DOI:
10.1039/D2IM00062H
(Review Article)
Ind. Chem. Mater., 2023,
1, 224-239
Ionic skin: from imitating natural skin to beyond
Received
19th December 2022
, Accepted 24th February 2023
First published on 28th February 2023
Abstract
Ionic skin, as an emerging subclass of artificial skin, has been proposed and developed for nearly a decade, which makes up for the partial shortcomings of electronic skin to some extent. Highly similar to the ion-sensing mechanism of natural skin, the ionic skin also acquires and conducts perceptual signals in the form of ions. During this decade, a great deal of effort has been devoted to the species amplification of ionic soft matter and the discovery of new mechanisms of artificial ion sensing. It is worth emphasizing that the deciphering of the perceptual mechanisms of natural skin has inspired a great number of bionic studies in pursuit of the reproduction of natural touch in ionic skin. Ionic skin, as a multi-functional operating platform, is also endowed with attractive functions that are beyond natural skin. The birth and appearance of ionic skin greatly promote the vigorous development of products in the era of the internet of things, such as human-machine interaction, prosthetics and wearable devices. In this review, on the basis of explaining the perceptual mechanism of natural skin, we deeply analyze the progressive sensing mechanism of bionic ionic skin. The typical cases of ionic skin that are beyond the ability of natural skin are also summarized in detail. Finally, constructive perspectives and common issues are presented for the future development of ionic skin.
Keywords: Ionic skin; Tactile perception; Bionic; Flexible sensor.
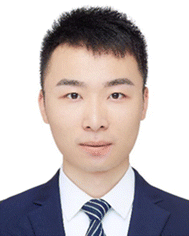 Zhiwu Chen | Zhiwu Chen received his B.E. degree from the Beijing University of Chemical Technology in 2017 and his M.E. degree from the Beijing University of Chemical Technology in 2020. He is currently a Ph.D. student under the supervision of Prof. Yapei Wang at the Department of Chemistry, Renmin University of China. His Ph.D. research is focused on dynamic ionic liquids. |
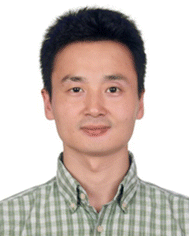 Yapei Wang | Yapei Wang is a professor in the Department of Chemistry at Renmin University of China. He earned his B.S. degree from Jilin University in 2004 and his Ph.D. degree from Tsinghua University in 2009. He finished his 2 year postdoctoral research at the University of North Carolina at Chapel Hill in 2011. Then he joined the Renmin University of China in 2012 and was promoted to full professor in 2015. His research interests lie in complex emulsions, photothermal conversion, and bioelectronic devices for clinical diagnosis. |
1 Introduction
As the largest human organ, the skin is the vital physical barrier for maintaining survival, and it is regarded as a multifunctional platform for the communication of information and substance exchange between the body and surroundings.1–3 The perception ability of skin originates from the delicate array of receptors that can discriminately convert external stimuli (such as tension, pressure and temperature) into bioelectrical signals available to the brain, which in turn innervate the body to respond to these external stimuli in a timely manner.4,5 Skin also serves as a physical barrier against pathogenic bacteria to stabilize the internal environment of humans. In the past decades, the booming era of the internet of things has spawned artificial skin as an alternative to natural skin. The ultimate goal of artificial skin development is to reproduce, strengthen and expand the function similar to natural skin. The emergence of these artificial products has gradually satisfied our imagination of this fascinating technology. More practically, artificial skin has shown great promise in wearable devices, prosthetics, medical treatment and health monitoring.6
As the first subclass of artificial skin, electronic skin, which utilizes the transfer of electrons or holes to conduct electrical signals, has made rapid development. Although electronic skin based on electronic conductors can meet the essential requirements of electrical conductivity and flexibility, it is limited in its ability to meet additional requirements in specific applications, such as biocompatibility, rapid self-healing and transparency.7,8 Since Whitesides and Suo first proposed the concept of ionic skin in 2014, the development of artificial skin has ushered in a new development opportunity.8 After nearly a decade of development, numerous novel materials and scientific phenomena have emerged in this field. Abundant ion species, such as inorganic salts, ionic liquids, dynamic ionic liquids, and ionic deep eutectic solvents, have been integrated into the soft matter.9–14 These examples of rich and expansive ionic soft matter have also promoted the diversification of ionic skin types, which have gradually expanded from the initial ionic hydrogels to ionic organic gels. Many new academic terms have emerged in the field of ion sensing, such as “ionic conductors”, “iontronics”, and “ionogels”. However, considering the similarity of their properties and functions, flexible devices based on them can all be assigned to the ionic skin. Thanks to this treasure trove of ionic soft matter, ionic skin with excellent self-healing, biocompatibility and transparency can be readily obtained by selecting applicable formulas of ionic soft matter.8,15 More importantly, the relatively mature development of resistive, capacitive, thermoelectric and triboelectric sensing mechanisms involved in ion sensing has also endowed ionic skin with the essential perception ability, which can respond to external temperature, tension and pressure stimulation in the form of electrical signals.
Particularly, the increasing clarity of the molecular mechanisms of natural skin perception, especially the case where the research on touch and temperature receptors won the 2021 Nobel Prize in Physiology or Medicine, has encouraged and inspired researchers in the field of artificial skin and prompted them to seek bionic strategies to achieve the artificial reproduction of natural touch. Similar to natural skin that uses ions to transmit signals, ionic skin using ions as a sensing medium is considered to be the most promising sensing platform for communication with living organisms.16 Moreover, ions, especially designable organic ions, are more likely to be manipulated. Therefore, many researchers are obsessed with the manipulation of ions to achieve a similar perception ability or even beyond that of natural skin. In addition to recreating the tactile perception of natural skin, the emerging ionic skin has expanding functions that are beyond natural skin to adapt to rigorous and diverse practical applications. On the one hand, visual perception and noncontact perception are incorporated into the ionic skin system, and adaptive ionic skin in extreme cold and underwater environments is gradually emerging. On the other hand, more additional functions have been creatively proposed, which greatly strengthen the application connotation of ionic skin.
This review introduces the emerging phenomena and strategies of ionic sensing that exclusively belong to ionic skin from the aspect of bionic tactile perception. Meanwhile, combined with the leading role played by ions, the functions of ionic skin that are beyond natural skin will be elaborated on. Finally, constructive perspectives and key issues that need to be addressed will be presented. We hope that this review will be conducive to the researchers in this field grasping the development and trends of ionic skin.
2 Bio-receptor-inspired tactile sensing
Information interaction with the outside world is the basic physiological function of humans to maintain survival. These acquired sensations can be collectively referred to as somatosensory, which are colloquially known as touch. It consists of four main categories: touch, proprioception (the perception of mechanical displacement of muscle and joints), thermo-sensation, and nociception (the perception of harmful mechanical, thermal, or chemical stimuli that cause pain).5 Skin, as the largest organ in the human body, can be considered as a window for touch and temperature perception. Temperature receptors and mechanoreceptors that are expressed in specific cells are key targets that dominate the acquisition of these perceptions (Fig. 1a). Patapoutian and Julius discovered and revealed the working mechanism of touch and temperature receptors, for which they were awarded the 2021 Nobel Prize in Physiology or Medicine.4 They found that the nature of these receptors is typical of ion channel proteins. Both PIEZO1 and PIEZO2 ion channel proteins are responsible for the sense of mechanical stimuli.4,17 The transient receptor potential (TRP) ion channel family are the molecular machines that detect temperature stimuli.4,5,18,19 When these channel proteins produce a conformational change under certain external stimuli, that is, the transition from the “closed state” to the “open state”, a large number of ions will flow in, and the corresponding action potential will be generated, and then the neurons will transmit this primary sensory information to the brain (Fig. 1b and c). Thanks to the presence of these ion channel proteins, humans can perceive and distinguish various types of stimuli accurately and in a timely manner, and then respond to them with matching interactions. The decryption of the molecular mechanism of the skin sensing system has also promoted the rapid development of artificial ionic skin. In recent years, some emerging ion sensing mechanisms have been extended from the imitation of the sensation function of natural skin, which give the ionic skin a tactile sensation similar to that of natural skin. This section will elaborate on the new ion sensing mechanism and material designs of these ionic skins from the perspective of bionics.
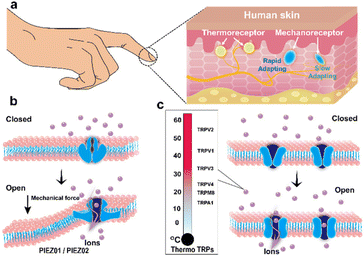 |
| Fig. 1 (a) Schematic illustration of the mechanoreceptors and thermo-receptors in human skin; (b) PIEZ01 and PIEZ02 are the specific ion channel proteins that govern mechanical force sensation. (c) A series of thermo-TRPs associated with temperature sensation. | |
2.1 Artificial mechanoreceptor
Generally, the mechanical force sensing mechanism of ionic skin mainly focuses on resistive, capacitive and triboelectric types. However, due to the inherent properties of traditional ion sensing models, it is still a challenge to give ionic skin a precise and wide range of force perception similar to that of natural skin. To this end, many researchers are looking to further grasp the sensing mechanism of natural mechanoreceptors. The natural skin's perception of mechanical forces is mainly controlled by PIEZO2 ion channel proteins distributed on the membrane of Merkel cells (Fig. 2a).20 The opening of the PIEZO2 pore channel, stimulated by mechanical forces, enables the inward flow of ions, which induces a transient change in the ion equilibrium inside and outside the cell membrane. During this process, the deformation behavior of the poroelastic membrane manipulates the migration of ions. Similar to the visco-poroelastic behavior of cell membranes, the intrinsic viscoelastic behavior of polymer structures can be used to regulate ion migration for the fabrication of artificial mechanoreceptors; i.e., the intrinsic free volume in the chain segment of the polymer can act as a nano-channel for ion storage. When the external force causes the deformation of the chain segment, the ions will be squeezed out from the free volume of the polymer matrix, resulting in a change in the ion concentration distribution.21–25 This novel phenomenon was discovered and studied by Kim and co-workers. This team designed and synthesized a viscoelastic capacitive ionic skin based on thermoplastic polyurethane (TPU) and ionic liquids ([EMIM][TFSI]).23 The ions squeezed out by the deformation of the chain segment strongly affected the electric double layer (EDL) at the electrode interface, causing a drastic change in capacitance (Fig. 2b). In order to enhance the sensitivity of force detection, ionic skins with micro-column arrays were prepared (Fig. 2c). This strategy improves the efficiency of mechano-transduction and produces the squeezing-out effect of ions even under weak pressure stimulation (Fig. 2d). The resulting ionic skin can accurately detect pressures ranging from 10 Pa to 100 kPa.
