 CrossRef CAS
.
- Z. Ding, X. Li, C. Kang, S. Yan, D. Zhao, H. Cai, S.-Y. Zhang and Y.-J. Zeng, Single Ru atoms confined into MOF/C3N4 for dual improved photocatalytic carbon dioxide reduction and nitrogen fixation, Chem. Eng. J., 2023, 473, 145256 CrossRef CAS
.
- M.-M. Shi, D. Bao, S.-J. Li, B.-R. Wulan, J.-M. Yan and Q. Jiang, Anchoring PdCu amorphous nanocluster on graphene for electrochemical reduction of N2 to NH3 under ambient conditions in aqueous solution, Adv. Energy Mater., 2018, 8, 1800124 CrossRef
.
- L. Han, Z. Ren, P. Ou, H. Cheng, N. Rui, L. Lin, X. Liu, L. Zhuo, J. Song, J. Sun, J. Luo and H. L. Xin, Modulating Single-atom palladium sites with copper for enhanced ambient ammonia electrosynthesis, Angew. Chem., Int. Ed., 2021, 60, 345–350 CrossRef CAS PubMed
.
- J. Li, Q. Li, Y. Chen, S. Lv, X. Liao and Y. Yao, Size effects of Ag nanoparticle for N2 photofixation over Ag/g-C3N4: Built-in electric fields determine photocatalytic performance, Colloids Surf., A, 2021, 626, 127053 CrossRef CAS
.
- Y. Wang, R. Zhao, F. Wang, Y. Liu, X. Yu, L. Chen, Y. Yao, S. Lu and X. Liao, Ultralow-temperature synthesis of small Ag-doped carbon nitride for nitrogen photofixation, Catal. Sci. Technol., 2020, 10, 7652–7660 RSC
.
- Y. Guo, J. Yang, D. Wu, H. Bai, Z. Yang, J. Wang and B. Yang, Au nanoparticle-embedded, nitrogen-deficient hollow mesoporous carbon nitride spheres for nitrogen photofixation, J. Mater. Chem. A, 2020, 8, 16218–16231 RSC
.
- G. Liu, Y. Huang, H. Lv, H. Wang, Y. Zeng, M. Yuan, Q. Meng and C. Wang, Confining single-atom Pd on g-C3N4 with carbon vacancies towards enhanced photocatalytic NO conversion, Appl. Catal., B, 2021, 284, 119683 CrossRef CAS
.
- J. Li, Q. Li, Y. Chen, S. Lu, X. Liao and Y. Yao, Size effects of Ag nanoparticle for N2 photofixation over Ag/g-C3N4: Built-in electric fields determine photocatalytic performance, Colloids Surf., A, 2021, 626, 127053 CrossRef CAS
.
- X.-W. Guo, S.-M. Chen, H.-J. Wang, Z.-M. Zhang, H. Lin, L. Song and T.-B. Lu, Single-atom molybdenum immobilized on photoactive carbon nitride as efficient photocatalysts for ambient nitrogen fixation in pure water, J. Mater. Chem. A, 2019, 7, 19831–19837 RSC
.
- Y. Kong, T. He, A. R. Puente Santiago, D. Liu, A. Du, S. Wang and H. Pan, Unravelling the reaction mechanisms of N2 fixation on molybdenum nitride: A full DFT study from the pristine surface to heteroatom anchoring, ChemSusChem, 2021, 14, 3257–3266 CrossRef CAS PubMed
.
- S.-Y. Lv, C.-X. Huang, G. Li and L.-M. Yang, Electrocatalytic mechanism of N2 reduction Reaction by single-atom catalyst rectangular TM-TCNQ monolayers, ACS Appl. Mater. Interfaces, 2021, 13, 29641–29653 CrossRef CAS PubMed
.
- Y. Ying, K. Fan, X. Luo, J. Qiao and H. Huang, Transition metal-tetracyanoquinodimethane monolayers as single-atom catalysts for the electrocatalytic nitrogen reduction reaction, Mater. Adv., 2020, 1, 1285–1292 RSC
.
- A. P. Hamsa, M. Arulprakasam and S. M. Unni, Electrochemical nitrogen fixation on single metal atom catalysts, Chem. Commun., 2023, 59, 10689–10710 RSC
.
