DOI:
10.1039/D2MA00700B
(Review Article)
Mater. Adv., 2022,
3, 7198-7211
Recent advancement in efficient metal oxide-based flexible perovskite solar cells: a short review
Received
17th June 2022
, Accepted 6th August 2022
First published on 9th August 2022
Abstract
Solar beams contain solar energy, which releases a great deal of energy. Over the last few years, the interest in and progress toward environmentally sound technologies has increased tremendously. Clean energy is being implemented, and solar cell technology is winning the race. Perovskite solar cells (PSCs) are a possible alternative to conventional Si-based solar cells, which are heavy, expensive, and fragile. As a result, PSC development has advanced very well in a short period of time. For the continuous and efficient conversion of solar energy, a variety of metal oxides have been used to develop different devices for terrestrial and space photovoltaics. These metal oxide materials have an encouraging perspective due to their vast accessibility, non-destructiveness, and chemical stability and can provide an extensive diversity of choices for designing and fabricating the device under ambient conditions. The efficiency and stability of PSC devices are increased thanks to the physical, chemical, and electrical features of metal oxides. An original, reliable, and comprehensive method for developing efficient metal oxide-based flexible PSCs is to optimize the preparative parameters for metal oxides. This short review is enthusiastically discusses about metal oxides and how they affect flexible perovskite solar cells, which have been integrated with energy-level compatibility. The recent advancement of metal oxide-based flexible perovskite solar cells has also been discussed and we expect the development of low-priced practicable power stock to advance ecofriendly feasibility in the coming years using flexible perovskite solar cells.
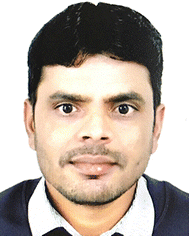
Subhash Chander
| Dr Subhash Chander is a Dr D. S. Kothari Postdoctoral Fellow at the Department of Physics at Panjab University, Chandigarh (India). He obtained his PhD from Mohanlal Sukhadia University, Udaipur (India). His area of research is mainly focused on solar photovoltaics and he has more than 60 publications in peer-referred journals with an h-index of 25. His research work is related to enhancing the performance and reduction in the cost of solar cells can help to provide more abundant electricity worldwide. |
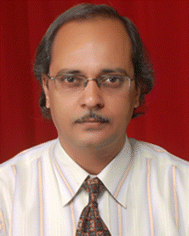
Surya Kant Tripathi
| Dr Surya Kant Tripathi is a Senior Professor at the Department of Physics at Panjab University, Chandigarh (India). He obtained his PhD in 1991 from Kanpur University, Kanpur (India). He has published more than 300 research papers in referred journals and has handled about 15 major research projects. His area of interest is in the field of experimental condensed-matter physics, nanoscience & nanotechnology, thin films, and device fabrication. |
1. Introduction
The requirement of energy is rising at an astonishing proportion to meet the stride of methodical developments and increasing world population. Currently, the three primary fossil fuels (oil, natural gas, and coal) accomplish more than 75–80% of global energy loads.1,2 The amount of energy consumed globally each year is currently 15 TW, but it is predicted to increase to 30 TW by 2050. Of this, around 80% of the demand will be met by these fossil fuels, while the rest come from nuclear power, hydropower, and renewable energy sources. On the one side is this increased power consumption, while the other is the depletion of natural resources and raw materials, increasing manufacturing prices, and serious ecological concerns such as the climate catastrophe brought on by the unjustified use of non-renewable energy sources.
Because fossil fuels contribute to global warming and produce greenhouse gases when they are burned, the threat to the earth's ability to support life is increased. These conventional energy sources are insufficient and based on current consumption rates, it is predicted that oil, natural gas, and coal reserves will run out in the next 50, 60, and 120–150 years, respectively. Although it may seem that fossil fuels will continue to dominate the energy sector, one must consider the high risks that these fuels pose to our planet's atmosphere. For example, a 35% increase in CO2 levels will result in an increase in the global temperature of 1 °C, which would induce a sea level to rise.3–5
The risk to the survival of civilization has increased due to the continuously rising energy consumption, which has a detrimental effect due to the greenhouse emissions and the climate catastrophe, it also indirectly sparks struggle over the availability of non-renewable energy sources. Although the situation has been significantly prevented by improvements in the renewable energy sector, many tasks still need to be completed. Less than 20% of the world's energy demand is met by renewable energy sources.3 Investments, government policies, industrial expansion and demand, public awareness, and other factors, all play a role in the rapid development of the renewable energy sector. Many nations, both developed and developing, are attempting to increase the production of renewable energy, but the demand for clean, sustainable resources has decreased due to a number of issues, including affordability, mobility, and usability. To meet these challenges, considerable financial and human resources are devoted to research and development in renewable energy. Due to the abundance of resources, the direct conversion of energy into electricity without any intermediate states, the stability and long life of solar cells, and photovoltaic energy is one of the primary concentrations among renewable energies. In a decade or two, electricity-based systems will replace hydrocarbon fuels due to the rapid development of electric vehicles by top manufacturers such as Tesla and Mahindra. It will be futile to generate power for cars utilizing fossil fuels. This issue can only be resolved through photovoltaic electricity production. The amorphous Si thin-film solar cell was developed in the late 1970s, and it is comparable to conventional crystalline Si-based solar cells in terms of flexibility and light weight. The structural rigidity and up to this point flexible thin-film modules provide incredibly original energy source possibilities. The mechanically rigid and thus far flexible modules based on thin films offer tremendously unique energy source solutions.6 In recent times, new material schemes (viz., organic semiconductors and metal halide-based perovskites) have make a swift advancement. Consequently, in the coming years, flexible solar cells are anticipated to be marketed along with several other commercial goods. The efficiency of the current commercial solar cell modules based on crystalline and amorphous Si is 25.1%, but they are too expensive. On the other hand, the biggest obstacles in expanding the commercialization of this solar cell technology are the price of manufacture and the complexity of manufacturing devices. Thin film-based solar cells using cadmium telluride (CdTe/CdZnTe) and copper–indium–gallium–selenide/sulfide (CIGS/CIGSSe) have thus been extensively explored and commercially produced. These cells have demonstrated photovoltaic cell efficiencies and are less expensive and material-intensive.7–9 The efficiencies of various single junction-based solar cell technologies are compiled in Table 1.
Table 1 Power conversion efficiency (PCE) of different single-junction solar cell devices at 25 °C with a global AM1.5 spectrum (1000 W m−2). Adapted and modified with permission from ref. 7, Copyright (2021), Wiley
Classifications |
V
OC (V) |
J
SC (mA cm−2) |
FF (%) |
PCE (%) |
Crystalline cell |
0.738 |
42.65 |
84.9 |
26.7 |
Large-crystalline cell |
0.747 |
40.60 |
83.8 |
25.5 |
GaAs (thin film cell) |
1.127 |
29.78 |
86.7 |
29.1 |
GaAs (multi-crystalline) |
0.994 |
23.2 |
79.7 |
18.4 |
InP (crystalline cell) |
0.939 |
31.15 |
82.6 |
24.2 |
GaInP |
1.493 |
16.31 |
87.7 |
21.4 |
|
Thin film solar cells |
CIGS |
0.992 |
35.70 |
77.6 |
22.9 |
CIGSS (Cd-free) |
0.685 |
39.91 |
76.4 |
20.9 |
CdTe |
0.887 |
31.69 |
78.5 |
22.1 |
CZTSS |
0.513 |
35.21 |
69.8 |
12.6 |
CZTS |
0.730 |
21.74 |
65.1 |
11.1 |
|
Amorphous/microcrystalline |
Amorphous silicon cell |
0.896 |
16.36 |
69.8 |
10.2 |
Microcrystalline silicon cell |
0.550 |
29.72 |
75.0 |
11.9 |
|
Other PV technology |
Dye-sensitized cell |
0.744 |
22.47 |
71.2 |
11.9 |
Organic cell |
0.896 |
25.72 |
78.9 |
18.2 |
Perovskite cell |
1.179 |
25.80 |
84.6 |
25.7 |
The highest theoretical efficiency of 33% (for single junction solar cell) predicted by Shockley–Queisser (known as the S–Q limit) is significantly higher than the reported efficiencies of single-junction Si and thin film solar cells, which range between 20 and 25%. Thus, there is a pressing need for simple, inexpensive technologies to produce solar cells that can attain high efficiencies. With the rapid advancement in recent times, some new material schemes, viz., organic semiconductors and metal halide-based perovskites10–15 and some emerging photovoltaics concepts such as water-based and integrated solar energy devices have to make a swift advancement;16,17 consequently, in the coming years, flexible solar cells are anticipated to be marketed along with several other commercial goods.
2. Metal oxide nanostructures
Metal oxides have such a wide range of physicochemical and optoelectronic features, and they have continued to be one of the most alluring materials. In comparison to conventional semiconducting materials, metal oxides are advantageous because they have more degrees of freedom, which allows for the better modification of the optoelectronic and other properties. For instance, altering the oxygen-octahedral inclines in perovskite oxides can have a significant impact on the oxides' characteristics. The vast uses of transition-metal oxides (TMOs) in sensors, solar cells, fuel cells, and other devices have drawn broad attention among the various classes of oxides.18 TMOs are composed of oxygen atoms and transition metals and can exist in the forms of monoxide, dioxide, trioxide, etc., due to the multivalent nature of transition metals. Fig. 1 demonstrates the molecular orbital (MO) diagram of 5d-orbitals, which play an essential part in several applications of TMOs.
