DOI:
10.1039/D3QO01010D
(Review Article)
Org. Chem. Front., 2023,
10, 4488-4515
Recent advances in three-component radical acylative difunctionalization of unsaturated carbon–carbon bonds
Received
3rd July 2023
, Accepted 3rd August 2023
First published on 8th August 2023
Abstract
The radical acylated difunctionalization of unsaturated carbon–carbon bonds, which introduces acyl and other groups simultaneously, represents an attractive approach for the synthesis of functionalized ketones or related derivatives from easily accessible feedstocks. A wide range of acylation reagents, including carbazates, α-keto acid, acyl-1,4-dihydropyridines, aldehydes, oxime esters, oxime oxalate, and carboxylic acid derivatives, etc., can be used to incorporate acyl groups into olefins, dienes, alkynes, 1,3-enynes, cyclopropanes, and allenes. This review highlights the recent advances in this topic and focuses on the mechanistic insights of these transformations.
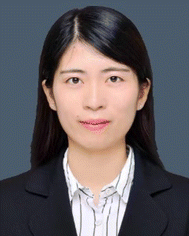 Jiaqiong Sun | Jiaqiong Sun was born in Henan, China in 1990. She received her BS-PhD dual degree in organic chemistry from Northeast Normal University (NENU) under the supervision of Professor Qian Zhang in 2018. She worked as an assistant research fellow at Shaanxi Normal University from 2018 to 2021 and joined NENU in 2021 as a lecturer. Her research interests focus on visible-light mediated transformations and green organic synthesis. |
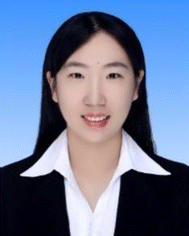 Lihong Wang | Lihong Wang was born in Henan, China in 1990. She received her B.S. degree from the College of Chemistry and Chemical Engineering, Anyang Normal University, in 2015. Then, she obtained her M.S. degree from the Faculty of Chemistry, Northeast Normal University (NENU), in 2019, under the guidance of Prof. Qian Zhang and Yan Li. Afterward, she obtained her Ph.D. degree from the Faculty of Chemistry, NENU, in 2023, under the supervision of Prof. Qian Zhang and Guangfan Zheng. Her research interests focus on NHCs/PC dual-catalyzed transformations. |
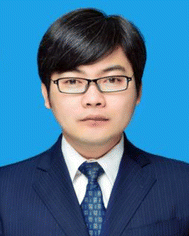 Guangfan Zheng | Guangfan Zheng was born in 1988 in Jilin, China. He received his B.S. degree from Jilin University, in 2010. He received his Ph.D. degree from the Northeast Normal University (NENU) under the guidance of Prof. Qian Zhang and Prof. Haizhu Sun in 2017. From 2017 to 2018, he worked at the Dalian Institute of Chemical Physics; from 2018 to 2020, he worked as an associate research fellow at Shaanxi Normal University. He joined NENU in 2020 as an associate professor and his research interests focus on NHCs-catalyzed cascade transformation, visible light catalysis, and asymmetric catalysis. |
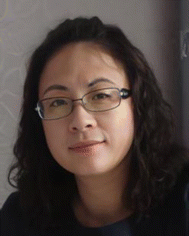 Qian Zhang | Prof. Qian Zhang received her BS and MS degrees from Northeast Normal University (1993 and 1996). She obtained her PhD degree (2003) from Changchun Institute of Applied Chemistry, Chinese Academy of Sciences, under the supervision of Professor Lixiang Wang in the research field of organic light emitting materials, especially for designing hole transporting materials. She has worked in Northeast Normal University since 1996, progressing through the ranks as assistant, lecturer, associate professor, and professor. Her research interests focus on the development of novel reactions, new reagents and strategies for organic synthesis. |
1. Introduction
The direct functionalization of unsaturated carbon–carbon bonds is the most fundamental and significant transformation in organic synthesis and has become a well-studied hot research topic. In particular, the radical-mediated1 difunctionalization strategy has received increasing attention due to its high reactivity, broad functional group tolerance, and high atom and step economy to access molecular complexity.2–7 Radical acylated difunctionalization of unsaturated carbon–carbon bonds can simultaneously introduce an acyl group and other groups in one step, providing straightforward access to ketones or related derivatives. The acyl radical is considered to be a transient nucleophilic σ-radical.8 Since the pioneering work of Kharasch in 1949,9 significant progress has been achieved in the acyl radical addition-initiated10–14 three-component difunctionalization of olefins to provide the β-acylation product. More recently, the use of transition metals (TM) in radical acylation has brought about a new Dawn for the α-acylative difunctionalization of the unsaturated carbon–carbon bond. The recently developed photoredox catalysis (PC) catalyzed, N-heterocyclic carbenes (NHCs) catalyzed, or NHCs/PC dual catalyzed strategies have injected new vitality into radical acylative difunctionalization chemistry, leading to the resurgence of the α-acylation15 reaction.
Based on the mechanism for the formation of C–C(acyl) bond, intermolecular acylative difunctionalization can be categorized into four different classes (Scheme 1): (1) acyl radical addition to carbon–carbon multiple-bonds followed by functionalization, such as functional group transfer, radical-polar cross-over etc.; (2) radical addition to carbon–carbon multiple-bonds generating alkyl/vinyl radicals, trapped by a TM-acyl species to perform reductive elimination; (3) PC catalyzed radical addition/single-electron-transfer (SET) reduction/electrophilic acylation cascade; and (4) the cross-coupling of the NHC stabilized acyl radical with the in situ generated carbon radical. The first class provides the β-acylated difunctionalization products, while classes 2–4 deliver α-acylated ones. A wide range of acylation reagents, including carbazates, α-keto acid, acyl-1,4-dihydropyridines, aldehydes, oxime esters, oxime oxalate, and carboxylic acid derivatives, etc. (Fig. 1), can deliver the acyl group selectively to the α- or β-position of unsaturated carbon–carbon bonds, providing various acylative difunctionalization products.
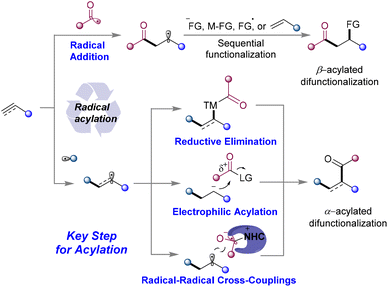 |
| Scheme 1 Representative strategies for radical acylated difunctionalization of carbon–carbon multi bonds. | |
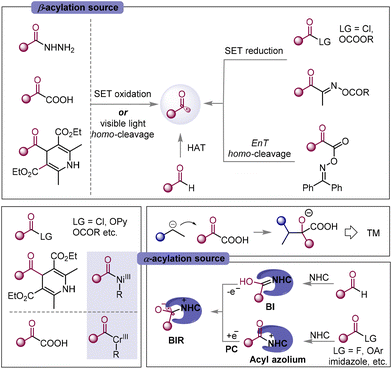 |
| Fig. 1 Representative acylation sources for three-component radical acylative difunctionalization. | |
This review offers a summary of the state-of-the-art progress in the field of radical-mediated simultaneous introduction of acyl and other groups to unsaturated carbon–carbon bonds, emphasizing the working models. The radical carbonylation (CO as C1 source)16 and radical-polar cross-over carboxylation (CO2 as C1 source)17–19 systems will not be discussed in this review.
2. Three-component radical β-acylated difunctionalization of olefins
As highly active nucleophilic radicals, acyl radicals can rapidly add to Michael olefins or styrene regioselectively, producing alkyl radical species. Then, the following radical-polar cross-over, reductive elimination of in situ generated high valent transition metal complex, functional group transfer, or addition to another unsaturated hydrocarbon, can provide the three-component acylative difunctionalization products.
2.1. Three-component radical acylative oxygenation of olefins
Alkene carbonylative oxygenation is a powerful tool for direct access to value-added β-O-substituted ketones and esters via simultaneously introducing acyl and O-centered groups in one step. Air is an ideal green oxidant, which could also serve as an oxygenation source in radical chemistry. In 2010, Taniguchi and co-workers developed iron-catalyzed 1,2-hydroxy alkoxycarbonylation of alkenes by employing bench stable and readily available carbazates 2-2 as alkoxycarbonyl radical precursors with air as the oxidant and hydroxy source (Scheme 2).20 Mechanistic studies indicated that single electron transfer (SET) between the carbazate and in situ generated FeIII delivered the radical cation intermediates 2-4, which underwent deprotonation to generate an N-centered radical. Tandem SET, deprotonation, and release of N2 afforded methoxycarbonyl radicals 2-8, which rapidly added to olefins 2-1. The resultant alkyl radical species 2-9 were trapped by molecular oxygen to afford peroxyl radicals 2-10, followed by cleavage of O–O bonds and hydron-atom-transfer (HAT), delivering β-hydroxy esters 2-3.
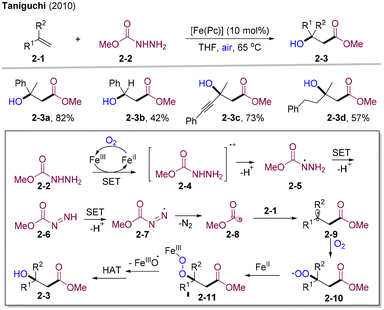 |
| Scheme 2 Fe-catalyzed radical alkoxycarbonyl oxygenation of olefins. | |
In 2019, Zhu and co-workers developed the copper-catalyzed radical 1,2-methoxy methoxycarbonylation of styrenes employing methyl formate 3-2 as both methoxycarbonyl and methoxy sources and tert-butyl peroxyacetate (TBPA) as the HAT reagent (Scheme 3).21 Various α-alkyl/aryl styrene compounds could be transformed into the corresponding β-methoxy alkanoates 3-3 in moderate to high yields. Interestingly, styrenes bearing intramolecular nucleophilic sites could undergo 1,2-methoxylation and subsequent nucleophilic cyclization, delivering tetrahydrofuran 3-3e, lactone, and pyrrolidine 3-3f. Mechanistically, Cu(I) could coordinate with alkenes to activate the C
C bonds. The tBuO˙ radical generated from the single electron transfer (SET) of Cu-cat. and TBPA could promote HAT of 3-2, delivering methoxycarbonyl radical 3-4. Radical addition of 3-4 to olefins activated by Cu(I) (3-5) afforded alkyl-Cu(II)-species 3-6, which were further trapped by 3-4 producing the Cu(III) intermediate 3-7. Decarbonylation and reductive elimination provide 1,2-methoxy methoxycarbonylation products 3-3.
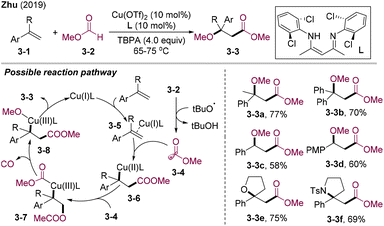 |
| Scheme 3 Cu-catalyzed 1,2-methoxy methoxycarbonylation of alkenes with methyl formate. | |
In 2021, the group of Hou and Li developed photocatalyst (PC) 4-CzIPN-catalyzed visible light-mediated radical difunctionalization of alkenes via a radical-polar cross-over strategy (Scheme 4).22 This methodology employing alkyl formates 3-2 as alkoxycarbonyl radical precursors and N-alkoxyazinium salt 4-3 as the HAT reagent provides diversified substituted alkanoates, including β-alkoxy 4-4, β-hydroxy 4-5, β-formyloxy 4-6, and β-dimethoxymethoxy alkanoates 4-7 under extremely mild reaction conditions.
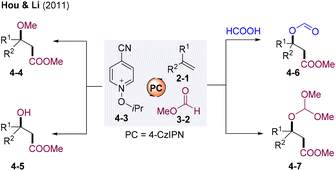 |
| Scheme 4 PC-catalyzed divergent alkoxycarbonyl difunctionalization of olefins. | |
Aldehydes could provide acyl radical species through HAT and underwent radical addition to C
C bonds resulting in alkyl radicals,11 which could be trapped by peroxyl radicals delivering β-peroxy ketones.
In 2011, Li and co-workers disclosed an elegant study on the iron-catalyzed three-component radical carbonylation-peroxidation cascade of alkenes, aldehydes, and hydroperoxides, leading to the straightforward construction of β-peroxy ketone products 5-3 (Scheme 5A).23 Interestingly, 1,8-diazabicyclo[5.4.0]undec-7-ene (DBU) could efficiently catalyze epoxidation, providing epoxides 5-4 in a one-pot process. Mechanistically, tBuOOH underwent the SET process with Fe(II) delivering tBuO˙ and Fe(III), which could oxidize THBP affording tBuOO˙. The alkyloxy radical abstracts the hydron atom of aldehydes and gives acyl radical 5-7. Subsequent radical addition to carbon–carbon double bonds followed by selective radical–radical cross-coupling with tBuOO˙ leads to 5-3. The β-peroxy ketone could undergo epoxidation to synthesize α-carbonyl epoxides 5-4. In 2012,24 the same group extended this carbonylation-peroxidation system to the α,β-unsaturated esters (Scheme 5B). The nucleophilic nature of the acyl radical caused regioselective radical addition to the β-site of α,β-unsaturated esters 5-5. Similarly, the Wang group25 disclosed dirhodium(II)-catalyzed transformations in 2017 (Scheme 5C). Tetra-n-butylammonium bromide (TBAB) also proved to be a suitable catalyst (Scheme 5D).26 Photocatalysis could promote a similar transformation under extremely mild conditions. Indole-3-carbaldehyde was employed in Zhu's system, leading to diversified β-peroxy-indole-ketones (Scheme 5E).27 TBHP could produce tBuO˙ via homolysis of O–O bonds under thermal conditions. The Li group28 and Lu group29 developed a catalytic amount of base-promoted epoxy acylation of olefins (Scheme 5F and G). In 2015, the group of Wang30 disclosed PC-catalyzed epoxy acylation of olefins (Scheme 5H).
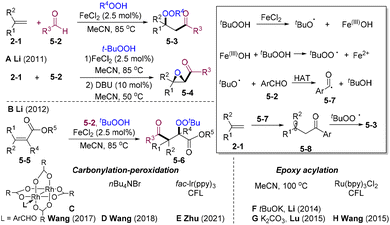 |
| Scheme 5 Carbonylation-peroxidation cascade of alkenes, aldehydes, and hydroperoxides. | |
In 2015, the Chen group disclosed the VO-catalyzed chemo-divergent β-hydroxylation– and β-peroxidation–carbonylation cascade of styrene (Scheme 6).31 Mechanistically, similar SET between vanadyl species 6-5 and THBP may occur, providing tBuO˙ and tBuOO˙ radicals. tBuO˙ mediates the HAT of aldehydes 5-2 affording the acyl radical 6-8, which is trapped by olefins providing benzyl radical species 6-9. When employing VOCl2 as the catalyst, radical–radical cross-coupling of benzyl and peroxyl radicals may occur, delivering the final β-peroxidation–carbonylation products 6-4. When switching the catalyst to VO(acac)2, vanadyl(V) hydroxide could act as an OH source (6-10) leading to carbonylative hydroxylation products 6-3.
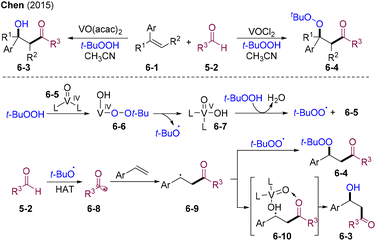 |
| Scheme 6 Chemo-divergent β-hydroxylation- and β-peroxidation-carbonylation cascade of styrene. | |
In 2018, Salles and co-workers developed a visible light-mediated methylene blue (MB)/persulfate system in water for the epoxy-acylation of styrene (Scheme 7).32 For non-conjugated olefins, hydroacylation products were observed. Potassium persulfate could release the hydroperoxide anion in water under alkaline conditions. The excited state MB* could undergo a reductive quenching process with hydroperoxide anion delivering the hydroperoxyl radical, which could mediate the HAA of benzaldehyde and also serve as a hydroperoxyl source to fulfil radical–radical cross-coupling delivering 7-4. Intramolecular epoxylation might occur to furnish the final products 7-3.
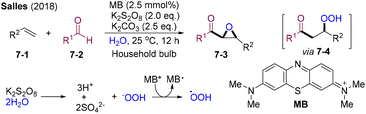 |
| Scheme 7 PC-catalyzed epoxy-acylation of styrene in water. | |
2.2. Three-component radical acylative amination of olefins
β-Amino ketone derivatives have found widespread applications in biologically active molecules,33,34 organic synthesis, and materials science. Therefore, the synthesis of β-amino ketones has received increasing attention. Direct acylative amination of olefins is one of the most straightforward routes to access β-amino ketones from readily available starting materials in one operation.