- Q. Liu, C. Zeng, Z. Xie, L. Ai, Y. Liu, Q. Zhou, J. Jiang, H. Sun and S. Wang, Cobalt@nitrogen-doped bamboo-structured carbon nanotube to boost photocatalytic hydrogen evolution on carbon nitride, Appl. Catal., B, 2019, 254, 443–451 CrossRef CAS
.
- S. Chen, Y. Hau Ng, J. Liao, Q. Gao, S. Yang, F. Peng, X. Zhong, Y. Fang and S. Zhang, FeCo alloy@N-doped graphitized carbon as an efficient cocatalyst for enhanced photocatalytic H2 evolution by inducing accelerated charge transfer, J. Energy Chem., 2021, 52, 92–101 CrossRef CAS
.
- L. Wang, Q. Zhang, T. Wei, F. Li, Z. Sun and L. Xu, WC and cobalt nanoparticles embedded in nitrogen-doped carbon 3D nanocage derived from H3PW12O40@ZIF-67 for photocatalytic nitrogen fixation, J. Mater. Chem. A, 2021, 9, 2912–2918 RSC
.
- W. Zhang, Y. Fu, Q. Peng, Q. Yao, X. Wang, A. Yu and Z. Chen, Supramolecular preorganization effect to access single cobalt sites for enhanced photocatalytic hydrogen evolution and nitrogen fixation, Chem. Eng. J., 2020, 394, 124822 CrossRef CAS
.
- P. Huang, W. Liu, Z. He, C. Xiao, T. Yao, Y. Zou, C. Wang, Z. Qi, W. Tong, B. Pan, S. Wei and Y. Xie, Single atom accelerates ammonia photosynthesis, Sci. China: Chem., 2018, 61, 1187–1196 CrossRef CAS
.
- G. Pan, W. Zhang, T. Liu, Q. Tan, B. Wei, K. Ye, Y. Yang, D. Han, Z. Liu and L. Niu, Atomically dispersed s-block metal calcium site modified mesoporous g-C3N4 for boosting photocatalytic N2 reduction, Catal. Sci. Technol., 2023, 13, 111–118 RSC
.
- G. Ren, M. Shi, S. Liu, Z. Li, Z. Zhang and X. Meng, Molecular-level insight into photocatalytic reduction of N2 over Ruthenium single atom modified TiO2 by electronic Metal-support interaction, Chem. Eng. J., 2023, 454, 140158 CrossRef CAS
.
- Y. Tang, W. Zhou, Q. Shang, Y. Guo, H. Hu, Z. Li, Y. Zhang, L. Liu, H. Wang, X. Tan, T. Yu and J. Ye, Discerning the mechanism of expedited interfacial electron transformation boosting photocatalytic hydrogen evolution by metallic 1T-WS2-induced photothermal effect, Appl. Catal., B, 2022, 310, 121295 CrossRef CAS
.
- J. Qin, W. Zhao, X. Hu, J. Li, P. Ndokoye and B. Liu, Exploring the N2 adsorption and activation mechanisms over the 2H/1T mixed-phase ultrathin Mo1−xWxS2 nanosheets for boosting N2 photosynthesis, ACS Appl. Mater. Interfaces, 2021, 13, 7127–7134 CrossRef CAS PubMed
.
- B. Hu, B.-H. Wang, Z.-J. Bai, L. Chen, J.-K. Guo, S. Shen, T.-L. Xie, C.-T. Au, L.-L. Jiang and S.-F. Yin, Regulating MoS2 edge site for photocatalytic nitrogen fixation: A theoretical and experimental study, Chem. Eng. J., 2022, 442, 136211 CrossRef CAS
.
- R. Yang, Y. Fan, Y. Zhang, L. Mei, R. Zhu, J. Qin, J. Hu, Z. Chen, Y. Hau Ng, D. Voiry, S. Li, Q. Lu, Q. Wang, J. C. Yu and Z. Zeng, 2D transition metal dichalcogenides for photocatalysis, Angew. Chem., Int. Ed., 2023, 62, e202218016 CrossRef CAS PubMed
.