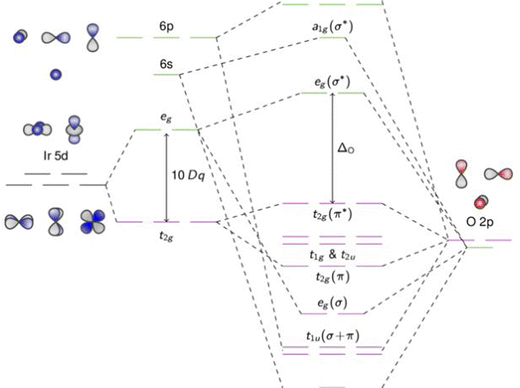 |
| Fig. 1 Molecular orbital (MO) diagram of 5d-orbitals transition-metal oxides (TMOs) cation octahedrally coordinated to six oxygen ligands. The MOs are hybridized and have character arising both from the transition metal and ligand atomic orbitals. Adapted from ref. 19. | |
One of the widely contested topics in the world of oxide electronics is the use of metal oxide nanostructures for various specific applications. Over the past three decades, scientists have used various chemical or physical evolution techniques with a wide selection of morphologies to produce metal oxide nanostructures (MONs). These nanostructures can be utilized in photovoltaics as a scaffold layer for hybrid perovskite solar cells and dye-sensitized solar cells (DSSCs) (HPSCs). In DSSCs and organic solar cells, these nanostructures can also be employed as the charge transport layers (electrons and holes). The transport layers are used to deport one type of charge carrier block to another type, while the function of the framework/scaffold layer is to separate and transfer the charges. Therefore, the changes to their photophysical characteristics are necessary to develop flexible solar cells with high efficiency.20 Such properties highlight different metal oxides such as titanium dioxide (TiO2), zinc oxide (ZnO), tungsten trioxide (WO3), and copper oxides (Cu2O, CuO) for their use in flexible solar cells. These materials are the most prevalent and are also the most reliable in terms of profitability, stability, and efficacy. This article also highlights the photophysical and physicochemical characteristics of such metal oxides, as well as new developments, experiments, and modifications made to such metal oxides to overcome their limitations and optimize their performance in photovoltaic applications. Table 2 lists the uses of some interesting materials based on such metal oxides prepared in various nanostructures. Several MONs have been reported, such as nanoparticles, nanowires, nanotubes, nanosheets, nanowhiskers, and nanospheres. These materials in nano form might be used to different applications, viz., catalysis, microelectronic circuits, transistors, energy storage and conversion devices, biomedicines, and sensors.18 Titanium dioxide (TiO2) nanostructures can be developed using a variety of processing methods such as sol–gel, hydrothermal, and co-precipitation methods,21 as shown in Fig. 2.
Table 2 Uses of some illustrative metal oxides-based materials with various structures. Adapted with permission from ref. 22, Copyright (2020), MDPI
Metal oxide-based nanostructures |
Structural design |
Preparation method |
Usages |
ZnO Nanosheets |
3D hierarchical flower-like architectures |
Solvothermal |
Adsorption of triphenylmethane dyes |
Fe3O4@UiO-66 composite |
Cube-shaped nanoparticles |
Sonication |
Adsorption |
Fe3O4@MIL-100(Fe) core–shell bio-nanocomposites |
Core–shell structure having Fe3O4 as core |
Sonication followed by attaching the nanoparticles on bacteria |
Adsorption |
ZnO + TiO2/clay |
TiO2 and ZnO nanoparticles mounted on the clay surface |
Sol–gel process |
Degradation of MG |
Cu/ZnO/Al2O3 |
Cu and ZnO-impregnated γ-Al2O3 |
Penetration method |
Removal of CO from renewed coal |
Co2+/Ni2+-Doped Fe3O4 nanoparticles |
Cubic lattice |
Co-precipitation process |
Photodegradation of Carbol Fuchsi |
Ce/Fe bimetallic oxides (CFBO) |
Flower-like 3D hierarchical architecture |
No-pattern hydrothermal process |
As5+ and Cr4+ correctness |
Perovskites titanate (ATiO3, A = Sr/Ca/Pb) |
Leaf-engineered 3D hierarchical structure |
Amalgamation of bio-synthesis from Cherry Blossom, heating, grinding, and photodeposition |
Artificial photosynthetic systems for the photoreduction of CO2 |
TiO2 polypyrrole |
Core–shell nanowires |
Seed-assisted hydrothermal process |
Elastic supercapacitors |
Fe3O4/WO3 |
Hierarchical core–shell structures |
Solvothermal growth + oxidation route |
Photodegradation of organic-dye materials |
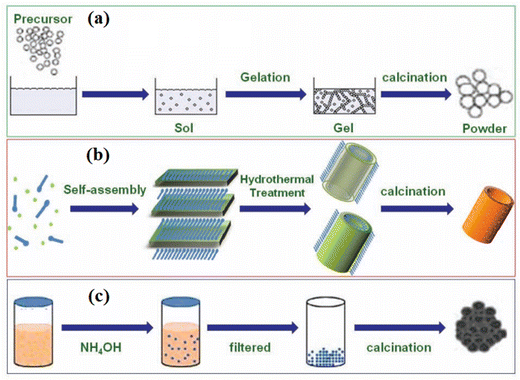 |
| Fig. 2 A schematic representation of the chemical synthesis of MONs employing different processing methods, i.e., (a) sol–gel, (b) hydrothermal, and (c) co-precipitation. Adapted from ref. 21. Copyright (2017), Royal Society of Chemistry. | |
The cross-sectional view of the reasonably homogeneous TiO2 nanostructure, with a thickness of 149 nm, is shown in the inset of the FESEM image (Fig. 3), which shows the spherical surface morphology of the SILAR (successive ionic layer adsorption and reaction)-processed TiO2 nanostructure. The elemental surface composition analysis (EDX) verified that the amounts of Ti and O in TiO2 were stoichiometric. The (101) crystal face of TiO2 was more stable than the other facets due to its lower surface energy and HR-TEM images that also showed the strongest ring pattern for the (101) plane than others in the SAED spectrum. The diffraction bright and sharp ring pattern approves the nanocrystalline nature of the TiO2 nanostructure. Based on these properties, a perovskite solar cell was fabricated using SILAR-grown TiO2 nanostructures as the electron transport layer and efficiency of 9.7% was attained.23 Besides the morphology of metal oxide nanomaterials, doping of metal oxides, which favors the low-temperature process of carrier transport layers for flexible metal oxide-based PCSs, can improve the device performances.24,25
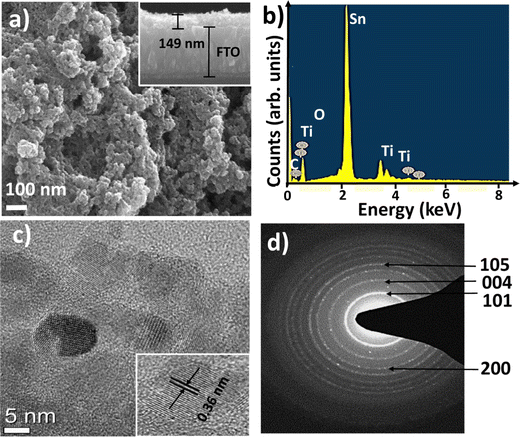 |
| Fig. 3 (a) FESEM plan-view image, (b) EDX pattern, (c) HR-TEM image, and (d) SAED pattern of SILAR-grown TiO2 film. Adapted with permission from ref. 23. Copyright (2018), Springer Nature Research. | |
The rutile TiO2 nanorods/films prepared by the hydrothermal method was also discussed, where these nanorods/films were integrated into the flexible PSCs.26 It was found that the efficiency was dependent on the nanorod length, while the current density and open-circuited voltage decreased with increasing nanorod length. The preparation of MONs with well-defined size and morphology is an imperative test in photovoltaic applications. The dimension of the MONs may be large, which could be useful for mesostructured-based flexible PCSs. Though, for planar flexible PCSs, which have been very widely used in both rigid and flexible photovoltaic, the large nanostructures have big concerns in roughness-related issues. The metal oxide nanomaterials prefer the planar structure-based flexible PSCs, while the process temperature needs to be low and the metal oxide layer needs to be high quality (well-pack, smooth, and pin-hole free) for such types of flexible PSCs.27,28
3. Metal oxide-based flexible solar cells
One of the most significant sources of renewable energy for sustainable development in the coming years are thin-film solar cells, quantum-dot solar cells, dye-sensitized solar cells, organic solar cells, and perovskite solar cells. Because they are inexpensive, simple to prepare, and have adjustable optical characteristics, these solar cell devices have received widespread attention.6,29 In the last decade or so, the efficiency of perovskite solar cells has grown significantly, rising from 3.8% to 25.7%, and is equivalent to conventional silicon solar cells.7 The metal-oxide-based electron transport layers (viz., TiO2, ZnO, SnO2, NiO, Al2O3, V2O5, ZrO2, CeO2, and Nb2O5) are used to achieve the high PCE. These devices have received widespread approval due to their affordable, high efficacy, and simple manufacturing processes.30 Scientists have also been attracted by the metal-oxide heterojunction devices because of their high stability and low price. The metal oxides are intrinsic semiconducting materials and they have both types of conductivities, i.e., n-type (viz. TiO2, Fe2O3, and ZnO) and p-type (viz. CuO, Cu2O, and Co3O4).31,32
Metal-oxide semiconducting materials with small bandgaps can be employed as an absorber layer, while metal-oxide semiconducting materials with broad bandgaps are typically used as window layers. To prevent any short-circuits, the metal oxide-based transport layers in perovskite solar cells can function as compact layers.33 Since TiO2 and ZnO have a broad bandgap more than 3 eV and are transparent to visible light, it makes them ideal alternatives for transparent window layers. ZnO is a direct bandgap material that is frequently utilized in metal oxides-based flexible solar cells.34 TiO2 is often used to fabricate DSSCs and is primarily found as indirect bandgap semiconductors.35 Among various p-type metal oxides, the copper oxides are the utmost widespread materials for solar cells. Stable copper oxides (Cu2O and CuO) are p-type semiconducting materials with a direct energy band gap of ∼2.1 eV and 1.2 eV, respectively. These semiconducting materials are ideal candidates as an absorber layer for solar cells owing to their high absorption (in the visible area) and decent minority charge carriers diffusion length.36 Cobalt oxide is another potential p-type semiconducting material having good visible-light absorption. Co3O4 has a spinel structure and mixed valence states, while CoO is a more stable state of cobalt oxide with a very high bandgap (∼5 eV); therefore, Co3O4 is the phase most frequently used in photovoltaic applications. Numerous metal oxide-based flexible solar cells with different combinations of these metal oxides have been reported in the literature. The most common combination is ZnO–Cu2O-based heterojunction solar cells, but despite having a theoretical efficiency of 18%, these cells exhibit very low efficiencies. Irrespective of the methods used by the investigators, the increase in the efficiency is still quite small. Among many of these reported works, a few outstanding efforts are examined here.
