DOI:
10.1039/D0SC05340F
(Edge Article)
Chem. Sci., 2021,
12, 2108-2113
Fast oxygen ion migration in Cu–In–oxide bulk and its utilization for effective CO2 conversion at lower temperature†
Received
26th September 2020
, Accepted 10th December 2020
First published on 23rd December 2020
Abstract
Efficient activation of CO2 at low temperature was achieved by reverse water–gas shift via chemical looping (RWGS-CL) by virtue of fast oxygen ion migration in a Cu–In structured oxide, even at lower temperatures. Results show that a novel Cu–In2O3 structured oxide can show a remarkably higher CO2 splitting rate than ever reported. Various analyses revealed that RWGS-CL on Cu–In2O3 is derived from redox between Cu–In2O3 and Cu–In alloy. Key factors for high CO2 splitting rate were fast migration of oxide ions in the alloy and the preferential oxidation of the interface of alloy–In2O3 in the bulk of the particles. The findings reported herein can open up new avenues to achieve effective CO2 conversion at lower temperatures.
Introduction
Anthropogenic emissions of greenhouse gases are regarded as a cause of global warming, which is expected to lead to severe future climate change. Particularly, carbon dioxide (CO2) derived from fossil fuels and industrial processes has long presented strong effects that continue to raise global mean temperatures.1,2 Therefore, development of CO2 capture and utilization (CCU) technologies2–9 represents an urgent task for reducing CO2 emissions into the atmosphere and for establishing a sustainable carbon cycle. Synthesis of fuels using electricity generation from renewable resources and using CO2, most notably sun-to-fuel (STF)5 and power-to-liquid6 processes, has been proposed as a means of developing CCU technologies. In fact, CO2-based fuels are anticipated as a technology that can increasingly incorporate renewable energy into the mobility sector as an “e-fuel”.8,9 For these fuel production technologies, the potential process route is a Fischer–Tropsch (FT) process using syngas, which includes hydrogen and carbon monoxide (CO) converted from captured CO2.2–4 As a method of efficient conversion of CO2 to CO through this process, reverse water–gas shift (RWGS) is a promising reaction that uses renewable H2.10,11 Mallapragada et al. proposed that the STF route consisting of RWGS followed by the FT reaction has higher efficiency than other routes with direct CO2 conversion by photosynthetic bacteria and biomass conversion.5 The RWGS is an equilibrium-limited reaction (eqn (1)) with an endothermic nature. | CO2 + H2 ↔ CO + H2O, ΔH0298 = 42.1 kJ mol−1 | (1) |
This reaction requires high reaction temperatures and separation of gas products to gain high conversion and a suitable H2/CO ratio in syngas. Furthermore, conventional catalytic RWGS processes include side reactions, as presented in eqn (2) and (3).
| CO + 3H2 ↔ CH4 + H2O, ΔH0298 = −206.5 kJ mol−1 | (2) |
| CO2 + 4H2 ↔ CH4 + 2H2O, ΔH0298 = −165.0 kJ mol−1 | (3) |
The CH4 produced via these side reactions makes gas separation more complex. Additionally, it loses energy because of the exothermic reaction. S.-C. Yang et al. reported a Cu/CeOx catalyst12 which demonstrates 100% CO selectivity at 573 K. However, at low temperatures, CO2 conversion is low because RWGS is an equilibrium-limited endothermic reaction, which results in a much higher H2/CO ratio of the obtained syngas than the suitable ratio (H2/CO = 2) for fuel synthesis by the FT process. One solution for these shortcomings is using chemical-looping reverse water–gas shift (RWGS-CL).13,14 The overall concept of the solar-driven fuel (chemical) synthesis process with RWGS-CL is presented in Fig. 1. Through this process, CO2 is converted to CO in two separate steps: reduction and re-oxidation of a metal oxide as an oxygen storage material (OSM). First, the OSM is reduced by H2 (reaction (4)). Subsequently, the reduced OSM is re-oxidized by CO2 to generate pure CO (reaction (5)).
