DOI:
10.1039/D1PY00271F
(Communication)
Polym. Chem., 2021,
12, 2695-2700
Inter-capsule fusion and capsule shell destruction using dynamic covalent polymers†
Received
1st March 2021
, Accepted 26th April 2021
First published on 26th April 2021
Abstract
Herein, capsule shells containing hindered urea bonds were prepared using interfacial polymerization in an oil-in-oil Pickering emulsion stabilized by functionalized graphene oxide nanosheets. After isolation and mild heating, the polymer shells can be fused into monoliths or destroyed. These dynamic polymer shells provide a route to control the morphology of composite materials with application in energy storage, separations, additive manufacturing, controlled release, and so on.
Introduction
Capsules are structures composed of a shell of one material with or without a different material inside-filled or hollow capsules, respectively.1–3 These structures have been used to improve handling of viscous liquids,4–6 can serve as microreactors,5,7,8 and can enhance surface area of active material.9 Therefore, capsules find application in energy storage,9,10 separations,11–15 catalysis,5,6 and controlled release,8,16,17 depending on the capsule composition. The most common techniques for capsule preparation are microfluidics, the hard template method (growth of shell on a solid particle followed by dissolution of the core), and interfacial reactions using an emulsion as template (i.e., soft template method). Of these, the most widely used approach is the soft template method. For example, Savin and coworkers successfully prepared hollow capsules with amphiphilic shells of cross-linked polycaprolactone-b-poly(ethylene glycol) which showed good hydrocarbon uptake efficiency.18 Likewise, our group has prepared capsules with a core of ionic liquid and composite shell of graphene oxide and polyurea for use in energy storage,10 oil remediation,19 and CO2 uptake.11,14
The chemical composition of capsule shells is critical to application-oriented properties such as mechanical strength,20–22 porosity,21–24 chemical stability and reactivity,25,26 and electrical and thermal characteristics,10,23,26,27 as well as shape.22,28 For example, do Nascimento and colleagues prepared polydimethylsiloxane shells where the elasticity was tuned by adjusting the amount of crosslinker.20 Likewise, Pisani et al. fabricated shells of poly(lactide-co-glycolide) (PLGA) and poly(lactide-co-glycolide)-polyethylene glycol (PLGA-PEG)28 where the surface morphology was controlled by the ratio of the polymers. Alternatively, Zhang et al. used imine condensation to prepare capsule shells of covalent organic frameworks (COFs), then attached gold particles which had pendant amines to the shell.29 In a similar vein, Hatai et al. prepared polymer shells containing hydrazone units and coumarin dimers which released the contents of the capsule core in response to changes in pH, light irradiation, or the presence of metal ions.30 Thus, integration of chemical functionalities that can undergo reactions under relatively mild conditions can be used to tailor properties.
Dynamic covalent bonds (DCBs) are those which can reversibly break and form under mild conditions or external stimuli.31,32 Polymers containing these bonds have promising application in self-healing,33–35 shape-memory,34,36,37 and sensors.38 Examples of DCBs include transesterification,39 amide/imine/aldol chemistry,39 alkene- or alkyne-metathesis,39–41 boronic acid condensation,42 Diels–Alder reactions,39,43 and so on. Hindered urea bonds (HUBs) are DCBs formed by the reaction of an isocyanate with a secondary amine, the latter of which bears a bulky alkyl group, such as isopropyl or tert-butyl. The sterically demanding nitrogen substituent leads to non-planarity of the urea bond and enables the reverse reaction (urea → amine + isocyanate) to occur under mild thermal conditions; this is in contrast to urea bonds formed from primary amines and isocyanates which are generally considered irreversible (Fig. 1 and Fig. S1†).44,45 Cheng and coworkers demonstrated that polymer films bearing HUBs, those formed by reaction of multifunctional secondary amines and multifunctional isocyanates, have self-healing,44 shape retentive,37 and recyclable properties.46
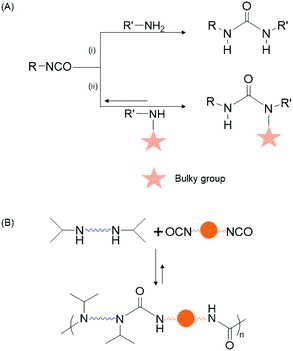 |
| Fig. 1 (A) Illustration of (i) irreversible urea bond formed by reaction of an isocyanate and primary amine, and (ii) reversible hindered urea bond (HUB) formed by reaction of an isocyanate and secondary amine bearing a bulky substituent; and (B) hindered polyurea chemistry. | |
Herein, we report polymer capsules with shells that incorporate HUBs and demonstrate that the presence of these bonds can be used to either fuse capsule shells into monoliths by subjecting isolated, dried capsules to mild heating, or to destroy capsule shells by exposing them to primary alkyl amine (Scheme 1). The shells containing hindered poly(urea-urethanes) are prepared by interfacial polymerization in an oil-in-oil Pickering emulsion stabilized by alkylated graphene oxide (GO) nanosheets. Optical microscopy imaging is used to follow the changes in capsule morphology, whereas Fourier transform infrared (FTIR) spectroscopy and thermogravimetric analysis (TGA) are used to characterize the composition of the shells. The tailored capsule chemistry allows for a monolith to be prepared, in any shape and at any time, supporting liquid transport for energy storage, molecule separation, and additive manufacturing, whereas capsule destruction on demand may find use in self-healing and controlled release applications.