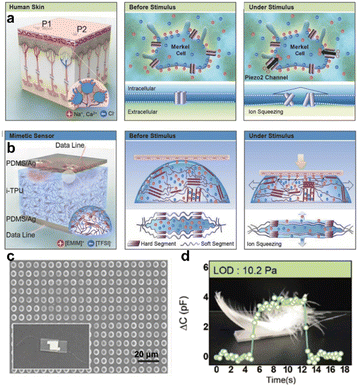 |
| Fig. 2 (a) PIEZO2 ion channel proteins distributed on Merkel cell membranes and the regulation of ion migration by the poroelastic behavior. (b) A visco-poroelastic elastomer based on TPU is used to regulate mechanically activated ion migration in capacitive ionic skin. (c) SEM image of the ionic skin with a columnar microstructure. (d) The precise perception of the weight of a feather by the ionic skin. Reproduced with permission from ref. 23, copyright 2017, Wiley-VCH. | |
For capacitive mechanoreceptors, optimizing the rate of changing the distance or the opposite area between two electrodes with the applied force to improve the sensitivity of the mechanoreceptor has reached a bottleneck. Expanding the rate of changing the number of charges induced on the electrode plates before and after force stimulation has become another alternative for the pursuit of ultra-sensitive capacitive mechanoreceptors, which is also an expected outcome inspired by the sensing mechanisms of the natural skin. With further reflection, we realize that the implementation of this strategy entirely depends on the elaborate control of the stimulated migration of ions in the bulk phase.26,27 Suggestive work led by Kim has demonstrated the powerful efficacy of regulating weak interactions in ion systems to enhance the sensitivity of artificial mechanoreceptors. Inspired by the cell structure, they designed a synthetic multicellular hybrid ion pump comprised of silica microspheres with ionic liquids confined on their surface, a TPU elastomeric matrix, silanol groups, which are analogous to cells, extracellular matrix, and conductive integrins (Fig. 3a).28 The dynamic confinement interface formed by hydrogen bonding and electrostatic interactions between the ionic liquid and the surface of the silica microsphere is the key to conferring ultra-sensitivity on this artificial mechanoreceptor (Fig. 3b). Under the stimulation of an external force, these mentioned supramolecular interactions at the interface are weakened so that ions are pumped out from the interface, which induces more charges at the electrode, resulting in a sharp increase in the capacitance change rate before and after the stimulus.
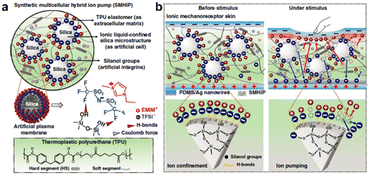 |
| Fig. 3 (a) A schematic diagram of the overall structure of a synthetic multicellular hybrid ion pump. (b) The force-induced breakdown of the hydrogen bond causes ions to be pumped from the confinement interface. Reproduced with permission from ref. 28, copyright 2019, Nature Publishing Group. | |
In the case of the capacitive mechanoreceptor as mentioned above, the ion species, driven by an applied voltage, respond to the applied force with a greater proportion of ions migrating in reverse. The same amount of dissimilar charges would be generated at the two electrode plates to balance the voltage of the external circuit at the final equilibrium stage. We can assume that there is no difference in the migration process of the cation and anion in the selected ion species. However, due to the differences in the size of ion species and their interactions with the matrix, the ion species confined in the polymer network will inevitably have differences in the migration rates during the process of responding to external forces. This difference will expectedly result in an inhomogeneous distribution of ions in the bulk phase, which is reflected in the form of open circuit voltage and short circuit current in the external circuit. This phenomenon is known as the “piezoionic effect” (Fig. 4a).29 This term can be traced back to 2015, which was proposed by Madden et al. This phenomenon was observed as early as the 1990s in the polyaniline experiment, and Takashima et al. called this sensing process the “mechanochemoelectrical effect”.30 However, many subsequent studies on conductive polymers and ionic polymer metal composites have confirmed that such phenomena are highly related to the ionic redistribution in response to external forces.31–34 Because only a simple mechanical force input (pressing or bending) can induce the output of electrical signals (Fig. 4b), ionic skin based on the “piezoionic effect” has attracted much attention in the fields of human-computer interaction and intelligent sensors in recent years.35 In particular, the underlying mechanism of the “piezoionic effect” is highly similar to that of the ion concentration redistribution between the cell membrane of natural mechanoreceptors under external stimulation, which opens a promising path for the fabrication of biomimetic mechanoreceptors. Recently, Madden et al. explored in detail the molecular origin of pressure-induced voltage using ionic hydrogels as soft material templates, and prepared piezoionic mechanoreceptors that were highly similar to natural mechanoreceptors in terms of charge, voltage, and response time.6 They found that the induced peak voltage was related to three types of ion fluxes generated during the pressure process, namely diffusive fluxes, electrophoretic fluxes and convective fluxes, in which the diffusive and electrophoretic fluxes together made up the back current opposite to the convective current. The competition between the back current and convective current determined the appearance of the peak voltage, which was also the fundamental reason why the induced voltage was instantaneous. More interestingly, the resting potential and action potential could be constructed analogously to the natural nerve, and the muscle twitching of mice was successfully activated by this piezoionic mechanoreceptor. This is a successful example of information exchange between machinery and living bodies.
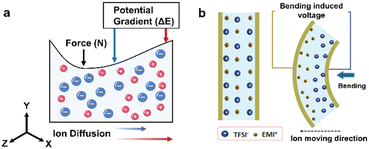 |
| Fig. 4 (a) Schematic diagram of the mechanism of the piezoionic effect. (b) Bending-induced inhomogeneous ion distribution. Reproduced with permission from ref. 35, copyright 2016, Wiley-VCH. | |
In addition to these emerging ion-sensing mechanisms that mimic stimulated ion migration and redistribution of natural mechanoreceptors, ionic skin is also struggling to reproduce the rapid and slow adaptations of natural skin to the perception of mechanical forces. Natural mechanoreceptors can be further subdivided into fast adaptive receptors (FA) and slow adaptive receptors (SA), which respond to dynamic, transient force stimuli and static, continuous force stimuli, respectively.36,37 Essentially, it is about the duration and signal pattern of the response of the receptors. Reproducing this adaptive feature requires that artificial receptors be able to produce a controlled response toward mechanical stimuli. In the research of Madden, it was found that the response duration of the “piezoionic effect” was closely related to the stress relaxation process of the ionic hydrogel.6 In the system with low solid content, there is greater permeability in the matrix, allowing ions to flow rapidly within the water-based pore structure, shortening the time to reach the peak voltage. At the same time, the time to complete the response decay is shorter. Therefore, the adaptive behavior of the piezoionic mechanoreceptors can be adjusted by changing the polymer solid content of the ionic hydrogel. Besides, due to the intrinsic differences in the duration and presentation of the sensing signals in different sensing modes (triboelectric, piezoelectric, piezoionic, resistive, capacitive), the fabrication of adaptive mechanoreceptors can also be achieved by integrating multiple sensing modes.37 Kim et al. combined the triboelectric effect with the piezoionic effect to achieve the dynamic switching of the ionic polarization state of the ionized gelatin.38 Upon contact with ionic skin, the triboelectric effect induces ion migration to the gel surface to balance the surface charge. When deformation occurs, the piezoionic effect dominates the redistribution of ions in the bulk phase, thus producing the opposite output signal (Fig. 5). Han et al. focused on the synergistic combination of the piezoelectric effect and artificial ion channel membrane (Fig. 6a).39 Through the charge polarization generated by the Au/PVDF piezoelectric effect film with a fast response similar to FA, a larger potential difference is formed at the interface between Au/PVDF film and polyaniline solution, which will promote ions passing through the porous film and generate a lasting voltage signal similar to SA (Fig. 6b). This artificial mechanoreceptor can effectively distinguish various types of touch and pressure and achieve the artificial reproduction of the functions of SA and FA.
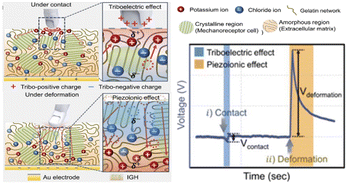 |
| Fig. 5 The piezoionic effect and the triboelectric effect are combined to produce an adaptive artificial mechanical perception. Reproduced with permission from ref. 38, copyright 2021, Wiley-VCH. | |
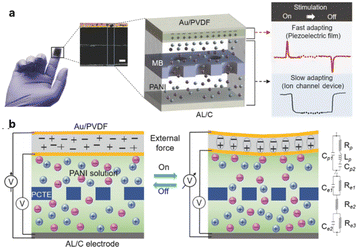 |
| Fig. 6 (a) An adaptive artificial mechanoreceptor based on an artificial ion channel system combined with a piezoelectric film. (b) A schematic diagram of the sensing mechanism of this adaptive mechanoreceptor. Reproduced with permission from ref. 39, copyright 2018, Wiley-VCH. | |
2.2 Artificial thermoreceptor
Broadly speaking, the sensor that can indicate temperature variations can be included in the artificial thermoreceptor. Its development has been relatively mature, and it has been widely used in daily life. However, for the development of artificial skin, ultra-sensitive temperature perception is an unavoidable basic function. In recent years, numerous flexible artificial skins have shown temperature response behavior, including the ionic skins mainly discussed in this review. The ionic soft matters that play the sensing function in the ionic skin have more outstanding sensing sensitivity than the traditional temperature response materials, especially the ionic liquids. Our research group and other researchers have done a lot of pioneering and fundamental research on the temperature-sensing mechanism of ionic liquids.40–44 Their temperature response behavior is mainly reflected in the changes in electrical signals (ionic conductivity, current and resistance) with temperature. In essence, the digital information obtained is also a reflection of the changes in ion migration in the system with temperature. For traditional ionic liquids, the electrostatic interaction between ions is the main interaction that controls the migration of ions. Therefore, the highly sensitive response characteristic of ionic liquids toward temperature is derived from the sensitivity of its own electrostatic interactions responding to heat, which is also reflected in the dramatic change in its viscosity and electrical signal with the change in temperature.45 It can be seen that regulating the migration of ions through heat is a feasible strategy for controlling the temperature response behavior of ions. This concept is similar to the sensing mechanism of natural thermoreceptors. The thermal perception of natural skin comes from TRP ion channels distributed in nerve endings that control the movement of ions across the cell membrane, and these ion channels have reversible heat-activation properties at specific temperatures. Analogous to the gating mechanism of TRP ion channels, reversible electrostatic interactions can be regarded as molecular gating controlling ion migration, which also has the characteristics of heat activation. In addition to electrostatic interactions, other thermal-sensitive interactions, including hydrogen bonds and dynamic covalent bonds, can also be selected to enrich this regulatory means.