| MOx + δH2 → MOx−δ + δH2O | (4) |
| MOx−δ + δCO2 → MOx + δCO | (5) |
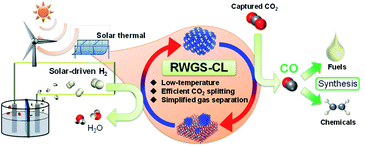 |
| Fig. 1 Concept of solar-driven fuels and chemical synthesis processes with RWGS-CL. | |
Actually, RWGS-CL has benefits deriving from the absence of undesirable side reactions, which drives the equilibrium to CO production, and simplified gas separation, which leads to its improved energy efficiency. In fact, Wenzel et al. investigated solar-to-syngas efficiency for RWGS-CL processes and reported that the energy demand for separation is reduced by 77% compared to conventional RWGS processes.13 In the RWGS-CL process, the development of the OSM is an indispensable factor to make this process feasible. The rates of reduction and oxidation, CO yield, and stability are emphasized as key factors affecting the OSM for the RWGS-CL cycles. Furthermore, the operation temperature is an important factor. Isothermal operation at lower temperatures is desired to reduce heat loss and to increase its feasibility.14 In recent years, great effort has been put forth for the development of OSMs with high redox properties for RWGS-CL.14–19 In previous studies, perovskite-type oxides14,15 and iron-based oxides16–18 have been well reported. Perovskite-type oxides are some of the promising OSMs thanks to their high stability and redox properties.20 Maiti et al. investigated a series of lanthanum-based perovskites and found that LaCa0.4Fe0.4Mn0.6O3 showed high CO2 splitting rate at low temperatures (723–823 K).15 Iron-based oxides are other candidates of OSMs for RWGS-CL because of their high oxygen capacity. Cu, Co, and Mn co-doped ferrites16,17 and Fe2O3 supported on ion-conductive oxides18 were developed, which showed high performance and stability for the RWGS-CL cycle at 923 K. However, in these OSMs, isothermal operations have been investigated at temperatures above 823 K, and the performance below 773 K is unclear. Although Utsis et al. reported isothermal RWGS-CL at 623–723 K using Fe-substituted Ba-hexa-aluminates,19 the CO2 splitting capacity was 0.4 mmol g−1, so higher performance of CO2 splitting is desired. This study has revealed that an indium-based oxide can be a novel OSM material able to exhibit extremely high isothermal RWGS-CL performance at low temperatures (673–773 K). Particularly, the performance of Cu-modified In2O3 formed from Cu2In2O5 as a parent material was found to be promising for this purpose. Characterization of OSMs was conducted using powder X-ray diffraction (XRD), a field emission transmission electron microscope equipped with an energy-dispersive X-ray spectrometer (STEM-EDX), X-ray photoelectron spectroscopy (XPS), and in situ X-ray absorption fine structure (XAFS) measurements.
Results and discussion
Isothermal RWGS-CL performance
After applying various oxides for RWGS-CL, we found that the Cu–In mixed oxide has high potential for RWGS-CL. The CO2 splitting performance obtained in this study is presented in Fig. 2 and Table S1† along with results described in earlier reports of the literature. Compared to other perovskite-type oxides14,15 and ferrites,16,17 which were reported earlier, Cu–In2O3 showed higher CO2 splitting rates and sufficient amounts of CO2 splitting even at low temperatures such as 673–773 K. Among all the oxides, Cu–In2O3 derived from Cu2In2O5 is the most promising material for low-temperature isothermal RWGS-CL. The cycling performance of isothermal RWGS-CL on Cu2In2O5 at 773 K is presented in ESI Fig. S1.