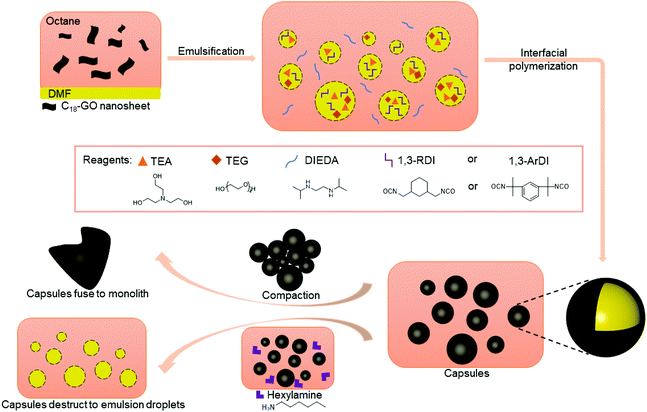 |
| Scheme 1 Overview of the work reported here: formation of capsules with shells containing hindered urea bonds (HUBs) and the fusion or destruction of the capsule shells. Capsules were prepared by interfacial polymerization in oil-in-oil Pickering emulsions stabilized by functionalized graphene oxide nanosheets. | |
Results and discussion
Oil-in-oil emulsions were selected as the template to prepare capsules with composite shells containing HUBs because these water-free emulsions enable control of the capsule composition; specifically, water hydrolyzes isocyanates to give primary amines and expel CO2, then this primary amine can react irreversibly with other isocyanate functionalities. We leveraged alkylated graphene oxide (GO) nanosheets as particle surfactant to stabilize DMF-in-octane emulsions. These particle surfactants were prepared as previously reported,19,47 by the modification of GO with 1-octadecyl amine, giving C18-GO, as verified by FTIR spectroscopy (Fig. S2†) and changes in dispersibility. With C18-GO as a particle surfactant dispersed in octane, a DMF-in-octane emulsion was prepared using a hand-held emulsifier (Fig. S3A†); even without interfacial polymerization, these emulsions were stable for at least ten days.
To prepare capsules with shells containing HUBs, a diisocyanate, triethanolamine, and tetra(ethylene glycol) were dissolved in DMF before emulsification, then an octane solution of N,N-diisopropyl ethylenediamine (DIEDA) and dibutyltin diacetate was added to the continuous phase. Triethanolamine and tetra(ethylene glycol) were used as polymer chain extenders, consistent with the work of Cheng.44 Two different diisocyanates were used, the primary isocyanate 1,3-bis(isocyanatomethyl)cyclohexane (1,3-RDI) and tertiary isocyanates 1,3-bis(2-isocyanato-2-propyl)benzene (1,3-ArDI), as they may have different rates of reactions with secondary amines. After emulsification and addition of all reagents, the emulsions were left unagitated for 5 days, then propylamine was added to quench any unreacted isocyanates and prevent undesired inter-capsule fusion. Dry powders of the capsules (Fig. S4†) were isolated by gravity filtration, dried under reduced pressure, and could be dispersed in dodecane. Of note, during preparation and isolation, the samples were kept at 5 °C for consistency across studies.