The development of three different forms of ZnO–Cu2O-based flexible solar cells have been done by Wei and co-workers,37 where they used ZnO as thin film, nanowire, and nanotube with a thin film of copper oxides. ZnO wires and tubes demonstrate somewhat greater efficiency as compared to thin films, of about 0.12%. ZnO thin films had relatively low efficiencies of 0.02%. The increased performance of the solar cells made of nanotubes and nanowires may be attributed to an expansion of the heterojunction region between Cu2O and ZnO. Similar solar cells have been discussed utilizing ZnO–Cu2O heterojunction, where the nanorod and nanotube of ZnO with Cu2O films have been used.38 ZnO nanorods demonstrated a photovoltaic efficiency of 0.4%, whereas ZnO nanotubes demonstrated a photovoltaic efficiency of 0.8%. Due to the greater surface area covered by ZnO nanotubes, the efficiency of the nanotubes was double that of nanorods.
Through this method, Zhang's team39 developed flexible solar cells made of ZnO and Cu2O thin films that were arranged in 3D square-patterned nanorod arrays. These cells showed a substantial improvement over cells without patterns, showing an efficiency of 1.52% and a current density of 9.89 mA cm−2. By experimenting with different deposition techniques, several other researchers have attempted to improve the performance of Cu2O-based heterojunction solar cell devices. Wee and colleagues40 used pulsed-layer deposition to deposit Cu2O thin films on the rough metallic substrate. They developed a flexible photovoltaic device, as shown in the schematic in Fig. 4a, with an epitaxial Cu2O absorber layer, a thick SrRuO3 conductive oxide layer (about 100 nm) between Cu2O and SrTiO3 layers, and then n-type transparent conductive oxide layers made of ZnO and Al-doped ZnO layers on top of the Cu2O layer. This pure epitaxial phase of Cu2O produced an efficiency of 1.65%. The flexible ZnO/Cu2O solar cells exploiting the electrodeposition method was designed by Pławecki and team,41 and they reported the influence of Cu2O thickness on the power conversion efficiency, which was found to be 2.7%.
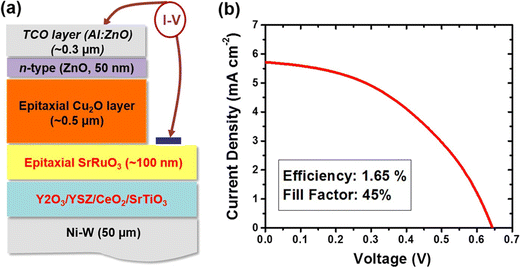 |
| Fig. 4 (a) Device design and (b) current–voltage characteristics of a flexible solar cell, which has an epitaxial Cu2O absorber-layer on the textured metallic substrate. Adapted from ref. 41, Copyright (2015), Springer Nature Research. | |
By applying SILAR approach, Chatterjee et al.42 coated an additional NiO layer above the Cu2O layer as the hole-transport and electron-block layer and SnO2 on the ZnO side, as demonstrated in Fig. 5. The prepared heterojunction solar cells with the design of NiO/Cu2O/ZnO/SnO2 made it easier for electrons and holes to flow through them efficiently (owing to staircase-like energy levels), resulting in a photon conversion efficiency of 1.12%, an FF of 50%, and a short-circuited current density of 3.50 mA cm−2. The high purity Cu2O layers formed using thermal oxidation produced a flexible solar cell with a high efficiency of 3.83%, where high purity copper sheets were being oxidized to Cu2O by heating at 1010 °C in a carefully controlled environment, whereas the ZnO layers were being doped with aluminium.43 In their study, Kelly's group44 used a bending test to examine various electrodes on the PET substrates and discovered that metal oxide electrodes had evident cracks and a rapid increase in the sheet resistance, while the PEDOT:PSS electrodes had barely changed.
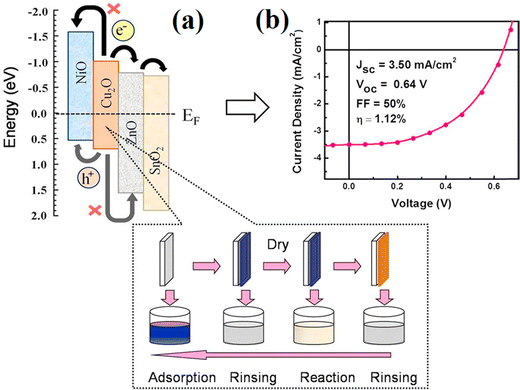 |
| Fig. 5 The schematic energy level diagram and current–voltage characteristics of the NiO/Cu2O/ZnO/SnO2 heterojunction flexible solar cell. Adapted with permission from ref. 42, Copyright (2016), Elsevier. | |
The above discussion make it clear that the purity of copper oxide plays a crucial role in increasing the effectiveness of solar cell devices, yet producing such high purity Cu2O makes the production of solar cells difficult and expensive. When compared to Cu2O, there are very few publications on the photophysical characteristics of CuO and the efficiency achieved was less than 2% due to a small carrier concentration, but it has a great bandgap for developing flexible solar cells. CuO-based flexible solar cells can also be made using n-type silicon, which showed an efficiency of 0.36%,45 but electroplated TiO2–CuO-based solar cells had an efficiency of about 1.6%.46 Cu2O and CuO are p-type materials with appropriate work functions and low-lying valence bands that can complement the perovskite layer well and reduce energy loss. Thus, researchers have spent a lot of time recently studying the flexible solar cells, which are crucial to the creation of third-generation solar cells because metal oxides are one of the most critical constituents of third-generation solar cells. Metal oxide electron collector layers are often in charge of transporting electrons in third-generation solar cells. The consistency of components, such as sensitizers in dye-sensitized solar cells, organic dye in organic solar cells, organic–inorganic perovskite in PSCs, quantum dots in quantum dot-based solar cells, and electrodes in CIGS solar cells, stimulated by the Sun and flexible transparent substrates, is another key role of metal oxide layers.