In 2019, Wu and co-workers developed visible light-mediated radical 1,2-acylative amination of styrenes (Scheme 8A).35 Oxime esters 8-1 could decompose into three components catalyzed by fac-Ir(ppy)3 to participate in this cascade functionalization delivering acylative amination products 8-3 with high atom and step economy. This strategy exhibits broad substrate scope and good functional group tolerance for both styrenes, oxime esters, and alkyl nitrile solvents. Mechanistically, excited Ir(III)* undergoes an oxidative quenching process with oxime esters 8-1 to promote fragmentation, providing acyl radical 8-4, acetonitrile, carboxylic anions 8-5, and Ir(IV). The acyl radical 8-4 was added to aryl olefins and subsequently oxidized by Ir(IV), delivering benzyl cation intermediate 8-7 and Ir(III), finishing the photoredox cycle. The nucleophilic N atom of the acetonitrile attacked 8-7 and underwent carboxylic anion-participated Mumm rearrangement to provide β-acyl substituted imide 8-3. In 2020, Klussmann et al. developed PC-catalyzed radical acylation and nucleophilic addition cascade, in which they disclosed similar transformations via the HAT process (Scheme 8B).36 When employing tertiary pivaldehyde as an acyl radical source, decarbonylation of acyl radicals occurred, delivering alkylative amination products 8-11c in 53% yield.
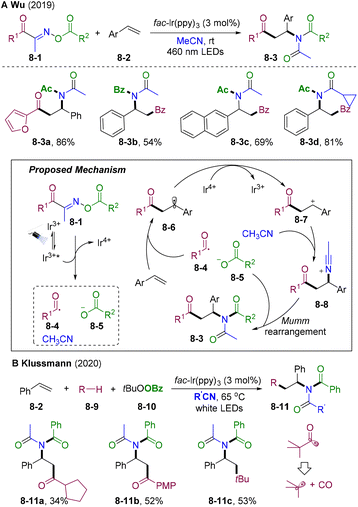 |
| Scheme 8 Visible light-mediated radical 1,2-acylative amination of styrenes via Mumm rearrangement. | |
In 2022, the group of Xia developed visible light-mediated radical alkoxycabonyl-imidation and carbonyl-imidation of olefins via alkyl and iminyl radical cross-couplings (Scheme 9A).37 The designed simple and easy-to-synthesize oxime oxalate and oxime phenylglyoxylate 9-1 were employed as bifunctional reagents that underwent addition to various kinds of olefines providing β-amino acid ester and β-amino ketone derivatives. A plausible mechanism was proposed in Scheme 9. Initially, the excited triplet Ir* underwent energy transfer (EnT) with oxime oxalate 9-1 to afford the excited state 9-1*, which undergoes subsequent cleavage of the N–O bond and decarboxylation, resulting in acyl radical 6-8 and iminyl radical 9-3. The transient acyl radical 6-8 was quickly added to olefins affording alkyl radicals 9-4, which were trapped by persistent iminyl radicals 9-3 to provide the final products. Soon after, Lin and co-workers developed photoinduced thioxanthone-catalyzed carboimination of alkenes, providing operatively simple and cost-effective access to β-amino acid and β-amino ketone motifs (Scheme 9B).38
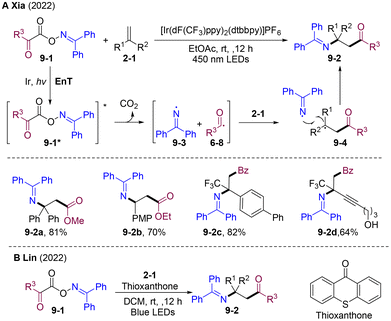 |
| Scheme 9 Visible light-mediated alkoxycarbonyl-imidation and carbonyl-imidation of olefins via radical–radical cross-couplings. | |
Organic azides have attracted significant attention owing to their unique applications in organic synthesis, material science, and click chemistry. In 2018, Wang and co-workers described a (salen)Mn(III)-catalyzed radical acyl-azidation of olefins (Scheme 10A). Readily available aldehydes and sodium azide were employed as acylation and azidation sources and iodosobenzene was used as an oxidant.39 This acyl-azidation tolerates various aryl olefins, including styrene, α-substituted styrene, β-substituted styrene, and α,β-unsaturated amides in mild conditions. By employing Jacobsen's chiral catalyst 10-5, moderate enantioselectivity was observed, which indicates the (salen)Mn(III) participates in C–N3 bond formation. Mechanistically, (salen)Mn(III)–N310-6 generated from ligand exchange was oxidized by PhIO affording the oxomanganese(V) intermediate 10-7, which undergoes HAT with the aldehyde to form the acyl radical and 10-8. Addition of the acyl radical resulted in the benzyl radical intermediate, which underwent azido-rebound delivering the β-azido ketones 10-3. In 2019, the Bao group developed iron-catalyzed acylative azidation of olefins employing TMSN3 as an azido source and aldehydes as acyl radical precursors (Scheme 10B).40 The obtained β-azido ketones 10-11 could easily be transformed into value-added N-containing compounds.
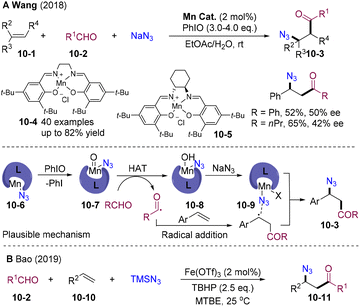 |
| Scheme 10 Radical acyl-azidation of olefins. | |
2.3. Three-component radical acyl-halogenation of olefins
Acyl-halogenation of olefins provides direct access to value-added β-halogenated ketones. Generally, the acyl radical trapped by C
C bonds generates alkyl radicals that undergo halogen atom transfer or radical-polar cross-over, delivering the final products.
In 2014, the Duan group developed silver-catalyzed acyl fluorination of olefins employing α-oxocarboxylic acids as the acyl radical precursor and Selectfluor as the oxidant and fluorination reagent (Scheme 11A).41 A wide range of styrenes and α-oxocarboxylic acid proved to be valuable substrates under mild conditions and aqueous media, leading to the formation of β-fluorinated 3-aryl ketones 11-3. However, aliphatic alkenes were not compatible (11-3d). The authors carried out control experiments and found the AgNO3/K2S2O8/KF system failed to give the desired products, indicating that other activated species might exist beyond the Ag(II)-intermediate. Mechanistically, Selectfluor oxidates the Ag catalyst, generating the Ag(III) species. Oxocarboxylic acid 11-2 undergoes SET with Ag(III) and decarboxylation offers nucleophilic acyl radicals 11-4 and then trapped by aryl olefins delivering alkyl radicals 11-5. The fluorine atom transfers from Ag(II)F to the benzyl radical delivering β-fluorinated ketones 11-3.
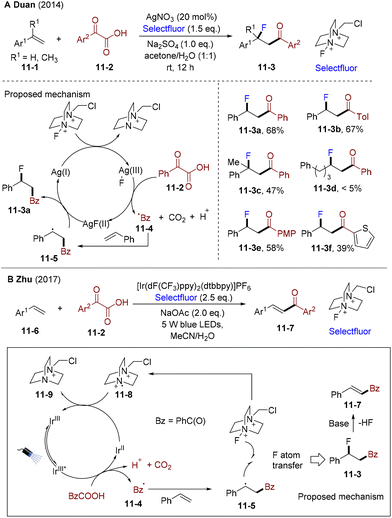 |
| Scheme 11 Radical acylfluorination and C–H acylation of olefins. | |
In 2017, Zhu and co-workers developed a base-promoted PC-catalyzed acylfluorination-defluorination cascade of aryl olefins with α-oxocarboxylic acid and Selectfluor (Scheme 11B). By employing Ir[dF(CF3)ppy]2(dtbbpy)PF6 as the photocatalyst and NaOAc as the base, diverse α,β-unsaturated ketones 11-7 were synthesized with high efficiency.42 The authors found that without the base, a small amount of the acyl-fluorination products was obtained, which could further be transformed into α,β-unsaturated ketones in the presence of NaOAc. Mechanistically, the excited state of PC could undergo a reductive quenching process leading to the formation of benzoyl radical 11-4 and Ir(II). The addition of the benzoyl radical, followed by the fluorine atom transfer from Selectfluor, formed the corresponding β-fluorinated 3-aryl ketones 11-3 and radical cation 11-8. Subsequently, 11-8 could oxidate Ir(II), finishing the catalytic cycle. 11-3 undergo elimination of HF assisted by NaOAc, providing α,β-unsaturated ketones 11-7.
In 2020, Oh and co-workers developed visible-light mediated PC-catalyzed chloroacylation of alkenes (Scheme 12).43 Acid chlorides 12-2 served as both acyl and chlorine sources, leading to the direct construction of β-chloroketone 12-3 under extremely mild conditions. In contrast to the previous fluorination system, the C–Cl bond was constructed via nucleophilic attack of the chlorine anion to benzyl carbon cations 12-4. The employment of insoluble KHCO3 could efficiently inhibit the elimination of the chlorine anion.
 |
| Scheme 12 Radical chloroacylation of olefins. | |
2.4. Three-component radical carbo-acylation of olefins
2.4.1 Radical aryl-acylation of olefins.
The radical-polar cross-over strategy can consecutively introduce radicals and nucleophiles into carbon–carbon double bonds in one step, providing attractive alternatives for olefin difunctionalization. N-Alkyl indoles could serve as efficient nucleophiles,44 providing C3-alkylated indoles. In 2020, the group of Klussmann developed the HAT-mediated three-component radical acylative arylation of olefins by employing N-alkyl indoles as an arylation source and aldehyde as an acyl radical precursor (Scheme 13).36 Various aryl/alkyl-aldehyde 5-2 and α-alkyl substituted styrenes 13-1 were tolerated, providing β-indolyl ketones 13-4 in good to excellent yields. Electron-donating styrenes exhibited increased reactivity, which could be attributed to the stability of the carbocation intermediate. When employing pivaldehyde as an acyl radical source, the decarbonylated alkyl-arylation product was observed, suggesting the possible involvement of acyl radicals. Stern–Volmer studies indicate that the excited state of PC* was preferred to be quenched by tBuOOBz. Mechanistically, this reaction proceeded via the oxidative quenching process of IrIII* by tBuOOBz, which gave the IrIV and tBuO˙ radical, which then underwent HAT to form the acyl radical. Consecutive radical addition and SET by IrIV generated a carbocation intermediate, which is trapped by the nucleophilic C3 site of N-alkyl indoles delivering β-indolyl ketones 13-4.
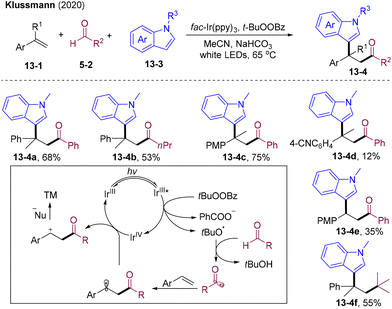 |
| Scheme 13 Radical aryl-acylation of olefins via PC-catalyzed radial-polar cross-over. | |
2.4.2 Radical acyl-cyanation of olefins.
The cyano group is not only prevalent in natural products and biologically active molecules, but also serves as a versatile building block in organic synthesis. Therefore, the direct introduction of the cyano group into easily accessible feedstocks is significant. 1,2-Acyl-cyanation of alkenes could provide β-cyano ketones in one step and has attracted great attention recently.
In 2019, the group of Bao disclosed the first HAT-mediated radical 1,2-acyl-cyanation of alkenes employing earth-abundant copper salt as a catalyst and TBHP as the radical initiator (Scheme 14).45 A wide range of aromatic/aliphatic aldehydes 14-2 and TMSCN could react with styrenes efficiently, providing structurally diversified β-cyano ketones 14-3 under mild conditions. Based on the mechanistic investigations and DFT studies, the proposed catalytic cycle is shown as Scheme 14. Initially, Cu(I) and TBHP undergo SET providing 14-4 and a tBuO˙ radical. HAT by alkyloxy radicals might occur, generating acyl radicals that are trapped by olefins to form benzyl radicals 14-6. Ligand exchange of Cu(II)–OH with TMSCN offered the Cu(II)–CN species 14-5, which underwent outer-sphere CN group transfer with the benzyl radical (via14-7) delivering β-cyano ketones 14-3.
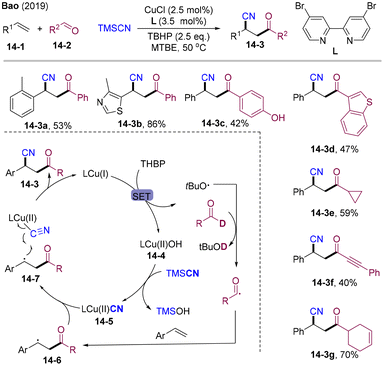 |
| Scheme 14 Copper-catalyzed radical acyl-cyanation of olefins via HAT. | |
In 2022, Cao and co-workers developed electrocatalytic three-component acyl-cyanation and amino-cyanation of olefins (Scheme 15).46 Both aryl and aliphatic olefins were tolerated, affording β-cyano ketones in moderate to high yields (30 examples, up to 81% yields). This strategy successfully extended the scope of the acyl-cyanation system to aliphatic olefins. Mechanistically, α-oxocarboxylic acid 15-3 oxidized to acyl radical 15-6via anodic oxidation and decarboxylation. Alkyl radical 15-7 generated by acyl radical addition could be trapped by CBX 15-2, affording acyl-cyanation product 15-4 and hypervalent iodine radical 15-8. Cathodic reduction might occur, generating a 2-iodobenzoic acid anion 15-9.
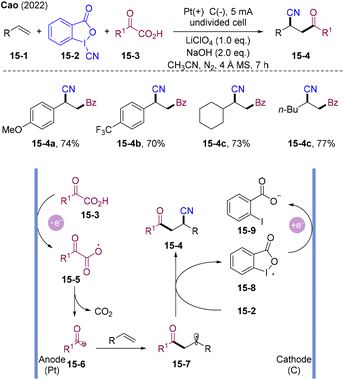 |
| Scheme 15 Electrocatalytic intermolecular acyl-cyanation of olefins. | |
Radical-mediated enantioselective acylative difunctionalization of olefins could be an attractive alternative for the construction of chiral ketones; however, despite this significance, it is still largely underdeveloped. In 2021, the group of Chen and Xiao developed the asymmetric three-component radical dicarbofunctionalization (DCF) of olefins employing copper and photoredox dual catalysis (Scheme 16).47 A wide range of redox-active oxime derivatives (16-2, 16-4) and aryl olefins could couple with TMSCN with high enantioselectivity. Extremely mild conditions and broad functional group tolerance allowed for late-strategy functionalization of natural products and biologically active molecules such as estrone (16-3g), febuxostat, and the amino acid derivative, highlighting the synthetical potential. This strategy provides a modularized platform for optically active β-cyano ketones 16-3 and alkyldinitriles 16-5 (>60 examples, up to 86% yield & 97% ee).
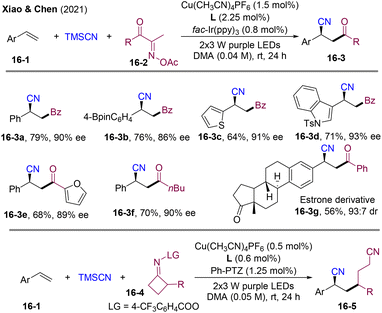 |
| Scheme 16 PC/Cu-dual catalyzed asymmetric three-component radical dicarbofunctionalization olefin. | |
Mechanistically (Scheme 17), oxidative quenching of excited Ir*-cat. by oxime esters 16-2 generates iminyl radical 16-6 and oxidated state PC with the release of the carboxylic anion. 16-6 undergoes radical β-carbon-elimination to form acyl radical 16-7 with the release of acetonitrile. Benzylic radicals 16-8 could be generated via regioselective addition of the acyl radical to C
C bonds, which are trapped by the Cu(II)–CN species affording the Cu(III) intermediate 16-9. Reductive elimination of the chiral high-valent Cu(III) complex 16-9 affords coupled products 16-3 and regenerates Cu(I), finishing the Cu catalysis cycle. Cu(I) species could be oxidated by the oxidative state of PC to form Cu(II) and regenerate ground state PC. However, the outer-sphere CN group transfer pathway could not be excluded. For cycloketone oxime esters 16-4, β-carbon elimination of iminyl radicals delivers the alkyl radical attached to the remote CN group (16-10), which participates in the cascade transformations.