To highlight the non-negligible role of dynamic interactions in enriching and developing the sensing functions of ionic liquids, we innovatively proposed the concept of dynamic ionic liquid.10,11,46 Meanwhile, inspired by the TRPV1 ion channel protein, the ability of dynamic covalent bonds with heat-activation properties to regulate the sensitivity of the ionic liquid temperature response was explored (Fig. 7a). We designed and synthesized a dynamic ionic liquid with a five-membered ring containing a disulfide bond, which has intrinsic spontaneous polymerization behavior. Due to the thermal sensitivity of the disulfide bond, this dynamic ionic liquid can be reversibly converted between the polymerized oligomers and free monomers (Fig. 7b). The heat-activated temperature of this reversible transformation was determined to be 45 °C, which is similar to the physiological noxious temperature. At the same time, this dynamic ionic liquid has an unprecedented intrinsic temperature response sensitivity of up to 156.79% °C−1, which is unmatched by traditional thermal-sensitive materials (Fig. 7c).11 Through energetics analysis, we also found that the temperature response sensitivity of the ionic liquids is positively correlated with activation energy for free ion migration. The activation energy is closely related to the proportion of confined ions in the system. This work not only attempts to fabricate artificial thermoreceptors with a similar ability to that of natural skin to distinguish between innocuous and noxious thermal stimuli, it also provides a feasible model for chemically regulating the thermal response behavior of ions.
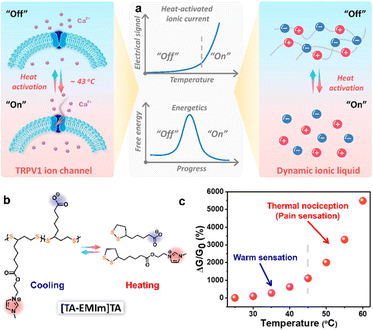 |
| Fig. 7 (a) Design concept of a TRPV1-like heat-activated dynamic ionic liquid; (b) the reversible conversion of the dynamic ionic liquid between the polymerized state and the monomorphic state; (c) the electrical response of [TA–EMIm]TA at different temperatures. Reproduced with permission from ref. 11, copyright 2022, American Chemical Society. | |
3 Visualization of perception
In addition to the response mode of neural electrical signals based on ion transport, certain organisms in nature have also evolved color-changeable skin in complex and diverse environments. These organisms use color changes to maintain their needs for essential survival functions, such as communication, camouflage, courtship, etc.47,48 Visual perception systems that mimic the color-variable mechanism of organisms have become another attractive aspect of the functional expansion of ionic skin. They not only engender the multi-signal response models of ionic skin but also, from the perspective of consumers, the sensing process with visual impact can bring users a higher level experience of human–machine interaction.49
In order to mimic the stimulation-responsive behavior of organisms with color-changing skin, their skin structures and sensing mechanisms need to be understood and have aroused extensive interest. In the case of chameleons, there are periodically arranged light-reflecting structures in their skin, forming what we call photonic crystals.50 A change in the lattice spacing or refractive index of a photonic crystal will affect its physical interaction with the incident light, which will appear as a change in the presented color. Therefore, the rational design of ionic skin with artificial photonic crystals is a feasible approach to visual perception, among which the most commonly adopted photonic crystals have the opal structure or anti-opal structure (Fig. 8a).49,51 The same effect can be achieved by adjusting the regular arrangement of the liquid crystal structure.47,52,53 For instance, He and Niu et al. constructed the lamellar liquid crystal structure in the aqueous phase through the self-assembly behavior of amphiphilic surfactants, and then fixed this regular structure into the ionic hydrogel network. According to the Bragg equation, the wavelength of reflected light is positively correlated with the lattice spacing at certain incident angles. Thus, the color of this ionic skin can respond to swelling, mechanical, and thermal stimuli that can cause changes in the lattice spacing (Fig. 8b).47 The change in turbidity caused by the LCST phase transition, which is also the result of the microstructure of the material physically interacting with incident light, can be deemed as another typical temperature-responsive medium for visualizing thermal perception.54,55 By confining tetrabutylphosphonium p-styrenesulfonate ([P4444][SS]) in a gelatin network, our research group prepared transparent and freestanding ionic hydrogels with LCST behavior, whose phase separation can occur at adjustable temperature thresholds and produce visual turbidity for the visualization of spatial thermal distribution (Fig. 9).55
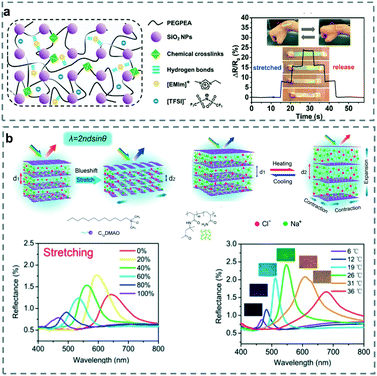 |
| Fig. 8 (a) Photonic ionic skin embedded with silica microspheres, and the electrical/optical responsive behavior. Reproduced with permission from ref. 49, copyright 2021, Wiley-VCH. (b) Photonic hydrogel structure based on a two-dimensional bilayer membrane and the mechanisms of optical changes by external stimulation. Reproduced with permission from ref. 47, copyright 2021, American Chemical Society. | |
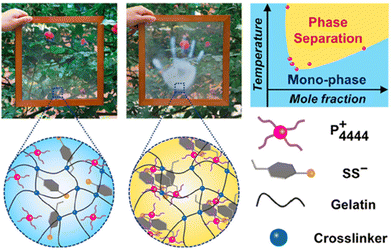 |
| Fig. 9 Ionic liquids with LCST behavior for the visualization of thermal perception. Reproduced with permission from ref. 55, copyright 2021, Cell Press. | |
Electroluminescence technology also allows us to engineer artificial chameleon skin for the visual perception of strain.56–61 The sandwich structure is a common choice for a working electroluminescence device, which consists of two electrode layers and a luminescent layer embedded with phosphor particles. Transparency is the basic requirement for the electrode layer to let light out. The stiffness and brittleness of the traditional transparent electrode (e.g., indium tin oxide) limit its application in skin-like functional devices. Ionic conductors with both transparency and stretchability are gradually considered the next generation of electrodes for flexible electroluminescence devices. However, high amplitude voltages are usually required to drive such devices to emit light. Therefore, the tolerance of their electrochemical windows to the applied voltage should be carefully considered. For the sandwich-structured electroluminescence devices, their structure can be understood as a series model of three capacitors (Fig. 10a), in which the dielectric capacitance of the emissive layer has a longer scale of charge separation, resulting in a small capacitance. The applied voltage is mainly distributed at the interface of the emissive layer, while the applied voltage at the interface of the ionic electrodes is insufficient to initiate electrolysis.57 Therefore, based on this working mechanism and distinctive attribute, various ionic conductors can satisfy the basic demands of flexible and stretchable electroluminescent devices for electrode materials, which can maintain a bright luminous state under strains (Fig. 10b). It should be pointed out that the increase in electric field intensity and the decrease in the areal number density of phosphor particles caused by material deformation will lead to the increase in luminescence intensity, which can be used to indicate different mechanical stimulation behaviors.48 In recent years, ionic electroluminescent fibers, fabrics, and other configurations have emerged, and their appearance has enriched and developed flexible ionotronic materials for visualized dynamic displays.59,62
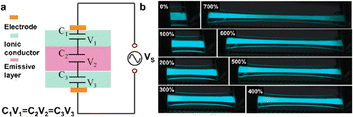 |
| Fig. 10 (a) Schematic diagram of an electroluminescent device with ionic conductors. (b) The luminescence states of electroluminescent devices under different strains. Reproduced with permission from ref. 57, copyright 2016, Wiley-VCH. | |
The operation voltage that drives phosphor-based electroluminescent devices is up to several thousand volts. The low operating voltage-controlled luminescence process is more adaptable to practical voltages. In nature, some organisms produce mechanically-stimulated luminescence, such as dinoflagellates in the ocean. Their luminescence process, known as ion-mediated chemical luminescence and electrochemical luminescence, can be performed at operating voltages below 1.0 V. Inspired by this natural phenomenon, Kim and Kang et al. transformed local force stimuli into spatially resolved optical responses by means of a low-voltage initiated electrochemical luminescence process.25 The specifically selected electrochemical luminescence reactions are shown in Fig. 11a, where tris(2,2′-bipyridine)ruthenium(II)hexafluorophosphate ([Ru(bpy)3][PF6]2) was selected as a luminophore that could undergo electrochemical reactions near the electrode plate interface to generate reduced species ([Ru(bpy)3]˙1+) and oxidized species ([Ru(bpy)3]˙3+). These two species collide to produce a luminescent excited state at low amplitude AC voltage. The intensity of the electrochemical luminescence is controlled by the ion redistribution caused by the piezoionic effect. In the process of applying force, the least mobile [Ru(bpy)3]2+ ions accumulate in the local area to produce a more differentiated light intensity response (Fig. 11b). Since this electrochemical luminescence reaction occurs near the electrode plate interface, it is the essential reason for pressure visualization to reversibly manipulate the concentration of luminescent ions near the electrode plate by mechanical force. More subtly, Moon et al. utilized the porous polymer skeleton to adsorb the ionic liquid mixed with [Ru(bpy)3][PF6]2. The existence of the porous structure made the direct contact area between the ionic gel and the electrode plate smaller, while the deformation of the flexible skeleton caused by the pressure increased the contact surface. The significant change in the contact area was accompanied by a change in the luminescent ion distribution on the electrode plate surface, which was reflected in a noticeable change in luminescence intensity against pressure (Fig. 11c).63
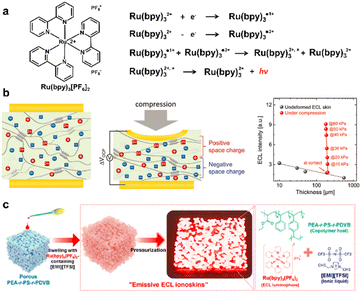 |
| Fig. 11 (a) The reaction process of the ionic electrochemical luminescence reaction. (b) The redistribution of luminescent ions and the change in the corresponding light intensity induced by the piezoionic effect. Reproduced with permission from ref. 25, copyright 2021, Wiley-VCH; (c) pressure visualization of porous ionic skin based on the electrochemical luminescence mentioned in Fig. 11a. Reproduced with permission from ref. 63, copyright 2021, American Chemical Society. | |
The appearance of ionic skin with visual perception capability has brought the products of science fiction into the real world. However, visual differences can only qualitatively reflect the external stimuli, and it is an inherent attribute that cannot be ignored to take accurate electrical signals as the presentation of perception. Therefore, it is a beneficial exploration and practice to integrate the dual modes of electrical signal response and visual response to strengthen the function of ionic skin.
