DOI:
10.1039/D4QO02079K
(Review Article)
Org. Chem. Front., 2025, Advance Article
Recent advances in visible light-driven phosphine-mediated transformations
Received
5th November 2024
, Accepted 26th December 2024
First published on 31st December 2024
Abstract
The literature on the use of trivalent phosphines in the activation of electrophilic unsaturated reagents is large. However, the application of such derivatives for the generation of radical intermediates has received attention only in the past decade. This review provides a detailed description of the phosphine-mediated-photocatalyzed and photoredox-catalyzed protocols for the formation of carbon–carbon and carbon–heteroatom bonds proposed in the last decade.
| Jiaxu Feng was born in 1993 in Shanxi, China. She received her PhD degree from Nankai University in 2020 under the guidance of Prof. You Huang. She joined the Tianjin University of Science & Technology in 2020. Her research interests focus on organic small-molecular catalysis and photochemistry. |
| Luca Nicchio was born in 1998 in Peschiera del Garda, Italy. In 2020, he received his Master's degree in Chemistry at the University of Pavia (Italy) under the supervision of Prof. Maurizio Fagnoni (Photogreen Lab). Luca is currently a PhD student in a joint PhD program between Université Paris-Saclay and University of Pavia (Université Paris-Saclay International Joint PhD Program-Cotutelle 2022), under the supervision of Luc Neuville (DR2) and Prof. Stefano Protti. His research is focused on the development of new and green synthetic approaches through visible-light generation of radical intermediates from arylazo sulfones and arylazo sulfonates. |
| Liting Liu was born in 1998 in Guangxi, China. She received her Bachelor's degree from Tianjin University of Science & Technology in 2020 and then pursued her Master's degree at Tianjin University of Science & Technology under the supervision of Prof. Kui Lu. Her research interests focus on photochemistry and organofluorine chemistry. |
| Yingying Wu was born in 2001 in Hebei, China. She received her Bachelor's degree from Langfang Normal University in 2023 and then pursued her Master's degree at Tianjin University of Science & Technology under the supervision of Dr Jiaxu Feng. Her research interests focus on organic small-molecular catalysis and photochemistry. |
| Kui Lu was born in Jiangxi Province, China, in 1981. He received his BS degree from China Agricultural University in 2002 and then a Ph.D. degree in organic chemistry from Peking University, China, in 2007 under the supervision of Professor Zhen Yang. After postdoctoral research in the laboratory of Professor Ohyun Kown at the University of California, Los Angeles, U.S.A., he came back to China and worked as a group leader of medicinal chemistry in Bioduro Co., Ltd. In 2011, he started his independent career as an associate professor at Tianjin University of Science & Technology and was promoted to full professor in 2020. His research involves photochemistry, organosulfur chemistry and organofluorine chemistry. |
| Stefano Protti completed his PhD in Pavia (2007). Later he moved to LASIR Laboratory (Lille, France) at the iBitTec-S laboratory (CEA Saclay, France) carrying out studies on photocatalyzed oxidation reactions for energy storage; then, he moved to the University of Pavia, where he has served as Associate Professor since 2018. His research is mainly focused on the development of synthetic strategies driven by visible light. |
| Xia Zhao was born in Tianjin, China, in 1983. She received her BS degree from Nan Kai University in 2006, and then a Ph.D. degree in organic chemistry from Peking University, China, in 2011 under the supervision of Professor Jianbo Wang. After that she started her independent career as an assistant professor at Tianjin Normal University and was promoted to full professor in 2021. Her research interests focus on photochemistry and organosulfur chemistry. |
1. Introduction
Since the seminal experiments published in the early-1960s, the potential of trivalent phosphines as organocatalysts in synthesis has been intensively exploited.1 However, in a large part of the literature available, the nucleophilic addition of the phosphine moiety on an electrophilic reactant (e.g. olefins, alkynes or allenes) promotes the formation of a reactive zwitterion species, which is then converted into the desired product2–10 via electron-pair-transfer reactions. On the other hand, only a few strategies based on a single-electron-transfer (SET) mechanism have been proposed.11 However, these SET strategies are based on the successful application of phosphines as redox active cocatalysts in photoredox-catalyzed processes.12 Such premises have increased attention on the development of phosphine-promoted visible light-driven protocols, enabling chemical paths that are poorly or not accessible via thermal approaches. As described in Scheme 1, phosphine photo-organocatalysis has been reported to occur via three paths, namely:
(a) β-Scission of a phosphoranyl radical (path a). Trivalent phosphine undergoes photoinduced SET and the resulting radical cation PR3˙+ is trapped by a suitable (thio)alcohol R–XH. The resulting phosphoranyl radical releases a carbon-centred radical via β-scission of the C–X bond. The process is thermodynamically driven by the stoichiometric formation of a stable phosphine oxide or sulfide as the byproduct.
(b) α-Scission of a phosphoranyl radical (path b). The α-scission occurring in the phosphoranyl radical described in path b releases, via C–P bond homolytic cleavage, a carbon-centred radical. Such behavior is kinetically favored by the presence of an unpaired electron occupying a P–X antibonding orbital. The competition between paths a and b was described to depend strictly on the relative bond strengths of C–X and P–C bonds.13
(c) Formation of an electron donor acceptor (EDA) complex. In some cases, a visible light-absorbing EDA complex14 could be formed via the in situ interaction of electron-poor alkyl halides (e.g., perfluoroalkyl iodides) and trivalent phosphines via SET. The photoinduced homolytic cleavage of the C–halogen bond then affords the desired alkyl radical.14
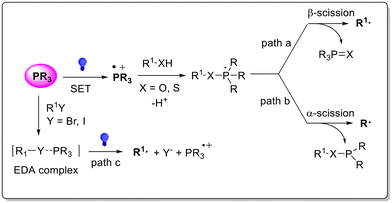 |
| Scheme 1 Conceptual overview of phosphine-mediated photoredox processes. | |
As all the examined paths have been applied to organic synthesis, we aim to provide an overview of the most recent applications of phosphines in the visible light-driven formation of carbon–carbon and carbon–heteroatom bonds. The classification is based on the first chemical bond formed, in accordance with the proposed mechanism. The use of continuous flow conditions as well as the application of the developed procedures in late-stage functionalization approaches have been considered and discussed in the following sections.
2. Formation of carbon–carbon bonds
2.1. Hydroalkylation reactions
The photocatalytic NaI-PPh3 mediated decarboxylative alkylation of silyl enol ether 2.2 and redox-active esters 2.1 affords α-substituted acetophenones 2.3 in discrete-to-satisfactory yield. The reaction occurs under transition metal-free conditions (Scheme 2) in the presence of a stoichiometric excess of NaI.15 The same strategy also promotes the Minisci-type alkylation of N-heterocycles and an analogous enantioselective α-amino alkylation with the assistance of a chiral phosphoric acid in catalytic amounts.
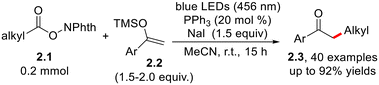 |
| Scheme 2 Light-driven, photocatalytic NaI-PPh3-mediated decarboxylative alkylation of silyl enol ethers and nitrogen-based heterocycles. | |
Shi and co-workers applied a photoredox-promoted hydroalkylation strategy to the preparation of a library of DNA-tagged alkenes using xanthates as the alkyl radical precursors in the presence of Ir[FCF3(CF3)ppy]2(dtbbpy)PF6 as the photoredox catalyst.16 Phosphine organocatalysis was merged with photoredox catalysis in the conversion of ynoates 3.1 to electron-poor olefins 3.3 under metal-free conditions, as described in Scheme 3.17 The procedure involves a Giese-like coupling of 3.1 with alkylating agents trifluoroborate salts 3.2 in the presence of 4-CzTPN as the photoredox catalyst.