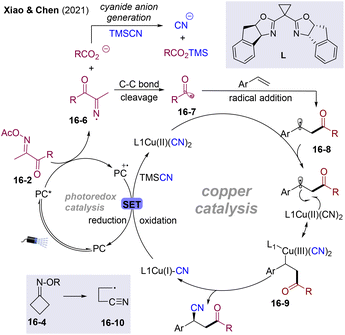 |
| Scheme 17 Proposed mechanism for asymmetric three-component dicarbofunctionalization. | |
2.4.3 Radical acyl-alkylation of olefins employing olefins as alkylation reagents.
Olefins are efficient radical acceptors; therefore, by rational design, in situ generated alkyl radicals could be trapped by another double bond, realizing the difunctionalization. However, this strategy requires significant kinetic differences in the rate of free radical addition to two different olefins to achieve selectivity. Early in 2003, the Hosomi and Ryu group developed a cascade carbonylation via the sequential addition of acyl radicals to electron-deficient alkenes and tin enolates, leading to direct access to 1,5-diketones.48 As nucleophilic carbon-centered radicals, acyl radicals react faster with electron-deficient olefins than aliphatic olefins and styrene.
In 2017, Ryu and co-workers developed bromine radical-mediated coupling of aldehydes with electron-deficient allyl bromides via a radical chain mechanism.49 Interestingly, this strategy could be extended to the three-component coupling of aldehydes 18-1, electron-deficient alkenes 18-2, and allyl bromides 18-3 (Scheme 18). Initially, bromine radicals could be generated from the reaction of AIBN and allyl bromides, which undergo HAA with aldehydes offering acyl radicals. Acyl radical preferentially added to electron-deficient olefins generating alkyl radicals. Subsequent addition to allyl bromides and elimination of bromine radicals delivered the acyl-allylation products 18-4.
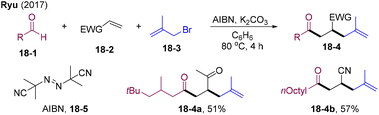 |
| Scheme 18 Three-component radical acyl-allylation of electron-deficient olefins. | |
In 2017, the group of Wallentin disclosed visible-light-mediated radical cascade reactions of carboxylic acids 19-1, electron-deficient alkenes 19-2, and silylenolether 19-3 (Scheme 19).50In situ generated anhydride 19-5 (carboxylic acids activated by DMDC) served as acyl radical precursors under visible light irradiation. Electron-rich aryl carboxylic acids exhibited higher reactivity; however, strong electron-withdrawing group substituted aryl carboxylic acids were unsuitable for this transformation. The reaction scope for electron-deficient alkenes and silylenolether was also broad, providing structurally diversified β-functionalized δ-diketones. The acyl radical generated from the oxidative quenching process of PC* with anhydride 19-5 initiates sequential radical addition to form the desired products. Polar matching effects contribute to the excellent chemo-selectivity control.
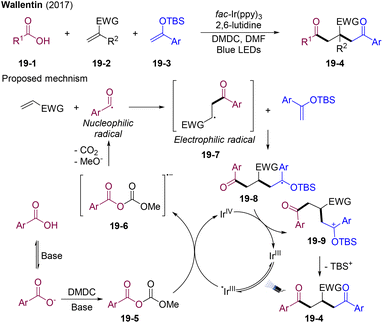 |
| Scheme 19 Three-component radical acyl-alkylation of olefins for δ-diketones. | |
In 2019, Yang and co-workers disclosed the Fe-catalyzed four-component radical coupling of two different olefins with aldehydes and THBP (Scheme 20).51 Aromatic and aliphatic aldehydes were all compatible with this reaction system. In situ generated acyl radicals 20-6 preferentially added to electron-deficient olefins 20-3 affording α-EWG substituted radicals 20-7, which underwent selective addition to aryl olefins 20-1 and were trapped by peroxyl radicals delivering peroxide intermediate 20-9. DBU-promoted Kornblum–DeLaMare rearrangement of the peroxide provided β-functionalized δ-diketones 20-5. This approach efficiently recognized acyl radical addition between the Michael acceptor and styrene.
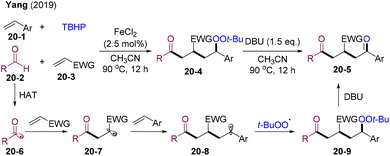 |
| Scheme 20 Fe-catalyzed four-component radical coupling of two different olefins with aldehydes and THBP. | |
3. Transition metal-catalyzed radical α-acylated difunctionalization
The cooperation of transition metals with radical acylation could forge a C–C (acyl) bond, which provides an opportunity for α-acylated difunctionalization of the olefin. Generally, regioselective addition of radicals to C–C double bonds deliver an alkyl radical, which is trapped by the M-acyl species to afford high valent alkyl-M-acyl species. Subsequent reductive elimination could deliver α-acylated difunctionalization products. Alternatively, high valent TM species could also be generated from the coupling of alkyl-M species with acyl radicals.
3.1. Ni-catalyzed reductive 1,2-alkylacylation of olefins
Alkyl radicals could be generated via single electron transfer of alkyl halides, N-(acyloxy)phthalimides (NHPI esters), etc. Highly active electrophilic fluoroalkyl radicals could add to various olefins; on the other hand, for nucleophilic alkyl radicals, electron-deficient olefins or styrene were required to achieve the reactivity and selectivity.
In 2018, Chu and co-workers disclosed the nickel-catalyzed three-component reductive perfluoroalkyl-acylation of olefins via a radical relay process (Scheme 21).52 Various aliphatic olefins 21-1 with different directing groups, including esters, amides, carbamates, carbonates, phosphates, and sulfonates, undergo a reductive relay reaction with good to excellent yields. Aryl vinyl ethers proved to be a valuable substrate. Aryl, heteroaryl, and alkyl acyl chloride were well tolerated. A series of perfluoroalkyl iodides, including ethyl iododifluoroacetate, could smoothly be introduced into carbon–carbon double bonds. The pendant chelating group could modulate the reactivity and selectivity. By employing this strategy, challenging selective alkene difunctionalization in the presence of multiple double bonds could be achieved, which further highlights the importance of transformations (21-4j, 21-4k). Mechanistically, in situ generated Ni(0) underwent oxidative addition with acyl chloride providing intermediate 21-5. The perfluoroalkyl radical originating from the SET process between RfI and Ni(0) or Ni(I) was trapped by the olefin, delivering the alkyl radical species 21-6. Coupling of 21-5 and 21-6 yielded chelating-stabilized Ni(III) intermediate 21-7, which underwent reductive elimination providing 21-4 and Ni(I). Ni(I) could be reduced by Mn(0), finishing the catalytic cycle. However, the coupling of alkyl radicals 21-6 and Ni(0), followed by oxidative addition by acyl chloride and sequence reductive elimination pathway, could not be excluded.
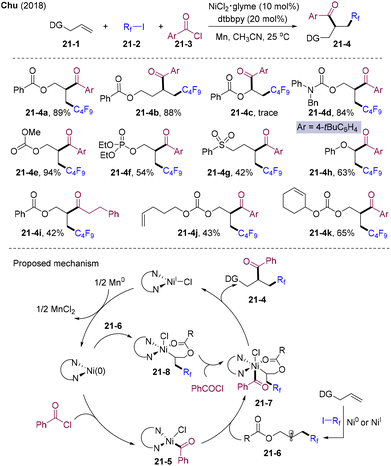 |
| Scheme 21 Ni-catalyzed reductive 1,2-perfluoroalkylacylation of olefins. | |
In 2020, the Wang group established Ni-catalyzed three-component reductive radical 1,2-alkylacylation of electron-deficient alkenes 22-1 employing tertiary alkyl bromides 22-2 as alkyl radical precursors and acid anhydrides 22-3 as acylation sources (Scheme 22A).53 Under mild reductive conditions, an array of activated alkenes, including α,β-unsaturated carbonyl compounds, acrylonitrile, and electron-deficient aryl-substituted styrenes could be transformed into structurally diversified ketones 22-4 (>50 examples, up to 91% yield). The authors provided two possible catalytic pathways. The alkyl radical was generated by the SET reduction of alkyl bromides 22-2 and underwent Giese addition to 22-1. The resulting alkyl radicals 22-7 were trapped by acyl-Ni(II) 22-5 originating from the oxidative addition of Ni(0) by 22-3, then underwent reductive elimination to provide the final products 22-4. Alternatively, α-EWG substituted alkyl radicals 22-7 could have underwent further single-electron-reduction affording carbon anion 22-8, providing target products 22-4 through tandem nucleophilic substitution.
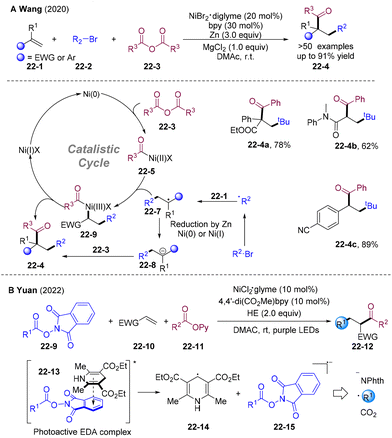 |
| Scheme 22 Ni-catalyzed three-component reductive 1,2-alkylacylation of olefins. | |
In 2022, the group of Yuan developed electron donor–acceptor (EDA) complex-enabled Ni-catalyzed reductive 1,2-alkylacylation of electro-deficient olefins employing N-(acyloxy)phthalimides (NHPI esters) 22-9 as the alkyl radical precursor and 2-pyridyl esters 22-11 as acyl donors (Scheme 22B).54 Acrylates, lactones, vinyl phosphates, and vinyl sulfones could efficiently transform into the desired products. Particularly, unprotected acrylamides were also tolerated. This reductive radical relay process avoids additional photocatalysts and metal reductants, delivering 1,3-dicarbonyls with good functional group tolerance and broad substrate scope. Hantzsch ester (HE) interacts with the NHPI ester to form a photoactive EDA complex 22-13. Irradiation by visible light promotes the decomposition of the EDA complex, generating alkyl radicals with the release of phthalimide anion and carbon dioxide, which undergo further transformations.
3.2. Transition metal-catalyzed 1,2-diacylation of olefins
1,4-Dicarbonyl compounds are important structural motifs prevalent in bioactive natural products,55 pharmaceutical molecules, and materials science. They also serve as an important intermediate in organic synthesis.56 Increasing attention has been paid to the direct 1,2-diacylation of olefins, which can deliver 1,4-dicarbonyl compounds57 from easily accessible starting materials in one step.
In 2020, the Xia group developed visible light-mediated chemo-divergent hydroacylation and diacylation of styrene employing 4-acyl-1,4-dihydropyridines as acyl radical precursors (Scheme 23).58 The addition of the nickel catalyst could modulate the chemo-selectivity, leading to diacylation products. Mechanistically, irradiation of acyl-1,4-dihydropyridines 23-1 could generate dihydropyridine radicals 23-5 and acyl radicals. The addition of the acyl radical to styrene offers benzyl radical 23-7. Concurrently, acyl radical could be trapped by Ni(I), generating Ni(II)-acyl intermediate 23-6, which undergoes coupling with 23-7 and subsequent reductive eliminations providing diacylation products 23-4.
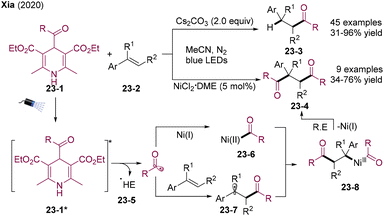 |
| Scheme 23 Visible-light mediated chemo-divergent hydroacylation and diacylation of alkenes. | |
In 2022, the Ackermann group presented an elegant carboacylation of alkene by employing nickel/PC dual-catalysis (Scheme 24).59 Sodium decatungstate was employed as the photocatalyst and HAT catalysis was used to activate the C–H bond of aldehydes/hydrocarbon under near-ultraviolet-light irradiation, generating corresponding acyl or alkyl radicals and [W]5−H+ (24-6). Disproportionation of 24-6 regenerates 24-5 and produces reducing [W]6−2H+ (24-7). Carbon-centered radicals are added to electron-deficient olefins and trapped by Ni(0) to give Ni(I)-alkyl intermediate 24-9. Oxidative addition and sequence C–C(acyl) reductive elimination delivered the final products 24-4 and Ni(I) (24-11). The SET process of 24-11 by 24-7 regenerates Ni(0), closing the Ni-catalytic cycle. This strategy provides straightforward access to diversified ketones from easily accessible and cost-effective olefins, acyl chlorides, and aldehydes/hydrocarbons. This transformation features mild conditions, easy operation, excellent selectivity, and good functional group tolerance.
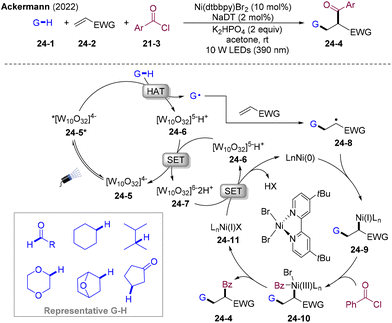 |
| Scheme 24 Visible-light mediated Ni-catalyzed carbo-acylation of alkenes via the HAT process. | |
Chromium can also mediate carbon–carbon(acyl) bond formation via reductive elimination. In 2022, Xiao and workers pioneered an elegant PC/Cr dual catalysis system for radical diacylation of olefins leading to value-added 1,4-diketones 25-3 (Scheme 25).60 Furthermore, the olefins could extend to dienes and vinyl cyclopropanes, generating 1,5-diketones 25-4 and 1,6-diketones 25-5. This methodology provides a general approach for the synthesis of 1,n-diketones. Mechanistically, the acyl radical could generate via reductive quenching of PC* with α-oxocarboxylate or direct visible light-promoted cleavage of α-keto acid 25-2. The acyl radical could add to olefins generating alkyl radical 25-7, which is trapped by CrII-acyl species 25-8 delivering CrIII intermediate 25-9. Reductive elimination delivered the final products 25-3. This PC/Cr dual catalysis could find broad applications in organo-chromium chemistry.
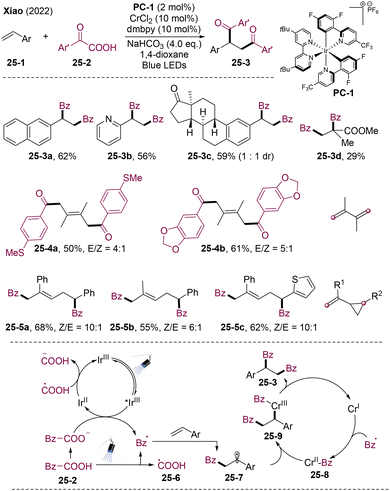 |
| Scheme 25 PC/Cr-dual catalyzed diacylation of olefins. | |
3.3. Ni-catalyzed three-component carboacylation of alkynes
Alkynes are fundamental feedstocks in organic synthesis and the selective introduction of two functional groups into alkyne multi-bonds has received increasing attention.61 Radical acylative difunctionalization of alkynes could provide direct access to functionalized enones; however, three-component acylative difunctionalization of alkynes remains a challenge due to the instability of alkenyl radicals.
In 2022, the group of Zhang and Tu developed the first Ni-catalyzed radical reductive 1,2-perfluoroalkylacylation of alkynes (Scheme 26).62 A broad range of terminal/internal aryl alkynes, fluoroalkyl iodides, and acyl chlorides was tolerated, delivering diversified perfluoroalkyl-incorporated enones 26-4 under mild conditions with excellent regio- and stereo-selectivity. SET of fluoroalkyl iodides 26-2 offers Rf radicals, which undergoes regioselective addition to alkynes producing vinyl radicals 26-6. Alternatively, the atom transfer radical addition reaction (ATRA) of fluoroalkyl iodides and aryl alkynes generates alkenyl iodine 26-5, which is reduced by Ni(I), offering vinyl radicals 26-6. Coupling of vinyl radicals and Ni(II)-acyl species 26-7 (originated from oxidative addition of Ni0 by acyl chlorides) produces Ni(III) intermediate 26-9 and subsequent reductive elimination delivers perfluoroalkyl-acylation products 26-4. However, the alternative pathway via oxidative addition of Ni(I)-vinyl species 26-8 by acyl chlorides could not be ruled out.