4. Metal oxides-derived flexible perovskite solar cells
Because metal oxide nanostructures are thought to be correlated with promising functional materials, there is a lot of current research being done on them. Metal oxide nanostructures (MONs) are good contenders as flexible transparent electrodes for perovskite solar cells because of their higher transmittance, lower sheet resistance, and superior bending property equated to those of the conventional transparent electrodes. The porous scaffolding layer underlying the perovskite active layer may assist in crystallizing the technology or expand the surface-active-area, while the metal oxide-based nanostructured layers serve as compact layers in PSCs to prevent any short-circuit problems.33 The sandwiched construction of typical PSCs normally consists of two electrodes and a photoactive layer, as shown in Fig. 6. In recent times, metal oxides have been widely studied as electron transport layers for high-efficiency PSCs owing to their excellent electronic properties, superb versatility, and great stability. The three common structures of PSCs are: (a) semiconductor mesoporous-designed PSCs, in which a semiconducting material is somewhat solution-administered as a porous scaffolding layer to carry a mesosuper-structured solar cell, (b) thin-film based PSCs, for which no pores is required, while a perovskite active layer is compressed between p- and n-type charge extracting electrodes, and (c) PSCs based on p–n heterojunction, where the perovskite active layer is porous.47
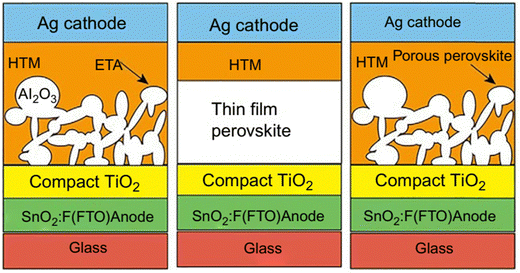 |
| Fig. 6 Typical configuration structures of a PSC. Adapted with permission from ref. 47, Copyright (2018), Elsevier. | |
In 1958, the Danish scientist Christian Mller48 revealed the first semiconducting properties of perovskites along with the first crystallographic studies of cesium–lead–halides ((CsPbX3, where X = halides, i.e., Cl, Br, or I)).48 After 20 years, Dieter Weber49 developed novel organic–inorganic hybrid three-dimensional perovskites in 1978, replacing cesium with methyl-ammonium cations. However, Zhai and colleagues were the first to show that organometallic halide perovskites have good performance.50 In the organic–inorganic hybrid PSCs, the morphological properties are strongly influenced by the various crystalline phases such as tetragonal, orthorhombic, and cubic, which vary with the operating solvent and temperature. However, the modification in the morphology can affect the electrical characteristics.51–53 Acting as a photoactive layer in flexible solar cells, the perovskite demonstrates numerous extraordinary aids as its tunable direct band gap can be altered between 1.24 eV and 3.55 eV by exploiting mixed ions for single-junction flexible solar cell devices. The perovskites having a slender bandgap (<1.65 eV) are suitable for single junction-based solar cell devices, while perovskites having a wide bandgap (1.70–1.80 eV) have been demonstrated to be appropriate for multi-function devices as a top sub-cell.54 At present, all efficient PSCs embrace an active absorber layer of intrinsic perovskites material (i), which is between the positive and negative charge-extracting layers (as known as p- and n-type interfaces); these layers are also labeled as ETL and HTL, respectively. The halide perovskite solar cells are typically categorized into three device architectures: mesoporous n–i–p, planar n–i–p, and planar p–i–n solar cell devices.55 The foremost effort was made in 2013 to apply perovskites as an absorber layer in the flexible PSCs, where the primary efficiency was about 2.6%.56 However, the poor long-term stability and relatively low performance are imperative aspects preventing the development of flexible PSCs. Compared to rigid PSC fabricated on a glass substrate, each layer that makes up the complete flexible PSC device has some special requirements, including the flexible substrate, transparent flexible electrodes, electron transport layer (ETL), perovskite layer (PL), hole transport layer (HTL), and top electrode (E), as demonstrated in Fig. 7.57 A sharp upsurge in the efficiency of PSCs is attained in the last few years, which is discussed in the next section.
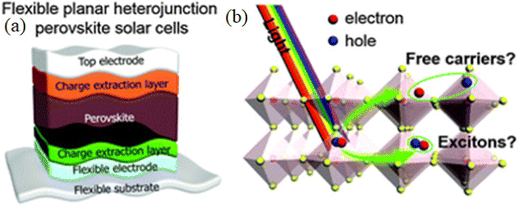 |
| Fig. 7 (a) Planar heterostructure of flexible perovskite solar cell and (b) charge carrier extraction after the photoexcitation of the perovskite. Modified and adapted from ref. 57, Copyright (2016), Royal Society of Chemistry (RSC). | |
5. Recent development in metal oxides-based flexible perovskite solar cells
In recent times, metal oxides have been widely studied as electron transport layers for high-efficiency PSCs owing to their excellent electronic properties, superb versatility, and great stability. Photovoltaic devices based on perovskites are undoubtedly the most promising competitors for next-generation high-efficiency solar cell technologies that are compatible with inexpensive, flexible substrates, and large-area construction developments. High-efficient PSCs are made of perovskites that have a 3D crystalline structure (in the form of ABX3) and are generally formed by an organic/inorganic mono-valent cation, (A = CH3NH3+, CH(NH2)2+, Cs+, Rb+), a bivalent metal-cation, (B = Pb2+; Sn2+), and halide anion motif (X3 = I−/Br−/Cl−). The scientists have become interested in perovskites owing to their easy fabrication methods based on solution precipitation or evaporation, such as a one-step deposition, two step successive deposition, and vapor-induced solution processing. The quality of the perovskite films is determined by the process of growth/deposition. The organic-inorganic hybrid perovskites (OIHPs), which have shown photoconversion efficiencies (PCEs) comparable to those of Si, CdTe, and CIGS solar cells, have advanced significantly over the past decade or so, replacing the methyl-ammonium-lead-halide (MAPbX3)-based perovskites as light harvesting materials.7,53 The most frequently utilized ETL in the systematic development of a PSC is TiO2, which is typically produced by sintering a precursor solution at a temperature between 450 °C and 500 °C. The aforementioned practice not merely raises the engineering budgets but also limits the applications of flexible plastic substrates as well. As a result, several alternatives as inorganic ETLs have been developed, including ZnO, SnO2, Fe2O3, In2S3, and WOx. Few triplex metal oxides, viz., Zn2SnO4, BaSnO3, BaTiO3, SrTiO3, and Nb:SnO2, have also been investigated as ETLs in perovskite-based solar cell systems.58 There are two different types of stabilities that are linked to flexible PSCs. One is the mechanical stability, which is frequently constrained by the electrodes' mechanical robustness. The interfacial layers and perovskite absorbers, which govern this, also contribute to long-term environmental stability. According to Miyasaka and colleagues,59 who developed the novel photovoltaic cell devices on a DSSCs outline with the thin perovskite layers of CH3NH3PbI3 (MAPbI3) and CH3NH3PbBr3 (MAPbBr3) on the mesoporous TiO2, the organic–inorganic perovskites were first used as a sensitizer in liquid-state DSSCs in 2009, where the devices demonstrated efficiencies within the range of 3.1–3.81%. However, because of the liquid electrolyte's scarring tendencies, the consistency of such devices remained below par. A similar perovskite-sensitized photovoltaic cell with an enhanced PCE of 6.5% was developed in 2011 by Park and his colleagues60 using perovskite quantum dots as sensitizers. Later, in 2012, his team designed solid-state mesoscopic PSCs employing solid-state spiro-MeOTAD as a hole transport material in order to improve the cell stability.61 This was done by looking into the corrosion processes connected to liquid electrolytes. They used a one-stage/step process to make a perovskite solar cell with a solid-state electrolyte using a solution-based process, and they were able to achieve an efficiency of 9.7%. In addition, they found that the hole-transporting material layer could dissolve in organic solvents (chlorobenzene and toluene), whereas the hybrid perovskite cannot, and that hybrid perovskite was easily deposited on top of this layer. The mesoporous alumina scaffold layer (Al2O3) was used by Snaith's group62 to develop the meso-superstructure solar cells and achieved a power conversion efficiency of 10.9% for the first time. The large bandgap of alumina prevents it from assisting in electron extraction, which suggests that perovskites can transport electrons effectively without the use of a nanoporous layer (the purpose of the nanoporous layer is to collect electrons that cannot be elevated to thin films by creating additional interfaces between metal oxides and perovskites).63 The PSCs produced using sequential deposition techniques showed a PCE of 15% with excellent repeatability.64 Later, Snaith's team developed planar heterojunction PSC for the further development in PCE up to 15.4%65 using the vapor deposition method. Subsequently, Seok's team66 removed the combined planar and scaffold layer of mixed halide CH3NH3Pb(I1−xBrx)3, which raised the efficiency upto 17.9%. In this case, the chemical compositions and layer thickness were both adjusted.
Choy and co-workers have reported several low-temperature processed metal oxides as hole67,68 and electron69,70 transport layers for high efficiency and stable perovskite solar cells. They demonstrated a controllable approach to form surface-nanostructured and flawless NiOx film as hole transport layers, while TiO2 and In2O3-based films as the electron transport layer through a room-temperature solution-processing technique for high-performance flexible perovskite solar cells. There are some reports available in the literature related to the device design and architecture flexibility of perovskites and flexible perovskite solar cells,71–79 but any report on the recent advancement in metal oxide-based flexible perovskite solar cells is not available yet; consequently, herein, we summarized the work based on efficient metal oxides flexible perovskite solar cells.