- X. Ma, J. Hu, M. Zheng, D. Li, H. Lv, H. He and C. Huang, N2 reduction using single transition-metal atom supported on defective WS2 monolayer as promising catalysts: A DFT study, Appl. Surf. Sci., 2019, 489, 684–692 CrossRef CAS
.
- H. H. Shin, W. Yang and D.-K. Lim, Gold and nanosized titanium carbide MXene heterozygotes as highly efficient visible-light responsive photocatalysts for ammonia synthesis, Carbon, 2023, 214, 118359 CrossRef CAS
.
- W. Gao, X. Li, S. Luo, Z. Luo, X. Zhang, R. Huang and M. Luo, In situ modification of cobalt on MXene/TiO2 as composite photocatalyst for efficient nitrogen fixation, J. Colloid Interface Sci., 2021, 585, 20–29 CrossRef CAS PubMed
.
- K. Huang, C. Li, H. Li, G. Ren, L. Wang, W. Wang and X. Meng, Photocatalytic applications of two-dimensional Ti3C2 MXenes: A Review, ACS Appl. Nano Mater., 2020, 3, 9581–9603 CrossRef CAS
.
- Q. Zhong, Y. Li and G. Zhang, Two-dimensional MXene-based and MXene-derived photocatalysts: Recent developments and perspectives, Chem. Eng. J., 2021, 409, 128099 CrossRef CAS
.
- S. H. W. Kok, J. Lee, L.-L. Tan, W.-J. Ong and S.-P. Chai, MXene—A new paradigm toward artificial nitrogen fixation for sustainable ammonia generation: synthesis, properties, and future outlook, ACS Mater. Lett., 2022, 4, 212–245 CrossRef CAS
.
- J. Sun, W. Kong, Z. Jin, Y. Han, L. Ma, X. Ding, Y. Niu and Y. Xu, Recent advances of MXene as promising catalysts for electrochemical nitrogen reduction reaction, Chin. Chem. Lett., 2020, 31, 953–960 CrossRef CAS
.
- R. Li, L. Zhang, L. Shi and P. Wang, MXene Ti3C2: An effective 2D light-to-heat conversion material, ACS Nano, 2017, 11, 3752–3759 CrossRef CAS PubMed
.
- B. Chang, Y. Guo, D. Wu, L. Li, B. Yang and J. Wang, Plasmon-enabled N2 photofixation on partially reduced Ti3C2 MXene, Chem. Sci., 2021, 12, 11213–11224 RSC
.
- Y.-S. Wei, M. Zhang, R. Zou and Q. Xu, Metal–organic framework-based catalysts with single metal sites, Chem. Rev., 2020, 120, 12089–12174 CrossRef CAS PubMed
.
- V. Hasija, S. Patial, P. Raizada, A. Aslam Parwaz Khan, A. M. Asiri, Q. Van Le, V.-H. Nguyen and P. Singh, Covalent organic frameworks promoted single metal atom catalysis: Strategies and applications, Coord. Chem. Rev., 2022, 452, 214298 CrossRef CAS
.
- D. Wang and Y. Zhao, Single-atom engineering of metal-organic frameworks toward healthcare, Chemistry, 2021, 7, 2635–2671 CrossRef CAS
.
- T. He and Y. Zhao, Engineering covalent/metal-organic frameworks with single atoms toward artificial photocatalytic nitrogen fixation, Chem Catal., 2023, 3, 100795 CrossRef CAS
.
- S. Shang, W. Xiong, C. Yang, B. Johannessen, R. Liu, H.-Y. Hsu, Q. Gu, M. K. H. Leung and J. Shang, Atomically dispersed iron metal site in a porphyrin-based metal–organic framework for photocatalytic nitrogen fixation, ACS Nano, 2021, 15, 9670–9678 CrossRef CAS PubMed
.
- Z. Chen, K. Mou, X. Wang and L. Liu, Nitrogen-doped graphene quantum dots enhance the activity of Bi2O3 nanosheets
for electrochemical reduction of CO2 in a wide negative potential region, Angew. Chem., Int. Ed., 2018, 57, 12790–12794 CrossRef CAS PubMed
.
- C. Dong, S. Lu, S. Yao, R. Ge, Z. Wang, Z. Wang, P. An, Y. Liu, B. Yang and H. Zhang, Colloidal synthesis of ultrathin monoclinic BiVO4 nanosheets for Z-scheme overall water splitting under visible light, ACS Catal., 2018, 8, 8649–8658 CrossRef CAS
.