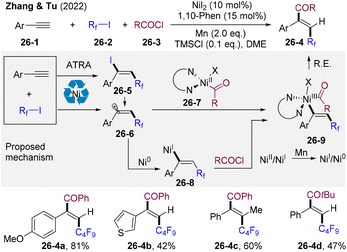 |
| Scheme 26 Ni-catalyzed perfluoroalkyl-acylation of alkynes. | |
4. Single PC-catalyzed radical α-acylated difunctionalization of olefins
Besides providing the β-acylated difunctionalization product, the α-keto acid could also serve as an electrophilic acylating agent to introduce the acyl group to the α-position of olefins. In 2021, the Wu group disclosed visible light-mediated PC-catalyzed 1,2-diacylation of alkenes employing α-keto acid as both the radical acylation and electrophilic acylating agent (Scheme 27A).63 The addition of N(nBu)4NO3 stabilizes the alkyl anion to inhibit the hydroacylation and activates the α-keto acid realizing α-acylation. A wide range of 1,4-diketones were assembled by varying olefins and α-keto acid. Olefins derived from natural products and bioactive molecules such as tigogenin, estrone, and cholesterol were tolerated. For unsymmetric 1,2-dicarbonylation, selectivity modulation remains a challenge. Wu and co-workers achieved a breakthrough by tuning the reductive and electrophilic ability of acyl precursors. By employing 4-carbamoyl-1,4-dihydropyridine 27-2 as an amide-carbonyl radical precursor, intermolecular unsymmetric 1,2-dicarbonylation was realized for the first time. 27-2 preferentially underwent SET with the excited state of PC*, generating amide-carbonyl radicals 27-6 and subsequent radical addition to double bonds delivered the alkyl radical species 27-7. SET reduction of the alkyl radical by strongly reductive PC˙− regenerates PC and alkyl anion 27-8, which undergo nucleophilic substitution with the more electrophilic α-keto acid, producing the unsymmetric 1,2-diacylation products. This photoredox-catalyzed radical-polar cross-over strategy opens a new avenue for α-acylated difunctionalization of olefins.
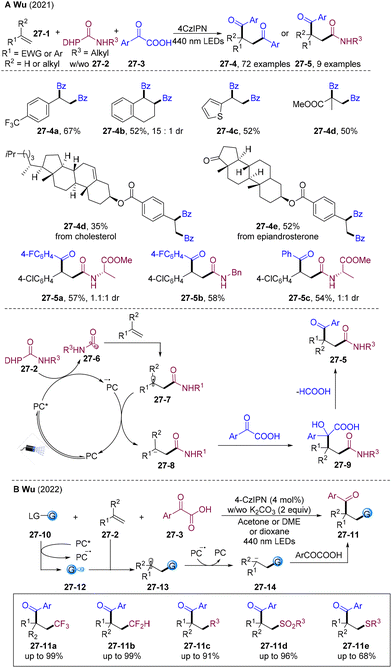 |
| Scheme 27 PC-catalyzed α-acylated difunctionalization of alkenes. | |
Later, the same group developed divergent α-acylated difunctionalization of olefins by introducing both acyl and fluoromethyl, alkyl, sulfonyl, or thioether groups via PC-catalyzed radical-polar cross-over (Scheme 27B).64 The kinetic inertness of the α-keto acid to the PC* leads to the selective reductive quenching process of PC* by kinetically favourable radical precursors to generate radical species 27-12. 27-12 added to olefins and the resulting alkyl radicals 27-13 undergo subsequent radical-polar cross-over realizing diversified α-acylated difunctionalization of olefins 27-11. The differences in the kinetics of radical generation by the reductive quenching process with different radical precursors results in excellent chemo- and regio-selectivity, which could provide an attractive platform for α-acylated difunctionalization of olefins.
5. NHCs-catalyzed radical α-acylated difunctionalization
Radical–radical cross-coupling65 is the chain termination process of the traditional radical chain mechanism, which proceeds via a near diffusion-controlled manner, features low activation barriers, and insensitivity to steric hindrance and electronic effects. However, the selectivity modulation remains a challenge. To achieve synthetically useful selectivity, transient radicals should be coupled with radicals that have entirely different stability according to the persistent radical effect (PRE),65 such as persistent radicals.
The acyl radical is a highly active nucleophilic transient radical, rarely employed in direct cross-couplings with other transient radicals. N-Heterocyclic carbene (NHC) catalysis could stabilize66 the acyl radical to a persistent ketyl radical, which could act as an acyl radical equivalent to participate in radical cross-couplings.67–73 Early in 1997, Fukuzumi and co-workers reported the SET oxidation of Breslow intermediate (28-1, BI) for NHCs-derived ketyl radicals 28-2 (Scheme 28A).74 In 2001, the Camps group disclosed the crystal structure of the free radical intermediate of pyruvate ferredoxin oxidoreductase.75 In 2008, the Studer group developed oxidative esterification of aldehydes employing NHC catalysis (Scheme 28B).76BI underwent two-step SET with (2,2,6,6-tetramethylpiperidin-1-yl)oxy radical (28-4, TEMPO) generating acyl azolium intermediate 28-9, which was subsequently substituted by the TEMPO anion delivering ester 28-5. This is the first radical-involved organic transformation catalyzed by NHCs. In 2014, the Chi group developed NHCs-catalyzed reductive homo-coupling of nitroethylenes 28-10 (Scheme 28C).77 BI undergoes SET with nitroethylenes 28-10 generating NHCs-attached ketyl radicals 28-13 and nitroalkene-derived radical anions 28-14, realizing reductive couplings. This approach represents the first example of generating carbon-cantered radicals via SET with BI, in which BI acted as a reductant. In 2015, Rehbein and co-workers re-evaluated NHCs-catalyzed benzoin condensation and proposed the involvement of radical species.78 In 2019, the group of Bertrand and Martin elucidated the persistent BI-derived radical species as key intermediates in oxidative NHC catalysis.79
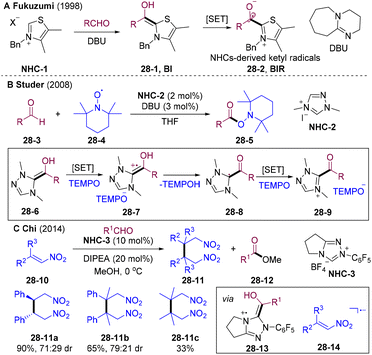 |
| Scheme 28 Pioneering studies based on NHC-stabilized acyl radicals. | |
In 2019, Ohmiya and co-workers achieved the main breakthrough in this area, and developed NHC-catalyzed decarboxylative coupling of NHPI esters8029-2 with aldehydes 29-1 (Scheme 29).81 Mechanistically, the reaction of NHC and aldehyde generated BI, which underwent deprotonation by bases delivering a highly reducing enolate form of BI. BI could be oxidized by redox-active esters 29-2 offering transient alkyl radicals 29-4 and persistent Breslow intermediate radicals (BIR). Radical–radical cross-coupling might occur and eliminate the NHC to deliver acylation products 29-3. This NHCs radical relay strategy constructs carbon–carbon (acyl) bonds via an unprecedented radical cross-coupling manner, opening a new avenue for radical acylation chemistry.
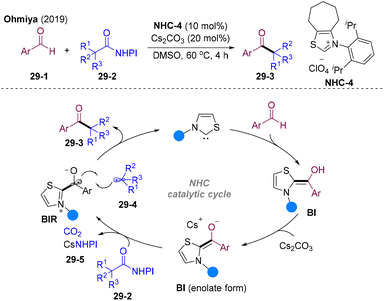 |
| Scheme 29 NHCs-catalyzed decarboxylative alkylation of aldehydes. | |
By reasonable design, transient radical and NHC-attached ketyl radicals could simultaneously be generated. Contributing to different properties, transient radicals may add to olefins, generating alkyl radicals that could be trapped by ketyl radicals delivering α-acylation products. This conceptually novel addition-trapping scenario provides a modular platform for α-acylated difunctionalization of carbon–carbon multi-bonds.
5.1. NHCs-catalyzed carbo-acylation of olefins
5.1.1 NHCs-catalyzed alkyl-acylation of olefins.
The highly reducing ability of BI (Ered ≈ −1.7)82 meant it could undergo SET with alkyl radical precursors delivering transient alkyl radicals and persistent NHC-attached ketyl radicals.81 In 2019, the Ohmiya and Nagao group realized state-of-the-art NHCs-catalyzed three-component radical alkylative acylation of olefins employing NHPI esters 30-3 as the alkyl radical precursors and aldehydes 30-2 as the acylating reagents under redox-neutral conditions (Scheme 30).83 The key to the success of this method is that the radical cross-coupling of tertiary alkyl radical with the ketyl radical was slower than the addition to styrene or electron-deficient olefins. Thus, this radical relay process was limited to tertiary alkyl radicals. For NHC-5, only aryl aldehydes were tolerated (Scheme 30A). By switching the catalyst to the newly designed thiazolium-type NHC-6, aliphatic aldehydes were suitable for decarboxylative radical coupling with NHPI ester and vicinal alkylacylation of styrenes (Scheme 30B).84 Mechanistically, SET of enolate BI and NHPI ester could generate tertiary alkyl radical 30-9 and NHC-attached ketyl radical 30-11. Radical addition of 30-9 to the olefin followed by alkyl radical 30-10 capture by ketyl radical 30-11 realized vicinal alkylacylation. This pioneering approach provides new insight into radical acylative difunctionalization of olefins.
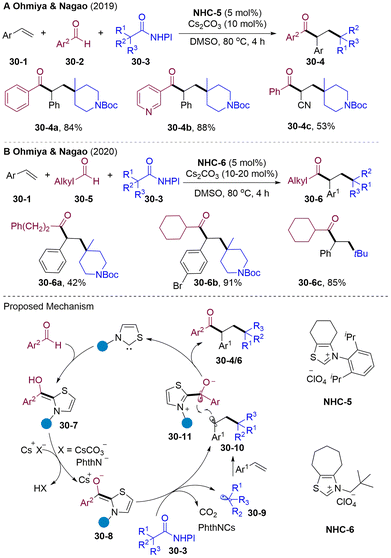 |
| Scheme 30 NHCs-catalyzed alkyl-acylation of olefins employing NHPI esters. | |
Katritzky pyridinium salts could be obtained from the condensation of primary amines with commercially available pyridium salts, regarded as attractive alternatives for the generation of alkyl radicals via SET reduction (Scheme 31).85 In 2020, the Hong group developed a cross-coupling of Katritzky salts 31-3 with aldehydes 31-2via an NHCs-catalyzed radical relay. Various secondary alkyl radicals generated from single-electron-reduction of Katritzky salts and rebounded with BIR generating functionalized ketones. Interestingly, this system could extend to the vicinal alkyl-acylation of olefins by introducing both secondary alkyl and acyl groups into double bonds under mild conditions.86
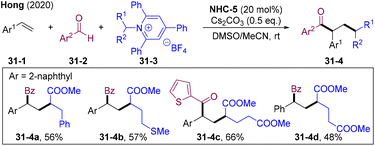 |
| Scheme 31 NHCs-catalyzed alkyl-acylation of olefins employing Katritzky pyridinium salts. | |
In 2021, the Guo87 group and Du88 group independently disclosed NHCs-catalyzed radical acyl-cyanoalkylation of olefins with cycloketone oxime esters 32-2/5 and aldehydes (Scheme 32). A wide range of primary and secondary alkyl radicals were generated via SET reduction of cycloketone oxime esters and underwent a radical addition-trapping cascade delivering value-added functionalized aliphatic keto nitrile cores 32-4. Interestingly, in Du's system addition of Mg(OTf)2 plays a critical role in reactivity. The author proposed a mechanism involving the EDA-complex 32-6 generated by the interaction of Mg2+ cation with cyclobutanone oxime ester 32-5 and enolate BI.
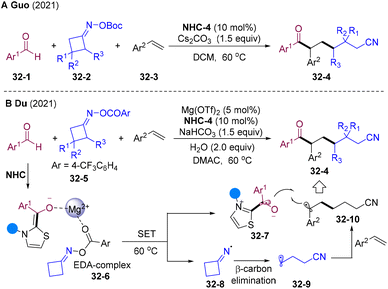 |
| Scheme 32 NHCs-catalyzed cyanoalkyl-acylation of olefins. | |
Aliphatic halides could also oxidize the BI, generating alkyl and ketyl radicals. The group of Ohmiya and Nagao developed alkylacylation89 and trichloromethylacyaltion90 of olefins employing tertiary α-bromocarbonyls 33-3 and tetrachloromethane 33-5 as radical sources via NHCs-catalyzed radical relay (Scheme 33).89,90 The radical addition rate of alkoxycarbonylalkyl radical to styrene is faster than the competing radical–radical cross-coupling rate, which causes excellent three-component selectivity. However, primary and secondary halides were not suitable for this cascade.
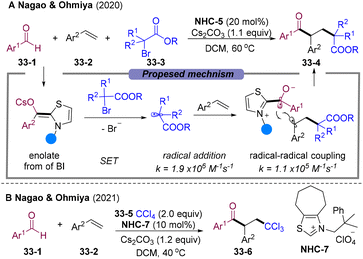 |
| Scheme 33 NHCs-catalyzed 1,2-alkyl-acylations of olefins employing tertiary halides. | |
The employment of simple and unactivated alkyl halides, especially for primary alkyl halides as an alkyl radical source, is challenging owing to their low reduction potential.91 In 2022, the Ye and Zhang group developed an elegant visible light-mediated NHCs/Pd dual-catalyzed radical alkylacylation of simple alkenes with unactivated alkyl halides 34-3 and aldehydes 34-2 under redox-neutral conditions (Scheme 34).92 The authors proposed that the radical cascade proceed via oxidative quenching of the excited state of Pd(0)-species by alkyl halides, affording the alkyl radical and Pd(I). Pd(I) underwent SET reduction by the BI generating NHC-attached ketyl radical and Pd(0), closing the photoredox cycle. 1,2-Alkylacylation might occur via an addition-trapping scenario. A wide range of simple alkyl halides (primary, secondary, and tertiary) and aromatic aldehydes could couple with olefins sequentially, providing functionalized ketones 34-4. By employing CHCl3 or CCl4 as the radical precursor, cascade cyclopropanation might occur, delivering otherwise difficult-to-access chlorocyclopropyl ketone 34-6.
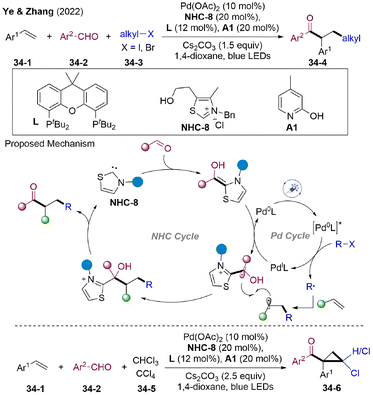 |
| Scheme 34 Visible light-mediated NHCs/Pd dual-catalyzed radical alkylacylation of simple alkenes. | |
Fluorinated ketones are prevalent motifs in bioactive molecules, drugs, and functional materials, and direct construction of these structures is in great demand.93 In 2020, the group of Li,94 Wang,95 and Wu96 independently developed 1,2-fuoroalkyl-acylations of alkenes employing electrophilic fluoroalkyl reagents 35-3 (Togni I reagent, perfluoroalkyl iodide, difluoroalkyl bromides etc.) as fluoroalkyl radical precursors (Scheme 35). Different from the alkylation system, the interrupted cross-coupling reactivity of the fluoroalkyl radical with the NHC-attached ketyl radical was the key for the three-component couplings and an extraordinarily broad scope for olefins was observed (for example, in Li's system >120 examples, up to 99%). Styrenes, indole double bonds, vinyl sulfide, vinyl ethers, vinyl esters, and aliphatic alkenes were well tolerated, delivering β-fluoroalkylated ketones with complete regioselectivity. This radical relay strategy also found applications in the late-stage functionalization of pharmaceutical skeletons.
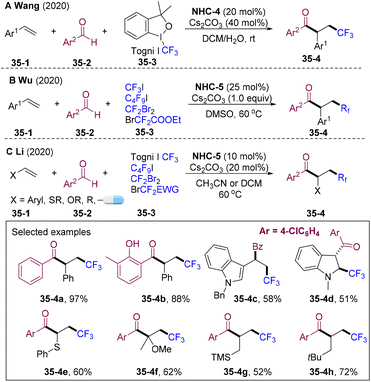 |
| Scheme 35 NHCs-catalyzed 1,2-fuoroalkyl-acylations of olefins. | |
In 2022, Wang and co-workers developed NHCs/PC dual-catalyzed acyl-difluoromethylation of inert olefins via radical relay (Scheme 36A).97 This process featured mild and redox-neutral conditions, good functional group tolerance, and broad substrate scope. Upon irradiation, excited IrIII* underwent reductive quenching by BI, generating reduced state IrII and BIR. SET of IrII with the CHF2 source 36-3 delivered the CHF2 radical 36-5 and regenerated IrIII. CHF2 radicals were added to the olefins and trapped by ketyl radicals providing β-CHF2 substituted ketones 36-4 as the final products. Independently, Wang's group developed an acyl-difluoromethylation system employing readily available and cost-effective HCF2SO2Na 36-9 as the HCF2 radical precursor, aldehyde as the acylating reagent under thermal conditions (Scheme 36B).98 (NH4)2S2O8 could serve as the oxidant undergoing single electron oxidation with HCF2SO2Na and BI generating both the HCF2 radical and NHC-attached ketyl radicals, mediating the radical relay process.