The foremost effort was made in 2013 to apply perovskites as an absorber layer in the flexible PSCs, where the primary efficiency was about 2.6%.56 However, the poor long-term stability and relatively low performance are imperative aspects preventing the development of flexible PSCs. As compared to the rigid perovskite solar cell developed on a glass substrate, each layer that makes up the complete flexible PSC device has some special requirements, as stated earlier in Section 4. As stated previously, a rapid increase in the efficiency for flexible perovskite solar cells has been attained in the last decade or so owing to outstanding photophysical and opto-electronic properties of the perovskites and to experiences obtained from OSCs and DSSCs. The flexible perovskite solar cell having a triple-cation configuration demonstrated an efficiency of 18.6%, when stable metal oxides were combined within an inverted device structure, while the efficiency was extremely stabilized at 17.7%.80 The flexible perovskite solar cell achieved a validated efficiency of 17.3% over the highly crystalline oxide-based transporting layer, whereas the flexible all-perovskites tandem photovoltaic systems showed an efficiency of 21.3%.81,82 The flexible PSCs fabricated on the PFN substrates showed a thousand times bending sturdiness and greater than 86% of the alleviated power output is reserved after aging of more than 1000 hours.83 The device made on a polyethylene terephthalate-based substrate demonstrated an excellent sturdiness and retained about 95% of its primary/initial efficacy after 5000 times of cyclic bending.84
The efficiencies of metal oxide-based PSCs has significantly increased to 25.7% (Fig. 8) at the laboratory scale in 2022 due to the several developments in this field, including altering perovskite characteristics through interface engineering, chemical composition, developing efficient growth methods, and hole transport materials optimization. The promising photophysical and optoelectrical characteristics of perovskite materials such as their high absorption coefficient, increased electron–hole mobility, great life span, and long diffusion-span of the transporting carriers largely contribute to their high efficiency.85 In addition, the organic–inorganic halide perovskite materials can produce weakly bonded excitons with low bonding energies, encouraging free-moving carriers with weak electromotive forces within the perovskite material.86 Therefore, by combining the designs and advancements of the perovskite materials, the rapid augmentation can actually be close to the potential efficacy limit.87–90
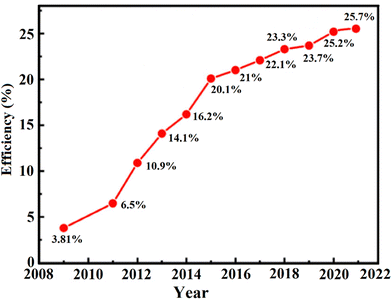 |
| Fig. 8 The efficiency progress with time for perovskite solar cells based on different metal oxide nanostructures. | |
The durability of PSCs can be controlled by a number of factors, including the unpredictability of halide-perovskites, interfaces, and charge-transporting layers (ETLs and HTLs). White LEDs or solar simulators with UV light filters are always used to test the stability of PSCs. The development of amalgamated or double-layered ETLs is considered as a key technique to enhance the power conversion efficiencies. Perovskite solar cells are therefore attracting a lot of interest as next-generation, highly efficient solar cell technologies in the pursuit of achieving affordable, usable energy stock in the future, while also increasing our ability to live sustainably. There are surface non-radiative recombination losses at the interface between metal oxides and perovskite layers, which may reduce the fill factor and Voc of the device, and can consequently decrease the device performance. Recent different approaches have been studied to eliminate recombination loss.91–93 Different low-temperature processed ternary oxide-based carrier transport layers have been reported because these low-temperature processed ternary oxide-based carrier transport layers offer some advantages of turnability of the band structure and electrical properties for preferring better band alignment and carrier extraction with different metal oxides-based stable perovskites.28,94,95 The preparation of the SnO2 film surface at a low-temperature by interface engineering using oxygen plasma treatment advances the efficiency of flexible perovskite solar cells since oxygen plasma recovers the conversion of the precursor-to-metal oxides layer.96
Thus, flexible perovskite solar cells have fascinated widespread research with notable advancements in the previous years. The performance of flexible PSCs has surpassed 20%, demonstrating a optimistic future of it. Notwithstanding the prominent enhancement in the performance and flexibility of flexible PSCs, manufacturing via an affordable and standard fabrication method is still stimulating. Nevertheless, presently, there are numerous problems that exist that hamper the commercialization of PSCs, mainly their hysteresis, reproducibility, durability, and stability under ambient conditions. These problems can be minimized by applying polymers-based interfacial layers or low-priced carbon and organic composite materials. Since the perovskite layer also plays a serious part in determining the performance of flexible PSCs, a high-quality perovskite layer having smooth surface morphology and vastly orientated crystalline structure, fully-exposed along with an appropriate bandgap is very important to develop a high efficient flexible PSCs. Furthermore, various studies have explored scalable and continuous roll-to-roll procedures, with the aim of mass production and commercialization of flexible perovskite solar cells in the last three years.97–100 The roll-to-roll manufacturing method is one of the most hopeful tactics to understand its marketable applications, while the in-depth investigation should also be thoroughly directed to speed up the development. Certainly, through the efforts of many scientists, the performance of flexible PSCs will be greatly improved in the near future. Seeing the progress in previous years, it is judicious to trust that the application of flexible perovskite solar cells has a bright future owing to its low-priced immense engineering and long-term stability.
6. Conclusion and future prospect
Excellent research has been done on the impact of metal oxide-based nanostructures on a wide range of applications linked to power conversion, particularly flexible solar cells, which have garnered a lot of attention owing to their suitability for roll-to-roll fabrication techniques and tremendous promise for practical applications in light, wearable, and portable electronic devices. The use of perovskites in flexible solar cells is fundamentally supported by the low-cost and wide availability of the precursor materials for perovskites as well as the straightforward synthesis of the thin films using low-temperature processes. Categorically, uniform shape, full coverage, a well-oriented crystalline structure, and an appropriate band gap are characteristics of perovskite thin films of excellent quality that are necessary for high-efficiency flexible PSCs. Several scientists are interested in using metal oxide-based nanostructures because of their affordable, environment friendly, and practical qualities. In order to use diverse metal-oxide nanostructures (TiO2, SnO2, NiO, Al2O3, CuO, Cu2O, CeO2, etc.) for flexible PSC applications, we have seen an exponential surge in research activity. These metal oxide nanostructures could manifest in various shapes and sizes, which would affect how well the relevant devices behave. MONs have increased the efficacy of solar cells due to their high surface area to volume ratio, optical results, favorable electron transporting capabilities, confinement effect, and formations of microstructural properties.101 The development of novel amalgamation techniques is crucial for boosting the properties of MONs. Perovskite materials have exceptional photophysical features such as high absorption coefficient, increased electron–hole mobility, excellent life-span, and long diffusion-span of the transporting carriers, which are responsible for their remarkable efficiency in solar cell applications. The metal oxide-based flexible PSCs showed remarkable desirability due to their unique advantages (viz., nearly impeccable crystalline behavior, lengthy diffusion of the transporting carriers, lower inherent loss and affordable, and relaxed fabrication approaches) over competing solar cell technologies. Thus, metal oxides flexible perovskite solar cells are attracting a lot of interest as next-generation high-efficiency solar cell technologies in the pursuit of achieving affordable usable power stock to progress our environmental viability in the near future. However, the commercialization of flexible PSCs is currently hampered by a number of issues, most notably, their hysteresis, repeatability, durability, and stability under ambient conditions. On the other hand, perovskites also have a few unavoidable disadvantages due to their extreme sensitivity to oxygen and water vapor, which can cause the materials to deliquesce and lose their crystalline structure, resulting in the long-term stability of PSCs being compromised. Thus, the growth of the perovskites layer ought to be executed in an inactive atmosphere. The introduction of A-site cations and X-site halide anions is one of the utmost encouraging ways to address the degradation and stability difficulties in PSCs. Few chemical-configuration manufacturing or amalgamation procedures have been used to develop lead-free perovskite PSCs for the solar cell industry, which could have significant advantages for both manufacturing hubs and academic institutions. Because of the advantages and disadvantages as described above, there are a few novel strategies that can be ingenious to improve the performance of flexible PSCs such as tuning the bandgap of perovskites, using high mobility and less expensive hole-transporting materials, improving the quality of the metal-contact layers, and designing a tandem solar cell device structure combining the PSC and the Si/CIGS/CZTSSe. These novel strategies can further increase the efficacy to 29.6%.
The mass manufacture of highly effective solar cells at a somewhat low temperature would be made possible by flexible perovskite-based technologies, which would significantly lower the cost of production. Due to the demands of the moderate growth processes, this photovoltaic technology may now be used to build high-throughput devices. It is anticipated that these new-generation PSCs will find wide applications and eventually result in photovoltaic products that compete with conventional Si-based solar cells. Thus, more effort is necessary to develop flexible electrodes with good electrical conductivity and light transmission for effective flexible PSCs and to bridge the gap between flexible and rigid/standard devices. Also, almost all reported flexible PSCs have small active areas of the concerned devices. It is generally known that the efficiency decreases when the device area grows to a large scale because the films inevitably lose their uniformity. Therefore, the performance of the large-area flexible PSCs is directly dependent on the deposition procedure for the thin films at a large scale. As a result, procedures for fabricating large surface areas are required for all of the layers in flexible PSCs. In order to further reduce the cost of manufacturing, the alternating vacuum deposition method should be included into the roll-to-roll system, which should presumably encourage the development of practical applications. In flexible perovskite solar cells, good encapsulation is more feasible and can give decent efficiency while reducing lead leakage in addition to the pursuit of lead-free devices. Further advancement and encouraging research on flexible PSCs are currently underway,102 and it is supposed that highly effective metal oxides will make flexible perovskite solar cells more commercially sustainable.
Conflicts of interest
There are no conflicts to declare.
Acknowledgements
One of the authors (Subhash Chander) would like to acknowledge the University Grants Commission, New Delhi for financial support through Dr D. S. Kothari Postdoctoral Fellowship (PH/19-20/0089).
References
- International Energy Agency, Report on ‘Key World Energy Statistics 2021’, Vienna, Austria.
-
N. Sönnichsen, Global primary energy consumption 2021, New York, USA.
- Annual Energy Outlook, US Energy Information Administration (EIA), Washington, DC, USA, 2022.
- S. Imperatives, Our Common Future: Report of the World Commission on Environment and Development, 2021, United Nations, Midtown Manhattan, New York City, USA.