† The reduction and oxidation (redox) amounts are defined as the moles of oxygen released or restored per gram of Cu2In2O5. In the first cycle, Cu2In2O5 showed reduction of 9.50 mmol g−1 and re-oxidation of 4.82 mmol g−1. Then, in cycles 2–5, the reduction and the oxidation amounts were, respectively, 5.06 ± 0.41 mmol g−1 and 4.80 ± 0.40 mmol g−1. Excess reduction in the first cycle (4.68 mmol g−1) was almost equivalent to the release of 2 mol oxygen atoms per mol of Cu2In2O5. This finding suggests that Cu2In2O5 has become Cu(0)–In2O3, which is confirmed later. Products of RWGS-CL were measured using a quadrupole mass spectrometer. The mass spectra of products are presented in ESI Fig. S2.† The products of reduction and oxidation steps were identified, respectively, as H2O (m/z = 18) and CO (m/z = 28) without production of other by-products. Results confirmed that RWGS-CL on Cu–In2O3 formed from Cu2In2O5 is a selective CO2 conversion to CO. Structural characterization of this material was achieved using a multi-point BET method (results and discussion are presented in ESI Tables S2, S3 and ESI text†), powder XRD, STEM-EDX, and in situ XAFS measurements. The XRD patterns obtained from fresh and post-reaction samples are shown in Fig. 3A. The XRD pattern of the fresh sample showed the formation of Cu2In2O5 (ICDD 01-070-1082) with no impurity phase. The XRD pattern of the reduced sample exhibited diffraction peaks assigned to Cu–In alloy and In2O3 (ICDD 01-089-4595). The main compositions of the alloy were Cu7In3 (ICDD 03-065-2249) and Cu9In4 (ICDD 00-042-1476) (Fig. 3B and S3†). From the phase diagram of the Cu–In system, these alloys are considered to be a solid phase during the RWGS-CL cycle.21 Then, after the oxidation step, diffraction peaks of the Cu–In alloy disappeared. Those of Cu metal (ICDD 01-071-4610) and In2O3 were observed. STEM-EDX images are presented in Fig. 3C, D and ESI Fig. S4.† The SEM micrographs of the reduced Cu–In2O3 (ESI Fig. S4A†) show the presence of ca. 1 μm particles. The elemental composition of the particles was identified using EDX mapping (copper, red; indium, green; oxygen, blue). Results of EDX mapping of the sample after reduction (Fig. 3C and ESI Fig. S4A†) show that indium is distributed evenly over the particles, whereas copper is localized and oxygen is scattered. These images indicate Cu–In alloy formation by reduction. For the re-oxidized sample, many particles with uniformly distributed copper, indium, and oxygen were observed as depicted in Fig. 3D and ESI Fig. S4B.† Using image processing and analysis of these EDX images with Python (see the ESI text and Fig. S5 for details†), it was estimated that 84% of In was overlapping with Cu, and 93% of Cu was overlapping with In. These results demonstrate that a fine matrix of Cu–In–O was formed in the re-oxidized particles. The Cu in re-oxidized particles was regarded as highly distributed on the surface or incorporated into the In2O3 structure. As additional information, in situ XAFS results are presented in Fig. 3E and F (see the ESI text, Table S4 and Fig. S6–S8 for details†). The sequential change of In K-edge XANES spectra indicates that the redox of In(III) ⇆ In(0) occurs during the RWGS-CL cycle. By contrast, the Cu K-edge XANES spectrum, which only slightly changed during the RWGS-CL cycle, represented the formation of the Cu–In alloy.22,23 This structural information obtained from XRD, STEM-EDX, and XAFS measurements demonstrates that the RWGS-CL cycle on Cu2In2O5, as a parent material, is based on the redox of indium with the formation and oxidation of the Cu–In alloy. This material during RWGS-CL is denoted hereinafter as Cu–In2O3.