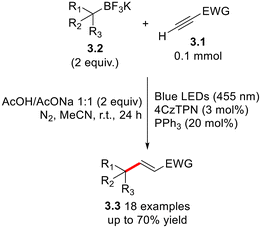 |
| Scheme 3 Giese-like functionalization of ynoates 3.2 by dual phosphine organocatalysis and photoredox catalysis. | |
Recently, mercaptans 4.2 have been proposed as electron-deficient precursors of carbon-centred radicals in the hydroalkylation of styrenes 4.1 under blue-light irradiation and in the presence of transition metal-based species [Au2(μ-dppm)2]Cl2 (Scheme 4a).18 Such a versatile strategy was successfully applied to the functionalization of small amino-acid fragments as well as the preparation of polymers (an example is given in Scheme 4b). The same target was achieved under photocatalyst-free conditions by Su and co-workers.19 They exploited the partial oxidation of triphenyl phosphine to the corresponding triphenylphosphine oxide which, in turn, forms an EDA complex with the yet unreacted PPh3; the species is responsible for both the absorption of light and initiation of the radical chain process.19
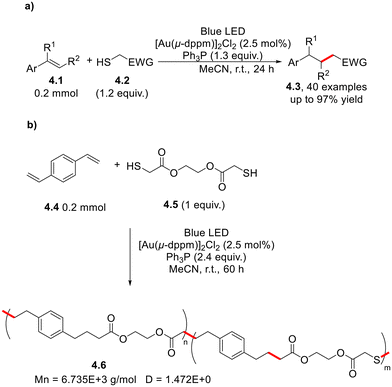 |
| Scheme 4 Visible light-driven gold-catalyzed hydroalkylation of styrenes 4.1 in (a) synthesis and (b) polymerization processes. | |
Perfluoroalkyl radicals have been generated and, in turn, employed for the hydroalkylation of electron-poor olefins (including fluoxetine and sertraline derivatives) from the hydrated form of hexafluoroacetone (5.2, Scheme 5) and trifluoro-acetaldehyde.20 In this case, a key role is played by the phosphoranyl radical 5.4, arising from the coupling between the triphenylphosphine radical cation and 5.2.20
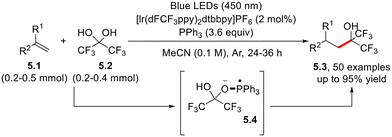 |
| Scheme 5 Photoredox-catalyzed phosphine-mediated bifunctionalization of electron-deficient alkenes. | |
In situ deprotonation of phenol 6.4 generates the o-phosphinophenolate anion, which acts as a photoredox organocatalyst for activating trifluoromethyl groups in trifluoroacetamides, acetates, trifluoromethyl (hetero)arenes (6.1, Scheme 6) and even trifluoroalkanes. This approach enables the difluoroalkylation of non-activated alkenes and polyfunctionalized aromatics, including the antipsychotic compound trifluoroperazine.21
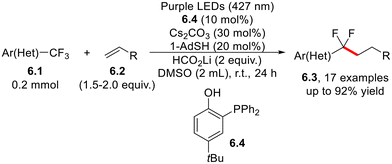 |
| Scheme 6 Photoredox-organocatalyzed difluoro(hetero)arylation and difluoroalkylation of non-activated olefins. | |
The difunctionalization of multiple bonds is one of the most widely adopted approaches for constructing structurally complex molecules.22–25 In this context, the 1,2-iodoperfluoroalkylation of alkenes via C–C and C–I bond formation was described to occur under visible light (461 nm) irradiation of a mixture of olefin 7.1 (Scheme 7), perfluoroiodoalkanes 7.2 and a catalytic amount of tri-tertbutylphosphine. The reaction mechanism involves an atom-transfer radical addition (ATRA) chain path initiated by the formation of an EDA complex between 7.2 and the tBu3P organocatalyst.26 The same synthetic target was achieved by having recourse to a merged photoredox/copper catalysed strategy, in the presence of fac-Ir(ppy)3 as the photoredox catalyst and Cu(MeCN)4− as the cocatalyst, respectively.27
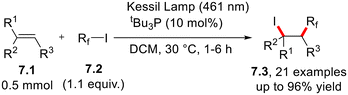 |
| Scheme 7 Visible light-induced photochemical 1,2-iodoperfluoroalkylation of alkenes. | |
A 1,3-carbobromination strategy for the conversion of allyl carboxylates 8.1 to isopropyl carboxylates (sIPC) 8.3 was reported recently in the literature (Scheme 8a).28 The suggested non-chain-radical mechanism is shown in Scheme 8b. It involves the formation of a visible light-absorbing EDA complex 8.4 between the phosphine (dppm) and 8.2. Upon irradiation, 8.4 decompose in the bromo-phosphine 8.6 and alkyl radical 8.5 (path a). The latter is trapped by the olefin moiety in allyl carboxylate 8.1 (path b). The obtained secondary alkyl radical 8.7 undergoes 1,2-radical migration (1,2-RaM) to generate the more stable intermediate 8.8 (path c). Subsequently, bromo-atom transfer from 8.6 to 8.8 affords the desired product (path d).28
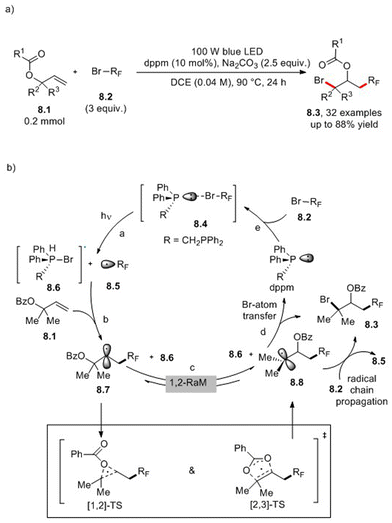 |
| Scheme 8 Photoinduced and phosphine-catalyzed difunctionalization of allyl carboxylates. | |
2.2. Acylation reactions
Aliphatic ketones play a crucial part in post-transformation and late-stage functionalization processes, making them privileged targets in organic synthesis.29,30 Symmetric and asymmetric dialkyl ketones 9.3 have been obtained via Ir(III)-photocatalyzed hydroacylation of styrenes (9.1, Scheme 9) by aliphatic acids 9.2 in the presence of diphenyl methyl phosphine, as reported in Scheme 9.31 In this case, a key role is played by the phosphoranyl radical 9.4 which, in turn, undergoes β-scission to release the desired acyl radical. Analogously, aromatic carboxylic acids have been employed as precursors of acyl radicals in the preparation of α,α′-diaryl carbonyls via photoredox-catalyzed acylation 1,6-conjugate addition to p-quinone derivatives.32 By following an analogous pathway, acyl oximes have been employed as the acyl radical precursors in the hydroacylation of 1,1-diarylethylenes and α-trifluoromethyl styrenes.33
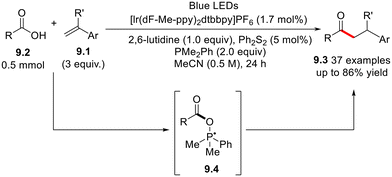 |
| Scheme 9 Hydroacylation of styrenes 9.1 by carboxylic acids. | |