- L. V. Alexander, X. Zhang, T. C. Peterson, J. Caesar, B. Gleason, A. M. G. K. Tank, M. Haylock, D. Collins, B. Trewin, F. Rahimzadeh, A. Tagipour, K. R. Kumar, J. Revadekar, G. Griffiths, L. Vincent, D. B. Stephenson, J. Burn, E. Aguilar, M. Brunet, M. Taylor, M. New, P. Zhai, M. Rusticucci and J. L. Vazquez-Aguirre, Global observed changes in daily climate extremes of temperature and precipitation, J. Geophys. Res. Atmos., 2006, 111, D05109 Search PubMed.
-
S. Chander, Advancement in CdIn2Se4/CdTe based photoelectrochemical solar cells, in Advances in Energy Materials. Advances in Material Research and Technology, ed. S. Ikhmayies, 2020, Springer Nature, Switzerland Search PubMed.
- M. A. Green, E. D. Dunlop, J. Hohl-Ebinger, M. Yoshita, N. Kopidakis, K. Bothe, D. Hinken, M. Rauer and X. Hao, Solar cell efficiency tables (Version 60), Prog. Photovoltaics, 2022, 30, 687–701 Search PubMed.
- S. Chander and M. S. Dhaka, Time evolution to CdCl2 treatment on Cd-based solar cell devices fabricated by vapor evaporation, Sol. Energy, 2017, 150, 577–583 CrossRef CAS.
- S. Chander, A. K. De and M. S. Dhaka, Towards CdZnTe solar cells: An evolution to post-treatment annealing atmosphere, Sol. Energy, 2018, 174, 757–761 CrossRef CAS.
- N. A. Rahman, S. A. Hanifah, N. N. Mobarak, A. Ahmad, N. A. Ludin, F. Bella and M. S. Suait, Chitosan as a paradigm for biopolymer electrolytes in solid-state dye-sensitised solar cells, Polymer, 2021, 230, 124092 CrossRef CAS.
- L. Fagiolari, E. Varaia, N. Mariotti, M. Bonomo, C. Barolo and F. Bella, Poly(3,4-ethylenedioxythiophene) in dye-sensitized solar cells: toward solid-state and platinum-free photovoltaics, Adv. Sustainable Syst., 2021, 5, 2100025 CrossRef CAS.
- J. C. Haro, E. Tatsi, L. Fagiolari, M. Bonomo, C. Barolo, S. Turri, F. Bella and G. Griffini, Lignin-based polymer electrolyte membranes for sustainable aqueous dye-sensitized solar cells, ACS Sustainable Chem. Eng., 2021, 9, 8550–8560 CrossRef PubMed.
- J. Bi, J. Zhang, P. Giannakou, T. Wickramanayake, X. Yao, M. Wang, X. Liu, M. Shkunov, W. Zhang and Y. Zhao, A highly integrated flexible photo-rechargeable system based on stable ultrahigh-rate quasi-solid-state zinc-ion micro-batteries and perovskite solar cells, Energy Storage Mater., 2022, 51, 239–248 CrossRef.
- T. M. W. J. Bandara, J. M. C. Hansadi and F. Bella, A review of textile dye-sensitized solar cells for wearable electronics, Ionics, 2022, 28, 2563–2583 CrossRef CAS.
- Z. Cai, X. Ma, J. Cai, Z. Zhan, D. Lin, K. Chen, P. Liu and W. Xie, Suppressing interface charge recombination for efficient integrated perovskite/organic bulk-heterojunction solar cells, J. Power Sources, 2022, 541, 231665 CrossRef CAS.
- L. Lavagna, G. Syrrokostas, L. Fagiolari, J. Amici, C. Francia, S. Bodoardo, G. Leftheriotis and F. Bella, Platinum-free photoelectrochromic devices working with copper-based electrolytes for ultrastable smart windows, J. Mater. Chem. A, 2021, 9, 19687–19691 RSC.
- L. Fagiolari, M. Sampò, A. Lamberti, J. Amici, C. Francia, S. Bodoardo and F. Bella, Integrated energy conversion and storage devices: Interfacing solar cells, batteries and supercapacitors, Energy Storage Mater., 2022, 51, 400–434 CrossRef.
-
S. B. Ogale, T. V. Venkatesan and M. Blamire, Functional Metal Oxides: New Science and Novel Applications, 2013, Wiley-VCH, Singapore Search PubMed.
-
M. Katukuri, Quantum chemical approach to spin-orbit excitations and magnetic interactions in iridium oxides, PhD thesis, Technische Universität Dresden, 2014.
- N. K. Elumalai, C. Vijila, R. Jose, A. Uddin and S. Ramakrishna, Metal oxide semiconducting interfacial layers for photovoltaic and photocatalytic applications, Mater. Renew. Sustain. Energy, 2015, 4, 11 CrossRef.
- R. Verma, J. Gangwar and A. K. Srivastava, Multiphase TiO2 nanostructures: a review ofefficient synthesis, growth mechanism, probing capabilities, and applications in bio-safety and health, RSC Adv., 2017, 7, 44199 RSC.
- M. S. S. Danish, A. Bhattacharya, D. Stepanova, A. Mikhaylov, M. L. Grilli, M. Khosravy and T. Senjyu, A Systematic Review of Metal Oxide Applications for Energy and Environmental Sustainability, Metals, 2020, 10, 1604 CrossRef CAS.
- S. F. Shaikh, B. G. Ghule, U. T. Nakate, P. V. Shinde, S. U. Ekar, C. O’Dwyer, K. H. Kim and R. S. Mane, Low-Temperature Ionic Layer Adsorption and Reaction Grown Anatase TiO2 Nanocrystalline Films for Efficient Perovskite Solar Cell and Gas Sensor Applications, Sci. Rep., 2018, 8, 11016 CrossRef PubMed.
- B. Yang, D. Ouyang, Z. Huang, X. Ren, H. Zhang and W. C. H. Choy, Multifunctional Synthesis Approach of In:CuCrO2 Nanoparticles for Hole Transport Layer in High-Performance Perovskite Solar Cells, Adv. Funct. Mater., 2019, 29, 1902600 CrossRef.
- D. Ouyang, J. Zheng, Z. Huang, L. Zhu and W. C. H. Choy, An efficacious multifunction codoping strategy on a room-temperature solution-processed hole transport layer for realizing high performance perovskite solar cells, J. Mater. Chem. A, 2021, 9, 371–379 RSC.
- H. S. Kim, J. W. Lee, N. Yantara, P. P. Boix, S. A. Kulkarni, S. Mhaisalkar, M. Grätzel and N. G. Park, High efficiency solid-state sensitized solar cell-based on submicrometer rutile TiO2 nanorod and CH3NH3PbI3 perovskite sensitizer, Nano Lett., 2013, 13, 2412–2417 CrossRef CAS PubMed.
- D. Ouyang, Z. Huang and W. C. H. Choy, Solution-Processed Metal Oxide Nanocrystals as Carrier Transport Layers in Organic and Perovskite Solar Cells, Adv. Function, Mater., 2018, 29, 1804660 Search PubMed.
- Z. Huang, D. Ouyang, C. J. Shih, B. Yang and W. C. H. Choy, Solution-Processed Ternary Oxides as Carrier Transport/Injection Layers in Optoelectronics, Adv. Energy Mater., 2019, 10, 1900903 CrossRef.
- S. Chander and M. S. Dhaka, Exploration of CdMnTe thin film solar cells, Sol. Energy, 2019, 183, 544–550 CrossRef CAS.
- Z. Yu, A. Hagfeldt and L. Sun, The application of transition metal complexes in hole-transporting layers for perovskite solar cells: Recent progress and future perspectives, Coord. Chem. Rev., 2020, 406, 213143 CrossRef CAS.
- B. K. Meyer, A. Polity, D. Reppin, M. Becker, P. Hering, P. J. Klar, T. Sander, C. Reindl, J. Benz, M. Eickhoff, C. Heiliger, M. Heinemann, J. Bläsing, A. Krost, S. Shokovets, C. Müller and C. Ronning, Binary copper oxide semiconductors: From materials towards devices, Phys. Status Solidi B, 2012, 249, 1487–1509 CrossRef CAS.
- M. S. S. Danish, L. L. Estrella, I. M. A. Alemaida, A. Lisin, N. Moiseev, M. Ahmadi, M. Nazari, M. Wali, H. Zaheb and T. Senjyu, Photocatalytic applications of metal oxides for sustainable environmental remediation, Metals, 2021, 11, 80 CrossRef CAS.
- K. Mahmood, S. Sarwar and M. T. Mehran, Current status of electron transport layers in perovskite solar cells: materials and properties, RSC Adv., 2017, 7, 17044–17062 RSC.
- J. Huang, Z. Yin and Q. Zheng, Applications of ZnO in organic and hybrid solar cells, Energy Environ. Sci., 2011, 4, 3861–3877 RSC.
- M. S. Ahmed, A. K. Pandey and N. A. Rahim, Advancements in the development of TiO2 photoanodes and its fabrication methods for dye sensitized solar cell (DSSC) applications: A review, Renewable Sustainable Energy Rev., 2017, 77, 89–108 CrossRef.
- T. Wong, S. Zhuk, S. Masudy-Panah and G. Dalapati, Current status and future prospects of copper oxide heterojunction solar cells, Materials, 2016, 9, 271 CrossRef PubMed.
- H. Wei, H. Gong, Y. Wang, X. Hu, L. Chen, H. Xu, P. Liu and B. Cao, Three kinds of Cu2O/ZnO heterostructure solar cells fabricated with electrochemical deposition and their structure-related photovoltaic properties, CrystEngComm, 2011, 13, 6065–6070 RSC.