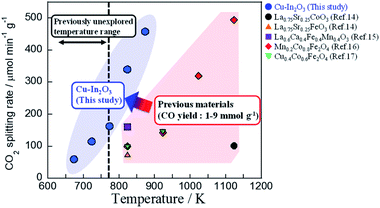 |
| Fig. 2 Average CO2 splitting rates on the Cu–In mixed oxide (this study) and on various oxides in earlier reports. | |
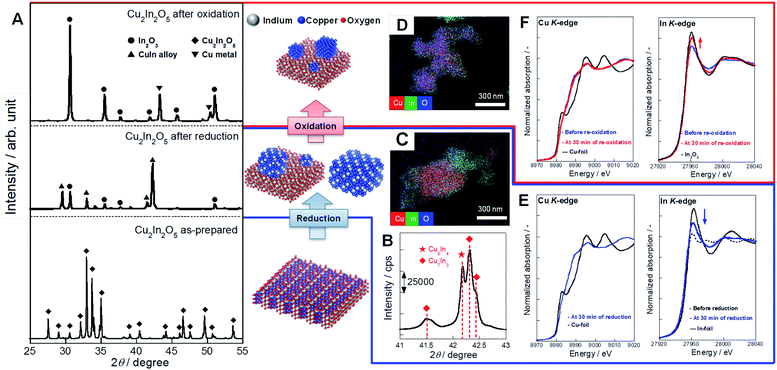 |
| Fig. 3 Structural characterization of Cu–In2O3 derived from Cu2In2O5 during each step of the RWGS-CL cycle. (A) XRD diffraction patterns of Cu2In2O5 as prepared, after reduction, and after oxidation. (B) Main diffraction peaks for the Cu–In alloy on the sample after reduction. EDX image of Cu–In2O3 after reduction (C) and re-oxidation (D) at 773 K (copper, red; indium, green; oxygen, blue). Cu and In K-edge XANES spectra of Cu–In2O3 during the reduction (E) and the re-oxidation (F). | |
To elucidate the role of Cu in Cu–In2O3, we compared the performance of isothermal RWGS-CL as a function of temperature on Cu–In2O3 (derived from Cu2In2O5), 10 wt% Cu supported on In2O3 (10 wt% Cu/In2O3) and pure In2O3 without Cu, as shown in Fig. 4. Results indicate that Cu–In2O3 is feasible for conducting RWGS-CL, even at 673 K. The performance at each temperature was much higher than that of 10 wt% Cu/In2O3 and pure In2O3 without Cu. Actually, the amount of redox on 10 wt% Cu/In2O3 was slightly higher than that of In2O3, although the CO2 splitting rate was improved only slightly. Results of structural characterization of 10 wt% Cu/In2O3 (ESI text and Fig. S9–S13† provide additional details) show that, because of the poor contact of Cu species with the In2O3 surface, 10 wt% Cu/In2O3 showed lower performance than Cu–In2O3 derived from Cu2In2O5.
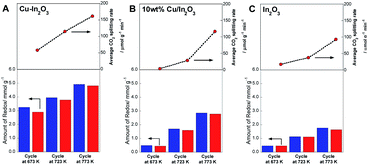 |
| Fig. 4 Dependence of RWGS-CL performance of each oxide on the reaction temperature. Amounts of redox and average CO2 splitting rate during the re-oxidation step on Cu–In2O3 (A), 10 wt% Cu/In2O3 (B), and In2O3 (C). Reduction was conducted in a 10% H2 atmosphere for 30 min; oxidation was conducted in a 10% CO2 atmosphere. | |
Kinetics investigations of oxides during the RWGS-CL cycle
Reduction step by H2.
Temperature-programmed reduction by H2 (H2-TPR) measurements, the results of which are presented in Fig. 5A, were performed to investigate the reducibility of oxides. The ESI presents additional details.† For Cu-containing oxides (Cu–In2O3 and 10 wt% Cu/In2O3), the H2-TPR profiles showed three notable reduction areas (ca. 400–550 K, 580–700 K, and 700–920 K). In the moderate temperature region of 580–700 K, the amount of reduction was equivalent to about 26% of oxygen contained in Cu2In2O5. Ploner et al. have observed that the Cu–In alloy formed at 640–723 K on the Cu–In2O3 system in an H2 atmosphere using in situ XRD measurement.24 Therefore, the reduction of In(III) with the formation of the Cu–In alloy, the composition of which finally approached Cu9In4, was regarded as proceeding in this moderate temperature region. Regarding the results of H2-TPR measurements, results show that Cu species promote the reduction of indium oxide at low temperatures. To investigate more details of the mechanism, the kinetics model for the reduction of these oxides was verified using the method presented by Hancock and Sharp for the isothermal solid-state reaction.25–27 The ESI text, Fig. S14–S17 and Tables S6–S8† present additional details. The kinetics models used for model fitting are shown in ESI Table S5.† As a result of the investigations, the phase-boundary-controlled reaction models (R2 and R3 models) were found to be suitable to describe the experimentally obtained data for all the oxides. These models are categorized as a classic shrinking core model in which the rate-controlling step is the surface chemical reaction.26,27 The activation energy was investigated using the reaction rate constant k values obtained from the model fitting described above (ESI Table S9†). Plots of ln
(kred/min−1) vs. 1/T (Arrhenius plot) for the three oxides are presented in Fig. 5B. The respective activation energies for Cu–In2O3 (45.2 kJ mol−1) and 10 wt% Cu/In2O3 (53.4 kJ mol−1) were much lower than that for In2O3 (76.6 kJ mol−1), confirming that the Cu species supported on In2O3 decreased the activation barrier for reactions between the surfaces of In2O3 and H2. The slight difference in the activation energy between Cu–In2O3 and 10 wt% Cu/In2O3 suggests that the effect of the Cu species on the reduction does not depend much on these morphologies.