- D. Jiang, W. Ma, P. Xiao, L. Shao, D. Li and M. Chen, Enhanced photocatalytic activity of graphitic carbon nitride/carbon nanotube/Bi2WO6 ternary Z-scheme heterojunction with carbon nanotube as efficient electron mediator, J. Colloid Interface Sci., 2018, 512, 693–700 CrossRef CAS PubMed
.
- S. Yang, X. Zhu and S. Peng, Prospect prediction of terminal clean power consumption in china via LSSVM algorithm based on improved evolutionary game theory, Energies, 2020, 13(8), 1–17 CrossRef PubMed
.
- H. Li, J. Shang, Z. Ai and L. Zhang, Efficient visible light nitrogen fixation with BiOBr nanosheets of oxygen vacancies on the exposed {001} Facets, J. Am. Chem. Soc., 2015, 137, 6393–6399 CrossRef CAS PubMed
.
- S. Wang, F. Ichihara, H. Pang, H. Chen and J. Ye, Nitrogen fixation reaction derived from nanostructured catalytic materials, Adv. Funct. Mater., 2018, 28, 1803309 CrossRef
.
- S. C. Jesudass, S. Surendran, J. Y. Kim, T.-Y. An, G. Janani, T.-H. Kim, J. K. Kim and U. Sim, Pathways of the electrochemical nitrogen reduction reaction: From ammonia synthesis to metal-N2 batteries, Electrochem. Energy Rev., 2023, 6, 27 CrossRef CAS
.
- S. Mallikarjun Sharada, R. K. B. Karlsson, Y. Maimaiti, J. Voss and T. Bligaard, Adsorption on transition metal surfaces: Transferability and accuracy of DFT using the ADS41 dataset, Phys. Rev. B, 2019, 100, 035439 CrossRef
.
- A. J. R. Hensley, K. Ghale, C. Rieg, T. Dang, E. Anderst, F. Studt, C. T. Campbell, J.-S. McEwen and Y. Xu, DFT-based method for more accurate adsorption energies: An adaptive sum of energies from RPBE and vdW density functionals, J. Phys. Chem. C, 2017, 121, 4937–4945 CrossRef CAS
.
- R. B. Araujo, G. L. S. Rodrigues, E. C. dos Santos and L. G. M. Pettersson, Adsorption energies on transition metal surfaces: towards an accurate and balanced description, Nat. Commun., 2022, 13, 6853 CrossRef CAS PubMed
.
- E. Skúlason, T. Bligaard, S. Gudmundsdóttir, F. Studt, J. Rossmeisl, F. Abild-Pedersen, T. Vegge, H. Jónsson and J. K. Nørskov, A theoretical evaluation of possible transition metal electro-catalysts for N2 reduction, Phys. Chem. Chem. Phys., 2012, 14, 1235–1245 RSC
.
- M. Li, Q. Fang, X. Zhao, C. Xia, A. Wang, Y. Xie, F. Ma, J. She and Z. Deng, Theoretical screening of a graphyne-supported transition metal single-atom catalyst for the N2 reduction reaction, Phys. Chem. Chem. Phys., 2023, 25, 18224–18232 RSC
.
- Y. Abghoui, A. L. Garden, J. G. Howalt, T. Vegge and E. Skúlason, Electroreduction of N2 to ammonia at ambient conditions on mononitrides of Zr, Nb, Cr, and V: A DFT guide for experiments, ACS Catal., 2016, 6, 635–646 CrossRef CAS
.
- V. Giulimondi, S. Mitchell and J. Pérez-Ramírez, Challenges and opportunities in engineering the electronic structure of single-atom catalysts, ACS Catal., 2023, 13, 2981–2997 CrossRef CAS PubMed
.
- C. Choi, S. Back, N.-Y. Kim, J. Lim, Y.-H. Kim and Y. Jung, Suppression of hydrogen evolution reaction in electrochemical N2 reduction using single-atom catalysts: A Computational guideline, ACS Catal., 2018, 8, 7517–7525 CrossRef CAS
.