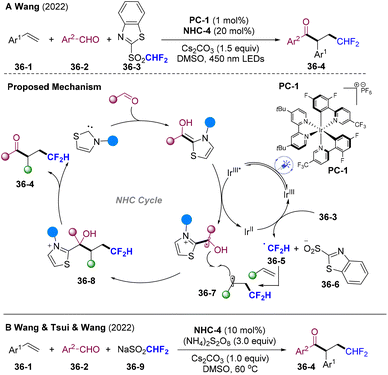 |
| Scheme 36 NHCs-catalyzed acyl-difluoromethylation of olefins. | |
The intermolecular HAT process is the most ideal strategy for obtaining carbon-centered radicals from general chemical feedstocks and has received significant attention.99 In 2023, Li and co-workers developed elegant diacylation and alkylacyaltion of olefins by combining intermolecular radical HAT strategy and oxidative NHC catalysis (Scheme 37).100 Various inert C–H bonds of hydrocarbons, such as aldehydes, CH2Cl2, CHCl3, CH2Br2, 1,4-dioxane, and THF, could undergo inert C–H activation acting as a carbon-centered radical source and providing structurally diversified 1,4-diketones 37-4 and γ-functionalized ketones 37-5. Mechanistic studies indicated that SET of peroxyesters 37-6 with BI generates BIR and t-butoxy radicals 37-7, which undergo an intermolecular HAT process delivering acyl or alkyl radicals 37-8, achieving the divergent difunctionalization of alkenes.
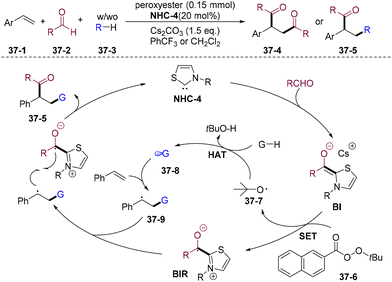 |
| Scheme 37 NHCs-catalyzed acyl-difluoromethylation of olefins. | |
In 2022, Wang and co-workers disclosed NHCs/PC dual-catalyzed alkylacylation of olefins employing diazo compounds 38-3 as an alkyl radical source via proton-coupled electron transfer (PCET) (Scheme 38).101 Luminescence quenching experiments pointed to the reductive quenching process of IrIII* by BI. The resulting reduced state IrII could undergo PCET with diazo ester 38-3 and proton generating alkyl radical species 38-5 and ground state IrIII. A selective radical addition-trapping cascade might occur to assemble two groups simultaneously. This represents the first case in NHC catalysis combined with PCET, providing a new avenue for NHCs/PC dual catalysis.
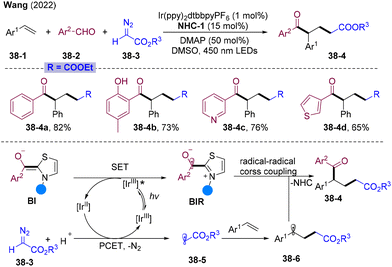 |
| Scheme 38 NHCs/PC dual-catalyzed radical alkylacylation of alkenes via a PCET process. | |
Alternatively, single electron reduction of the NHC-bound acyl azolium ion with reductive radical sources could offer BIR and transient radicals; however, because of the low oxidation potential of the acyl azolium ion,82 photocatalysis was required to mediate the SET process.
In 2020, the Studer group developed state-of-the-art radical 1,2-trifluoromethyl-acylation of olefins employing bench-stable and readily accessible NaSO2CF3 as the trifluoromethyl radical precursor and acyl fluorides as the acylation reagents (Scheme 39).102 Under LED irradiation, the generated excited state IrIII* underwent a reductive quenching process with Langlois reagent affording the trimethyl radical and IrII. The IrII could be oxidated by the NHC-bound acyl azolium ion generated in situ providing persistent BIR and regenerating IrIII, closing the photo redox cycle. Independently, the group of Scheidt developed NHCs/PC dual-catalyzed radical cross-coupling of acyl imidazole and Hantzsch ester.99,103,104 Shortly thereafter, the group of Chi developed visible light-mediated cross-coupling of the carboxylic ester and alkyl-substituted Hantzsch ester employing thiazolium-based NHC-4.105 Those pioneering studies opened a new avenue for generating BIR via SET reduction of acyl azolium ions under visible-light mediated conditions, which is the critical step for NHC-catalyzed radical transformation of carboxylic acid derivatives.
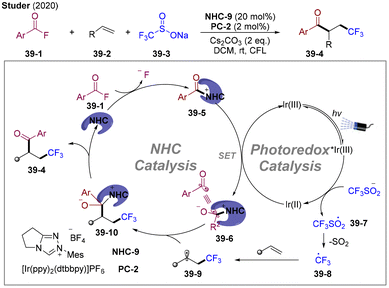 |
| Scheme 39 NHCs/PC dual-catalyzed radical trifluoromethyl-acylation of alkenes. | |
In 2021, the Ohmiya group developed visible-light driven NHCs-catalyzed cross-coupling of alkylborates 40-1 and acyl imidazoles 40-2, and alkylacylation of alkenes (Scheme 40A).106 Interestingly, designed alkyl borates 40-1 were used as photosensitizers to undergo SET with acyl azolium 40-2 generating alkyl radical 40-5 and BIR under visible light irradiation, to mediate the radical cascade transformations. Similarly, Scheidt and co-workers developed a radical alkylacylation system employing trifluoroborate salts 40-7 as an alkyl radical precursor (Scheme 40B).107 The excited state of 4-CzIPN could undergo a reductive quenching process with 40-7 to afford alkyl radicals. Independently, the Chi group disclosed state-of-the-art NHCs/PC dual-catalyzed radical coupling of carboxylic acids 40-9 and acyl imidazoles 40-2, which could extend to three-component coupling by employing alkene as a radical acceptor (Scheme 40C).108 Stern–Volmer quenching experiments showed that the excited state PC* was efficiently quenched by carboxylic acids 40-9, which pointed to a reductive quenching process for forming alkyl radicals. As acyl imidazoles 40-2 could be generated in situ from the carboxylic acid and CDI, the presented method provides a creationary approach for the radical coupling of two carboxylic acids. Independently, the Studer group developed an NHCs/PC-dual catalyzed system for alkylacylation of olefins employing alcohol 40-14 and aroyl fluorides (Scheme 40D).109 NHC-B1 was employed to form the NHC-adduct 40-15 to activate the C–O bonds of alcohols.110 Oxidative quenching of PC* by acyl azolium offered BIR and highly oxidizing IrIV, which could undergo SET with the NHC-adduct 40-15, generating alkyl radicals 40-12 and triggering the radical relay.
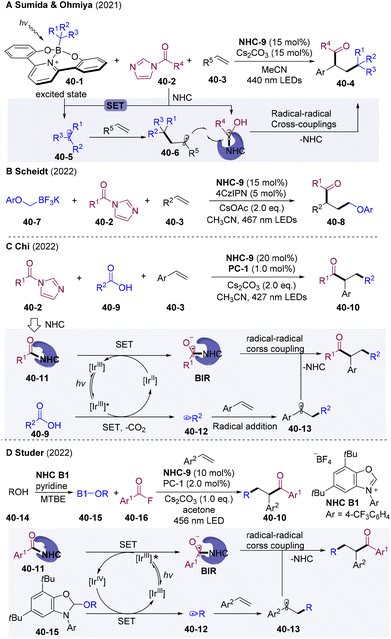 |
| Scheme 40 Visible light-mediated NHCs-catalyzed radical alkyl-acylation of alkenes. | |
5.1.2 NHCs-catalyzed aryl-acylation of olefins.
Aryl halides are readily available and bench-stable arylation sources via oxidative additions; they were also found to undergo single electron reduction providing aryl radicals.111 However, direct SET of iodobenzene with BI was challenging owing to the gap in redox potential. In a pioneering study, Ohmiya, Nagao, and co-workers developed NHCs-catalyzed arylacylation of olefins employing iodobenzene 41-2 as an aryl radical precursor via challenging thermodynamically unfavoured SET with BI (Scheme 41A).112 The rational design could extend this system to radical C–H acylation via the 1,5-HAT process. Independently, the Yan and Bertrand group developed similar transformations by employing designed mesoionic carbene (MIC)113 as the catalyst (Scheme 41B).114 The BI4-10 derived from MIC could serve as an organic super electron donor, undergo SET with various iodobenzene compounds, and allows for inter- and intramolecular arylacylation of olefins under mild reaction conditions delivering 41-4 and 41-9. Highly reducing MIC-based BI could find new applications for designing novel NHC radical catalysis.
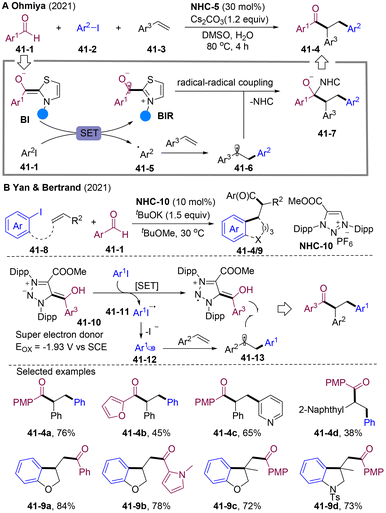 |
| Scheme 41 NHCs-catalyzed aryl-acylation of olefins. | |
5.1.3 NHCs-catalyzed 1,2-diacylation of olefins.
Radical 1,2-diacylation of olefins can provide direct access to value-added 1,4-diketones and have received increasing attention. NHCs can modulate the reactivity of acyl radials, which is advantageous in challenging unsymmetric 1,2-diacylation. In 2022, the Larionov group developed EDA-complex mediated 1,2-diacylation of olefins under visible light irradiation (Scheme 42A).115BI and oxime ester 42-2 could form the EDA-complex 42-5via hydrogen bonding and undergo SET, providing acyl radical 42-6 and BIR species under irradiation conditions. The different properties of those radicals contribute to the excellent regio- and chemo-selective addition-trapping cascade delivering diversified 1,4-diketones. However, the acyl radical source was limited to aryl-substituted oxime ester 42-2. Independently, the group of Zhang and Zheng disclosed NHCs/PC dual-catalyzed 1,2-diacylation of olefins by employing readily available α-keto acids 42-10 and aroyl fluorides 42-9 (Scheme 42B).116 Alkyl-substituted α-keto acids were also applicable for this transformation. Stern–Volmer quenching studies were conducted and the excited state of PC* was preferentially quenched by an acyl azolium ion instead of pyruvic acid. The oxidative quenching process seems more favourable for pyruvic acid; however, the energy transfer pathway could not be excluded. This strategy features mild conditions, a broad substrate scope (21 symmetric examples, 56 unsymmetric examples, and 5 examples for olefins derived from drugs or natural products), and excellent selectivity, providing a modular platform for the diacylation of olefins.
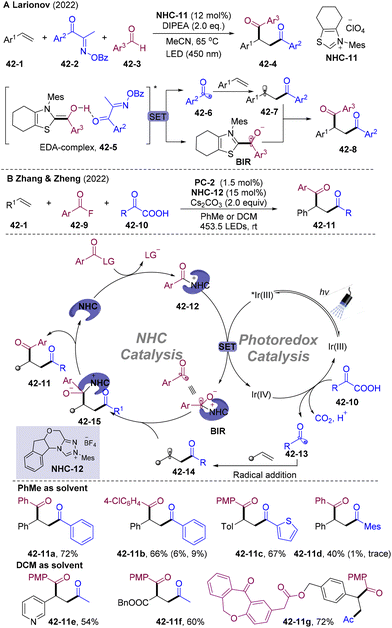 |
| Scheme 42 NHCs-catalyzed 1,2-diacylation of olefins. | |
Soon after, the Smith117 group and Han118 group disclosed a similar diacylation system (Scheme 43A and B). However, a reductive quenching mechanism of PC* by α-keto acid 43-3 was proposed for the generation of transient acyl radicals. The group of Feng and Fan developed NHCs/PC dual-catalyzed diacylation of olefins with aroyl fluorides 43-2 (Scheme 43C).119 Stoichiometric PPh3 was employed to activate in situ generated carboxy anion 43-10 delivering an acyl radical. This strategy could extend to unsymmetric diacylation employing carboxylic acid and acyl azolium salt 43-13. In 2023, the same group developed NHCs/PC dual-catalyzed alkoxycarbonyl-acylation of styrenes employing potassium 2-ethoxy-2-oxoacetate 43-14 as an alkoxycarbonyl radical source via a reductive quenching process (Scheme 43D).120
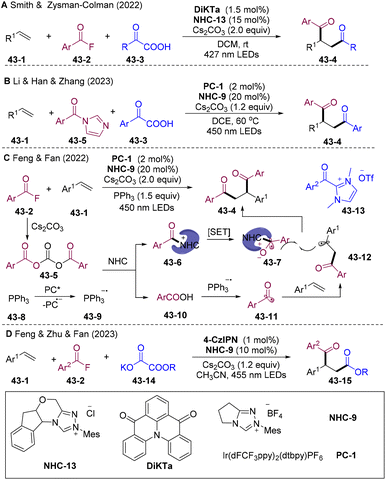 |
| Scheme 43 NHCs/PC dual-catalyzed 1,2-diacylation of olefins. | |
5.2. NHCs-catalyzed silyl-acylation of olefins
Despite the explosive development of NHCs-catalyzed three-component acylative difunctionalization of olefins, the transient radical partner has been mainly limited to carbon-centered radicals. In 2022, the Ohmiya and Sumida group developed NHCs/PC dual-catalyzed silyl-acylation of olefins employing silylboronic acid pinacol esters (R3SiBpin) 44-3 as the reductive silyl radical precursor (Scheme 44).121 Mechanistically, reductive quenching of 4-CzIPN* by base-activated R3SiBpin 44-6 generates silyl radicals 44-7 to participate in cascade transformations. This methodology provides attractive alternatives for directly constructing otherwise difficult to synthesize β-silyl ketones 44-4.
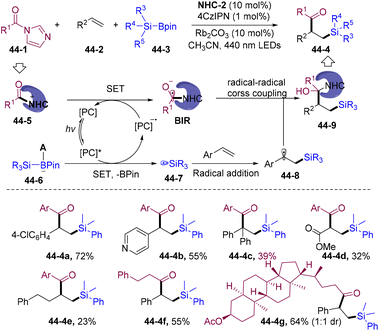 |
| Scheme 44 NHCs/PC dual-catalyzed silyl-acylation alkenes. | |
5.3. NHCs-catalyzed sulfonyl-/thiol-acylation of olefins
In 2021, the Studer group developed conceptually novel α-C–H acylation of olefins by employing unprecedented NHCs/PC/sulfinate cooperative triple catalysis.122 Shortly after, Shu and co-workers also reported a similar α-acylation system employing benzaldehyde as the acylation source and DQ as the oxidant.123 Those transformations proceed via a sulfonylacylation/sulfonyl-elimination cascade.
In 2022, the Shu group developed NHCs/PC dual-catalyzed thiol-acylation of olefins with disulfides and aldehydes (Scheme 45).124 Excited state *RuII undergoes reductive quenching generating BIR and a reductive form of RuI, which reduce disulfides 45-2 affording S-centered radical 45-6 by releasing a thiophenol anion. A subsequent radical addition-trapping cascade might occur, delivering β-thiolated-α-arylated ketones 45-4 under mild conditions with a broad subtract scope and good functional group tolerance.
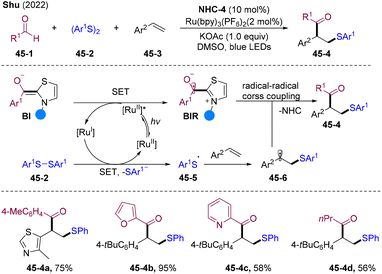 |
| Scheme 45 NHCs/PC dual-catalyzed thiol-acylation of alkenes. | |
5.4. NHCs-catalyzed dearomatizing fluoro-acylation of benzofurans
Direct construction of the 2,3-dihydrobenzofuran scaffold is highly attractive owing to its widespread applications in natural products and bioactive compounds.125 In 2022, Studer and co-workers developed NHCs/PC dual-catalyzed fluor-aroylation of benzofurans 46-2 employing acyl fluorides 46-1 for both acylation and fluorination via radical cation/radical cross-coupling (Scheme 46).126 This bifunctional reagent could extend to anhydrides, realizing aroyloxyacylation. In a preliminary attempt employing the chiral NHC-14, the fluoro-acylation product 46-3d was obtained with 53% yield and 30% ee (>20
:
1 dr), which indicates that enantio-determining cross-coupling may occur prior to the F-anion attacking, thus pointing to the rare radical–radical cation cross-couplings.127 The Volmer quenching study indicates the reductive quenching mechanism of PC* by benzofuran 46-2. Single electron oxidation of benzofuran by the PC* generated the radical cation 46-5. Nucleophilic substitution of NHC with aroyl fluoride gave the F anion and acyl azolium ion 46-4, which underwent SET with reducing state PC˙−, regenerating the PC and affording BIR. Subsequent cross-coupling of BIR with the benzofuran radical cation generated the oxocarbenium ion. Diastereoselective nucleophilic addition by the F-anion and NHC fragmentation gave the fluoro-acylation products. The successful application of difunctional reagents in NHC catalysis might open a new avenue for designing atom- and step-economic cascade transformations.