The photoinduced phosphine-catalyzed cyclization of bromodifluoroacyl arenes 10.1 with olefins has been applied recently to the preparation of a wide range of cyclic gem-difluoroacyl compounds 10.3 under photocatalyst-free conditions (Scheme 10).34
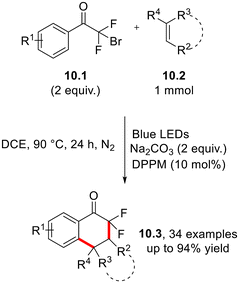 |
| Scheme 10 Blue light-mediated, photocatalyst-free preparation of cyclic ketones 10.3. | |
Anhydrides 11.2 have been employed as acylating radical agents in the photocatalyzed conversion of alkenes 11.1 into 1,4-dicarbonyl derivatives (11.3, Scheme 11). In the proposed mechanism, the photoexcited photocatalyst (lr[dF(CF3)ppy]2(dtbbpy)PF6)* undergoes reductive quenching by triphenyl phosphine. Then, the resulting radical cation PPh3˙+ is trapped by 11.2 to form the complex 11.4, which allows the otherwise unfavorable reduction of the carboxylic moiety in 11.2 (Ered1/2 = −1.41 V vs. SCE) by Ir(II). The acyl radical 11.6 arising from the decomposition of intermediate 11.5 is, in turn, trapped by the C
C double bond of 11.1. The resulting radical 11.7 undergoes reduction to carbanion 11.8, which reacts with another molecule of 11.2 to form the diacylated product. In the presence of a proton source (e.g., water), 11.8 is finally converted into the corresponding hydroacylated derivative 11.9.35
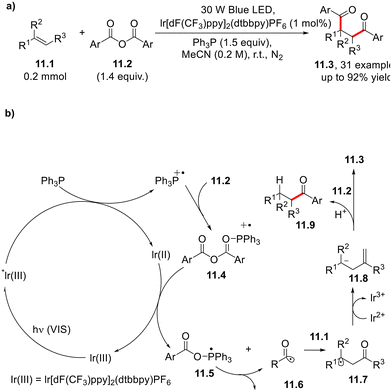 |
| Scheme 11 (a) Visible light-driven photoredox-catalyzed 1,2-difunctionalization of olefins. (b) Suggested mechanism. | |
1,6-Dicarbonyl derivatives 12.336–38 are suitable, via a regioselective 1,6-addition of acyl radicals (in turn generated from carboxylic acids 12.1 under Ir(II) photoredox-catalyzed conditions), onto electron-deficient 1,3-dienes (12.2, Scheme 12a).39 If exposed to visible light, the excited photoredox catalyst undergoes oxidative quenching by 12.2, generating the radical anion 12.6 (Scheme 12b, paths a and b). As a result, triarylphosphine is oxidized through a SET, while restoring the starting photoredox catalyst (path c). The resulting phosphoranyl radical intermediate 12.4 (path d) decomposes and releases the acyl radical 12.5 (path e), which then couples with 12.6 (path f). Protonation of the so-generated carbanion 12.7 by water (path g) affords the desired product 12.3.39
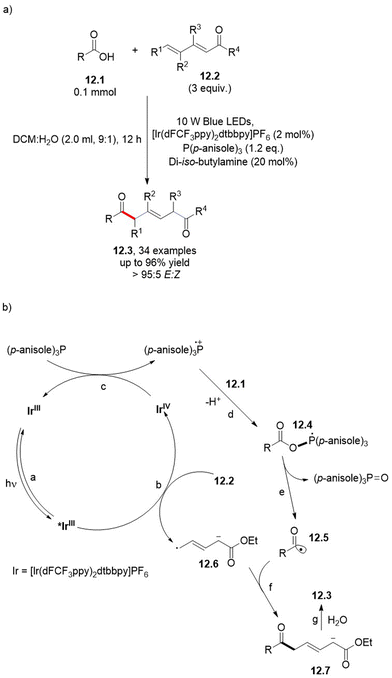 |
| Scheme 12 Regioselective, photoredox-catalyzed decarboxylative acylation of electron-poor 1,3-dienes 12.2. | |
A divergent approach for the selective preparation of alkyl nitriles (13.2, Scheme 13a), alkenyl nitriles (13.3) and alkyl dinitriles (13.4) from alkynes has been proposed by Chu and co-workers.40 As shown in Scheme 13a, the choice of phosphine is critical in determining the reaction outcome. Notably, a wide range of polyfunctionalized substrates, including warfarin analogues, pharmaceutics, sugars and vitamin E, can be selectively functionalized under the tunable conditions (some examples are depicted in Scheme 13b).
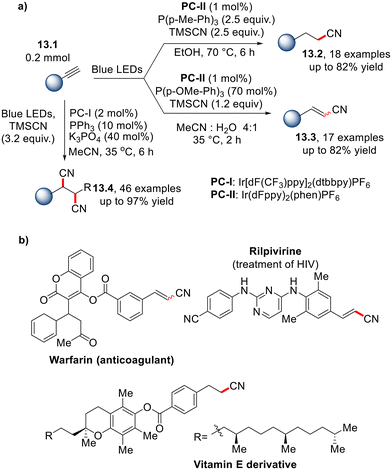 |
| Scheme 13 (a) Phosphine/photoredox-catalyzed divergent cyanation of alkynes and (b) selected applications. | |
2.3. Arylations
The incorporation of fluorine atoms and fluorine-containing functional groups into complex (hetero)aromatic cores is a flourishing research area in view of the opportunity to “fine tune” key physicochemical properties, including (among others), solubility, metabolic stability and bioavailability.41–48 In this context, the use of phosphine as a radical initiator in the perfluoroalkylation of electron-rich heterocycles 14.1 (including indoles) has been proposed only recently. In the case described by Jin and co-workers,49 and suitable for Scheme 14a, an EDA complex (14.4) between the chosen perfluoroalkyl iodide 14.2 and the phosphine catalyst is involved. Visible light-irradiation promotes a SET, and the so generated perfluoroalkyl radical RF˙ which is, in turn, trapped by the heteroaromatic core. Thus, obtained radical species 14.5 is oxidized by another molecule of 14.2 to release, after deprotonation of the Wheland intermediate 14.6, the desired product 14.3 and so restarting, simultaneously, the radical chain. The protocol was found to be successful also when starting from indoles conjugated to bioactive fragments such as zomipitran and melatonine.49
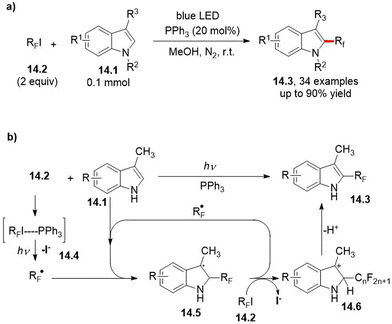 |
| Scheme 14 Visible light-driven phosphine-catalyzed perfluoroalkylation of indoles. | |
Analogously, the introduction of a gem-difluoromethyl group into alkenes and (hetero)cyclic cores (including dimethyl uracil) has been reported to occur via a visible light-promoted phosphine-catalyzed difluoroalkylation strategy.50
As mentioned above, nitrile is one of the most prevalent and versatile units in organic synthesis, acting as a precursor of different functional groups, including amines, aldehydes, amidines, ketones, carbamates and carboxylic acids.51 The research group of Yi accomplished an intriguing photoredox-neutral ring-opening pyridylation of cyclic oximes 15.1 (Scheme 15) by cyanopyridines 15.2 to afford a wide array of pyridyl-alkylnitriles 15.3 with satisfactory selectivity (Scheme 15).52
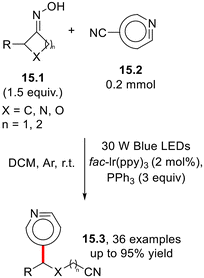 |
| Scheme 15 Photoredox-neutral ring-opening pyridylation of cyclic oximes. | |
2.4. Extrusion reactions