The optical microscopy images in Fig. 2A and 3A show that nearly-spherical, individual capsules of 1,3-RDI-DIEDA and 1,3-ArDI-DIEDA were obtained. Upon isolation, some of the inner oil phase may be removed, and thus the wrinkled morphology can be attributed to changes in the internal volume. For 1,3-RDI-DIEDA, most of the capsules range from 25 μm to 45 μm, with some smaller capsules between 5 μm and 10 μm also present. Most of the 1,3-ArDI-DIEDA capsules are slightly smaller and approximately 20–30 μm with a few smaller ones are also around 10 μm. This slight difference of capsule size range may be attributed to the use of different diisocyanate monomers, which can lead to different rates of crosslinking and shell thickness (our group is investigating this phenomenon further). The FTIR spectra of the monomers and capsule shells are shown in Fig. 4, demonstrating the successful formation of polyurea. For both capsule shells, there is no peak corresponding to the N
C
O of the isocyanate (∼2241 cm−1), and the stretching and bending frequencies at 3314 cm−1 and 1534 cm−1 support presence of N–H, whereas the stretching frequency at 1700 cm−1 supports formation of the urea carbonyl. Characterization of the capsules by thermogravimetric analysis (TGA) is shown by the weight loss profiles in Fig. 5. For both capsules, the majority of weight loss occurred between 210 °C and 400 °C, which can be attributed to the polymer; the minor weight loss below 100 °C is consistent with the bulk polymer48 and may be due to solvent evaporation or reaction of adsorbed water with thermally liberated isocyanates, which would cause loss of gaseous CO2.
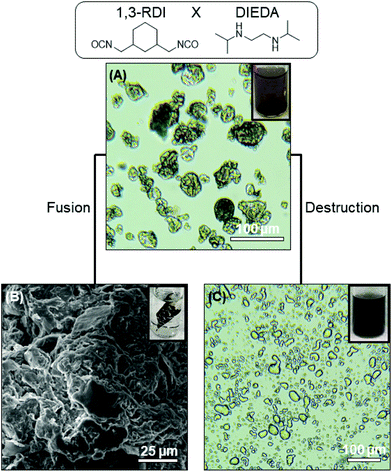 |
| Fig. 2 1,3-RDI-DIEDA capsules: (A) optical microscopy image of capsules dispersed in dodecane; (B) SEM image of monolith after capsule fusion; (C) optical microscopy image of droplets after capsule destruction by hexylamine. Inset images are photographs of the samples. | |
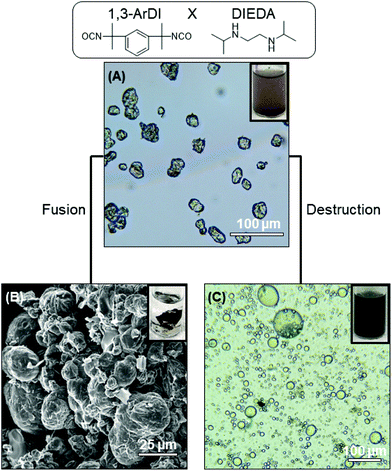 |
| Fig. 3 1,3-ArDI-DIEDA capsules: (A) optical microscopy image of capsules dispersed in dodecane; (B) SEM image of monolith after capsule fusion; (C) optical microscopy image of droplets after capsule destruction by hexylamine. Inset images are photographs of the samples. | |
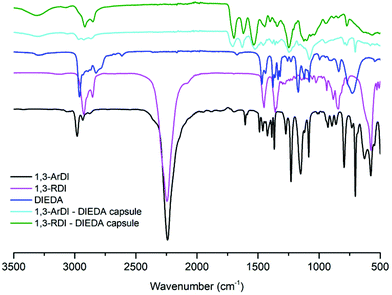 |
| Fig. 4 Offset FTIR spectra (% transmission) of the diisocyanates 1,3-ArDI (black line) and 1,3-RDI (purple line); diamine DIEDA (dark blue line); and capsules of 1,3-ArDI-DIEDA (light blue line) and capsules of 1,3-RDI-DIEDA (olive green line). | |
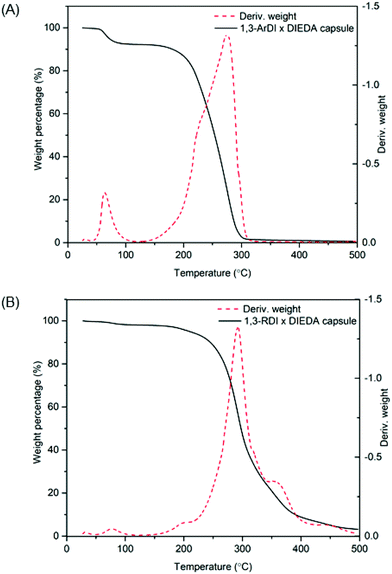 |
| Fig. 5 TGA weight loss profiles and their first derivatives of (A) 1,3-ArDI-DIEDA capsules and (B)1,3-RDI-DIEDA capsules. | |
The ability of the DCBs in the polymer shell to either fuse the capsule shells into monoliths or destroy the polymer component of the shell was then evaluated. Fusion of capsule shells requires them to be in close contact with each other, which was achieved by compacting the capsules during the isolation process. The compacted capsules were placed in a vial that was heated at ∼50 °C overnight, resulting in formation of a monolith for both capsule systems, as shown in Fig. 2B and 3B. This capsule shell fusion is attributed to bond exchange between the polymers of the neighboring capsules based on the reversible nature of the HUBs. The inset pictures of Fig. 2B and 3B further illustrate the formation of a monolith. Subjecting capsules that do not contain HUBs (i.e., were prepared using a primary diamine) to the same conditions did not lead to monolith formation, but the capsules remained dispersible in dodecane (Fig. S5 and S6†).