- M. Abd-Ellah, J. P. Thomas, L. Zhang and K. T. Leung, Enhancement of solar cell performance of p-Cu2O/n-ZnO-nanotube and nanorod heterojunction devices, Sol. Energy Mater. Sol. Cells, 2016, 152, 87–93 CrossRef CAS.
- X. Chen, P. Lin, X. Yan, Z. Bai, H. Yuan, Y. Shen, Y. Liu, G. Zhang, Z. Zhang and Y. Zhang, Three-dimensional ordered ZnO/Cu2O nano-heterojunctions for efficient metal-oxide solar cells, ACS Appl. Mater. Interfaces, 2015, 7, 3216–3223 CrossRef CAS PubMed.
- S. H. Wee, P. S. Huang, J. K. Lee and A. Goyal, Heteroepitaxial Cu2O thin film solar cell on metallic substrates, Sci. Rep., 2015, 5, 16272 CrossRef CAS PubMed.
- M. Pławecki, E. Rówiński and Ł. Mieszczak, Zinc oxide/cuprous(I) oxide-based solar cells prepared by electrodeposition, Acta Phys. Pol., A, 2016, 130, 1144–1146 CrossRef.
- S. Chatterjee, S. K. Saha and A. J. Pal, Formation of all-oxide solar cells in atmospheric condition based on Cu2O thin-films grown through SILAR technique, Sol. Energy Mater. Sol. Cells, 2016, 147, 17–26 CrossRef CAS.
- T. Minami, Y. Nishi, T. Miyata and J. Nomoto, High-efficiency oxide solar cells with ZnO/Cu2O heterojunction fabricated on thermally oxidized Cu2O sheets, Appl. Phys. Exp., 2011, 4, 062301 CrossRef.
- K. Poorkazem, D. Liu and T. L. Kelly, Fatigue resistance of a flexible, efficient, and metal oxide-free perovskite solar cell, J. Mater. Chem. A, 2015, 3, 9241–9248 RSC.
- S. Masudy-Panah, G. K. Dalapati, K. Radhakrishnan, A. Kumar, H. R. Tan, N. K. Elumalai, C. Vijila, C. C. Tan and D. Chi, p-CuO/n-Si heterojunction solar cells with high open circuit voltage and photocurrent through interfacial engineering, Prog. Photovoltaics, 2015, 23, 637–645 CAS.
- M. Rokhmat and E. Wibowo, Sutisna, Khairurrijal and M. Abdullah, Performance improvement of TiO2/CuO solar cell by growing copper particle using fix current electroplating method, Procedia Eng., 2017, 170, 72–77 CrossRef CAS.
-
W. Guo, Z. Xu and T. Li, Metal-based semiconductor nanomaterials for thin-film solar cells, in Multifunctional Photocatalytic Materials for Energy, ed. Lin Z., Ye M. and Wang M., Woodhead Publishing, Cambridge, UK, 2018 Search PubMed.
- C. K. Møller, Crystal structure and photoconductivity of cesium plumbohalides, Nat., 1958, 182, 1436 CrossRef.
- D. Weber, CH3NH3PbX3, ein Pb(II)-System mit kubischer Perowskitstruktur/CH3NH3PbX3, a Pb(II)-system with cubic perovskite structure, Z. Naturforsch., B: Anorg. Chem., Org. Chem., 1978, 33, 1443–1445 CrossRef.
- J. Chen, S. Zhou, S. Jin, H. Li and T. Zhai, Crystal organometal halide perovskites with promising optoelectronic applications, J. Mater. Chem. C, 2016, 4, 11–27 RSC.
- R. Munir, A. D. Sheikh, M. Abdelsamie, H. Hu, L. Yu, K. Zhao, T. Kim, O. E. Tall, R. Li, D. M. Smilgies and A. Amassian, Hybrid perovskite thin film photovoltaics: in-situ diagnostics and importance of the precursor solvate phases, Adv. Mater., 2017, 29, 1604113 CrossRef PubMed.
- L. K. Ono, E. J. Juarez-Perez and Y. Qi, Progress on perovskite materials and solar cells with mixed cations and halide anions, ACS Appl. Mater. Interfaces, 2017, 9, 3019730246 CrossRef PubMed.
-
W. Wang and Z. Shao, Perovskite Materials in Photovoltaics, in Revolution of Perovskite, ed. Arul N. S. and Nithya V. D., Springer Nature, Singapore, 2020 Search PubMed.
- Energy Information Administrator, Report on ‘World Consumption of Primary Energy by Energy Type and Selected Country’, Washington, D.C., United States, 2006.
- H. J. Snaith, Perovskites: the emergence of a new era for low-cost, high-efficiency solar cells, J. Phys. Chem. Lett., 2013, 4, 3623–3630 CrossRef CAS.
- P. Docampo, J. M. Ball, M. Darwich, G. E. Eperon and H. J. Snaith, Efficient organometal trihalide perovskite planar-heterojunction solar cells on flexible polymer substrates, Nat. Commun., 2013, 4, 2761 CrossRef PubMed.
- H. Kim, K.-G. Lim and T.-W. Lee, Planar heterojunction organometal halide perovskite solar cells: roles of interfacial layers, Energy Environ. Sci., 2016, 9, 12–30 RSC.
- J. Dong, J. Wu, J. Jia, L. Fan, Y. Lin, J. Lin and M. Huang, Efficient perovskite solar cells employing a simply-processed CdS electron transport layer, J. Mater. Chem. C, 2017, 5, 10023 RSC.
- A. Kojima, K. Teshima, Y. Shirai and T. Miyasaka, Organometal halide perovskites as visible-light sensitizers for photovoltaic cells, J. Am. Chem. Soc., 2009, 131, 6050–6051 CrossRef CAS PubMed.
- J. H. Im, C. R. Lee, J. W. Lee, S. W. Park and N. G. Park, 6.5% efficient perovskite quantum-dot-sensitized solar cell, Nanoscale, 2011, 3, 4088–4093 RSC.
- H. S. Kim, C. R. Lee, J. H. Im, K. B. Lee, T. Moehl, A. Marchioro, S. J. Moon, R. Humphy-Baker, J. H. Yum, J. E. Moser, M. Grätzel and N. G. Park, Lead iodide perovskite sensitized all-solid-state submicron thin film mesoscopic solar cell with efficiency exceeding 9%, Sci. Rep., 2011, 2, 591 CrossRef PubMed.
- M. M. Lee, J. Teuscher, T. Miyasaka, T. N. Murakami and H. J. Snaith, Efficient hybrid solar cells based on meso-superstructured organometal halide perovskites, Sci., 2012, 338, 643–647 CrossRef CAS PubMed.
- W. J. Yin, J. H. Yang, J. Kang, Y. Yan and S. H. Wei, Halide perovskite materials for solar cells: a theoretical review, J. Mater. Chem. A, 2015, 3, 8926–8942 RSC.
- J. Burschka, N. Pellet, S. J. Moon, R. Humphry-Baker, P. Gao, M. K. Nazeeruddin and M. Grätzel, Sequential deposition as a route to high-performance perovskite-sensitized solar cells, Nature, 2013, 499, 316–319 CrossRef CAS PubMed.
- M. Liu, M. B. Johnston and H. J. Snaith, Efficient planar heterojunction perovskite solar cells by vapour deposition, Nature, 2013, 501, 395–398 CrossRef CAS PubMed.
- N. J. Jeon, J. H. Noh, Y. C. Kim, W. S. Yang, S. Ryu and S. I. Seok, Solvent engineering for high-performance inorganic-organic hybrid perovskite solar cells, Nat. Mater., 2014, 13, 897–903 CrossRef CAS PubMed.
- H. Zhang, J. Cheng, F. Lin, H. He, J. Mao, K. S. Wong, A. K. Y. Jen and W. C. H. Choy, Pinhole-Free and Surface-Nanostructured NiOx Film by Room-Temperature Solution Process for High-Performance Flexible Perovskite Solar Cells with Good Stability and Reproducibility, ACS Nano, 2016, 10, 1503–1511 CrossRef CAS PubMed.
- H. Zhang, J. Cheng, D. Li, F. Lin, J. Mao, C. Liang, A. K. Y. Jen, M. Grätzel and W. C. H. Choy, Toward All Room-Temperature, Solution-Processed, High-Performance Planar Perovskite Solar Cells: A New Scheme of Pyridine-Promoted Perovskite Formation, Adv. Mater., 2017, 29, 1604695 CrossRef PubMed.
- Y. Zhao, H. Zhang, X. Ren, H. L. Zhu, Z. Huang, F. Ye, D. Ouyang, K. W. Cheah, A. K. Y. Jen and W. C. H. Choy, Thick TiO2-Based Top Electron Transport Layer on Perovskite for Highly Efficient and Stable Solar Cells, ACS Energy Lett., 2018, 3, 2891–2898 CrossRef CAS.
- B. Yang, R. Ma, Z. Wang, D. Ouyang, Z. Huang, J. Lu, X. Duan, L. Yue, N. Xu and W. C. H. Choy, Efficient Gradient Potential Top Electron Transport Structures Achieved by Combining an Oxide Family for Inverted Perovskite Solar Cells with High Efficiency and Stability, ACS Appl. Mater. Interfaces, 2021, 13, 27179–27187 CrossRef CAS PubMed.