 |
| Fig. 5 Kinetics analysis of each oxide in the reduction step. (A) H2-TPR profiles for Cu–In2O3 (derived from Cu2In2O5), 10 wt% Cu/In2O3 and In2O3. (B) Arrhenius plots for the reduction of Cu–In2O3 (derived from Cu2In2O5), 10 wt% Cu/In2O3 and In2O3. | |
Oxidation step by CO2 for CO formation.
Next, kinetics investigations were conducted for the oxidation step by CO2 in the RWGS-CL cycle. The kinetics model for the oxidation of the oxide was also verified using the Hancock and Sharp method. As a consequence of the model fitting (details of which are presented in ESI Fig. S18–S21 and Tables S10–S12†), the most suitable models were concluded to be a zero-order model (R1 model)25–27 for Cu–In2O3 and the nucleation model, known as the Avrami–Erofe'ev model (AE1 model), for the other two oxides.28–30Fig. 6A presents the Arrhenius plot for the oxidation of these three oxides. The reaction rate constant k values obtained from model fitting are presented in Table S13.† The activation energy for Cu–In2O3 is 50.7 kJ mol−1, which is markedly lower than that for either of the other two oxides. As the results of the model fitting show, the large difference in the activation energy is attributable to the different mechanisms of oxidation among these oxides. Considering that AE1 is suitable as a model for In2O3 and 10 wt% Cu/In2O3, the overall oxidation of these two oxides is determined by the rate of formation and growth of the indium oxide nuclei. At the beginning of the reaction, the rate of oxidation is high because of random nucleation on the surface of the reduced oxide. However, as the nucleation and growth of nuclei proceed, the rate of oxidation plummets because of the decrease of the reduced surface. As a result, a long time is necessary to complete oxidation by CO2. Fig. 6B presents different rates of CO2 splitting over these oxides during oxidation by CO2. For all oxides, the degree of reduction before re-oxidation was fixed at 3.0 mmol g−1. On In2O3 and 10 wt% Cu/In2O3, the CO2 splitting rate decreased drastically with the progress of oxidation. The oxidation of Cu–In2O3 proceeded while maintaining a constant CO2 splitting rate of about 340 μmol g−1 min−1 until conversion reached 60%. As a result, re-oxidation of the reduced Cu–In2O3 is completed twice as rapidly as that of the other two oxides. These results obtained from the kinetics investigation of Cu–In2O3 suggest that the amount of active sites for CO2 splitting remains constant while oxidation is proceeding. The oxidation state of the Cu–In2O3 surface during the oxidation step was analyzed by XPS. Compared with the In 3d5/2 peak of fully re-oxidized Cu–In2O3, that of reduced Cu–In2O3 was shifted to the lower binding energy (details in ESI Fig. S22 and ESI text†), which demonstrates the existence of In(0) species on the surface.31Fig. 6C shows the surface In/O ratio at each oxidation rate of the reduced Cu–In2O3 and 10 wt% Cu/In2O3, as measured by XPS. The In/O ratio of the Cu–In2O3 surface remained above 0.9, even at an oxidation rate of 75%, which indicates that bulk oxidation proceeds preferentially while the surface remains in a highly reduced state. This particular CO2 splitting mechanism of Cu–In2O3 is explainable as shown in Fig. 6D. First, CO2 splits on the surface of the Cu–In alloy; CO and oxide ions (O2−) are generated. Subsequently, the O2− ions migrate to the bulk of the particle. They presumably oxidize the Cu–In alloy–In2O3 interface. As a result, the alloy surface, as the active site, remains in a highly reduced state. Rapid CO2 splitting proceeds continuously.