4 Noncontact perception
As a sensing mode beyond the sensation of the human skin, noncontact perception has gradually attracted extensive attention due to growing demand. Especially in the era of the COVID-19 epidemic, contactless human-machine interaction can reduce the risk of contact cross-infection. The perception of noncontact is also an effective approach to advance warning, which will provide convenience for the blind to avoid some unnecessary dangerous contact in their daily life.64 Achieving noncontact perception requires that a surrounding object can remotely stimulate the sensing device and cause changes in the detectable signal. At present, the methods of noncontact perception mainly include humidity sensing,65,66 triboelectric sensing,67–69 infrared radiation sensing,70 magnetic sensing71 and capacitive sensing.72,73 The sensing modes suitable for ionic skin are mainly based on triboelectric sensors, humidity and capacitive sensors.
Intriguingly, natural organisms with remote sensing ability are also available, and these biological sensing modes provide a referable model for the fabrication of artificial noncontact sensing devices. The most famous are elasmobranch fishes, including sharks and rays. Their hydrogel-type ionic electroreceptors distributed on the skin surface can sense the intensity of the surrounding electric field, and realize the remote location of prey by comparing the signal differences of multiple electroreceptors. Inspired by this kind of bio-electroreceptor, Wang and Sun respectively fabricated artificial electroreceptors based on ionic hydrogels whose sensing mechanisms were based on triboelectric and electrostatic induction effects.67,68 Because of the common triboelectric phenomenon, the surface of the surrounding object usually has a specific charge. With the object approaching the ionic hydrogel receiver, the ions will locally accumulate on account of the electrostatic induction effect, and then the induced electric current will be generated across the external load (Fig. 12). By measuring the voltage intensity at both ends of the external load, the electric strength and polarity of the target can be determined. Since the charge properties of the object depends on the individual, and the distance of the charged object also affects the ion polarization state of the receiver, the category and relative distance of the object can also be detected. However, due to the universality of the triboelectric phenomenon, the sensing process of these electroreceptors is easily disturbed by the external environment, resulting in the uncertainty and instability of the sensing signal. Although some beneficial attempts have been carried out to improve the accuracy, such as arrays of electroreceptors and deep learning assistance,67,69 the perceptual accuracy and stability are still not enough to support their application in intricate practice.
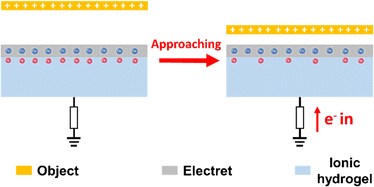 |
| Fig. 12 The structure and sensing mechanism of an artificial electroreceptor. | |
The electrical properties of ionic conductors, such as resistance and capacitance, are usually highly sensitive to moisture, which can be attributed to the changes in ion migration and concentration with the absorption and desorption of water molecules. Besides, there is a certain humidity gradient on the surface of the human hand, hence, humidity is another kind of widely adopted sensing medium in the noncontact perception of human-machine interaction. However, such ionic humidity-sensing materials often have the disadvantage of a long time response, which is caused by the difficulty of the reversible desorption of water molecules. This issue was also addressed in our previous review.9 Recently, Zhang et al. designed and fabricated a self-powered flexible humidity sensor based on a metal-air battery for noncontact perception (Fig. 13).66 On the one hand, this flexible ionic sensor can quantitatively monitor the humidity gradient by the influence of the absorbed water molecules on the migration of ions in the electrolyte. On the other hand, because the adsorbed water molecules can participate in the chemical reaction of the battery and be consumed, this will accelerate the recovery of the sensing material to the original state, and greatly reduce the response recovery time. This kind of sensor material can be called a water molecular consumable humidity sensor, which provides a feasible scheme for the humidity sensor with a fast response time. Of course, there is a consumption process of the electrode material, which will restrict the service life of this kind of sensor.
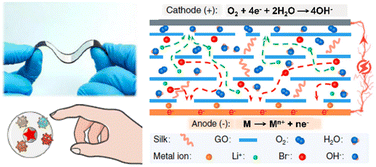 |
| Fig. 13 Humidity-sensitive chemoelectric flexible ionic sensors for noncontact perception. Reproduced with permission from ref. 66, copyright 2022, Nature Publishing Group. | |
The above cases indicate a possible sensing mechanism for ionic skin to acquire noncontact perception. However, the existing noncontact sensing mechanisms have their own unavoidable defects. Future developments in this field should focus on expanding sensing modes or coupling multiple sensing mechanisms to improve sensing accuracy and durability.
5 Perception under harsh conditions
As human footprints appear in the highlands, polar regions, abyssal sea, deep space and other unusually harsh environmental areas, there are additional requirements for the operability of ionic skin in different environments. In order to adapt to harsh environments, ionic skin has been developed in recent years, considering its material and molecular design, to ensure the effectiveness of its perception function in harsh conditions. These additional functions toward harsh environments not only expand the range of operating scenarios of ionic skin but also provide a timely warning about environmental conditions that may cause harm to humans. This part will be divided into two summaries to introduce the material design principles and research progress of ionic skin in freeze-tolerance, and underwater perception.
5.1 Freezing-tolerant ionic skin
The change of seasons and the differences in altitude and latitude make the ambient temperature range of the earth usually between −40 °C and 50 °C. With humans venturing into cold areas, ionic skin with freezing-tolerant properties is desirable. This purpose is to expand the application scenarios of ionic skin by maintaining its flexibility, and its stable and reliable sensing performance under extremely cold conditions. For different types of ionic skin, such as ionic hydrogel and ionic organic gel, there are obvious differences in their intrinsic freezing tolerance. This section analyzes the materials design strategy of the existing ionic skin with the property of freezing tolerance from the perspective of the composition types of ionic skin.
The freezing tolerance of ionic skin utilizing water as the ion conduction medium is dominated by the formation of ice. When the water in the ionic hydrogel freezes into ice, the mechanical properties and ion migration will be affected disastrously. Therefore, reducing the freezing point of water and inhibiting the large-scale growth of ice crystals are the universal strategies to improve the freezing resistance of this type of ionic skin.74 The formation and growth of ice nuclei are mediated by high-density hydrogen bonds between water molecules. Breaking the hydrogen bond between water molecules is the essential reason to produce the reduced freezing point. Ethylene glycol, glycerol, DMSO, etc. can provide external hydrogen bond interactions to break the intrinsic hydrogen bonds within water molecules, thereby inhibiting the formation of ice nuclei.75–81 For example, He et al. prepared ionic hydrogels based on polyvinyl alcohol via the co-nonsolvency effect of the binary system of DMSO and water.82 According to the phase diagram, the gel with the content of 60 wt% DMSO exhibits the lowest freezing point (Fig. 14a). At the same time, after introducing H2SO4, this gel still maintains good mechanical and electrical properties at −50 °C (Fig. 14b and c). In addition, organic salt and inorganic salt are frequently used as antifreeze additives to depress the freezing point.83–86 The incorporation of organic salt or inorganic salt into the hydrogel not only endows it with ionic conductivity but also significantly disturbs the hydrogen bond interaction between water molecules by the electrostatic interaction. Typically, calcium chloride is often used as a deicing agent to reduce the freezing point of water to prevent road icing. Similarly, it can be regarded as a functional additive to prepare freezing-tolerant ionic skin with excellent mechanical properties. Vlassak et al. divided the freezing behavior of hydrogels with decreasing temperature into three stages: 1) a regular hydrogel, in which the aqueous phase is in a fluid state; 2) a slurry gel, the aqueous phase of which is in the partially frozen state; 3) a frozen gel, the aqueous phase of which is in the completely frozen state (Fig. 15a).83 Their research found that with the increase of calcium chloride content, the temperature required for the hydrogel to reach its slurry gel and frozen gel state is lower. Meanwhile, the slurry gel state exhibits relatively enhanced fracture toughness due to the introduction of toughening mechanisms such as crack pinning, crack deflection and energy dissipation by the micro-cavity formed around the ice crystals (Fig. 15b). The basic analysis of this simple system provides a new viewpoint for the preparation of freezing-tolerant ionic hydrogels with robust toughness. The inspiration for another strategy for inhibiting the growth of ice crystals can be traced back to natural organisms. There are numerous freezing-tolerant organisms in nature and their tolerance to extremely cold environments is attributed to the presence of antifreeze proteins. Antifreeze proteins are the typical hybrids of hydrophilic and hydrophobic structures in which the hydrophobic surface can bind to the tiny ice crystals to inhibit their further expansion. Therefore, studies have been conducted to add natural antifreeze proteins to the ionic hydrogel system to ensure the effectiveness of its sensing performance in low-temperature environments.87
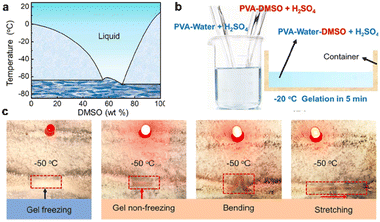 |
| Fig. 14 (a) Phase diagram of a mixed solution of water and DMSO. (b) The fabrication process of freeze-tolerant ionic skin based on the mixed solvent of water and DMSO. (c) This ionic skin can still light LED lights at −50 °C. Reproduced with permission from ref. 82, copyright 2021, Wiley-VCH. | |
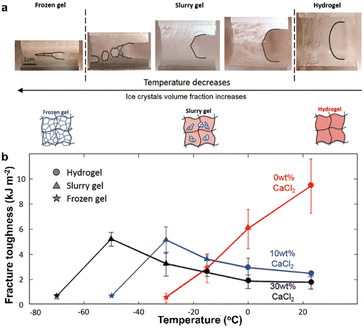 |
| Fig. 15 (a) Crack growth process of a hydrogel containing calcium chloride during the cooling process. The hydrogel consists of three stages: 1) regular hydrogel; 2) slurry gel; 3) frozen gel. (b) The fracture toughness of hydrogels with different calcium chloride contents at sub-zero temperatures. Reproduced with permission from ref. 83, copyright 2018, Wiley-VCH. | |
Compared with the ionic hydrogels as mentioned above, ionic skin based on ionic organic gels has more prominent advantages in freezing tolerance, which is due to the intrinsic low freezing point of organic ionic materials such as ionic liquids.88,89 Of course, the influence of the glass transition temperature of the polymer skeleton on the freezing tolerance cannot be ignored.90 The doped ionic liquid can exert a plasticizing effect to reduce the glass transition temperature of the material, thus assisting it to maintain excellent stretchability at low temperatures.