- C. Ling, Y. Ouyang, Q. Li, X. Bai, X. Mao, A. Du and J. Wang, A General two-step strategy–based high-throughput screening of single atom catalysts for nitrogen fixation, Small Methods, 2019, 3, 1800376 CrossRef
.
- X. Guo, J. Gu, S. Lin, S. Zhang, Z. Chen and S. Huang, Tackling the activity and selectivity challenges of electrocatalysts toward the nitrogen reduction reaction via atomically dispersed biatom catalysts, J. Am. Chem. Soc., 2020, 142, 5709–5721 CrossRef CAS PubMed
.
- Z. Allal, H. N. Noura, O. Salman and K. Chahine, Machine learning solutions for renewable energy systems: Applications, challenges, limitations, and future directions, J. Environ. Manage., 2024, 354, 120392 CrossRef PubMed
.
- P. Thakkar, S. Khatri, D. Dobariya, D. Patel, B. Dey and A. K. Singh, Advances in materials and machine learning techniques for energy storage devices: A comprehensive review, J. Energy Storage, 2024, 81, 110452 CrossRef
.
- H. Yang, Z. He, M. Zhang, X. Tan, K. Sun, H. Liu, N. Wang, L. Guan, C. Wang, Y. Wan, W. Wang, H. Hu and M. Wu, Reshaping the material research paradigm of electrochemical energy storage and conversion by machine learning, EcoMat, 2023, 5, e12330 CrossRef CAS
.
- J. Liu, W. Luo, L. Wang, J. Zhang, X.-Z. Fu and J.-L. Luo, Toward excellence of electrocatalyst design by emerging descriptor-oriented machine learning, Adv. Funct. Mater., 2022, 32, 2110748 CrossRef CAS
.
- Y. Gu, Q. Zhu, Z. Liu, C. Fu, J. Wu, Q. Zhu, Q. Jia and J. Ma, Nitrogen reduction reaction energy and pathways in metal-zeolites: deep learning and explainable machine learning with local acidity and hydrogen bonding features, J. Mater. Chem. A, 2022, 10, 14976–14988 RSC
.
- M. Kim, B. C. Yeo, Y. Park, H. M. Lee, S. S. Han and D. Kim, Artificial intelligence to accelerate the discovery of N2 electroreduction catalysts, Chem. Mater., 2020, 32, 709–720 CrossRef CAS
.
- C. Sutton, L. M. Ghiringhelli, T. Yamamoto, Y. Lysogorskiy, L. Blumenthal, T. Hammerschmidt, J. R. Golebiowski, X. Liu, A. Ziletti and M. Scheffler, Crowd-sourcing materials-science challenges with the NOMAD 2018 Kaggle competition, npj Comput. Mater., 2019, 5, 111 CrossRef
.
- A. Belsky, M. Hellenbrandt, V. L. Karen and P. Luksch, New developments in the Inorganic Crystal Structure Database (ICSD): accessibility in support of materials research and design, Acta Crystallogr., Sect. B: Struct. Sci., 2002, 58, 364–369 CrossRef PubMed
.
- R. Allmann and R. Hinek, The introduction of structure types into the inorganic crystal structure database ICSD, Acta Crystallogr., Sect. A: Found. Crystallogr., 2007, 63, 412–417 CrossRef CAS PubMed
.
- A. N. Abdalla, M. S. Nazir, H. Tao, S. Cao, R. Ji, M. Jiang and L. Yao, Integration of energy storage system and renewable energy sources based on artificial intelligence: An overview, J. Energy Storage, 2021, 40, 102811 CrossRef
.
- A. N. Singh, R. Anand, M. Zafari, M. Ha and K. S. Kim, Progress in single/multi atoms and 2D-nanomaterials for electro/photocatalytic nitrogen reduction: experimental, computational and machine learning developments, Adv. Energy Mater., 2024, 14, 2304106 CrossRef CAS
.
- B. B. Hoar, S. Lu and C. Liu, Machine-learning-enabled exploration of morphology influence on wire-array electrodes for electrochemical nitrogen fixation, J. Phys. Chem. Lett., 2020, 11, 4625–4630 CrossRef CAS PubMed
.
|
This journal is © the Partner Organisations 2025 |
Click here to see how this site uses Cookies. View our privacy policy here.