The polymer component of the shell can alternatively be destroyed in the presence of a primary amine at an appropriate temperature. Reversion of the HUB gives a secondary amine and an isocyanate, the latter of which undergoes irreversible reaction with the primary amine. To destroy the polymer component of the capsule shells, isolated capsules were dispersed in octane, then hexylamine was added and the mixture heated to 50 °C. The optical microscopy images in Fig. 2C and 3C show that the capsule shells were destroyed, as indicated in the change in contrast, with the system reverting to the emulsion. From these microscopy images, the stabilizing agent of the emulsion is unclear, and may be attributed to small molecules or oligomers produced or C18-GO nanosheets. The majority of the newly-formed emulsion droplets are smaller (<25 μm) than that of corresponding capsules, and are more similar to droplets in the DMF-in-octane emulsion templates (Fig. S3†). This supports that interfacial polymerization can lead to differences between the size of droplets and capsules. Additionally, some non-spherical droplets are observed in the 1,3-RDI-DIEDA sample after capsule destruction, which may be due to the uneven distribution of various surfactants at the fluid–fluid interface.
Summary
In this communication, we report the preparation of capsule shells containing hindered urea bonds (HUBs) and use them to form monoliths by inter-shell fusion, or destroy them by addition of a primary amine. The capsules are prepared using a water-free oil-in-oil emulsion stabilized by C18-GO. We demonstrate that diisopropyl ethylenediamine (DIEDA) can be used with two different diisocyanates to form these dynamic shells. Importantly, at and below room temperature, the dynamicity of the bonds within the shell is slow enough such that individual capsules remain intact, but a slight increase in temperature to ∼50 °C enables capsule fusion or destruction, depending on the conditions used. This work provides the ability to form monoliths from dry capsule powder or destroy the shell on demand, and is expected to find use across a broad spectrum of applications. Ongoing work in our lab focuses on further tuning the polymer chemistry of the capsule shell to control the temperature at which fusion and destruction occurs, as well as incorporating other core materials.
Author contributions
Y. W. and E. P. designed the experiments. Y. W. carried out the experiments with the assistance of K. Q. and performed all characterization. The manuscript was written through contributions of all authors. All authors have given approval to the final version of the manuscript.
Conflicts of interest
The authors declare no competing financial interest.
Acknowledgements
The authors thank NSF CAREER Award #1955170 and Texas A&M University for financial support. The authors acknowledge the use of the Soft Matter Facility and Microscopy and Imaging Center at Texas A&M.
References
- Z. Fang and B. Bhandari, Encapsulation of polyphenols – a review, Trends Food Sci. Technol., 2010, 21(10), 510–523 CrossRef CAS.
- M. G. Bah, H. M. Bilal and J. Wang, Fabrication and application of complex microcapsules: a review, Soft Matter, 2020, 16(3), 570–590 RSC.