5.2 Underwater perception
Wearable underwater sensing devices are of great significance for humans to get involved in deep-sea exploration and underwater sports. It can provide the timely transmission and feedback of human physiological signals to ensure safety. Moreover, it also can allow professional underwater athletes to grasp movement posture information and assist in the improvement of their professional skills. Giving ionic skin the ability of underwater perception requires overcoming two tricky issues: 1) swelling capacity; for the common ionic skin with a hydrophilic skeleton and conducting ions, swelling will occur unexpectedly and cause damage to the mechanical properties. 2) Diffusion of conducting ions; due to the effect of ion concentration, the ions in the ionic skin tend to diffuse into the water, which leads to the instability of its sensing performance. Therefore, given the above two issues, the fabrication strategies for water-resistant ionic skin can be roughly divided into two categories. The first is to decrease the osmotic pressure of the ionic hydrogel system to reduce the loss of conductive ions as much as possible. For example, Lü and Wei et al. elaborately took advantage of the positive charge characteristic of zwitterionic hydrogels in acidic environments.91 The existence of electrostatic repulsion reduced the osmotic pressure and the prepared ionic hydrogel showed excellent swelling resistance and ion retention ability. Secondly, regulating the hydrophobicity of ionic skin to shield external water is the most common means. Typically, the anion [TFSI] is the most selected hydrophobic conducting ion for ionic liquids.92–103 In addition, hydrophobic polymer skeletons, such as P(VDF–HFP),94,102 fluorinated polyurethane,96 fluorinated poly(ionic liquid),93,95,100etc., have also been frequently tried for the preparation of water-resistant ionic skin.
6 Other attractive functions of ionic skin
Over the course of a decade, in addition to the aforementioned perceptual functions, several emergent properties have been incorporated into ionic skin to respond to practical needs. Some recent reports on the extended functions of ionic skin are summarized and listed below.
6.1 Self-healing
Similar to natural skin, ionic skin is also expected to gain self-healing properties to extend its service life. In general, ionic skin systems with abundant dynamic covalent bonds or supramolecular interactions often exhibit self-healing properties after mechanical damage. Of course, self-healing is a commonplace topic and has been deeply discussed in numerous previous reports,104,105 so it will not be repeated in this paper.
6.2 UV filtering
In high-altitude environments, intense ultraviolet light can accelerate melanin deposits, damage DNA and even cause skin cancer. Some recent studies have sought to obtain ionic skin with UV filtering capability to broaden the range of applications. The incorporation of UV light absorption and refraction materials, such as tannic acid-coated inorganic nanomaterials, is the main contributor to the UV filtering capability of these ionic skins.106,107 Besides, there is also ionic skin that relies on the physical structure formed by the polymer network itself to block UV. Wu et al. used a nanoscale network formed by chitosan and poly(methacrylic acid) to scatter and absorb UV.108
6.3 Antibacterial
Antibacterial properties, an additional function that is the icing on the cake of ionic skin, are also attracting widespread attention. Hydrogel-based ionic skin with its suitable water environment is conducive to the growth of bacteria. In contrast, those systems that utilize organic ions, especially imidazole cations, as conducting ions, have intrinsic antimicrobial properties, which are due to the non-specific adsorption of positive imidazole cations to the surface of the cell membrane and the disturbance of the phospholipid bilayer by its hydrophobic alkyl chains.109,110 There are also strategies to introduce nitric oxide as an antimicrobial agent into ionic skin to achieve a broad spectrum of antibacterial properties.111
6.4 Theranostic
Ionic skin can also be regarded as a powerful management platform for wound healing and the local delivery of drugs. The ionic skin, as a container, can reserve stimulant factors that promote wound healing or therapeutic drugs, and forwardly establish a substance transport channel between the tissue interfaces by means of the existing concentration gradient.111,112 Ionic skin patches can also act as an application window for electrical stimulation to accelerate wound healing.113
6.5 Self-cleanable
Self-cleanable ionic skin can be customized, meaning that specific materials are designed for specific cleaning objects. For instance, the cleaning of ink stains can depend on the hydrophobic effect of fluorinated ionic skin.114 Ionic skin based on zwitterions has excellent efficacy against the adhesion of bacteria and biofilm.115
7 Conclusion and perspectives
The last decade has witnessed the birth and development of the ionic skin, which uses ions as the sensory signal acquisition and transmission medium. Compared with traditional electronic skin, ionic skin can easily achieve ultra-stretchability, transparency and biocompatibility while taking into account excellent sensing performance. More importantly, its sensing process based on ion migration is highly similar to that of natural skin. Therefore, many bionic strategies that regulate ion migration have been adopted to prepare ionic skin with high sensitivity and a wide range of mechanical and thermal perceptions. In addition, the richness and diversity of ionic soft materials make ionic skin a multi-functional application platform, and various additional functions can be derived that are beyond the natural skin. Concerted efforts over the past decade have resulted in the progress of ionic skin by leaps and bounds. The definition of ionic skin is evolving with the times, that is, ionic skin is a multifunctional flexible sensing device with ionic soft matter as the sensing medium.
However, ionic skin is still in the development stages and the existing ionic skins cannot fully reproduce the perception ability of natural skin, especially in the face of complex and varied external stimuli. The powerful perception of natural skin comes from the synergy of the various receptors. The future development of ionic skin should not only expand the types of artificial receptors to obtain the response-ability to various forms of stimulation but should also pay attention to the coordination of the feedback regulation system of each artificial receptor on the premise of ensuring the perception acquisition without interference. Besides, the density of receptors distributed in the skin is staggering, so the integration of a high density of artificial receptors into ionic skin is a potential requirement to recreate the high-resolution perception of natural skin. At the same time, with the expansion of the functions of ionic skin, the future development of this field should pay more attention to the integrated design of multi-functions in specific application scenarios. For example, ionic skin suitable for nursing injured plateau soldiers should have multiple functions, such as freezing resistance, UV shielding, motion sensing and auxiliary wound treatment. Although ionic skin and natural skin both acquire and transmit external stimuli in the form of ions, there are still great challenges in achieving direct information exchange between them. The suitable soft interface for ion conduction may be the feasible bridge for effective communication between ionic skin and nerves.
Conflicts of interest
The authors declare that they have no known competing financial interests or personal relationships that could have appeared to influence the work reported in this paper.
Acknowledgements
This work was financially supported by the National Natural Science Foundation of China (21825503).
References
- M. Xia, N. Pan, C. Zhang, C. Zhang, W. Fan, Y. Xia, Z. Wang and K. Sui, Self-powered multifunction ionic skins based on gradient polyelectrolyte hydrogels, ACS Nano, 2022, 16, 4714–4725 CrossRef CAS PubMed.
- M. Wang, Y. Luo, T. Wang, C. Wan, L. Pan, S. Pan, K. He, A. Neo and X. Chen, Artificial skin perception, Adv. Mater., 2021, 33, 2003014 CrossRef CAS PubMed.
- A. Zimmerman, L. Bai and D. D. Ginty, The gentle touch receptors of mammalian skin, Science, 2014, 346, 950–954 CrossRef CAS PubMed.
- M. J. Caterina, How do you feel? A warm and touching 2021 Nobel tribute, J. Clin. Invest., 2021, 131, e156587 CrossRef CAS PubMed.
- D. Julius, TRP channels and pain, Annu. Rev. Cell Dev. Biol., 2013, 29, 355–384 CrossRef CAS PubMed.
- Y. Dobashi, D. Yao, Y. Petel, T. N. Nguyen, M. S. Sarwar, Y. Thabet, C. L. W. Ng, E. S. Glitz, G. T. M. Nguyen, C. Plesse, F. Vidal, C. A. Michal and J. D. W. Madden, Piezoionic mechanoreceptors: Force-induced current generation in hydrogels, Science, 2022, 376, 502–507 CrossRef CAS PubMed.
- C.-C. Kim, H.-H. Lee, K. H. Oh and J.-Y. Sun, Highly stretchable, transparent ionic touch panel, Science, 2016, 353, 682–687 CrossRef CAS PubMed.
- J.-Y. Sun, C. Keplinger, G. M. Whitesides and Z. Suo, Ionic skin, Adv. Mater., 2014, 26, 7608–7614 CrossRef CAS PubMed.
- Z. Chen and Y. Wang, Organic ionic fluid-based wearable sensors for healthcare, Sens. Diagn., 2022, 1, 598–613 RSC.
- Z. Chen, N. Gao, Y. Chu, Y. He and Y. Wang, Ionic network based on dynamic ionic liquids for electronic tattoo application, ACS Appl. Mater. Interfaces, 2021, 13, 33557–33565 CrossRef CAS PubMed.