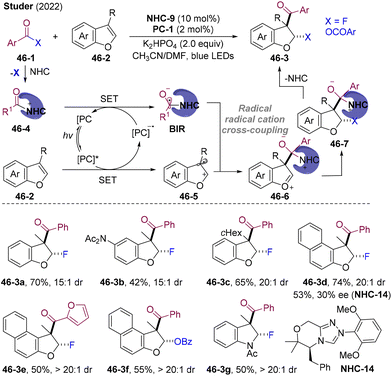 |
| Scheme 46 NHCs/PC dual-catalyzed dearomatizing fluoro-acylation of benzofurans. | |
5.5. NHCs-catalyzed acylated-difunctionalization of other unsaturated systems
5.5.1 NHCs-catalyzed 1,3-acylative esterification of aryl cyclopropanes.
Cyclopropane, the small ring with a “double bond character”,128 could undergo single electron oxidation generating corresponding radical cation intermediate to realize 1,3-difunctionalization. In 2021, Studer and co-workers developed 1,3-aroyloxylacylation of aryl cyclopropanes 47-2 enabled by NHCs/PC dual catalysis (Scheme 47).129 Author demonstrated the reductive quenching of PC* by cyclopropane generating radical cation 47-8, which undergoes sequence nucleophilic addition and radical/radical cross-coupling affording γ-aroyloxylated ketones 47-3. Because of aryl cyclopropane's high oxidation potential, the scope was limited to electron-rich ones.
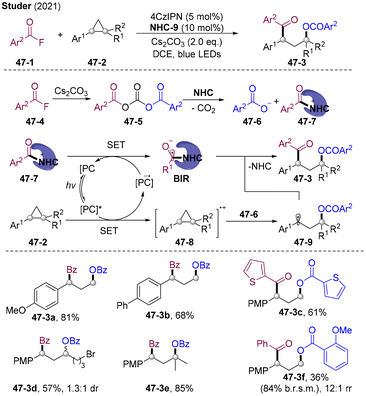 |
| Scheme 47 NHCs/PC dual-catalyzed 1,3-aroyloxylacylation of aryl cyclopropanes. | |
5.5.2 NHCs-catalyzed 1,4-acylative difunctionalization of 1,3-enyne.
1,3-Enynes are versatile feedstocks that undergo radical 1,4-difunctionalization delivering allenes.130,131 Transient radicals generated via SET of BI with a radical precursor can be added to 1,3-enynes, affording allene radicals, which could further be trapped by BIR, generating allenyl ketones. In 2021, Du,132 Huang,133 and Li134 group independently developed alkyl/perfluoroalkyl radical addition initiated radical 1,4-alkylacyaltion of 1,3-enynes employing NHC catalysis (Scheme 48A). A wide range of alkyl/perfluoroalkyl radical precursors 48-3, including CF3I and activated alkyl halides 48-3a, Togni I reagent 48-3b, cycloketone oxime esters 48-3c, redox-active esters 48-3d, and Katritzky pyridinium salts 48-4e, were tolerated, providing direct access to otherwise difficult to synthesize tetra-substituted allenyl ketones 48-4 efficiently. Mechanistically, SET of BI with radical precursor 48-3 generates alkyl radical and BIR. Chemoselective addition of transient radical 48-5 to the olefin unit of 1,3-enynes 48-2 and resonance generating allene radicals 48-7, which undergo unprecedented allenyl radical and BIR cross-coupling, provides allenyl ketones 48-4. Soon after, the Du group135 extended this system to the difluoro olefination of 1,3-enynes (Scheme 48B). Commercially available CF2Br248-9 was employed as the CF2Br˙ source and underwent 1,4-difunctionalization and tandem elimination providing gem-difluorovinyl allenes 48-10.
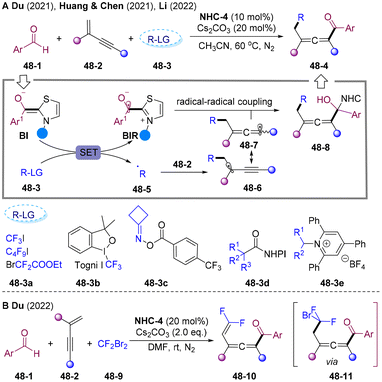 |
| Scheme 48 NHCs-catalyzed 1,4-alkylacyaltion of 1,3-enynes. | |
Independently, in 2022, the group of Zheng and Zhang developed NHCs/PC dual-catalyzed 1,4-sulfonylacylation of 1,3-enynes leading to direct construction of α-sulfonyl substituted allenyl ketones (Scheme 49A).136 This strategy could be further applied in the construction of the hetero-1,3,5-trisubstituted benzene core (Scheme 49B).137 Interestingly, Stern–Volmer quenching studies show that excited state PC* was preferentially quenched by the azolium ion instead of TolSO2Na, which points to the oxidative quenching mechanism. Mechanistically, the oxidative quenching process *IrIII by the acyl azolium ion generated IrIV and BIR. Highly oxidizing IrIV undergoes SET with TolSO2Na delivering the transient Ts˙ radical and regenerating the IrIII closing PC-cycle. The subsequent radical addition/resonance/trapping cascade afforded allenyl ketones. This transformation represents the first oxidative quenching mechanism in NHCs/PC dual catalysis, which could find further applications in designing cascade reactions with a high oxidation potential radical precursor.
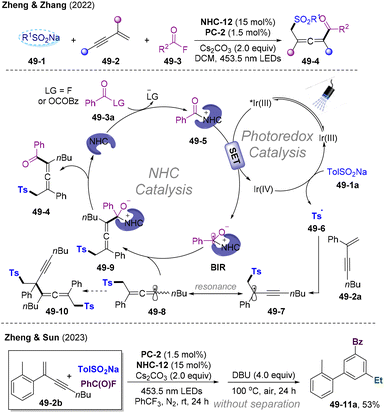 |
| Scheme 49 NHCs/PC dual-catalyzed 1,4-sulfonylacylation of 1,3-enynes. | |
Very recently, Hong and co-workers developed visible-light mediated NHCs-catalyzed cross-coupling of alcohols and carboxylic acid derivatives in the absence of a photocatalyst (Scheme 50).138 This state-of-the-art coupling system could extend to alkyl-acylation of olefins 50-4 and 1,3-enynes 50-5, leading to structurally diversified ketones 50-6 and 50-7 with good functional group tolerance. Mechanistically, the reaction of alcohol with CS2 could generate xanthate 50-8 to activate the C–O bonds of alcohol and undergo photoexcitation through LED irradiation, affording 50-8*. Photoinduced charge transfer between 50-8* and 50-10 might occur, delivering xanthate radical intermediate 50-9 and BIR. 50-9 was trapped by PR3, generating the phosphoranyl radical 50-11, which underwent efficient β-scission processes to obtain alkyl radicals 50-13. The subsequent radical addition-trapping cascade provided the final products 50-6 and 50-7.
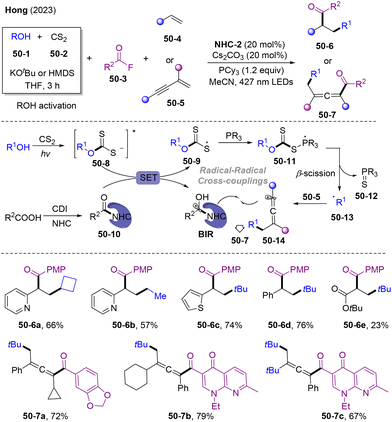 |
| Scheme 50 Visible light mediated NHCs-catalyzed alkyl-acylation of olefins and 1,3-enynes. | |
5.5.3 NHCs-catalyzed 1,2-sulfonylacylation of allenes.
Allenes are versatile building blocks in organic synthesis.139 Transient radical addition to allenes delivered allyl radicals,140 which underwent further functionalization to deliver substituted olefins. In 2023, the Zheng and Zhang group developed NHCs/PC dual-catalyzed radical 1,2-sulfonyl acylation of allenes 51-1 with sulfinates 51-3 and aroyl fluoride 51-2 (Scheme 51).141 Extremely mild conditions allowed for a broad substrate scope and functional group tolerance. Similar to previous reports, in situ generated sulfonyl radicals 51-7 underwent regioselective addition to allenes generating allyl radicals 51-8, which are trapped by persistent BIR affording sulfonyl-substituted olefins. This addition-trapping scenario could open up new avenues for radical difunctionalization of allenes.
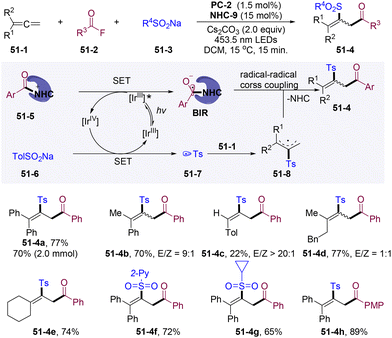 |
| Scheme 51 NHCs/PC dual-catalyzed 1,2-sulfonylacylation of allenes. | |
6. Conclusions
As demonstrated by the reactions summarized in this review, radical-mediated acylative three-component difunctionalization of unsaturated carbon–carbon bonds has emerged as a practical and attractive approach for the synthesis of ketones or related derivatives. As the key steps, C–C(acyl) bond formations proceeded via acyl radical addition, C–M-acyl reductive elimination, nucleophilic substitution, or radical–radical cross-coupling with NHC-attached ketyl radicals. Significant progress has been made in the past years, including acylative difunctionalization of olefins, dienes, alkynes, 1,3-enynes, cyclopropanes, and allenes, leading to α-acylation or β-acylation (Scheme 52). In this research field, several challenges still exist: (1) the three-component acylative difunctionalization has focused on olefins. Further application for a large variety of unsaturated carbon–carbon bonds is highly desirable. (2) Enantioselectivity control is challenging. The development of a chiral NHC catalytic system could be promising for asymmetric C–C(acyl) bond formation. It is expected that significant attention needs to be focused on this fascinating area.
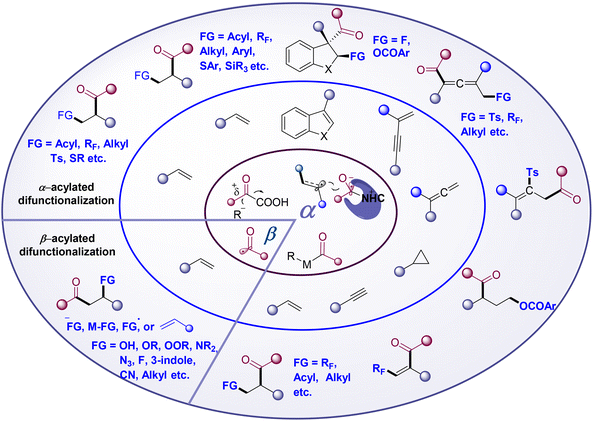 |
| Scheme 52 Three-component radical acylated difunctionalization of unsaturated carbon–carbon bonds. | |
Conflicts of interest
There are no conflicts to declare.
Acknowledgements
We acknowledge the Natural Science Foundation of Jilin Province (YDZJ202201ZYTS338), NSFC (22001157, 21831002, 22193012, and 22201033), Ten Thousand Talents Program, Jilin Educational Committee (JJKH20231295KJ and JJKH20231302KJ), and the Fundamental Research Funds for the Central Universities (2412022QD016, 2412021QD007 and 2412022ZD012) for generous financial support.
References
- M. Yan, J. C. Lo, J. T. Edwards and P. S. Baran, Radicals: Reactive Intermediates with Translational Potential, J. Am. Chem. Soc., 2016, 138, 12692–12714 CrossRef CAS PubMed
.
- M.-J. Luo, Q. Xiao and J.-H. Li, Electro-/photocatalytic alkene-derived radical cation chemistry: recent advances in synthetic applications, Chem. Soc. Rev., 2022, 51, 7206–7237 RSC
.
- Z.-L. Li, G.-C. Fang, Q.-S. Gu and X.-Y. Liu, Recent advances in copper-catalysed radical-involved asymmetric 1,2-difunctionalization of alkenes, Chem. Soc. Rev., 2020, 49, 32–48 RSC
.
- S. Engl and O. Reiser, Copper-photocatalyzed ATRA reactions: concepts, applications, and opportunities, Chem. Soc. Rev., 2022, 51, 5287–5299 RSC
.
- H. Jiang and A. Studer, Intermolecular radical carboamination of alkenes, Chem. Soc. Rev., 2020, 49, 1790–1811 RSC
.
- H.-M. Huang, P. Bellotti and F. Glorius, Transition metal-catalysed allylic functionalization reactions involving radicals, Chem. Soc. Rev., 2020, 49, 6186–6197 RSC
.
- S. Zhu, X. Zhao, H. Li and L. Chu, Catalytic three-component dicarbofunctionalization reactions involving radical capture by nickel, Chem. Soc. Rev., 2021, 50, 10836–10856 RSC
.
- C. Chatgilialoglu, D. Crich, M. Komatsu and I. Ryu, Chemistry of Acyl Radicals, Chem. Rev., 1999, 99, 1991–2070 CrossRef CAS PubMed
.
- M. S. Kharasch, W. H. Urry and B. M. Kuderna, Reactions of atoms and FREE radicals in solution. xx. The addition of aldehydes to olefins, J. Org. Chem., 1949, 14, 248–253 CrossRef CAS PubMed
.
- A. Banerjee, Z. Lei and M.-Y. Ngai, Acyl Radical Chemistry via Visible-Light Photoredox Catalysis, Synthesis, 2019, 51, 303–333 CrossRef CAS PubMed
.
- Y.-L. Liu, Y.-J. Ouyang, H. Zheng, H. Liu and W.-T. Wei, Recent advances in acyl radical enabled reactions between aldehydes and alkenes, Chem. Commun., 2021, 57, 6111–6120 RSC
.
- C. Raviola, S. Protti, D. Ravelli and M. Fagnoni, Photogenerated acyl/alkoxycarbonyl/carbamoyl radicals for sustainable synthesis, Green Chem., 2019, 21, 748–764 RSC
.
- L. Ruan, C. Chen, X. Zhang and J. Sun, Recent Advances on the Photo-Induced Reactions of Acyl Radical, Chin. J. Org. Chem., 2018, 38, 3155–3164 CrossRef CAS
.
- H. Zhang, S. Liang, D. Wei, K. Xu and C. Zeng, Electrocatalytic Generation of Acyl Radicals and Their Applications, Eur. J. Org. Chem., 2022, 39, e202200794 Search PubMed
.
- H. Hou, B. Chen, C. Tung and L. Wu,
α-Acylation of Olefins via Photocatalysis, Chin. J. Org. Chem., 2023, 43, 1012–1022 CrossRef
.
- J.-B. Peng, H.-Q. Geng and X.-F. Wu, The Chemistry of CO: Carbonylation, Chem, 2019, 5, 526–552 CAS
.
- C.-K. Ran, H.-Z. Xiao, L.-L. Liao, T. Ju, W. Zhang and D.-G. Yu, Progress and challenges in dicarboxylation with CO2, Natl. Sci. Open, 2023, 2, 20220024 CrossRef
.
- C.-K. Ran, L.-L. Liao, T.-Y. Gao, Y.-Y. Gui and D.-G. Yu, Recent progress and challenges in carboxylation with CO2, Curr. Res. Green Sustainable Chem., 2021, 32, 100525 CrossRef CAS
.
- J.-H. Ye, T. Ju, H. Huang, L.-L. Liao and D.-G. Yu, Radical Carboxylative Cyclizations and Carboxylations with CO2, Acc. Chem. Res., 2021, 54, 2518–2531 CrossRef CAS PubMed
.
- T. Taniguchi, Y. Sugiura, H. Zaimoku and H. Ishibashi, Iron-Catalyzed Oxidative Addition of Alkoxycarbonyl Radicals to Alkenes with Carbazates and Air, Angew. Chem., Int. Ed., 2010, 49, 10154–10157 CrossRef CAS PubMed
.
- B. Budai, A. Leclair, Q. Wang and J. Zhu, Copper–Catalyzed 1,2−Methoxy Methoxycarbonylation of Alkenes with Methyl Formate, Angew. Chem., Int. Ed., 2019, 58, 10305–10309 CrossRef CAS PubMed
.