In 2023, Tobisu and co-workers described a nickel/Ir(II) photoredox/phosphine-promoted intramolecular C–C coupling occurring in allyl esters 16.1 for the preparation of asymmetric ketones 16.2. in good yield (Scheme 16a).53 The same conditions have been applied to obtain a cyclic ketone (16.4, Scheme 16b) via functionalization of allyl esters bearing a tethered alkene moiety (16.3).53
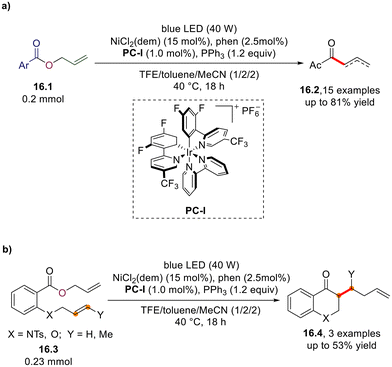 |
| Scheme 16 Nickel/photoredox/phosphine-catalyzed preparation of ketones from allyl esters. | |
In 2019, Xu and co-workers described a single example of phosphine/iodide-based photocatalytic decarboxylative [2 + 2 + 1] annulation of 1,6-enynes and N-hydroxyphthalimide esters, providing the corresponding cyclopenta[a]indene 17.3 in 60% yield (Scheme 17). The procedure can be considered to be a transition metal-free alternative to fac-Ir(ppy)3-catalyzed approach described in the same study.54
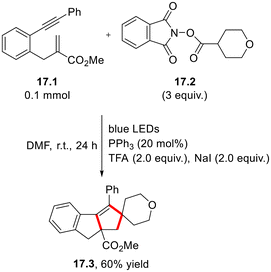 |
| Scheme 17 Preparation of spyroderivative 17.3 via phosphine/iodide-based photocatalytic decarboxylative [2 + 2 + 1] annulation. | |
3. Formation of carbon–heteroatom bonds
3.1. C–S bond formation
The incorporation of a trifluoromethylthio moiety (–SCF3) in pharmaceuticals and agrochemicals enables fine tuning of their lipophilicity and electronic properties.44,47,55 Qing and co-workers disclosed a facile and mild strategy for the Ir(II)-photoredox-catalyzed hydrotrifluoromethylthiolation of unactivated alkenes (18.1) or alkynes (18.2) using trifluoromethanesulfonic anhydride (Tf2O) as the SCF3 radical precursor in the presence of H2O as the proton source (Scheme 18).56
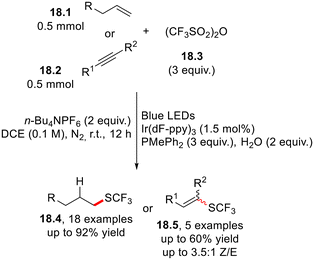 |
| Scheme 18 Visible light-mediated hydrotrifluoromethylthiolation of unactivated alkenes and alkynes. | |
The visible light-induced hydrosulfonylation of alkenes in the presence of N-amidopyridium salts 19.1 as precursors of the sulfonyl radical has recently been proposed in a photocatalyst-free fashion via the formation of a photoreactive EDA complex between 19.1 and triphenyl phosphine (or HCO3− anion, Scheme 19). Notably, the protocol was extended to the functionalization of bioactive molecules such as estrone and fructopiranose.57
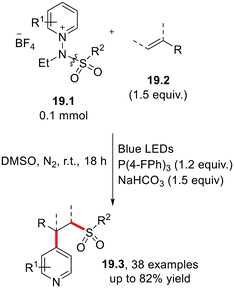 |
| Scheme 19 1,2-Difunctionalization of olefins via blue light-driven C–aryl and C–S bond formation. | |
Disulfides 20.1 have been employed as the sulfur source in the photoredox-catalyzed, alkoxy phosphine-mediated thioesterification of carboxylic acids 20.2 that was successfully applied to the functionalization of complex pharmaceutical-derived carboxylic acids, including the cholesteryl ester transfer protein inhibitor dalcetrapib (Scheme 20).58
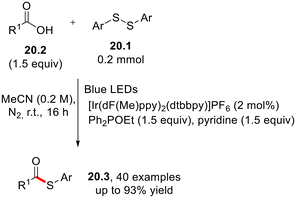 |
| Scheme 20 Phosphine-mediated thioesterification of carboxylic acids 20.2. | |
In 2021, Jiang and co-workers reported the photoredox catalytic phosphite-mediated deoxygenation and Wolff rearrangement of 1,2-diketones via ketenes to afford the corresponding (thio)esters (21.4, Scheme 21, path a) using the pyrazine derivative DPZ as the visible light-absorbing photocatalyst.59 The same approach is on the basis of the preparation of β-lactams 21.5 (path b) via a Staudinger [2 + 2] cycloaddition with imines.60
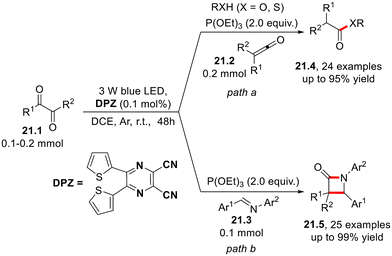 |
| Scheme 21 Photoredox catalytic phosphite-mediated deoxygenation and Wolff rearrangement of α-diketones. | |
3.2. C–N bond formation
A photoredox-catalyzed anti-Markovnikov hydroamination of olefins 22.2 by sulphonamides 22.1 in the presence of tricyclohexylphosphine and TRIP-SH as the cocatalysts was described recently (Scheme 22a).61 As illustrated in Scheme 22b, the mechanism involves a merged photoredox/hydrogen atom transfer (HAT)/phosphine organocatalysis cycle. The P–N bond α-cleavage occurring in the radical intermediate 22.4, in turn generated by coupling of PCy3˙+ with 22.1, plays a key role in the generation of the amido radical 22.5 which, in turn, is trapped by 22.2 to afford the intermediate 22.6. The latter undergoes HAT with TRIP-SH to release the desired product.61 A similar visible light-driven anti-Markovnikov hydroamination of alkenes (including cyclic olefins and vinyl ethers) was carried out using N-hydroxyphthalimide as the functionalizing agent and triethyl phosphite as the cocatalyst.62
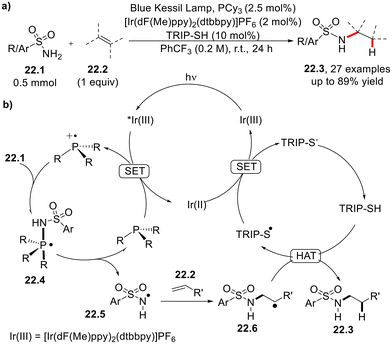 |
| Scheme 22 Visible light-driven anti-Markovnikov hydroamination of olefins. | |
The synthesis of pyrrolines from γ,δ-unsaturated oximes (Scheme 23) was recently described to take place in the presence of Ir(dF(CF3)ppy)2(dtbbpy)PF6, tris(4-methoxyphenyl)phosphine and 4-methoxyphenyl disulphide as the photoredox catalysts and cocatalysts, respectively.63 This strategy is based on the reductive quenching of the photoexcited iridium catalyst by tris(4-methoxyphenyl)phosphine (Ep/2[Ar3P˙+/Ar3P] = +0.87 V vs. SCE), which is converted to the corresponding radical cation and adds itself onto the oxime moiety. The so-generated phosphoranyl radical 23.3 undergoes N–O bond β-cleavage to deliver the iminyl radical 23.4 which, in turn, undergoes intramolecular 5-exo-trig cyclization. HAT from 4-methoxythiophenol (arising from the corresponding disulfide) to the carbon-centered radical 23.5 affords the desired product.