- D. Yang, Y. Yang, S. Priya and S. F. Liu, Recent Advances in Flexible Perovskite Solar Cells: Fabrication and Applications, Angew. Chem., Int. Ed., 2018, 58, 4466–4483 CrossRef PubMed.
- I. Hussain, P. H. Tran, J. Jeksik, J. Moore, N. Islam and M. J. Uddin, Functional materials, device architecture, and flexibility of perovskite solar cell, Emerg. Mater., 2018, 1, 133–154 CrossRef CAS.
- H. S. Jung, G. S. Han, N.-G. Park and M. J. Ko, Flexible Perovskite Solar Cells, Joule, 2019, 3, 1850–1880 CrossRef CAS.
- X. Liang, C. Ge, Q. Fang, W. Deng, S. Dey, H. Lin, Y. Zhang, X. Zhang, Q. Zhu and H. Hu, Flexible Perovskite Solar Cells: Progress and Prospects, Front. Mater., 2021, 8, 634363 Search PubMed.
- J. Tian and G. Cao, Design, fabrication and modification of metal oxide semiconductor for improving conversion efficiency of excitonic solar cells, Coord. Chem. Rev., 2016, 320–321, 193–215 CrossRef CAS.
- A. R. B. M. Yusoff, P. Gao and M. K. Nazeeruddin, Recent progress in organohalide lead perovskites for photovoltaic and optoelectronic applications, Coord. Chem. Rev., 2018, 373, 258–294 CrossRef.
- R. Zhou, Z. Yang, J. Xu and G. Cao, Synergistic combination of semiconductor quantum dots and organic-inorganic halide perovskites for hybrid solar cells, Coord. Chem. Rev., 2018, 374, 279–313 CrossRef CAS.
- A. E. Shalan, Challenges and approaches towards upscaling the assembly of hybrid perovskite solar cells, Mater. Adv., 2021, 1, 292–309 RSC.
- P. Mahajan, R. Datt, W. S. Tsoi, V. Gupta, A. Tomar and S. Arya, Recent progress, fabrication challenges and stability issues of lead-free tin-based perovskite thin films in the field of photovoltaics, Coord. Chem. Rev., 2021, 429, 213633 CrossRef CAS.
- M. Najafi, F. D. Giacomo, D. Zhang, S. Shanmugam, A. Senes, W. Verhees, A. Hadipour, Y. Galagan, A. Aernouts, S. Veenstra and R. Andriessen, Highly efficient and stable flexible perovskite solar cells with metal oxides nanoparticle charge extraction layers, Small, 2018, 14, 1702775 CrossRef PubMed.
- C. Liu, L. Zhang, X. Zhou, J. Gao, W. Chen, X. Wang and B. Xu, Hydrothermally treated SnO2 as the electron transport layer in high-efficiency flexible perovskite solar cells with a certificated efficiency of 17.3%, Adv. Funct. Mater., 2019, 29, 1807604 CrossRef CAS.
- A. F. Palmstrom, G. E. Eperon, T. Leijtens, R. Prasanna, S. N. Habisreutinger, W. Nemeth, E. A. Gaulding, S. P. Dunfield, M. Reese, S. Nanayakkara, T. Moot, J. Werner, J. Liu, B. To, S. T. Christensen, M. D. McGehee, M. F. A. M. van Hest, J. M. Luther, J. J. Berry and D. T. Moore, Enabling flexible all-perovskite tandem solar cells, Joule, 2019, 3, 2193–2204 CrossRef CAS.
- B. J. Kim, D. H. Kim, Y.-Y. Lee, H.-W. Shin, G. S. Han, J. S. Hong, K. Mahmood, T. K. Ahn, Y.-C. Joo, K. S. Hong, N.-G. Park, S. Lee and H. S. Jung, Highly efficient and bending durable perovskite solar cells: Toward a wearable power source, Energy Environ. Sci., 2015, 8, 916–921 RSC.
- Y. Li, L. Meng, Y. M. Yang, G. Xu, Z. Hong, Q. Chen, J. You, G. Li, Y. Yang and Y. Li, High-efficiency robust perovskite solar cells on ultrathin flexible substrates, Nat. Commun., 2016, 7, 1–10 Search PubMed.
- S. M. Vorpahl, S. D. Stranks, H. Nagaoka, G. E. Eperon, M. E. Ziffer, H. J. Snaith and D. S. Ginger, Impact of microstructure on local carrier lifetime in perovskite solar cells, Science, 2015, 348, 683–686 CrossRef PubMed.
- V. D’Innocenzo, G. Grancini, M. J. Alcocer, A. R. S. Kandada, S. D. Stranks, M. M. Lee, G. Lanzani, H. J. Snaith and A. Petrozza, Excitons versus free charges in organo-lead tri-halide perovskites, Nat. Commun., 2014, 5, 3586 CrossRef PubMed.
- A. Kojima, K. Teshima, Y. Shirai and T. Miyasaka, Organometal halide perovskites as visible-light sensitizers for photovoltaic cells, J. Am. Chem. Soc., 2009, 131, 6050–6051 CrossRef CAS PubMed.
- H. Zhou, Q. Chen, G. Li, S. Luo, T. B. Song, H. S. Duan, Z. Hong, J. B. You, Y. Liu and Y. Yang, Interface engineering of highly efficient perovskite solar cells, Science, 2014, 345, 542–546 CrossRef CAS PubMed.
- B. V. Lotsch, New light on an old story: perovskites go solar, Angew. Chem., Int. Ed., 2014, 53, 635–637 CrossRef CAS PubMed.
- C. Zuo, H. J. Bolink, H. Han, J. Huang, D. Cahen and L. Ding, Advances in perovskite solar cells, Adv. Sci., 2016, 3, 1500324 CrossRef PubMed.
- Z. W. Gao, Y. Wang, D. Ouyang, H. Liu, Z. Huang, J. Kim and W. C. H. Choy, Triple Interface Passivation Strategy-Enabled Efficient and Stable Inverted Perovskite Solar Cells, Small Methods, 2020, 2020, 2000478 CrossRef.
- R. Ma, Z. Ren, C. Li, Y. Wang, Z. Huang, Y. Zhao, T. Yang, Y. Liang, X. W. Sun and W. C. H. Choy, Establishing Multifunctional Interface Layer of Perovskite Ligand Modified Lead Sulfide Quantum Dots for Improving the Performance and Stability of Perovskite Solar Cells, Small, 2020, 2020, 2002628 CrossRef PubMed.
- R. Ma, J. Zheng, Y. Tian, C. Li, B. Lyu, L. Lu, Z. Su, L. Chen, X. Gao, J. X. Tang and W. C. H. Choy, Self-Polymerization of Monomer and Induced Interactions with Perovskite for Highly Performed and Stable Perovskite
Solar Cells, Adv. Funct. Mater., 2021, 2021, 2105290 Search PubMed.
- Z. Huang, D. Ouyang, R. Ma, W. Wu, V. A. L. Roy and W. C. H. Choy, A General Method: Designing a Hypocrystalline Hydroxide Intermediate to Achieve Ultrasmall and Well-Dispersed Ternary Metal Oxide for Efficient Photovoltaic Devices, Adv. Funct. Mater., 2021, 29, 1904684 CrossRef.
- J. X. Song, X. X. Yin, Z. F. Li and Y. W. Li, Low-temperature-processed metal oxide electron transport layers for efficient planar flexible perovskite solar cells, Rare Met., 2021, 40, 2730–2746 CrossRef CAS.
- A. P. Muthukrishnan, J. Lee, J. Kim, C. S. Kim and S. Jo, Low-temperature solution-processed SnO2 electron transport layer modified by oxygen plasma for planar flexible perovskite solar cells, RSC Adv., 2022, 12, 4883–4890 RSC.
- G. S. Han, H. S. Jung and N. G. Park, Recent cutting-edge strategies for flexible perovskite solar cells toward commercialization, Chem. Commun., 2021, 57, 11604–11612 RSC.
- G. Tang and F. Yan, Flexible perovskite solar cells: Materials and devices, J. Semicond., 2021, 42, 101606 CrossRef CAS.
- X. Zhang, Y. Ma, X. Chen, X. Li, W. Zhou, N. A. N. Ouedraogo, Y. Shirai, Y. Zhang and H. Yan, Improved efficiency and stability of flexible perovskite solar cells by a new spacer cation additive, RSC Adv., 2021, 11, 33637–33645 RSC.
- X. Liang, C. Ge, Q. Fang, W. Deng, S. Dey, H. Lin, Y. Zhang, X. Zhang, Q. Zhu and H. Hu, Flexible perovskite solar cells: Progress and Prospects, Front. Mater., 2021, 8, 634353 CrossRef.
- Z. Sun, T. Liao and L. Kou, Strategies for designing metal oxide nanostructures, Sci. China Mater., 2017, 60, 1–24 CrossRef CAS.
- Y. Xu, Z. Lin, W. Wei, Y. Hao, S. Liu, J. Ouyang and J. Chang, Recent progress of electrode materials for flexible perovskite solar cells, Nano-Micro Lett., 2022, 14, 117 CrossRef CAS PubMed.
|
This journal is © The Royal Society of Chemistry 2022 |
Click here to see how this site uses Cookies. View our privacy policy here.