- M. Zheng, J. Hou, L.-W. Zhan, Y. Huang, L. Chen, L.-L. Hua, Y. Li, W.-Y. Tang and B.-D. Li, Visible-Light-Driven, Metal-Free Divergent Difunctionalization of Alkenes Using Alkyl Formates, ACS Catal., 2020, 11, 542–553 CrossRef
.
- W. Liu, Y. Li, K. Liu and Z. Li, Iron-Catalyzed Carbonylation-Peroxidation of Alkenes with Aldehydes and Hydroperoxides, J. Am. Chem. Soc., 2011, 133, 10756–10759 CrossRef CAS PubMed
.
- K. Liu, Y. Li, X. Zheng, W. Liu and Z. Li, Synthesis of α-ester–β-keto peroxides via iron-catalyzed carbonylation–peroxidation of α,β-unsaturated esters, Tetrahedron, 2012, 68, 10333–10337 CrossRef CAS
.
- L. Zhao, Y. Wang, Z. Ma and Y. Wang, Dirhodium(II)-Catalyzed Carbonylation Peroxidation of α,β-Unsaturated Esters: Mechanistic Insight into the Role of Aryl Aldehydes, Inorg. Chem., 2017, 56, 8166–8174 CrossRef CAS PubMed
.
- Y. Yao, Z. Wang and B. Wang, Tetra-n-butylammonium bromide (TBAB)-initiated carbonylation–peroxidation of styrene derivatives with aldehydes and hydroperoxides, Org. Chem. Front., 2018, 5, 2501–2504 RSC
.
- D. Zhao, Y. Pan, X. Chen, Y. Han, C. Yan, Y. Shi, H. Hou and S. Zhu, Three–Component Acylation/Peroxidation of Alkenes through Visible–Light Photocatalysis, ChemistrySelect, 2021, 6, 10834–10838 CrossRef CAS
.
- W.-T. Wei, X.-H. Yang, H.-B. Li and J.-H. Li, Oxidative Coupling of Alkenes with Aldehydes and Hydroperoxides: One-Pot Synthesis of 2,3-Epoxy Ketones, Adv. Synth. Catal., 2015, 357, 59–63 CrossRef CAS
.
- Q. Ke, B. Zhang, B. Hu, Y. Jin and G. Lu, A transition-metal-free, one-pot procedure for the synthesis of α,β-epoxy ketones by oxidative coupling of alkenes and aldehydes via base catalysis, Chem. Commun., 2015, 51, 1012–1015 RSC
.
- J. Li and D. Z. Wang, Visible-Light-Promoted Photoredox Syntheses of α,β-Epoxy Ketones from Styrenes and Benzaldehydes under Alkaline Conditions, Org. Lett., 2015, 17, 5260–5263 CrossRef CAS PubMed
.
- W.-C. Yang, S.-S. Weng, A. Ramasamy, G. Rajeshwaren, Y.-Y. Liao and C.-T. Chen, Vanadyl species-catalyzed complementary β-oxidative carbonylation of styrene derivatives with aldehydes, Org. Biomol. Chem., 2015, 13, 2385–2392 RSC
.
- G. F. P. de Souza, J. A. Bonacin and A. G. Salles Jr., Visible-Light-Driven Epoxyacylation and Hydroacylation of Olefins Using Methylene Blue/Persulfate System in Water, J. Org. Chem., 2018, 83, 8331–8340 CrossRef CAS PubMed
.
- C. Zhou, G. Wu, Y. Feng, Q. Li, H. Su, D. E. Mais, Y. Zhu, N. Li, Y. Deng, D. Yang and M. W. Wang, Discovery and biological characterization of a novel series of androgen receptor modulators, Br. J. Pharmacol., 2008, 154, 440–450 CrossRef CAS PubMed
.
- F. Kudo, A. Miyanaga and T. Eguchi, Biosynthesis of natural products containing β-amino acids, Nat. Prod. Rep., 2014, 31, 1056–1073 RSC
.
- Y.-Y. Cheng, T. Lei, L. Su, X. Fan, B. Chen, C.-H. Tung and L.-Z. Wu, Visible Light Irradiation of Acyl Oxime Esters and Styrenes Efficiently Constructs β-Carbonyl Imides by a Scission and Four-Component Reassembly Process, Org. Lett., 2019, 21, 8789–8794 CrossRef CAS PubMed
.
- M. Lux and M. Klussmann, Additions of Aldehyde-Derived Radicals and Nucleophilic N-Alkylindoles to Styrenes by Photoredox Catalysis, Org. Lett., 2020, 22, 3697–3701 CrossRef CAS PubMed
.
- P.-J. Xia, F. Liu, Y.-M. Pan, M.-P. Yang and Y.-Y. Yang, Efficient access to β-amino acid ester/β-amino ketone derivatives via photocatalytic radical alkoxycabonylimidation/carbonylimidation of alkenes, Org. Chem. Front., 2022, 9, 2522–2528 RSC
.
- X. Zhao, C. Zhang, H. Zhang, X. Zheng, J. Liang, Q. Liang and W. Lin, Metal-free photosensitized intermolecular carboimination of alkenes: a green and direct access to both β-amino acids and β-amino ketones, Org. Biomol. Chem., 2022, 20, 7593–7598 RSC
.
- L. Zhang, S. Liu, Z. Zhao, H. Su, J. Hao and Y. Wang, (Salen)Mn(iii)-catalyzed chemoselective acylazidation of olefins, Chem. Sci., 2018, 9, 6085–6090 RSC
.
- L. Ge, Y. Li and H. Bao, Iron-Catalyzed Radical Acyl-Azidation of Alkenes with Aldehydes: Synthesis of Unsymmetrical β-Azido Ketones, Org. Lett., 2018, 21, 256–260 CrossRef PubMed
.
- H. Wang, L.-N. Guo and X.-H. Duan, Silver-catalyzed decarboxylative acylfluorination of styrenes in aqueous media, Chem. Commun., 2014, 50, 7382 RSC
.
- M. Zhang, J. Xi, R. Ruzi, N. Li, Z. Wu, W. Li and C. Zhu, Domino-Fluorination–Protodefluorination Enables Decarboxylative Cross-Coupling of α-Oxocarboxylic Acids with Styrene via Photoredox Catalysis, J. Org. Chem., 2017, 82, 9305–9311 CrossRef CAS PubMed
.
- D. V. Patil, H. Y. Kim and K. Oh, Visible Light-Promoted Friedel–Crafts-Type Chloroacylation of Alkenes to β-Chloroketones, Org. Lett., 2020, 22, 3018–3022 CrossRef CAS PubMed
.
- H. Fang, C. Empel, I. Atodiresei and R. M. Koenigs, Photoinduced Palladium-Catalyzed 1,2-Difunctionalization of Electron-Rich Olefins via a Reductive Radical-Polar Crossover Reaction, ACS Catal., 2023, 13, 6445–6451 CrossRef CAS
.
- Y. Jiao, M.-F. Chiou, Y. Li and H. Bao, Copper-Catalyzed Radical Acyl-Cyanation of Alkenes with Mechanistic Studies on the tert-Butoxy Radical, ACS Catal., 2019, 9, 5191–5197 CrossRef CAS
.
- X. Kong, X. Chen, Y. Chen and Z.-Y. Cao, Scalable Electrocatalytic Intermolecular Acylcyanation and Aminocyanation of Alkenes, J. Org. Chem., 2022, 87, 7013–7021 CrossRef CAS PubMed
.
- P.-Z. Wang, Y. Gao, J. Chen, X.-D. Huan, W.-J. Xiao and J.-R. Chen, Asymmetric three-component olefin dicarbofunctionalization enabled by photoredox and copper dual catalysis, Nat. Commun., 2021, 12, 1815 CrossRef CAS PubMed
.
- K. Miura, M. Tojino, N. Fujisawa, A. Hosomi and I. Ryu, Cascade Carbonylation Methods Leading to β-Diketones and β-Functionalized δ-Diketones, Angew. Chem., Int. Ed., 2004, 43, 2423–2425 CrossRef CAS PubMed
.
- T. Kippo, Y. Kimura, M. Ueda, T. Fukuyama and I. Ryu, Bromine-Radical-Mediated Synthesis of β-Functionalized β,γ- and δ,ε-Unsaturated Ketones via C–H Functionalization of Aldehydes, Synlett, 2017, 28, 1733–1737 CrossRef CAS
.
- F. Pettersson, G. Bergonzini, C. Cassani and C.-J. Wallentin, Redox-Neutral Dual Functionalization of Electron-Deficient Alkenes, Chem. – Eur. J., 2017, 23, 7444–7447 CrossRef CAS PubMed
.
- C.-S. Wu, R.-X. Liu, D.-Y. Ma, C.-P. Luo and L. Yang, Four-Component Radical Dual Difunctionalization (RDD) of Two Different Alkenes with Aldehydes and tert-Butyl Hydroperoxide (TBHP): An Easy Access to β,δ-Functionalized Ketones, Org. Lett., 2019, 21, 6117–6121 CrossRef CAS PubMed
.
- X. Zhao, H.-Y. Tu, L. Guo, S. Zhu, F.-L. Qing and L. Chu, Intermolecular selective carboacylation of alkenes via nickel-catalyzed reductive radical relay, Nat. Commun., 2018, 9, 3488 CrossRef PubMed
.
- L. Wang and C. Wang, Nickel-Catalyzed Three-Component Reductive Alkylacylation of Electron-Deficient Activated Alkenes, Org. Lett., 2020, 22, 8829–8835 CrossRef CAS PubMed
.
- X. Xi, Y. Chen and W. Yuan, Nickel-Catalyzed Three-Component Alkylacylation of Alkenes Enabled by a Photoactive Electron Donor–Acceptor Complex, Org. Lett., 2022, 24, 3938–3943 CrossRef CAS PubMed
.
- T. Fujisawa, K. Igeta, S. Odake, Y. Morita, J. Yasuda and T. Morikawa, Highly water-soluble matrix metalloproteinases inhibitors and their effects in a rat adjuvant-induced arthritis model, Bioorg. Med. Chem., 2002, 10, 2569–2581 CrossRef CAS PubMed
.
- B. H. Lipshutz, Five-membered heteroaromatic rings as intermediates in organic synthesis, Chem. Rev., 1986, 86, 795–819 CrossRef CAS
.
- M. Lemmerer, M. Schupp, D. Kaiser and N. Maulide, Synthetic approaches to 1,4-dicarbonyl compounds, Nat. Synth., 2022, 1, 923–935 CrossRef
.
- X. Zhao, B. Li and W. Xia, Visible-Light-Promoted Photocatalyst-Free Hydroacylation and Diacylation of Alkenes Tuned by NiCl2·DME, Org. Lett., 2020, 22, 1056–1061 CrossRef CAS PubMed
.
- D. Wang and L. Ackermann, Three-component carboacylation of alkenes via cooperative nickelaphotoredox catalysis, Chem. Sci., 2022, 13, 7256–7263 RSC
.
- J. Liu, L. Q. Lu, Y. X. Luo, W. Zhao, P. C. Sun, W. W. Jin, X. T. Qi, Y. Cheng and W. J. Xiao, Photoredox-Enabled Chromium-Catalyzed Alkene Diacylations, ACS Catal., 2022, 12, 1879–1885 CrossRef CAS
.
- G. Fang and X. Bi, Silver-catalysed reactions of alkynes: recent advances, Chem. Soc. Rev., 2015, 44, 8124–8173 RSC
.
- Z.-Z. Zhang, J.-J. Lei, X.-H. Zhang, X.-G. Zhang and H.-Y. Tu, Ni-Catalyzed Reductive Fluoroalkylacylation of Alkynes for the Steroselective Synthesis of Fluoroalkylated Enones, Org. Lett., 2022, 24, 6192–6196 CrossRef CAS PubMed
.
- Y.-Y. Cheng, J.-X. Yu, T. Lei, H.-Y. Hou, B. Chen, C.-H. Tung and L.-Z. Wu, Direct 1,2-Dicarbonylation of Alkenes towards 1,4-Diketones via Photocatalysis, Angew. Chem., Int. Ed., 2021, 60, 26822–26828 CrossRef CAS PubMed
.
- Y.-Y. Cheng, H.-Y. Hou, Y. Liu, J.-X. Yu, B. Chen, C.-H. Tung and L.-Z. Wu, α-Acylation of Alkenes by a Single Photocatalyst, Angew. Chem., Int. Ed., 2022, 61, e202208831 CrossRef CAS PubMed
.
- D. Leifert and A. Studer, The Persistent Radical Effect in Organic Synthesis, Angew. Chem., Int. Ed., 2020, 59, 74–108 CrossRef CAS
.
- K. Breitwieser, H. Bahmann, R. Weiss and D. Munz, Gauging Radical Stabilization with Carbenes, Angew. Chem., Int. Ed., 2022, 61, e202206390 CrossRef CAS PubMed
.
- Q.-Z. Li, R. Zeng, B. Han and J.-L. Li, Single-Electron Transfer Reactions Enabled by N-Heterocyclic Carbene Organocatalysis, Chem. – Eur. J., 2021, 27, 3238–3250 CrossRef CAS PubMed
.
- K.-Q. Chen, H. Sheng, Q. Liu, P.-L. Shao and X.-Y. Chen, N-heterocyclic carbene-catalyzed radical reactions, Sci. China: Chem., 2021, 64, 7–16 CrossRef CAS
.
- H. Ohmiya, N-Heterocyclic Carbene-Based Catalysis Enabling Cross-Coupling Reactions, ACS Catal., 2020, 10, 6862–6869 CrossRef CAS
.
- Q. Liu and X.-Y. Chen, Dual N-heterocyclic carbene/photocatalysis: a new strategy for radical processes, Org. Chem. Front., 2020, 7, 2082–2087 RSC
.
- T. Ishii, K. Nagao and H. Ohmiya, Recent advances in N-heterocyclic carbene-based radical catalysis, Chem. Sci., 2020, 11, 5630–5636 RSC
.
- K. Liu, M. Schwenzer and A. Studer, Radical NHC Catalysis, ACS Catal., 2022, 12, 11984–11999 CrossRef CAS
.
- R. Song and Y. R. Chi, N–Heterocyclic Carbene Catalyzed Radical Coupling of Aldehydes with Redox–Active Esters, Angew. Chem., Int. Ed., 2019, 58, 8628–8630 CrossRef CAS PubMed
.
- I. Nakanishi, S. Itoh, T. Suenobu and S. Fukuzumi, Direct Observation of Radical Intermediates While Investigating the Redox Behavior of Thiamin Coenzyme Models, Angew. Chem., Int. Ed., 1998, 37, 992–994 CrossRef CAS PubMed
.
- E. Chabrière, X. Vernède, B. Guigliarelli, M.-H. Charon, E. C. Hatchikian and J. C. Fontecilla-Camps, Crystal Structure of the Free Radical Intermediate of Pyruvate:Ferredoxin Oxidoreductase, Science, 2001, 294, 2559–2563 CrossRef PubMed
.
- J. Guin, S. De Sarkar, S. Grimme and A. Studer, Biomimetic Carbene-Catalyzed Oxidations of Aldehydes Using TEMPO, Angew. Chem., Int. Ed., 2008, 47, 8727–8730 CrossRef CAS PubMed
.
- Y. Du, Y. Wang, X. Li, Y. Shao, G. Li, R. D. Webster and Y. R. Chi, N-Heterocyclic Carbene Organocatalytic Reductive β,β-Coupling Reactions of Nitroalkenes via Radical Intermediates, Org. Lett., 2014, 16, 5678–5681 CrossRef CAS PubMed
.
- J. Rehbein, S.-M. Ruser and J. Phan, NHC-catalysed benzoin condensation – is it all down to the Breslow intermediate?, Chem. Sci., 2015, 6, 6013–6018 RSC
.
- V. Regnier, E. A. Romero, F. Molton, R. Jazzar, G. Bertrand and D. Martin, What Are the Radical Intermediates in Oxidative N-Heterocyclic Carbene Organocatalysis?, J. Am. Chem. Soc., 2019, 141, 1109–1117 CrossRef CAS PubMed
.
- L. Candish, M. Teders and F. Glorius, Transition-Metal-Free, Visible-Light-Enabled Decarboxylative Borylation of Aryl N-Hydroxyphthalimide Esters, J. Am. Chem. Soc., 2017, 139, 7440–7443 CrossRef CAS PubMed
.
- T. Ishii, Y. Kakeno, K. Nagao and H. Ohmiya, N-Heterocyclic Carbene-Catalyzed Decarboxylative Alkylation of Aldehydes, J. Am. Chem. Soc., 2019, 141, 3854–3858 CrossRef CAS PubMed
.