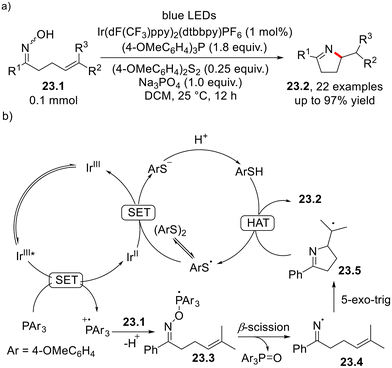 |
| Scheme 23 Light-driven intramolecular hydroimination of oximes 23.1. | |
N-Hydroxyl azoles 24.2 (Scheme 24) were successfully employed in the regioselective hydroazolylation of alkenes under Iridium photoredox catalysis in the presence of triphenylphosphine. The N–O bond cleavage that occurs in the phosphoranyl radical cation 24.4 has been suggested to be the key step in the reaction mechanism. An analogous mechanism was exploited for the preparation of N-arylphthalimides via the photocatalyzed radical N-arylation of (hetero)aryl derivatives by N-hydroxyphthalimides.64
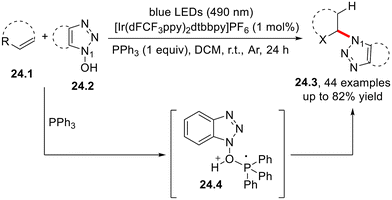 |
| Scheme 24 Photoredox-catalyzed hydroazolylation of alkenes. | |
The development of innovative strategies for the amidation of carboxylic acids is of interest in organic synthesis due to the widespread presence of amide moieties in fine chemicals, peptides, as well as in polymeric materials.65–70
In this context, we noted the preparation of differently substituted amides via a merged photoredox/cobaloxime-catalyzed deoxygenative activation of carboxylic acid.71 The protocol, which requires PPh3 as the redox active cocatalyst, has been employed on different biorelevant substrates and was considered for late-stage functionalization. Furthermore, the process has been optimized under continuous-flow conditions for the preparation of peptides in satisfactory yield (Scheme 25).71,72 Carboxylic acids have been used also as the acyl radical precursor in the preparation of differently substituted acyl hydrazides by deoxygenative hydroacylation of azobenzenes.73
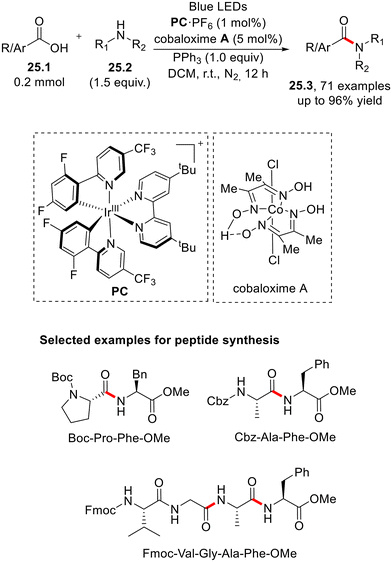 |
| Scheme 25 Merged photoredox and cobaloxime preparation of amides via deoxygenative amidation of carboxylic acids. | |
3.3. Carbon–halogen bond formation
Carboxylic acid derivatives have been employed in the preparation of halogenated substrates via an acyl radical. N-(Acyloxy)phthalimides have been subject to triphenylphosphine-photocatalyzed iododecarboxylation using lithium iodide as the iodine source to afford a wide range of both cyclic and acyclic alkyl iodides (26.2, Scheme 26).74 Notably, if an organic base (DBU) is added, 26.2 undergoes elimination to afford the corresponding alkene in a one-pot fashion.74
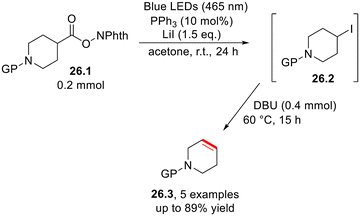 |
| Scheme 26 Triphenylphosphine-photocatalyzed iododecarboxylation of N-(acyloxy)phthalimide. | |
3.4. Carbon–hydrogen bond formation
In 2023, Studer and co-workers established the photocatalyzed activation of water by the interaction of a phosphine radical, which could provide an H atom to react further with alkenes (27.1 or 27.2) under mild conditions (Scheme 27).75 This transfer hydrogenation methodology has been applied to the reduction of naphthalenes 27.5. Furthermore, photochemical skeletal editing of 2-substituted quinolines can be achieved to afford 2,3-disubstituted indoles via a hydrogenative rearrangement in slightly modified conditions.75
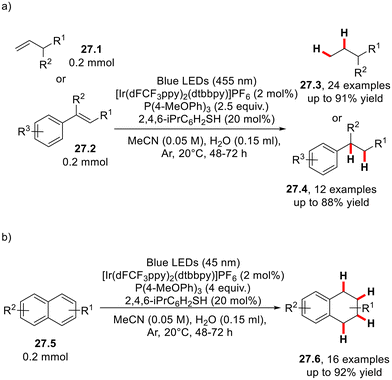 |
| Scheme 27 Photocatalyzed activation of water and further application. | |
4. Conclusions
In recent years, radical phosphine chemistry has emerged as an elegant and prominent strategy for broadening the horizons of (visible) light-driven methodologies. Attention has been focused on the design of late-stage functionalization protocols for pharmaceutical design and synthesis of bioactive and natural molecules. Despite the advancements reported in the literature, there are several drawbacks that have not been solved. This is because the design of stereoselective processes is still underdeveloped and the use of triarylphosphines in stoichiometric excess56,75 involves the release of rather noxious phosphorous-based byproducts. In this context, research should focus on methodologies that employ trisubstituted phosphines as effective organocatalysts (e.g., where an EDA complex with an electron poor alkyl halide is formed)28 as a promising strategy to overcome these limitations.
Data availability
No primary research results, software or code have been included, and no new data were generated or analysed as part of this review.
Conflicts of interest
There are no conflicts of interest to declare.
Acknowledgements
K. L. and X. Z. thank National Natural Science Foundation of China (22077095 and 22277089, respectively). L. N. thanks IDEX Paris-Saclay, “ADI 2022” ANR-11-IDEX-0003-02 and Fondazione Cariplo (Photo and Mechano-Chemistry for the Upgrading of Agro- and Sea-food Waste to advanced polymers and nanocarbon materials, CUBWAM, project 2021-0751) for doctoral fellowships. L. N. and S. P. acknowledge support from the Ministero dell'Università e della Ricerca (MUR) and the University of Pavia through the program “Dipartimenti di Eccellenza 2023–2027”. S. P. is grateful to the Italian Ministry for Universities and Research, PRIN-PNRR, “Xilonite” project P2022HSF3R, for financial support.
References
-
(a) J. L. Methot and W. R. Roush, Nucleophilic Phosphine Organocatalysis, Adv. Synth. Catal., 2004, 346, 1035–1050 CrossRef CAS;
(b) C. Gomez, J.-F. Betzer, A. Voituriez and A. Marinetti, Phosphine Organocatalysis in the Synthesis of Natural Products and Bioactive Compounds, ChemCatChem, 2013, 5, 1055–1065 CrossRef CAS;
(c) Y. Wei and M. Shi, Multifunctional Chiral Phosphine Organocatalysts in Catalytic Asymmetric Morita–Baylis–Hillman and Related Reactions, Acc. Chem. Res., 2010, 43, 1005–1018 CrossRef CAS PubMed;
(d) A. Fraile, A. Parra, M. Tortosa and J. Aleman, Organocatalytic transformations of alkynals, alkynones, propriolates, and related electron-deficient alkynes, Tetrahedron, 2014, 70, 9145–9173 CrossRef CAS;
(e) C. Xie, A. J. Smaligo, X.-R. Song and O. Kwon, Phosphorus-Based Catalysis, ACS Cent. Sci., 2021, 7, 536–558 Search PubMed;
(f) S. Xu and Z. He, Recent advances in stoichiometric phosphine-mediated organic synthetic reactions, RSC Adv., 2013, 3, 16885–16904 RSC;
(g) S. Xu and Y. Tang, Catalytic Approaches to Stoichiometric Phosphine-Mediated Organic Reactions, Lett. Org. Chem., 2014, 11, 524–533 CrossRef CAS Trivalent phosphines also found wide application as ligands in several catalytic strategies, see for recent reviews: Y. Sun, B. Wang and Z. Lu, Ligand relay catalysis: a newly emerged synthetic strategy, Org. Chem. Front., 2023, 10, 4146–4160 Search PubMed; S. S. Ng, W. H. Pang, O. Y. Yuen and C. M. So, Recent advances in the application of ligands in palladium-catalyzed chemoselective coupling reactions at C–Br, C–OTf, and C–Cl sites, Org. Chem. Front., 2023, 10, 4408–4436 Search PubMed.