 |
| Fig. 6 Kinetics analysis of each oxide in re-oxidation steps. (A) Arrhenius plots for the oxidation of Cu–In2O3 (derived from Cu2In2O5), 10 wt% Cu/In2O3 and In2O3. (B) Dependence of differential CO2 splitting rate on conversion of oxides. (C) Surface In/O ratios on Cu–In2O3 and 10 wt% Cu/In2O3 during the re-oxidation step. (D) Presumed mechanism of CO2 splitting on the reduced Cu–In2O3. | |
Conclusion
As reported herein, Cu–In2O3 was synthesized. Then its performance for RWGS-CL was investigated at the low temperatures of 673–773 K. Results of RWGS-CL cycle tests demonstrated that, even at low temperatures, Cu–In2O3 exhibited much higher CO2 splitting performance than ever reported. Results of XRD, STEM-EDX, and in situ XAFS measurements showed that the oxide has a structure of reduced Cu supported on In2O3 (Cu–In2O3) under the RWGS-CL conditions, and showed that its redox properties are based on Cu–In alloy formation and re-oxidation. In addition, because low performance was exhibited by pure In2O3 and Cu supported In2O3 that were prepared using an impregnation method, the structured state of Cu–In2O3 has extremely important roles in its high RWGS-CL performance. H2-TPR and kinetics investigations revealed that Cu–In2O3 shows high reducibility, even at low temperatures. We concluded that Cu species promote the reaction of H2 with the oxide surface. Results of kinetics investigations in the oxidation step indicate that the Cu–In alloy particle surface conserved a highly reduced state even under oxidation condition, resulting in rapid completion of re-oxidation by CO2. The interesting oxidation behavior is attributed to rapid O2− migration from the surface to the bulk of the Cu–In alloy and preferential oxidation of the interface of alloy–In2O3. The combination of high reducibility and the specific re-oxidation mechanism engenders the high performance found for RWGS-CL on Cu–In2O3.
Conflicts of interest
There are no conflicts to declare.
Acknowledgements
In situ XAFS measurements were performed at the beamline BL07 of the SAGA Light Source (Proposal No. 1910093P/BL07). The authors thank Dr H. Setoyama for help with measurements of XANES spectra.
Notes and references
-
IPCC, www.ipcc.ch/site/assets/uploads/2018/02/SYR_AR5_FINAL_full.pdf Search PubMed.
- S. J. Davis, K. Caldeira and H. D. Matthews, Science, 2010, 329, 1330–1333 CrossRef CAS.
- S. Perathoner and G. Centi, ChemSusChem, 2014, 7, 1274–1282 CrossRef CAS.
- J. Artz, T. E. Müller, K. Thenert, J. Kleinekorte, R. Meys, A. Sternberg, A. Bardow and W. Leitner, Chem. Rev., 2018, 118, 434–504 CrossRef CAS.
- D. S. Mallapragada, N. R. Singh, V. Curteanu and R. Agrawal, Ind. Eng. Chem. Res., 2013, 52, 5136–5144 CrossRef CAS.
- F. V. Vázquez, J. Koponen, V. Ruuskanen, C. Bajamundi, A. Kosonen, P. Simell, J. Ahola, C. Frilund, J. Elfving, M. Reinikainen, N. Heikkinen, J. Kauppinen and P. Piermartini, J. CO2 Util., 2018, 28, 235–246 CrossRef.
- I. Dimitriou, P. García-Gutiérrez, R. H. Elder, R. M. Cuéllar-Franca, A. Azapagic and R. W. K. Allen, Energy Environ. Sci., 2015, 8, 1775–1789 RSC.
- M. Romero and A. Steinfeld, Energy Environ. Sci., 2012, 5, 9234–9245 RSC.
- P. Kaiser, R. B. Unde, C. Kern and A. Jess, Chem. Ing. Tech., 2013, 85, 489–499 CrossRef CAS.