- K. C. Bentz and D. A. Savin, Hollow polymer nanocapsules: synthesis, properties, and applications, Polym. Chem., 2018, 9(16), 2059–2081 RSC.
- R. Santiago, J. Lemus, C. Moya, D. Moreno, N. Alonso-Morales and J. Palomar, Encapsulated Ionic Liquids to Enable the Practical Application of Amino Acid-Based Ionic Liquids in CO2 Capture, ACS Sustainable Chem. Eng., 2018, 6(11), 14178–14187 CrossRef CAS.
- E. Weiss and R. Abu-Reziq, Ionic liquid-based polymeric microreactors and their applicability, J. Mater. Sci., 2017, 52(17), 10637–10647 CrossRef CAS.
- E. Weiss, B. Dutta, A. Kirschning and R. Abu-Reziq, BMIm-PF6@SiO2 Microcapsules: Particulated Ionic Liquid as A New Material for the Heterogenization of Catalysts, Chem. Mater., 2014, 26(16), 4781–4787 CrossRef CAS.
- J. Gaitzsch, X. Huang and B. Voit, Engineering Functional Polymer Capsules toward Smart Nanoreactors, Chem. Rev., 2016, 116(3), 1053–1093 CrossRef CAS PubMed.
- J. Szafraniec-Szczesny, M. Janik-Hazuka, J. Odrobinska and S. Zapotoczny, Polymer Capsules with Hydrophobic Liquid Cores as Functional Nanocarriers, Polymers, 2020, 12(9), 1999 CrossRef CAS PubMed.
- E. M. Shchukina, M. Graham, Z. Zheng and D. G. Shchukin, Nanoencapsulation of phase change materials for advanced thermal energy storage systems, Chem. Soc. Rev., 2018, 47(11), 4156–4175 RSC.
- Q. Luo, P. Wei, Q. Huang, B. Gurkan and E. B. Pentzer, Carbon Capsules of Ionic Liquid for Enhanced Performance of Electrochemical Double-Layer Capacitors, ACS Appl. Mater. Interfaces, 2018, 10(19), 16707–16714 CrossRef CAS PubMed.
- Y. Y. Lee, K. Edgehouse, A. Klemm, H. Mao, E. Pentzer and B. Gurkan, Capsules of Reactive Ionic Liquids for Selective Capture of Carbon Dioxide at Low Concentrations, ACS Appl. Mater. Interfaces, 2020, 12(16), 19184–19193 CrossRef CAS PubMed.
- J. Lemus, F. A. Da Silva F., J. Palomar, P. J. Carvalho and J. A. P. Coutinho, Solubility of carbon dioxide in encapsulated ionic liquids, Sep. Purif. Technol., 2018, 196, 41–46 CrossRef CAS.
- V. A. Poteryaeva, M. A. Bubenchikov, A. M. Bubenchikov and A. V. Lun-Fu, The interaction of atoms and molecules with nanocapsules and hollow nanowires, Sci. Rep., 2020, 10(1), 15631 CrossRef CAS PubMed.
- Q. Huang, Q. Luo, Y. Wang, E. Pentzer and B. Gurkan, Hybrid Ionic Liquid Capsules for Rapid CO2 Capture, Ind. Eng. Chem. Res., 2019, 58(24), 10503–10509 CrossRef CAS PubMed.
- W. Yu, T. Wang, A.-H. A. Park and M. Fang, Toward Sustainable Energy and Materials: CO2 Capture Using Microencapsulated Sorbents, Ind. Eng. Chem. Res., 2020, 59(21), 9746–9759 CrossRef CAS.
- J. P. Goertz, K. C. DeMella, B. R. Thompson, I. M. White and S. R. Raghavan, Responsive capsules that enable hermetic encapsulation of contents and their thermally triggered burst-release, Mater. Horiz., 2019, 6(6), 1238–1243 RSC.
- B. Andrade, Z. Song, J. Li, S. C. Zimmerman, J. Cheng, J. S. Moore, K. Harris and J. S. Katz, New frontiers for encapsulation in the chemical industry, ACS Appl. Mater. Interfaces, 2015, 7(12), 6359–6368 CrossRef CAS PubMed.
- K. C. Bentz, M. Ejaz, S. Arencibia, N. Sultan, S. M. Grayson and D. A. Savin, Hollow amphiphilic crosslinked nanocapsules from sacrificial silica nanoparticle templates and their application as dispersants for oil spill remediation, Polym. Chem., 2017, 8(34), 5129–5138 RSC.