- Y. Huang, J. Liao, W. Wang, H. Liu and H. Guo, Synthesis of heterocyclic compounds through nucleophilic phosphine catalysis, Chem. Commun., 2020, 56, 15235–15281 RSC.
- P. Karanam, G. M. Reddy, S. R. Koppolu and W. Lin, Recent topics of phosphine-mediated reactions, Tetrahedron Lett., 2018, 59, 59–76 CrossRef CAS.
- H. Guo, Y. C. Fan, Z. Sun, Y. Wu and O. Kwon, Phosphine Organocatalysis, Chem. Rev., 2018, 118, 10049–10293 CrossRef CAS.
- H. Li and Y. Lu, Enantioselective Construction of All-Carbon Quaternary Stereogenic Centers by Using Phosphine Catalysis, Asian J. Org. Chem., 2017, 6, 1130–1145 CrossRef CAS.
- A. Voituriez and N. Saleh, From phosphine-promoted to phosphine-catalyzed reactions by in situ phosphine oxide reduction, Tetrahedron Lett., 2016, 57, 4443–4451 CrossRef CAS.
- W. Li and J. Zhang, Recent developments in the synthesis and utilization of chiral β-aminophosphine derivatives as catalysts or ligands, Chem. Soc. Rev., 2016, 45, 1657–1677 RSC.
- J.-L. Montchamp, Phosphinate Chemistry in the 21st Century: A Viable Alternative to the Use of Phosphorus Trichloride in Organophosphorus Synthesis, Acc. Chem. Res., 2014, 47, 77–87 CrossRef CAS PubMed.
- L.-W. Ye, J. Zhou and Y. Tang, Phosphine-triggered synthesis of functionalized cyclic compounds, Chem. Soc. Rev., 2008, 37, 1140–1152 RSC; Y.-S. Chen, Y. Zheng, K. Tang, Z.-Z. Xie, Z.-P. Ye, M.-Z. Li, K. Chen, H.-Y. Xiang and H. Yang, [3 + 2] vs. [4 + 1] Annulation: revisiting mechanism studies on the phosphine-catalysed domino sequence of alkynoates and activated methylenes, Org. Biomol. Chem., 2022, 20, 4415–4420 RSC.
- An example merging thermal reactivity of trivalent phosphine and photocatalysis has been recently described: D. M. Whalley, L. Carlino, O. D. Putra, N. A. Anderson, S. C. Coote and O. Lorthioir, Merging Nucleophilic Phosphine Catalysis and Photocatalysis for the Rapid Assembly of 2-Oxabicyclo-[2.1.1]hexane Scaffolds from Feedstock Allyl Alcohols, Chem. Sci., 2024, 15, 19564–19570 RSC.
- D. Pan, G. Nie, S. Jiang, T. Li and Z. Jin, Radical reactions promoted by trivalent tertiary phosphines, Org. Chem. Front., 2020, 7, 2349–2371 RSC.
- K. Luo, W.-C. Yang and L. Wu, Photoredox Catalysis in Organophosphorus Chemistry, Asian J. Org. Chem., 2017, 6, 350–367 CrossRef CAS See for a recent example: K. Sedillo, F. Fan, R. R. Knowles and A. G. Doyle, Cooperative Phosphine-Photoredox Catalysis Enables N-H Activation of Azoles for Intermolecular Olefin Hydroamination, J. Am. Chem. Soc., 2024, 146, 20349–20356 CrossRef PubMed.
- Z.-Z. Xie, Z.-H. Liao, Y. Zheng, C.-P. Yuan, J.-P. Guan, M.-Z. Li, K.-Y. Deng, H.-Y. Xiang, K. Chen, X.-Q. Chen and H. Yang, Photoredox-catalyzed α-Scission vs. β-Scission of PR3–OH Radicals: Synthetic Utilizations and Mechanistic Studies, Chemrxiv, 2023, preprint, DOI:10.26434/chemrxiv-2023-c645s.
- P. Wawrzyniak, A. L. Fuller, A. M. Z. Slawin and P. Kilian, Intramolecular Phosphine-Phosphine Donor-Acceptor Complexes, Inorg. Chem., 2009, 48, 2500–2506 CrossRef CAS; Z. Yang, Y. Liu, K. Cao, X. Zhang, H. Jiang and J. Li, Synthetic reactions driven by electron-donor–acceptor (EDA) complexes, Beilstein J. Org. Chem., 2021, 17, 771–799 CrossRef PubMed; G. E. M. Crisenza, D. Mazzarella and P. Melchiorre, Synthetic Methods Driven by the Photoactivity of Electron Donor-Acceptor Complexes, J. Am. Chem. Soc., 2020, 142, 5461–5476 CrossRef PubMed; T. Tasnim, M. J. Ayodele and S. P. Pitre, Recent Advances in Employing Catalytic Donors and Acceptors in Electron Donor-Acceptor Complex Photochemistry, J. Org. Chem., 2022, 87, 10555–10563 CrossRef.
- M.-C. Fu, R. Shang, B. Zhao, B. Wang and Y. Fu, Photocatalytic decarboxylative alkylations mediated by triphenylphosphine and sodium iodide, Science, 2019, 363, 1429–1434 CrossRef CAS PubMed.
- P. R. Chheda, N. Simmons, D. P. Schuman and Z. Shi, Photoredox-Mediated Deoxygenative Alkylation of DNA-Tagged Alkenes with Activated Alcohols, Org. Lett., 2022, 24, 9514–9519 CrossRef CAS.
- J. Cao, A. Seitz, J. A. Forni, A. Polyzos and D. W. Lupton, Radical Coupling Initiated by Organophosphine Addition to Ynoates, Angew. Chem., Int. Ed., 2023, 62, e202303869 CrossRef CAS PubMed.
- L. Zhang, X. Si, Y. Yang, S. Witzel, K. Sekine, M. Rudolph, F. Rominger and A. S. K. Hashmi, Reductive C–C Coupling by Desulfurizing Gold-Catalyzed Photoreactions, ACS Catal., 2019, 9, 6118–6123 CrossRef CAS.
- J. Su, J.-N. Mo, X. Chen, A. Umanzor, Z. Zhang, K. N. Houk and J. Zhao, Generation of Oxyphosphonium Ions by Photoredox/Cobaloxime Catalysis for Scalable Amide and Peptide Synthesis in Batch and Continuous-Flow, Angew. Chem., Int. Ed., 2022, 61, e202112668 CrossRef CAS.
- Z.-Z. Xie, Y. Zheng, C.-P. Yuan, J.-P. Guan, Z.-P. Ye, J.-A. Xiao, H.-Y. Xiang, K. Chen, X.-Q. Chen and H. Yang, Photoredox-Catalyzed Deoxygenation of Hexafluoroacetone Hydrate Enables Hydroxypolyfluoroalkylation of Alkenes, Angew. Chem., Int. Ed., 2022, 61, e202211035 CrossRef CAS.