- Z. Chen, Y. He, X. Tao, Y. Ma, J. Jia and Y. Wang, Thermal nociception of ionic skin: TRPV1 ion channel-inspired heat-activated dynamic ionic liquid, J. Phys. Chem. Lett., 2022, 13, 10076–10084 CrossRef CAS PubMed.
- Y. Gong, Y.-Z. Zhang, S. Fang, C. Liu, J. Niu, G. Li, F. Li, X. Li, T. Cheng and W.-Y. Lai, Artificial intelligent optoelectronic skin with anisotropic electrical and optical responses for multi-dimensional sensing, Appl. Phys. Rev., 2022, 9, 021403 CAS.
- S. Wang, M. Bai, C. Liu, G. Li, X. Lu, H. Cai, C. Liu and W.-Y. Lai, Highly stretchable multifunctional polymer ionic conductor with high conductivity based on organic-inorganic dual networks, Chem. Eng. J., 2022, 440, 135824 CrossRef CAS.
- Y. Gong, Y.-Z. Zhang, S. Fang, Y. Sun, J. Niu and W.-Y. Lai, Wireless human−machine interface based on artificial bionic skin with damage reconfiguration and multisensing capabilities, ACS Appl. Mater. Interfaces, 2022, 14, 47300–47309 CrossRef CAS PubMed.
- C. Yang and Z. Suo, Hydrogel ionotronics, Nat. Rev. Mater., 2018, 3, 125–142 CrossRef CAS.
- K. Xiao, C. Wan, L. Jiang, X. Chen and M. Antonietti, Bioinspired ionic sensory systems: The successor of electronics, Adv. Mater., 2020, 32, 2000218 CrossRef CAS PubMed.
- J. M. Kefauver, A. B. Ward and A. Patapoutian, Discoveries in structure and physiology of mechanically activated ion channels, Nature, 2020, 587, 567–576 CrossRef CAS PubMed.
- M. J. Caterina, M. A. Schumacher, M. Tominaga, T. A. Rosen, J. D. Levine and D. Julius, The capsaicin receptor: a heat-activated ion channel in the pain pathway, Nature, 1997, 389, 816–824 CrossRef CAS PubMed.
- A. Dhaka, V. Viswanath and A. Patapoutian, TRP ion channels and temperature sensation, Annu. Rev. Neurosci., 2006, 29, 135–161 CrossRef CAS PubMed.
- S. Maksimovic, M. Nakatani, Y. Baba, A. M. Nelson, K. L. Marshall, S. A. Wellnitz, P. Firozi, S.-H. Woo, S. Ranade, A. Patapoutian and E. A. Lumpkin, Epidermal Merkel cells are mechanosensory cells that tune mammalian touch receptors, Nature, 2014, 509, 617–621 CrossRef CAS PubMed.
- C. Chen, W. B. Ying, J. Li, Z. Kong, F. Li, H. Hu, Y. Tian, D. H. Kim, R. Zhang and J. Zhu, A self-healing and ionic liquid affiliative polyurethane toward a Piezo 2 protein inspired ionic skin, Adv. Funct. Mater., 2022, 32, 2106341 CrossRef CAS.
- H. J. Hwang, J. S. Kim, W. Kim, H. Park, D. Bhatia, E. Jee, Y. S. Chung, D. H. Kim and D. Choi, An ultra-mechanosensitive visco-poroelastic polymer ion pump for continuous self-powering kinematic triboelectric nanogenerators, Adv. Energy Mater., 2019, 9, 1803786 CrossRef.
- M. L. Jin, S. Park, Y. Lee, J. H. Lee, J. Chung, J. S. Kim, J.-S. Kim, S. Y. Kim, E. Jee, D. W. Kim, J. W. Chung, S. G. Lee, D. Choi, H.-T. Jung and D. H. Kim, An ultrasensitive, visco-poroelastic artificial mechanotransducer skin inspired by Piezo2 protein in mammalian merkel cells, Adv. Mater., 2017, 29, 1605973 CrossRef PubMed.
- J. S. Kim, H. Choi, H. J. Hwang, D. Choi and D. H. Kim, All-printed electronic skin based on deformable and ionic mechanotransducer array, Macromol. Biosci., 2020, 20, 2000147 CrossRef CAS PubMed.
- J. I. Lee, H. Choi, S. H. Kong, S. Park, D. Park, J. S. Kim, S. H. Kwon, J. Kim, S. H. Choi, S. G. Lee, D. H. Kim and M. S. Kang, Visco-poroelastic electrochemiluminescence skin with piezo-ionic effect, Adv. Mater., 2021, 33, 2100321 CrossRef CAS PubMed.
- J. Zhang, H. Yuan, L. Li, J. Zhang, M. Wang, Y. Le and J.-F. Chen, Hydrogel from chrome shavings as a sustainable, highly sensitive ionic skin for pressure sensing, ACS Sustainable Chem. Eng., 2022, 10, 8172–8183 CrossRef CAS.
- J. Yang, Z. Li, X. Zhang, B. Chen, J. Feng and T. Wang, Merkel cell-like artificial mechanoreceptor with high sensitivity and high resolution over a wide linear range, Cell Rep. Phys. Sci., 2022, 3, 101101 CrossRef.
- V. Amoli, J. S. Kim, E. Jee, Y. S. Chung, S. Y. Kim, J. Koo, H. Choi, Y. Kim and D. H. Kim, A bioinspired hydrogen bond-triggered ultrasensitive ionic mechanoreceptor skin, Nat. Commun., 2019, 10, 4019 CrossRef PubMed.
- M. S. U. Sarwar, Y. Dobashi, E. F. S. Glitz, M. Farajollahi, S. Mirabbasi, S. Nafici, G. M. Spinks and J. D. W. Madden, Transparent and conformal ‘piezoionic’ touch sensor, Proc. SPIE, 2015, 9430, 943026 CrossRef.
- W. Takashima, T. Uesugi, M. Fukui, M. Kaneko and K. Kaneto, Mechanochemoelectrical effect of polyaniline film, Synth. Met., 1997, 85, 1395–1396 CrossRef CAS.
- B. Bhandari, G.-Y. Lee and S.-H. Ahn, A review on IPMC material as actuators and sensors: Fabrications, characteristics and applications, Int. J. Precis. Eng. Manuf., 2012, 13, 141–163 CrossRef.
- Y. Wu, G. Alici, J. D. W. Madden, G. M. Spinks and G. G. Wallace, Soft mechanical sensors through reverse actuation in polypyrrole, Adv. Funct. Mater., 2007, 17, 3216–3222 CrossRef CAS.
- T. Shoa, J. D. W. Madden, T. Mirfakhrai, G. Alici, G. M. Spinks and G. G. Wallace, Electromechanical coupling in polypyrrole sensors and actuators, Sens. Actuators, A, 2010, 161, 127–133 CrossRef CAS.
- M. Shahinpoor, Y. Bar-Cohen, J. O. Simpson and J. Smith, Ionic polymer-metal composites (IPMCs) as biomimetic sensors, actuators and artificial muscles - a review, Smart Mater. Struct., 1998, 7, R15 CrossRef CAS.
- Y. Liu, Y. Hu, J. Zhao, G. Wu, X. Tao and W. Chen, Self-powered piezoionic strain sensor toward the monitoring of human activities, Small, 2016, 12, 5074–5080 CrossRef CAS PubMed.
- V. E. Abraira and D. D. Ginty, The sensory neurons of touch, Neuron, 2013, 79, 618–639 CrossRef CAS PubMed.
- S. Chun, J.-S. Kim, Y. Yoo, Y. Choi, S. J. Jung, D. Jang, G. Lee, K.-I. Song, K. S. Nam, I. Youn, D. Son, C. Pang, Y. Jeong, H. Jung, Y.-J. Kim, B.-D. Choi, J. Kim, S.-P. Kim, W. Park and S. Park, An artificial neural tactile sensing system, Nat. Electron., 2021, 4, 429–438 CrossRef.
- H.-J. Yoon, D.-M. Lee, Y.-J. Kim, S. Jeon, J.-H. Jung, S. S. Kwak, J. Kim, S. Kim, Y. Kim and S.-W. Kim, Mechanoreceptor-inspired dynamic mechanical stimuli perception based on switchable ionic polarization, Adv. Funct. Mater., 2021, 31, 2100649 CrossRef CAS.
- K.-Y. Chun, Y. J. Son, E.-S. Jeon, S. Lee and C.-S. Han, A self-powered sensor mimicking slow- and fast-adapting cutaneous mechanoreceptors, Adv. Mater., 2018, 30, 1706299 CrossRef PubMed.
- Y. He, S. Liao, H. Jia, Y. Cao, Z. Wang and Y. Wang, A self-healing electronic sensor based on thermal-sensitive fluids, Adv. Mater., 2015, 27, 4622–4627 CrossRef CAS PubMed.
- S. Sun, Z. Duan, X. Wang, G. Lai, X. Zhang, H. Wei, L. Liu and N. Ma, Cheap, flexible, and thermal-sensitive paper sensor through writing with ionic liquids containing pencil leads, ACS Appl. Mater. Interfaces, 2017, 9, 29140–29146 CrossRef CAS PubMed.
- N. Gao, Y. He, X. Tao, X.-Q. Xu, X. Wu and Y. Wang, Crystal-confined freestanding ionic liquids for reconfigurable and repairable electronics, Nat. Commun., 2019, 10, 547 CrossRef CAS PubMed.
- H. Jia, Y. He, X. Zhang, W. Du and Y. Wang, Integrating ultra-thermal-sensitive fluids into elastomers for multifunctional flexible sensors, Adv. Electron. Mater., 2015, 1, 1500029 CrossRef.
- X. Tao, H. Jia, Y. He, S. Liao and Y. Wang, Ultrafast paper thermometers based on a green sensing ink, ACS Sens., 2017, 2, 449–454 CrossRef CAS PubMed.
- H. Liao, S. Liao, X. Tao, C. Liu and Y. Wang, Intrinsically recyclable and self-healable conductive supramolecular polymers for customizable electronic sensors, J. Mater. Chem. C, 2018, 6, 12992–12999 RSC.