- Q. Luo, Y. Wang, Z. Chen, P. Wei, E. Yoo and E. Pentzer, Pickering Emulsion-Templated Encapsulation of Ionic Liquids for Contaminant Removal, ACS Appl. Mater. Interfaces, 2019, 11(9), 9612–9620 CrossRef CAS PubMed.
- D. F. do Nascimento, J. A. Avendano, A. Mehl, M. J. B. Moura, M. S. Carvalho and W. J. Duncanson, Flow of Tunable Elastic Microcapsules through Constrictions, Sci. Rep., 2017, 7(1), 11898 CrossRef PubMed.
- Z. Wu, J. G. Werner and D. A. Weitz, Microfluidic Fabrication of Phase-Inverted Microcapsules with Asymmetric Shell Membranes with Graded Porosity, ACS Macro Lett., 2020, 10(1), 116–121 CrossRef.
- O. Shchepelina, M. O. Lisunova, I. Drachuk and V. V. Tsukruk, Morphology and Properties of Microcapsules with Different Core Releases, Chem. Mater., 2012, 24(7), 1245–1254 CrossRef CAS.
- G. A. Ferrero, A. B. Fuertes and M. Sevilla, N-doped porous carbon capsules with tunable porosity for high-performance supercapacitors, J. Mater. Chem. A, 2015, 3(6), 2914–2923 RSC.
- X. P. Zhang, J. Luo, D. X. Zhang, T. F. Jing, B. X. Li and F. Liu, Porous microcapsules with tunable pore sizes provide easily controllable release and bioactivity, J. Colloid Interface Sci., 2018, 517, 86–92 CrossRef CAS PubMed.
- A. Borbora and U. Manna, Impact of chemistry on the preparation and post-modification of multilayered hollow microcapsules, Chem. Commun., 2021, 57, 2110–2123 RSC.
- M. Graham, J. Smith, M. Bilton, E. Shchukina, A. A. Novikov, V. Vinokurov and D. G. Shchukin, Highly Stable Energy Capsules with Nano-SiO2 Pickering Shell for Thermal Energy Storage and Release, ACS Nano, 2020, 14(7), 8894–8901 CrossRef CAS PubMed.
- T. Do, Y. G. Ko, Y. Jung and U. S. Choi, Highly Durable and Thermally Conductive Shell-Coated Phase-Change Capsule as a Thermal Energy Battery, ACS Appl. Mater. Interfaces, 2020, 12(5), 5759–5766 CrossRef CAS PubMed.
- E. Pisani, C. Ringard, V. Nicolas, E. Raphaël, V. Rosilio, L. Moine, E. Fattal and N. Tsapis, Tuning microcapsules surface morphology using blends of homo- and copolymers of PLGA and PLGA-PEG, Soft Matter, 2009, 5(16), 3054–3060 RSC.
- F. Zhang, Z. Yang, J. Hao, K. Zhao, M. Hua, Y. Yang and J. Wei, Dynamic covalent chemistry steers synchronizing nanoparticle self-assembly with interfacial polymerization, Commun. Chem., 2019, 2(1), 123 CrossRef.
- J. Hatai, C. Hirschhauser, J. Niemeyer and C. Schmuck, Multi-Stimuli-Responsive Supramolecular Polymers Based on Noncovalent and Dynamic Covalent Bonds, ACS Appl. Mater. Interfaces, 2020, 12(2), 2107–2115 CrossRef CAS PubMed.
- P. Chakma and D. Konkolewicz, Dynamic Covalent Bonds in Polymeric Materials, Angew. Chem., Int. Ed., 2019, 58(29), 9682–9695 CrossRef CAS PubMed.
- S. Huang, X. Kong, Y. Xiong, X. Zhang, H. Chen, W. Jiang, Y. Niu, W. Xu and C. Ren, An overview of dynamic covalent bonds in polymer material and their applications, Eur. Polym. J., 2020, 141, 110094 CrossRef CAS.
- Z. Wang, X. Lu, S. Sun, C. Yu and H. Xia, Preparation, characterization and properties of intrinsic self-healing elastomers, J. Mater. Chem. B, 2019, 7(32), 4876–4926 RSC.