- Y. A. Daza and J. N. Kuhn, RSC Adv., 2016, 6, 49675–49691 RSC.
- K. Oshima, T. Shinagawa, Y. Nogami, R. Manabe, S. Ogo and Y. Sekine, Catal. Today, 2016, 232, 27–32 CrossRef.
- S.-C. Yang, S. H. Pang, T. P. Sulmonetti, W.-N. Su, J.-F. Lee, B.-J. Hwang and C. W. Jones, ACS Catal., 2018, 8, 12056–12066 CrossRef CAS.
- M. Wenzel, L. Rihko-Struckmann and K. Sundmacher, AIChE J., 2017, 63, 15–22 CrossRef CAS.
- Y. A. Daza, D. Maiti, R. A. Kent, V. R. Bhethanabotla and J. N. Kuhn, Catal. Today, 2015, 258, 691–698 CrossRef CAS.
- D. Maiti, B. J. Hare, Y. A. Daza, A. E. Ramos, J. N. Kuhn and V. R. Bhethanabotla, Energy Environ. Sci., 2018, 11, 648–659 RSC.
- L. Ma, Y. Qiu, M. Li, D. Cui, S. Zhang, D. Zheng and R. Xao, Ind. Eng. Chem. Res., 2020, 59, 6924–6930 CrossRef CAS.
- Y. Qiu, L. Ma, D. Zeng, M. Li, D. Cui, Y. Lv, S. Zhang and R. Xiao, J. Energy Chem., 2020, 46, 123–132 CrossRef.
- D. Zeng, Y. Qiu, L. Ma, M. Li, D. Cui, S. Zhang and R. Xiao, Environ. Sci. Technol., 2020, 54, 12467–12475 CrossRef CAS.
- N. Utsis, M. V. Landau, A. Erenburg and M. Herskowitz, Catalysts, 2020, 10, 1082 CrossRef CAS.
- X. Zhu, K. Li, L. Neal and F. Li, ACS Catal., 2018, 8, 8213–8236 CrossRef CAS.
- Z. Bahari, E. Dichi, B. Legendre and J. Dugué, Thermochim. Acta, 2003, 401, 131–138 CrossRef CAS.
- M. G. Kim, S. Sim and J. Cho, Adv. Mater., 2010, 22, 5154–5158 CrossRef CAS.
- W.-R. Lee, M. G. Kim, J.-R. Choi, J.-I. Park, S. J. Ko, S. J. Oh and J. Cheon, J. Am. Chem. Soc., 2005, 127, 16090–16097 CrossRef CAS.
- K. Ploner, L. Schlicker, A. Gili, A. Gurlo, A. Doran, L. Zhang, M. Armbrüster, D. Obendorf, J. Bernardi, B. Klötzer and S. Penner, Sci. Technol. Adv. Mater., 2019, 20, 356–366 CrossRef CAS.
- J. D. Hancock and J. H. Sharp, J. Am. Ceram. Soc., 1972, 55, 74–77 CrossRef CAS.
- K. Piotrowski, K. Mondal, H. Lorethova, L. Stonawski, T. Szymański and T. Wiltowski, Int. J. Hydrogen Energy, 2005, 30, 1543–1554 CrossRef CAS.
- Z. Zhou, L. Han and G. M. Bollas, Int. J. Hydrogen Energy, 2014, 39, 8535–8556 CrossRef CAS.
- M. Avrami, J. Chem. Phys., 1939, 7, 1103–1112 CrossRef CAS.
- M. Avrami, J. Chem. Phys., 1940, 8, 212–224 CrossRef CAS.
- M. Avrami, J. Chem. Phys., 1941, 9, 177–184 CrossRef CAS.
- J. Gao, F. Song, Y. Li, W. Cheng, H. Yuan and Q. Xu, Ind. Eng. Chem. Res., 2020, 59, 12331–12337 CrossRef CAS.
Footnote |
† Electronic supplementary information (ESI) available: Experimental procedure, supporting data etc. See DOI: 10.1039/d0sc05340f |
|
This journal is © The Royal Society of Chemistry 2021 |
Click here to see how this site uses Cookies. View our privacy policy here.