- Z. Chen, Q. Gui and Y. Wang, Dynamic
chemistry in ionic liquid-based conductor, Green Chem. Eng., 2021, 2, 346–358 CrossRef.
- Y. Wang, X. Cao, J. Cheng, B. Yao, Y. Zhao, S. Wu, B. Ju, S. Zhang, X. He and W. Niu, Cephalopod-inspired chromotropic ionic skin with rapid visual sensing capabilities to multiple stimuli, ACS Nano, 2021, 15, 3509–3521 CrossRef CAS PubMed.
- C. Larson, B. Peele, S. Li, S. Robinson, M. Totaro, L. Beccai, B. Mazzolai and R. Shepherd, Highly stretchable electroluminescent skin for optical signaling and tactile sensing, Science, 2016, 351, 1071–1074 CrossRef CAS PubMed.
- Q. Lyu, S. Wang, B. Peng, X. Chen, S. Du, M. Li, L. Zhang and J. Zhu, Bioinspired photonic ionogels as interactively visual ionic skin with optical and electrical synergy, Small, 2021, 17, 2103271 CrossRef CAS PubMed.
- Y. Zhao, Z. Xie, H. Gu, C. Zhu and Z. Gu, Bio-inspired variable structural color materials, Chem. Soc. Rev., 2012, 41, 3297–3317 RSC.
- L. Bai, Y. Jin, X. Shang, L. Shi, H. Jin, R. Zhou and S. Lai, Bio-inspired visual multi-sensing interactive ionic skin with asymmetrical adhesive, antibacterial and self-powered functions, Chem. Eng. J., 2022, 438, 135596 CrossRef CAS.
- L. Bai, Y. Jin, X. Shang, H. Jin, Y. Zhou and L. Shi, Highly synergistic, electromechanical and mechanochromic dual-sensing ionic skin with multiple monitoring, antibacterial, self-healing, and anti-freezing functions, J. Mater. Chem. A, 2021, 9, 23916–23928 RSC.
- Y. Wang, W. Niu, C.-Y. Lo, Y. Zhao, X. He, G. Zhang, S. Wu, B. Ju and S. Zhang, Interactively full-color changeable electronic fiber sensor with high stretchability and rapid response, Adv. Funct. Mater., 2020, 30, 2000356 CrossRef CAS.
- N. Yin, C. Zhao, X. Zhang, F. Huo, Y. Shu and J. Wang, Tailoring the phase transition and luminescence behaviors of a poly(ionic liquid) to ensure visual temperature sensing, ACS Appl. Polym. Mater., 2022, 4, 191–199 CrossRef CAS.
- Q. Gui, B. Fu, Y. He, S. Lyu, Y. Ma and Y. Wang, Visualizing thermal distribution through hydrogel confined ionic system, iScience, 2021, 24, 102085 CrossRef CAS PubMed.
- S. W. Lee, S. H. Cho, H. S. Kang, G. Kim, J. S. Kim, B. Jeong, E. H. Kim, S. Yu, I. Hwang, H. Han, T. H. Park, S.-H. Jung, J. K. Lee, W. Shim and C. Park, Electroluminescent pressure-sensing displays, ACS Appl. Mater. Interfaces, 2018, 10, 13757–13766 CrossRef CAS PubMed.
- J. Wang, C. Yan, G. Cai, M. Cui, A. Lee-Sie Eh and P. See Lee, Extremely stretchable electroluminescent devices with ionic conductors, Adv. Mater., 2016, 28, 4490–4496 CrossRef CAS PubMed.
- C. H. Yang, B. Chen, J. Zhou, Y. M. Chen and Z. Suo, Electroluminescence of giant stretchability, Adv. Mater., 2016, 28, 4480–4484 CrossRef CAS PubMed.
- C. Yang, S. Cheng, X. Yao, G. Nian, Q. Liu and Z. Suo, Ionotronic luminescent fibers, fabrics, and other configurations, Adv. Mater., 2020, 32, 2005545 CrossRef CAS PubMed.
- P. Zhang, W. Guo, Z. H. Guo, Y. Ma, L. Gao, Z. Cong, X. J. Zhao, L. Qiao, X. Pu and Z. L. Wang, Dynamically crosslinked dry ion-conducting elastomers for soft iontronics, Adv. Mater., 2021, 33, 2101396 CrossRef CAS PubMed.
- L. Wang, L. Xiao, H. Gu and H. Sun, Advances in alternating current electroluminescent devices, Adv. Opt. Mater., 2019, 7, 1801154 CrossRef.
- X. Shi, Y. Zuo, P. Zhai, J. Shen, Y. Yang, Z. Gao, M. Liao, J. Wu, J. Wang, X. Xu, Q. Tong, B. Zhang, B. Wang, X. Sun, L. Zhang, Q. Pei, D. Jin, P. Chen and H. Peng, Large-area display textiles integrated with functional systems, Nature, 2021, 591, 240–245 CrossRef CAS PubMed.
- J. H. Kwon, Y. M. Kim and H. C. Moon, Porous ion gel: A versatile ionotronic sensory platform for high-performance, wearable ionoskins with electrical and optical dual output, ACS Nano, 2021, 15, 15132–15141 CrossRef CAS PubMed.
- Y. Lu, G. Yang, Y. Shen, H. Yang and K. Xu, Multifunctional flexible humidity sensor systems towards noncontact wearable electronics, Nano-Micro Lett., 2022, 14, 150 CrossRef CAS PubMed.
- L. Lu, C. Jiang, G. Hu, J. Liu and B. Yang, Flexible noncontact sensing for human–machine interaction, Adv. Mater., 2021, 33, 2100218 CrossRef CAS PubMed.
- S. Li, Y. Zhang, X. Liang, H. Wang, H. Lu, M. Zhu, H. Wang, M. Zhang, X. Qiu, Y. Song and Y. Zhang, Humidity-sensitive chemoelectric flexible sensors based on metal-air redox reaction for health management, Nat. Commun., 2022, 13, 5416 CrossRef CAS PubMed.
- Z. H. Guo, H. L. Wang, J. Shao, Y. Shao, L. Jia, L. Li, X. Pu and Z. L. Wang, Bioinspired soft electroreceptors for artificial precontact somatosensation, Sci. Adv., 2022, 8, eabo5201 CrossRef CAS PubMed.
- W. J. Song, Y. Lee, Y. Jung, Y.-W. Kang, J. Kim, J.-M. Park, Y.-L. Park, H.-Y. Kim and J.-Y. Sun, Soft artificial electroreceptors for noncontact spatial perception, Sci. Adv., 2021, 7, eabg9203 CrossRef CAS PubMed.
- H. Zhou, W. Huang, Z. Xiao, S. Zhang, W. Li, J. Hu, T. Feng, J. Wu, P. Zhu and Y. Mao, Deep-learning-assisted noncontact gesture-recognition system for touchless human-machine interfaces, Adv. Funct. Mater., 2022, 32, 2208271 CrossRef CAS.
- S. An, H. Zhu, C. Guo, B. Fu, C. Song, P. Tao, W. Shang and T. Deng, Noncontact human-machine interaction based on hand-responsive infrared structural color, Nat. Commun., 2022, 13, 1446 CrossRef CAS PubMed.
- P. Makushko, E. S. Oliveros Mata, G. S. Cañón Bermúdez, M. Hassan, S. Laureti, C. Rinaldi, F. Fagiani, G. Barucca, N. Schmidt, Y. Zabila, T. Kosub, R. Illing, O. Volkov, I. Vladymyrskyi, J. Fassbender, M. Albrecht, G. Varvaro and D. Makarov, Flexible magnetoreceptor with tunable intrinsic logic for on-skin touchless human-machine interfaces, Adv. Funct. Mater., 2021, 31, 2101089 CrossRef CAS.
- J. Huang, H. Wang, J. Li, S. Zhang, H. Li, Z. Ma, M. Xin, K. Yan, W. Cheng, D. He, X. Wang, Y. Shi and L. Pan, High-performance flexible capacitive proximity and pressure sensors with spiral electrodes for continuous human−machine interaction, ACS Mater. Lett., 2022, 4, 2261–2272 CrossRef CAS.
- Q. Wang, H. Ding, X. Hu, X. Liang, M. Wang, Q. Liu, Z. Li and G. Sun, A dual-trigger-mode ionic hydrogel sensor for contact or contactless motion recognition, Mater. Horiz., 2020, 7, 2673–2682 RSC.
- Z. Wang, C. Valenzuela, J. Wu, Y. Chen, L. Wang and W. Feng, Bioinspired freeze-tolerant soft materials: Design, properties, and applications, Small, 2022, 18, 2201597 CrossRef CAS PubMed.
- Z. Wang, L. Chen, Y. Chen, P. Liu, H. Duan and P. Cheng, 3D printed ultrastretchable, hyper-antifreezing conductive hydrogel for sensitive motion and electrophysiological signal monitoring, Research, 2020, 2020, 1426078 CAS.
- Y. Ye, Y. Zhang, Y. Chen, X. Han and F. Jiang, Cellulose nanofibrils enhanced, strong, stretchable, freezing-tolerant ionic conductive organohydrogel for multi-functional sensors, Adv. Funct. Mater., 2020, 30, 2003430 CrossRef CAS.
- J. Liu, Z. Chen, Y. Chen, H. U. Rehman, Y. Guo, H. Li and H. Liu, Ionic conductive organohydrogels with dynamic pattern behavior and multi-environmental stability, Adv. Funct. Mater., 2021, 31, 2101464 CrossRef CAS.
- B. Ying, R. Z. Chen, R. Zuo, J. Li and X. Liu, An anti-freezing, ambient-stable and highly stretchable ionic skin with strong surface adhesion for wearable sensing and soft robotics, Adv. Funct. Mater., 2021, 31, 2104665 CrossRef CAS.
- B. Ying, R. Zuo, Y. Wan and X. Liu, An ionic hydrogel-based antifreezing triboelectric nanogenerator, ACS Appl. Electron. Mater., 2022, 4, 1930–1938 CrossRef CAS.
- Z. Xu, F. Zhou, H. Yan, G. Gao, H. Li, R. Li and T. Chen, Anti-freezing organohydrogel triboelectric nanogenerator toward highly efficient and flexible human-machine interaction at −30 °C, Nano Energy, 2021, 90, 106614 CrossRef CAS.