- M. M. Perera and N. Ayres, Dynamic covalent bonds in self-healing, shape memory, and controllable stiffness hydrogels, Polym. Chem., 2020, 11(8), 1410–1423 RSC.
- Q. An, I. D. Wessely, Y. Matt, Z. Hassan, S. Bräse and M. Tsotsalas, Recycling and self-healing of dynamic covalent polymer networks with a precisely tuneable crosslinking degree, Polym. Chem., 2019, 10(6), 672–678 RSC.
- Y. Wang, Y. Pan, Z. Zheng and X. Ding, Reconfigurable and Reprocessable Thermoset Shape Memory Polymer with Synergetic Triple Dynamic Covalent Bonds, Macromol. Rapid Commun., 2018, 39(10), 1800128 CrossRef PubMed.
- Y. Jia, H. Ying, Y. Zhang, H. He and J. Cheng, Reconfigurable Poly(urea–urethane) Thermoset Based on Hindered Urea Bonds with Triple–Shape–Memory Performance, Macromol. Chem. Phys., 2019, 220(12), 1900148 CrossRef.
- S. D. Bull, M. G. Davidson, J. M. Van den Elsen, J. S. Fossey, A. T. A. Jenkins, Y.-B. Jiang, Y. Kubo, F. Marken, K. Sakurai and J. Zhao, Exploiting the reversible covalent bonding of boronic acids: recognition, sensing, and assembly, Acc. Chem. Res., 2013, 46(2), 312–326 CrossRef CAS PubMed.
- W. Zou, J. Dong, Y. Luo, Q. Zhao and T. Xie, Dynamic Covalent Polymer Networks: from Old Chemistry to Modern Day Innovations, Adv. Mater., 2017, 29(14), 1606100 CrossRef PubMed.
- Y. X. Lu and Z. Guan, Olefin metathesis for effective polymer healing via dynamic exchange of strong carbon-carbon double bonds, J. Am. Chem. Soc., 2012, 134(34), 14226–14231 CrossRef CAS PubMed.
- Q. Wang, C. Yu, C. Zhang, H. Long, S. Azarnoush, Y. Jin and W. Zhang, Dynamic covalent synthesis of aryleneethynylene cages through alkyne metathesis: dimer, tetramer, or interlocked complex?, Chem. Sci., 2016, 7(5), 3370–3376 RSC.
- B. Marco-Dufort and M. W. Tibbitt, Design of moldable hydrogels for biomedical applications using dynamic covalent boronic esters, Mater. Today Chem., 2019, 12, 16–33 CrossRef CAS.
- A. P. Bapat, J. G. Ray, D. A. Savin, E. A. Hoff, D. L. Patton and B. S. Sumerlin, Dynamic-covalent nanostructures prepared by Diels–Alder reactions of styrene-maleic anhydride-derived copolymers obtained by one-step cascade block copolymerization, Polym. Chem., 2012, 3(11), 3112–3120 RSC.
- H. Ying, Y. Zhang and J. Cheng, Dynamic urea bond for the design of reversible and self-healing polymers, Nat. Commun., 2014, 5, 3218 CrossRef PubMed.
- H. Ying and J. Cheng, Hydrolyzable polyureas bearing hindered urea bonds, J. Am. Chem. Soc., 2014, 136(49), 16974–16977 CrossRef CAS PubMed.
- Y. Zhang, H. Ying, K. R. Hart, Y. Wu, A. J. Hsu, A. M. Coppola, T. A. Kim, K. Yang, N. R. Sottos, S. R. White and J. Cheng, Malleable and Recyclable Poly(urea-urethane) Thermosets bearing Hindered Urea Bonds, Adv. Mater., 2016, 28(35), 7646–7651 CrossRef CAS PubMed.
- Q. Luo, Y. Wang, E. Yoo, P. Wei and E. Pentzer, Ionic Liquid-Containing Pickering Emulsions Stabilized by Graphene Oxide-Based Surfactants, Langmuir, 2018, 34(34), 10114–10122 CrossRef CAS PubMed.
-
H. Ying, Hindered urea bond for the design of dynamic materials, University of Illinois at Urbana-Champaign, 2017 Search PubMed.
Footnote |
† Electronic supplementary information (ESI) available. See DOI: 10.1039/d1py00271f |
|
This journal is © The Royal Society of Chemistry 2021 |
Click here to see how this site uses Cookies. View our privacy policy here.