- C. Liu, N. Shen and R. Shang, Photocatalytic defluoroalkylation and hydrodefluorination of trifluoromethyls using o-phosphinophenolate, Nat. Commun., 2022, 13, 354 CrossRef CAS.
- F. Zhou, M. Li, H. Jiang and W. Wu, Recent Advances in Transformations Involving Electron-Rich Alkenes: Functionalization, Cyclization, and Cross-Metathesis Reactions, Adv. Synth. Catal., 2021, 363, 4841–4855 CrossRef CAS.
- H. Yao, W. Hu and W. Zhang, Difunctionalization of Alkenes and Alkynes via Intermolecular Radical and Nucleophilic Additions, Molecules, 2021, 26, 105 CrossRef CAS PubMed.
- M. Patel, B. Desai, A. Sheth, B. Z. Dholakiya and T. Naveen, Recent Advances in Mono- and Difunctionalization of Unactivated Olefins, Asian J. Org. Chem., 2021, 10, 3201–3232 CrossRef CAS.
- X. Chen, F. Xiao and W.-M. He, Recent developments in the difunctionalization of alkenes with C–N bond formation, Org. Chem. Front., 2021, 8, 5206–5228 RSC.
- L. Helmecke, M. Spittler, K. Baumgarten and C. Czekelius, Metal-Free Activation of C–I Bonds and Perfluoroalkylation of Alkenes with Visible Light Using Phosphine Catalysts, Org. Lett., 2019, 21, 7823–7827 CrossRef CAS.
- V. S. Kostromitin, V. V. Levin and A. D. Dilman, Dual Photoredox/Copper Catalyzed Fluoroalkylative Alkene Difunctionalization, J. Org. Chem., 2023, 88, 6252–6262 CrossRef CAS PubMed.
- G. Zhao, S. Lim, D. G. Musaev and M.-Y. Ngai, Expanding Reaction Profile of Allyl Carboxylates via 1,2-Radical Migration (RaM): Visible-Light-Induced Phosphine-Catalyzed 1,3-Carbobromination of Allyl Carboxylates, J. Am. Chem. Soc., 2023, 145, 8275–8284 CrossRef CAS PubMed.
- P. K. Prasad, R. N. Reddi and S. Arumugam, Recent methods for the synthesis of α-acyloxy ketones, Org. Biomol. Chem., 2018, 16, 9334–9348 RSC.
- M. A. Fischbach and C. T. Walsh, Assembly-Line Enzymology for Polyketide and Nonribosomal Peptide Antibiotics: Logic, Machinery, and Mechanisms, Chem. Rev., 2006, 106, 3468–3496 CrossRef CAS.
- J. I. Martinez Alvarado, A. B. Ertel, A. Stegner, E. E. Stache and A. G. Doyle, Direct Use of Carboxylic Acids in the Photocatalytic Hydroacylation of Styrenes To Generate Dialkyl Ketones, Org. Lett., 2019, 21, 9940–9944 CrossRef CAS PubMed.
- G.-N. Li, H.-C. Li, M.-R. Wang, Z. Lu and B. Yu, Visible-Light-Induced Deoxygenative Acylation Aromatization of p-Quinone Methides with Carboxylic Acids, Adv. Synth. Catal., 2022, 364, 3927–3931 CrossRef CAS.
- L. Zheng, P.-J. Xia, Q.-L. Zhao, Y.-E. Qian, W.-N. Jiang, H.-Y. Xiang and H. Yang, Photocatalytic Hydroacylation of Alkenes by Directly Using Acyl Oximes, J. Org. Chem., 2020, 85, 11989–11996 CrossRef CAS.
- R. Li, Y. Li, Y. Liu, Y. Wang, J. Wu and F. Wu, Metal-free phosphine-catalyzed visible-light-induced radical cyclization of alkenes: access to cyclic gem-difluoroacyl scaffolds, Org. Chem. Front., 2024, 11, 3717–3723 RSC.
- Y. Zhou, L. Zhao, M. Hu, X.-H. Duan and L. Liu, Visible-Light Photoredox-Catalyzed Divergent 1,2-Diacylation and Hydroacylation of Alkenes with Carboxylic Acid Anhydride, Org. Lett., 2023, 25, 5268–5272 CrossRef CAS.
- Y. Zhou, L. Zhao, M. Hu, X.-H. Duan and L. Liu, Visible-Light Photoredox-Catalyzed Divergent 1,2-Diacylation and Hydroacylation of Alkenes with Carboxylic Acid Anhydride, Org. Lett., 2023, 25, 5268–5272 CrossRef CAS PubMed.
- R. Suresh, I. Massad and I. Marek, Stereoselective tandem iridium-catalyzed alkene isomerization-cope rearrangement of ω-diene epoxides: efficient access to acyclic 1,6-dicarbonyl compounds, Chem. Sci., 2021, 12, 9328–9332 RSC.
- D. Zhang, H. Cai, Y. Chen, L. Yin, J. Zhong, Y. Zhang and Q.-F. Zhang, Ring Opening of Donor–Acceptor Cyclopropanes with Acyclic 1,3-Diketones for the Synthesis of 1,6-Dicarbonyl Compounds, J. Org. Chem., 2020, 85, 14262–14270 CrossRef CAS PubMed; L. Fu, D. M. Guptill and H. M. L. Davies, Rhodium(II)-Catalyzed C–H Functionalization of Electron-Deficient Methyl Groups, J. Am. Chem. Soc., 2016, 138, 5761–5764 CrossRef PubMed.
- L. Zhang, Y. Li, Z. Guo, Y. Li, N. Li, W. Li, C. Zhu and J. Xie, Photoredox deoxygenative allylation of carboxylic acids via selective 1,6-addition of acyl radicals to electron-deficient 1,3-dienes, Chin. J. Catal., 2023, 50, 215–221 CrossRef CAS.
- Y. Zhang, Y. Han, S. Zhu, F.-L. Qing, X.-S. Xue and L. Chu, Light-Induced Divergent Cyanation of Alkynes Enabled by Phosphorus Radicals, Angew. Chem., Int. Ed., 2022, 61, e202210838 CrossRef CAS.
- R. Szpera, D. F. J. Moseley, L. B. Smith, A. J. Sterling and V. Gouverneur, The Fluorination of C–H Bonds: Developments and Perspectives, Angew. Chem., Int. Ed., 2019, 58, 14824–14848 CrossRef CAS PubMed.
- E. P. Gillis, K. J. Eastman, M. D. Hill, D. J. Donnelly and N. A. Meanwell, Applications of Fluorine in Medicinal Chemistry, J. Med. Chem., 2015, 58, 8315–8359 CrossRef CAS.
- J. Wang, M. Sánchez-Roselló, J. L. Aceña, C. del Pozo, A. E. Sorochinsky, S. Fustero, V. A. Soloshonok and H. Liu, Fluorine in Pharmaceutical Industry: Fluorine-Containing Drugs Introduced to the Market in the Last Decade (2001–2011), Chem. Rev., 2014, 114, 2432–2506 CrossRef CAS.
- Q. Liu, C. Ni and J. Hu, China's flourishing synthetic organofluorine chemistry: innovations in the new millennium, Natl. Sci. Rev., 2017, 4, 303–325 CrossRef CAS.
- V. Gouverneur and K. Seppelt, Introduction: Fluorine Chemistry, Chem. Rev., 2015, 115, 563–565 CrossRef CAS PubMed.
- R. Berger, G. Resnati, P. Metrangolo, E. Weber and J. Hulliger, Organic fluorine compounds: a great opportunity for enhanced materials properties, Chem. Soc. Rev., 2011, 40, 3496–3508 RSC.