- Q. Yu, Z. Qin, F. Ji, S. Chen, S. Luo, M. Yao, X. Wu, W. Liu, X. Sun, H. Zhang, Y. Zhao, F. Yao and J. Li, Low-temperature tolerant strain sensors based on triple crosslinked organohydrogels with ultrastretchability, Chem. Eng. J., 2021, 404, 126559 CrossRef CAS.
- S. Wu, Y. Alsaid, B. Yao, Y. Yan, Y. Zhao, M. Hua, D. Wu, X. Zhu and X. He, Rapid and scalable fabrication of ultra-stretchable, anti-freezing conductive gels by cononsolvency effect, EcoMat, 2021, 3, e12085 CAS.
- X. P. Morelle, W. R. Illeperuma, K. Tian, R. Bai, Z. Suo and J. J. Vlassak, Highly stretchable and tough hydrogels below water freezing temperature, Adv. Mater., 2018, 30, 1801541 CrossRef PubMed.
- W. Wang, Y. Liu, S. Wang, X. Fu, T. Zhao, X. Chen and Z. Shao, Physically cross-linked silk fibroin-based tough hydrogel electrolyte with exceptional water retention and freezing tolerance, ACS Appl. Mater. Interfaces, 2020, 12, 25353–25362 CrossRef CAS PubMed.
- Z. Liu, Y. Wang, Y. Ren, G. Jin, C. Zhang, W. Chen and F. Yan, Poly(ionic liquid) hydrogel-based anti-freezing ionic skin for a soft robotic gripper, Mater. Horiz., 2020, 7, 919–927 RSC.
- Y. Liu, W. Wang, K. Gu, J. Yao, Z. Shao and X. Chen, Poly(vinyl alcohol)
hydrogels with integrated toughness, conductivity, and freezing tolerance based on ionic liquid/water binary solvent systems, ACS Appl. Mater. Interfaces, 2021, 13, 29008–29020 CrossRef CAS PubMed.
- Y. Wang, Y. Xia, P. Xiang, Y. Dai, Y. Gao, H. Xu, J. Yu, G. Gao and K. Chen, Protein-assisted freeze-tolerant hydrogel with switchable performance toward customizable flexible sensor, Chem. Eng. J., 2022, 428, 131171 CrossRef CAS.
- P. Lv, L. Shi, C. Fan, Y. Gao, A. Yang, X. Wang, S. Ding and M. Rong, Hydrophobic ionic liquid gel-based triboelectric nanogenerator: Next generation of ultrastable, flexible, and transparent power sources for sustainable electronics, ACS Appl. Mater. Interfaces, 2020, 12, 15012–15022 CrossRef CAS PubMed.
- L. Zhao, B. Wang, Z. Mao, X. Sui and X. Feng, Nonvolatile, stretchable and adhesive ionogel fiber sensor designed for extreme environments, Chem. Eng. J., 2022, 433, 133500 CrossRef CAS.
- Q. Ma, S. Liao, Y. Ma, Y. Chu and Y. Wang, An ultra-low-temperature elastomer with excellent mechanical performance and solvent resistance, Adv. Mater., 2021, 33, 2102096 CrossRef CAS PubMed.
- J. Ren, Y. Liu, Z. Wang, S. Chen, Y. Ma, H. Wei and S. Lü, An anti-swellable hydrogel strain sensor for underwater motion detection, Adv. Funct. Mater., 2022, 32, 2107404 CrossRef CAS.
- J. Wei, Y. Zheng and T. Chen, A fully hydrophobic ionogel enables highly efficient wearable underwater sensors and communicators, Mater. Horiz., 2021, 8, 2761–2770 RSC.
- L. Rong, X. Xie, W. Yuan and Y. Fu, Superior, environmentally tolerant, flexible, and adhesive poly(ionic liquid) gel as a multifaceted underwater sensor, ACS Appl. Mater. Interfaces, 2022, 14, 29273–29283 CrossRef CAS PubMed.
- J. Xu, H. Wang, X. Wen, S. Wang and H. Wang, Mechanically strong, wet adhesive, and self-healing polyurethane ionogel enhanced with a semi-interpenetrating network for underwater motion detection, ACS Appl. Mater. Interfaces, 2022, 14, 54203–54214 CrossRef CAS PubMed.
- Z. Yu and P. Wu, Water-resistant ionogel electrode with tailorable mechanical properties for aquatic ambulatory physiological signal monitoring, Adv. Funct. Mater., 2021, 31, 2107226 CrossRef CAS.
- T. Chen, G. Ye, H. Wu, S. Qi, G. Ma, Y. Zhang, Y. Zhao, J. Zhu, X. Gu and N. Liu, Highly conductive and underwater stable ionic skin for all-day epidermal biopotential monitoring, Adv. Funct. Mater., 2022, 32, 2206424 CrossRef CAS.
- Y. Cheng, C. Guo, S. Li, K. Deng, J. Tang, Q. Luo, S. Zhang, Y. Chang and T. Pan, Aquatic skin enabled by multi-modality iontronic sensing, Adv. Funct. Mater., 2022, 32, 2205947 CrossRef CAS.
- P. Shi, Y. Wang, K. Wan, C. Zhang and T. Liu, A waterproof ion-conducting fluorinated elastomer with 6000% stretchability, superior ionic conductivity, and harsh environment tolerance, Adv. Funct. Mater., 2022, 32, 2112293 CrossRef CAS.
- L. Xu, Z. Huang, Z. Deng, Z. Du, T. L. Sun, Z.-H. Guo and K. Yue, A transparent, highly stretchable, solvent-resistant, recyclable multifunctional ionogel with underwater self-healing and adhesion for reliable strain sensors, Adv. Mater., 2021, 33, 2105306 CrossRef CAS PubMed.
- Z. Yu and P. Wu, Underwater communication and optical camouflage ionogels, Adv. Mater., 2021, 33, 2008479 CrossRef CAS PubMed.
- P. Shi, Y. Wang, W. W. Tjiu, C. Zhang and T. Liu, Highly stretchable, fast self-healing, and waterproof fluorinated copolymer ionogels with selectively enriched ionic liquids for human-motion detection, ACS Appl. Mater. Interfaces, 2021, 13, 49358–49368 CrossRef CAS PubMed.
- Y. Cao, Y. J. Tan, S. Li, W. W. Lee, H. Guo, Y. Cai, C. Wang and B. C. K. Tee, Self-healing electronic skins for aquatic environments, Nat. Electron., 2019, 2, 75–82 CrossRef.
- L. Ma, J. Wang, J. He, Y. Yao, X. Zhu, L. Peng, J. Yang, X. Liu and M. Qu, Ultra-sensitive, durable and stretchable ionic skins with biomimetic micronanostructures for multisignal detection, high-precision motion monitoring, and underwater sensing, J. Mater. Chem. A, 2021, 9, 26949–26962 RSC.
- S. Liao, X. Lian and Y. Wang, Self-healing ionic liquid-based electronics and beyond, Chin. J. Polym. Sci., 2021, 39, 1235–1245 CrossRef CAS.
- J. Kang, J. B. H. Tok and Z. Bao, Self-healing soft electronics, Nat. Electron., 2019, 2, 144–150 CrossRef.
- J. Wen, J. Tang, H. Ning, N. Hu, Y. Zhu, Y. Gong, C. Xu, Q. Zhao, X. Jiang, X. Hu, L. Lei, D. Wu and T. Huang, Multifunctional ionic skin with sensing, UV-filtering, water-retaining, and anti-freezing capabilities, Adv. Funct. Mater., 2021, 31, 2011176 CrossRef CAS.
- X. Pan, Q. Wang, R. Guo, Y. Ni, K. Liu, X. Ouyang, L. Chen, L. Huang, S. Cao and M. Xie, An integrated transparent, UV-filtering organohydrogel sensor via molecular-level ion conductive channels, J. Mater. Chem. A, 2019, 7, 4525–4535 RSC.
- X. Shi, Z. Lei and P. Wu, An intelligent light-managing ionic skin for UV-protection, IR stealth, and optical camouflaged Morse codes, Sci. China Mater., 2022, 65, 2281–2288 CrossRef CAS.
- N. Jiang, X. Chang, D. Hu, L. Chen, Y. Wang, J. Chen and Y. Zhu, Flexible, transparent, and antibacterial ionogels toward highly sensitive strain and temperature sensors, Chem. Eng. J., 2021, 424, 130418 CrossRef CAS.
- Y.-R. Gao, J.-F. Cao, Y. Shu and J.-H. Wang, Research progress of ionic liquids-based gels in energy storage, sensors and antibacterial, Green Chem. Eng., 2021, 2, 368–383 CrossRef.
- X. Lin, Y. Mao, P. Li, Y. Bai, T. Chen, K. Wu, D. Chen, H. Yang and L. Yang, Ultra-conformable ionic skin with multi-modal sensing, broad-spectrum antimicrobial and regenerative capabilities for smart and expedited wound care, Adv. Sci., 2021, 8, 2004627 CrossRef CAS PubMed.
- Z. Lei, W. Zhu, X. Zhang, X. Wang and P. Wu, Bio-inspired ionic skin for theranostics, Adv. Funct. Mater., 2021, 31, 2008020 CrossRef CAS.
- S.-H. Jeong, Y. Lee, M.-G. Lee, W. J. Song, J.-U. Park and J.-Y. Sun, Accelerated wound healing with an ionic patch assisted by a triboelectric nanogenerator, Nano Energy, 2021, 79, 105463 CrossRef CAS.
- Y. Lee, S. H. Cha, Y.-W. Kim, D. Choi and J.-Y. Sun, Transparent and attachable ionic communicators based on self-cleanable triboelectric nanogenerators, Nat. Commun., 2018, 9, 1804 CrossRef PubMed.
- H. Guo, M. Bai, C. Wen, M. Liu, S. Tian, S. Xu, X. Liu, Y. Ma, P. Chen, Q. Li, X. Zhang, J. Yang and L. Zhang, A zwitterionic-aromatic motif-based ionic skin for highly biocompatible and glucose-responsive sensor, J. Colloid Interface Sci., 2021, 600, 561–571 CrossRef CAS PubMed.
|
This journal is © Institute of Process Engineering of CAS 2023 |
Click here to see how this site uses Cookies. View our privacy policy here.