- S. Purser, P. R. Moore, S. Swallow and V. Gouverneur, Fluorine in medicinal chemistry, Chem. Soc. Rev., 2008, 37, 320–330 RSC.
- K. Müller, C. Faeh and F. Diederich, Fluorine in Pharmaceuticals: Looking Beyond Intuition, Science, 2007, 317, 1881–1886 CrossRef PubMed.
- X. Gao, X. Pan, P. Wang and Z. Jin, Visible light-induced phosphine-catalyzed perfluoroalkylation of indoles, Org. Chem. Front., 2022, 9, 5790–5797 RSC.
- H. Lu, D.-y. Wang and A. Zhang, Visible Light-Promoted Phosphine-Catalyzed Difluoroalkylation of Arenes and Heterocycles, J. Org. Chem., 2020, 85, 942–951 CrossRef CAS.
- X. Shu, Y.-Y. Jiang, L. Kang and L. Yang, Ni-Catalyzed hydrocyanation of alkenes with formamide as the cyano source, Green Chem., 2020, 22, 2734–2738 RSC.
- T. Zou, Y. He, R. Liu, Y. Zhang, S. Wei, J. Lu, J. Wang, L. Wang, Q. Fu and D. Yi, Photoredox-neutral ring-opening pyridylation of cyclic oximes via phosphoranyl radical-mediated NO/CC bond cleavages and sequential radical-radical coupling, Chin. Chem. Lett., 2023, 34, 107822 CrossRef CAS.
- R. Shimazumi, R. Tanimoto and M. Tobisu, Nickel/Photoredox Dual-Catalyzed Conversion of Allyl Esters to Ketones via the Formal Deletion of Oxygen, Org. Lett., 2023, 25, 6440–6445 CrossRef CAS.
- M.-J. Jiao, D. Liu, X.-Q. Hu and P.-F. Xu, Photocatalytic decarboxylative [2 + 2 + 1] annulation of 1,6-enynes with N-hydroxyphthalimide esters for the synthesis of indene-containing polycyclic compounds, Org. Chem. Front., 2019, 6, 3834–3838 RSC.
- N. A. Meanwell, Fluorine and Fluorinated Motifs in the Design and Application of Bioisosteres for Drug Design, J. Med. Chem., 2018, 61, 5822–5880 CrossRef CAS.
- Y. Ouyang, X.-H. Xu and F.-L. Qing, Hydrotrifluoromethylthiolation of Unactivated Alkenes and Alkynes with Trifluoromethanesulfonic Anhydride through Deoxygenative Reduction and Photoredox Radical Processes, Angew. Chem., Int. Ed., 2019, 58, 18508–18512 CrossRef CAS.
- J. Kim, M. Kim, J. Jeong and S. Hong, Unlocking the Potential of β-Fragmentation of Aminophosphoranyl Radicals for Sulfonyl Radical Reactions, J. Am. Chem. Soc., 2023, 145, 14510–14518 CrossRef CAS PubMed.
- J. J. Su, A. Chen, G. Zhang, Z. Jiang and J. Zhao, Photocatalytic Phosphine-Mediated Thioesterification of Carboxylic Acids with Disulfides, Org. Lett., 2023, 25, 8033–8037 CrossRef CAS PubMed.
- H. Yang, H. Li, G. Wei and Z. Jiang, Photoredox Catalytic Phosphite-Mediated Deoxygenation of α-Diketones Enables Wolff Rearrangement and Staudinger Synthesis of β-Lactams, Angew. Chem., Int. Ed., 2021, 60, 19696–19700 CrossRef CAS.
- L. Decuyper, M. Jukič, I. Sosič, A. Žula, M. D'Hooghe and S. Gobec, Antibacterial and β-Lactamase Inhibitory Activity of Monocyclic β-Lactams, Med. Res. Rev., 2018, 38, 426–503 CrossRef CAS PubMed.
- A. J. Chinn, K. Sedillo and A. G. Doyle, Phosphine/Photoredox Catalyzed Anti-Markovnikov Hydroamination of Olefins with Primary Sulfonamides via α-Scission from Phosphoranyl Radicals, J. Am. Chem. Soc., 2021, 143, 18331–18338 CrossRef CAS.
- Z.-P. Ye, Y.-Z. Hu, P.-J. Xia, H.-Y. Xiang, K. Chen and H. Yang, Photocatalytic intermolecular anti-Markovnikov hydroamination of unactivated alkenes with N-hydroxyphthalimide, Org. Chem. Front., 2021, 8, 273–277 RSC.
- W. Han, W.-D. Liu, J. Su and J. Zhao, Deoxygenation of oximes for the synthesis of pyrrolines via hydroimination cyclization, Org. Biomol. Chem., 2023, 21, 3350–3354 RSC.
- F. Zhu, Z. Qiao, N. He, C. Nong, Q. He, M. Xi, X. Song, J. Lin, J. Chen and Y. Jin, Photoredox catalyzed hydroazolylation of alkenes via phosphoranyl radicals, Org. Chem. Front., 2024, 11, 2839–2844 RSC.
- B. G. de la Torre and F. Albericio, The Pharmaceutical Industry in 2021. An Analysis of FDA Drug Approvals from the Perspective of Molecules, Molecules, 2022, 27, 1075 CrossRef CAS.
- M. Muttenthaler, G. F. King, D. J. Adams and P. F. Alewood, Trends in peptide drug discovery, Nat. Rev. Drug Discovery, 2021, 20, 309–325 CrossRef CAS.
- D. J. Drucker, Advances in oral peptide therapeutics, Nat. Rev. Drug Discovery, 2020, 19, 277–289 CrossRef CAS PubMed.
- C. Morrison, Constrained peptides’ time to shine?, Nat. Rev. Drug Discovery, 2018, 17, 531–533 CrossRef CAS.
- A. Henninot, J. C. Collins and J. M. Nuss, The Current State of Peptide Drug Discovery: Back to the Future?, J. Med. Chem., 2018, 61, 1382–1414 CrossRef CAS PubMed.
- D. J. Craik, D. P. Fairlie, S. Liras and D. Price, The Future of Peptide-based Drugs, Chem. Biol. Drug Des., 2013, 81, 136–147 CrossRef CAS PubMed.
- J. Su, J.-N. Mo, X. Chen, A. Umanzor, Z. Zhang, K. N. Houk and J. Zhao, Generation of Oxyphosphonium Ions by Photoredox/Cobaloxime Catalysis for Scalable Amide and Peptide Synthesis in Batch and Continuous-Flow, Angew. Chem., Int. Ed., 2022, 61, e202112668 CrossRef CAS PubMed.
- J. Su, J.-N. Mo, G. Zhang, Z. Jiang and J. Zhao, A practical approach for oligopeptide synthesis via synergistic photoredox, cobaloxime and organophosphorus triple catalysis, Org. Chem. Front., 2023, 10, 4895–4904 RSC.
- J. Yang, C. Wang, B. Huang, H. Zhou, J. Li and X. Liu, Photoredox Catalytic Phosphine-Mediated Deoxygenative Hydroacylation of Azobenzenes with Carboxylic Acids, Org. Lett., 2024, 26, 498–502 CrossRef CAS.
- M.-C. Fu, J.-X. Wang and R. Shang, Triphenylphosphine-Catalyzed Alkylative Iododecarboxylation with Lithium Iodide under Visible Light, Org. Lett., 2020, 22, 8572–8577 CrossRef CAS.
- J. Zhang, C. Mück-Lichtenfeld and A. Studer, Photocatalytic phosphine-mediated water activation for radical hydrogenation, Nature, 2023, 619, 506–513 CrossRef CAS PubMed.
|
This journal is © the Partner Organisations 2025 |
Click here to see how this site uses Cookies. View our privacy policy here.