- L. Delfau, S. Nichilo, F. Molton, J. Broggi, E. Tomás-Mendivil and D. Martin, Critical Assessment of the Reducing Ability of Breslow-type Derivatives and Implications for Carbene-Catalyzed Radical Reactions, Angew. Chem., Int. Ed., 2021, 60, 26783–26789 CrossRef CAS PubMed
.
- T. Ishii, K. Ota, K. Nagao and H. Ohmiya, N-Heterocyclic Carbene-Catalyzed Radical Relay Enabling Vicinal Alkylacylation of Alkenes, J. Am. Chem. Soc., 2019, 141, 14073–14077 CrossRef CAS PubMed
.
- Y. Kakeno, M. Kusakabe, K. Nagao and H. Ohmiya, Direct Synthesis of Dialkyl Ketones from Aliphatic Aldehydes through Radical N-Heterocyclic Carbene Catalysis, ACS Catal., 2020, 10, 8524–8529 CrossRef CAS
.
- Y. Gao, S. Jiang, N.-D. Mao, H. Xiang, J.-L. Duan, X.-Y. Ye, L.-W. Wang, Y. Ye and T. Xie, Recent Progress in Fragmentation of Katritzky Salts Enabling Formation of C–C, C–B, and C–S Bonds, Top. Curr. Chem., 2022, 380, 25 CrossRef CAS PubMed
.
- I. Kim, H. Im, H. Lee and S. Hong, N-Heterocyclic carbene-catalyzed deaminative cross-coupling of aldehydes with Katritzky pyridinium salts, Chem. Sci., 2020, 11, 3192–3197 RSC
.
- Y. Gao, Y. Quan, Z. Li, L. Gao, Z. Zhang, X. Zou, R. Yan, Y. Qu and K. Guo, Organocatalytic Three-Component 1,2-Cyanoalkylacylation of Alkenes via Radical Relay, Org. Lett., 2021, 23, 183–189 CrossRef CAS PubMed
.
- L. Chen, S. Jin, J. Gao, T. Liu, Y. Shao, J. Feng, K. Wang, T. Lu and D. Du, N-Heterocyclic Carbene/Magnesium Cocatalyzed Radical Relay Assembly of Aliphatic Keto Nitriles, Org. Lett., 2021, 23, 394–399 CrossRef CAS PubMed
.
- K. Ota, K. Nagao and H. Ohmiya, N-Heterocyclic Carbene-Catalyzed Radical Relay Enabling Synthesis of δ-Ketocarbonyls, Org. Lett., 2020, 22, 3922–3925 CrossRef CAS PubMed
.
- M. Kusakabe, K. Nagao and H. Ohmiya, Radical Relay Trichloromethylacylation of Alkenes through N-Heterocyclic Carbene Catalysis, Org. Lett., 2021, 23, 7242–7247 CrossRef CAS PubMed
.
- B. Górski, A.-L. Barthelemy, J. J. Douglas, F. Juliá and D. Leonori, Copper-catalysed amination of alkyl iodides enabled by halogen-atom transfer, Nat. Catal., 2021, 4, 623–630 CrossRef
.
- Y.-F. Han, Y. Huang, H. Liu, Z.-H. Gao, C.-L. Zhang and S. Ye, Photoredox cooperative N-heterocyclic carbene/palladium-catalysed alkylacylation of alkenes, Nat. Commun., 2022, 13, 5754 CrossRef CAS PubMed
.
- Y. Zeng, C. Ni and J. Hu, Recent Advances in the One-Step Synthesis of Distally Fluorinated Ketones, Chem. – Eur. J., 2016, 22, 3210–3223 CrossRef CAS PubMed
.
- J. L. Li, Y. Q. Liu, W. L. Zou, R. Zeng, X. Zhang, Y. Liu, B. Han, Y. He, H. J. Leng and Q. Z. Li, Radical Acylfluoroalkylation of Olefins through N–Heterocyclic Carbene Organocatalysis, Angew. Chem., Int. Ed., 2020, 59, 1863–1870 CrossRef CAS PubMed
.
- B. Zhang, Q. Peng, D. Guo and J. Wang, NHC-Catalyzed Radical Trifluoromethylation Enabled by Togni Reagent, Org. Lett., 2020, 22, 443–447 CrossRef CAS PubMed
.
- H.-B. Yang, Z.-H. Wang, J.-M. Li and C. Wu, Modular synthesis of α-aryl β-perfluoroalkyl ketones via N-heterocyclic carbene catalysis, Chem. Commun., 2020, 56, 3801–3804 RSC
.
- B. Zhang and J. Wang, Acyldifluoromethylation Enabled by NHC-Photoredox Cocatalysis, Org. Lett., 2022, 24, 3721–3725 CrossRef CAS PubMed
.
- K. She, F. Liang, S. Tian, H. Wang, G. C. Tsui and Q. Wang, Organocatalytic Three-Component Acyldifluoromethylation of Vinylarenes via N-Heterocyclic Carbene-Catalyzed Radical Relay, Org. Lett., 2022, 24, 4840–4844 CrossRef CAS PubMed
.
- Q.-Z. Li, X.-X. Kou, T. Qi and J.-L. Li, Merging N-Heterocyclic Carbene Organocatalysis with Hydrogen Atom Transfer Strategy, ChemCatChem, 2023, 15, e202201320 CrossRef CAS
.
- Q.-Z. Li, Y.-Q. Liu, X.-X. Kou, W.-L. Zou, T. Qi, P. Xiang, J.-D. Xing, X. Zhang and J.-L. Li, Oxidative Radical NHC Catalysis: Divergent Difunctionalization of Olefins through Intermolecular Hydrogen Atom Transfer, Angew. Chem., Int. Ed., 2022, 61, e202207824 CAS
.
- B. Zhang, J.-Q. Qi, Y. Liu, Z. Li and J. Wang, Visible-Light-Driven Bisfunctionalization of Unactivated Olefins via the Merger of Proton-Coupled Electron Transfer and Carbene Catalysis, Org. Lett., 2022, 24, 279–283 CrossRef CAS PubMed
.
- Q.-Y. Meng, N. Döben and A. Studer, Cooperative NHC and Photoredox Catalysis for the Synthesis of β-Trifluoromethylated Alkyl Aryl Ketones, Angew. Chem., Int. Ed., 2020, 59, 19956–19960 CrossRef CAS PubMed
.
- A. V. Bay, K. P. Fitzpatrick, R. C. Betori and K. A. Scheidt, Combined Photoredox and Carbene Catalysis for the Synthesis of Ketones from Carboxylic Acids, Angew. Chem., Int. Ed., 2020, 59, 9143–9148 CrossRef CAS PubMed
.
- A. V. Bay, K. P. Fitzpatrick, G. A. González-Montiel, A. O. Farah, P. H. Y. Cheong and K. A. Scheidt, Light–Driven Carbene Catalysis for the Synthesis of Aliphatic and α–Amino Ketones, Angew. Chem., Int. Ed., 2021, 60, 17925–17931 CrossRef CAS PubMed
.
- S.-C. Ren, W.-X. Lv, X. Yang, J.-L. Yan, J. Xu, F.-X. Wang, L. Hao, H. Chai, Z. Jin and Y. R. Chi, Carbene-Catalyzed Alkylation of Carboxylic Esters via Direct Photoexcitation of Acyl Azolium Intermediates, ACS Catal., 2021, 11, 2925–2934 CrossRef CAS
.
- Y. Sato, Y. Goto, K. Nakamura, Y. Miyamoto, Y. Sumida and H. Ohmiya, Light-Driven N-Heterocyclic Carbene Catalysis Using Alkylborates, ACS Catal., 2021, 11, 12886–12892 CrossRef CAS
.
- P. Wang, K. P. Fitzpatrick and K. A. Scheidt, Combined Photoredox and Carbene Catalysis for the Synthesis of gamma-Aryloxy Ketones, Adv. Synth. Catal., 2022, 364, 518–524 CrossRef CAS PubMed
.
- S.-C. Ren, X. Yang, B. Mondal, C. Mou, W. Tian, Z. Jin and Y. R. Chi, Carbene and photocatalyst-catalyzed decarboxylative radical coupling of carboxylic acids and acyl imidazoles to form ketones, Nat. Commun., 2022, 13, 2846 CrossRef CAS PubMed
.
- N. Doeben, J. Reimler and A. Studer, Cooperative NHC/Photoredox Catalysis: Three Component Radical Coupling of Aroyl Fluorides, Styrenes and Alcohols, Adv. Synth. Catal., 2022, 364, 3348–3353 CrossRef
.
- Z. Dong and D. W. C. MacMillan, Metallaphotoredox-enabled deoxygenative arylation of alcohols, Nature, 2021, 598, 451–456 CrossRef CAS PubMed
.
- L. Pause, M. Robert and J.-M. Savéant, Can Single-Electron Transfer Break an Aromatic Carbon−Heteroatom Bond in One Step? A Novel Example of Transition between Stepwise and Concerted Mechanisms in the Reduction of Aromatic Iodides, J. Am. Chem. Soc., 1999, 121, 7158–7159 CrossRef CAS
.
- Y. Matsuki, N. Ohnishi, Y. Kakeno, S. Takemoto, T. Ishii, K. Nagao and H. Ohmiya, Aryl radical-mediated N-heterocyclic carbene catalysis, Nat. Commun., 2021, 12, 3848 CrossRef CAS PubMed
.
- W. Liu, L.-L. Zhao, M. Melaimi, L. Cao, X. Xu, J. Bouffard, G. Bertrand and X. Yan, Mesoionic Carbene (MIC)-Catalyzed H/D Exchange at Formyl Groups, Chem, 2019, 5, 2484–2494 CAS
.
- W. Liu, A. Vianna, Z. Y. Zhang, S. Q. Huang, L. W. Huang, M. Melaimi, G. Bertrand and X. Y. Yan, Mesoionic carbene-Breslow intermediates as super electron donors: Application to the metal-free arylacylation of alkenes, Chem Catal., 2021, 1, 196–206 CrossRef CAS
.
- S. F. Jin, X. W. Sui, G. C. Haug, V. D. Nguyen, H. T. Dang, H. D. Arman and O. V. Larionov, N-Heterocyclic Carbene-Photocatalyzed Tricomponent Regioselective 1,2-Diacylation of Alkenes Illuminates the Mechanistic Details of the Electron Donor-Acceptor Complex-Mediated Radical Relay Processes, ACS Catal., 2022, 12, 285–294 CrossRef CAS
.
- L. Wang, J. Sun, J. Xia, M. Li, L. Zhang, R. Ma, G. Zheng and Q. Zhang, Visible light-mediated NHCs and photoredox co-catalyzed radical 1,2-dicarbonylation of alkenes for 1,4-diketones, Sci. China: Chem., 2022, 65, 1938–1944 CrossRef CAS
.
- C. Prentice, J. Morrison, E. Zysman-Colman and A. D. Smith, Dual NHC/photoredox catalytic synthesis of 1,4-diketones using an MR-TADF photocatalyst (DiKTa), Chem. Commun., 2022, 58, 13624–13627 RSC
.
- J.-L. Li, S.-L. Yang, Q.-S. Dai, H. Huang, L. Jiang, Q.-Z. Li, Q.-W. Wang, X. Zhang and B. Han, Modular synthesis of 1,4-diketones through regioselective bis-acylation of olefins by merging NHC and photoredox catalysis, Chin. Chem. Lett., 2023, 34, 108271 CrossRef CAS
.
- S. Li, H. Shu, S. Wang, W. Yang, F. Tang, X.-X. Li, S. Fan and Y.-S. Feng, Cooperative NHC and Photoredox Catalysis for the Synthesis of 1,4-Dicarbonyl Compounds via Diacylation of Alkenes, Org. Lett., 2022, 24, 5710–5714 CrossRef CAS PubMed
.
- Y. Liu, Z. Wu, W. Li, M. Zhang, Y. Zhang, S. Deng, S. Fan, Y. Zhu and Y.-S. Feng, Direct access to β-arylketoesters by NHC/photoredox catalyzed alkoxycarbonylation–carbonylation of styrenes, Org. Chem. Front., 2023, 10, 3288–3292 RSC
.
- N. Takemura, Y. Sumida and H. Ohmiya, Organic Photoredox-Catalyzed Silyl Radical Generation from Silylboronate, ACS Catal., 2022, 12, 7804–7810 CrossRef CAS
.
- K. Liu and A. Studer, Direct α-Acylation of Alkenes via N-Heterocyclic Carbene, Sulfinate, and Photoredox Cooperative Triple Catalysis, J. Am. Chem. Soc., 2021, 143, 4903–4909 CrossRef CAS PubMed
.
- M.-S. Liu, L. Min, B.-H. Chen and W. Shu, Dual Catalysis Relay: Coupling of Aldehydes and Alkenes Enabled by Visible-Light and NHC-Catalyzed Cross-Double C–H Functionalizations, ACS Catal., 2021, 11, 9715–9721 CrossRef CAS
.
- H. W. Du, M. S. Liu and W. Shu, Synthesis of β-Thiolated-α-arylated Ketones Enabled by Photoredox and N-Heterocyclic Carbene-Catalyzed Radical Relay of Alkenes with Disulfides and Aldehydes, Org. Lett., 2022, 24, 5519–5524 CrossRef CAS PubMed
.
- T. Laurita, R. D'Orsi, L. Chiummiento, M. Funicello and P. Lupattelli, Recent Advances in Synthetic Strategies to 2,3-Dihydrobenzofurans, Synthesis, 2020, 52, 1451–1477 CrossRef CAS
.
- X. Yu, Q.-Y. Meng, C. G. Daniliuc and A. Studer, Aroyl Fluorides as Bifunctional Reagents for Dearomatizing Fluoroaroylation of Benzofurans, J. Am. Chem. Soc., 2022, 144, 7072–7079 CrossRef CAS PubMed
.
- J. Reimler, X.-Y. Yu, N. Spreckelmeyer, C. G. Daniliuc and A. Studer, Regiodivergent C−H Acylation of Arenes by Switching from Ionic- to Radical-Type Chemistry Using NHC Catalysis, Angew. Chem., Int. Ed., 2023, 62, e202303222 CrossRef CAS PubMed
.
- A. de Meijere, Bonding Properties of Cyclopropane and Their Chemical Consequences, Angew. Chem., Int. Ed. Engl., 1979, 18, 809–826 CrossRef
.
- Z. Zuo, C. G. Daniliuc and A. Studer, Cooperative NHC/Photoredox Catalyzed Ring–Opening of Aryl Cyclopropanes to 1−Aroyloxylated–3−Acylated Alkanes, Angew. Chem., Int. Ed., 2021, 60, 25252–25257 CrossRef CAS PubMed
.
- L. Fu, S. Greßies, P. Chen and G. Liu, Recent Advances and Perspectives in Transition Metal-Catalyzed 1,4-Functionalizations of Unactivated 1,3-Enynes for the Synthesis of Allenes, Chin. J. Chem., 2020, 38, 91–100 CrossRef CAS
.
- Y. Li and H. Bao, Radical transformations for allene synthesis, Chem. Sci., 2022, 13, 8491–8506 RSC
.
- L. Chen, C. Lin, S. Zhang, X. Zhang, J. Zhang, L. Xing, Y. Guo, J. Feng, J. Gao and D. Du, 1,4-Alkylcarbonylation of 1,3-Enynes to Access Tetra-Substituted Allenyl Ketones via an NHC-Catalyzed Radical Relay, ACS Catal., 2021, 11, 13363–13373 CrossRef CAS
.
- Y. Cai, J. Chen and Y. Huang, N-Heterocyclic Carbene-Catalyzed 1,4-Alkylacylation of 1,3-Enynes, Org. Lett., 2021, 23, 9251–9255 CrossRef CAS PubMed
.
- Y.-Q. Liu, Q.-Z. Li, X.-X. Kou, R. Zeng, T. Qi, X. Zhang, C. Peng, B. Han and J.-L. Li, Radical Acylalkylation of 1,3-Enynes To Access Allenic Ketones via N-Heterocyclic Carbene Organocatalysis, J. Org. Chem., 2022, 87, 5229–5241 CrossRef CAS PubMed
.
- L. Chen, J. Wang, C. Lin, Y. Zhu and D. Du, CF2Br2 as a Source for Difluoroolefination of 1,3-Enynes via N-Heterocyclic Carbene Catalysis, Org. Lett., 2022, 24, 7047–7051 CrossRef CAS PubMed
.
- L. Wang, R. Ma, J. Sun, G. Zheng and Q. Zhang, NHC and visible light-mediated photoredox co-catalyzed 1,4-sulfonylacylation of 1,3-enynes for tetrasubstituted allenyl ketones, Chem. Sci., 2022, 13, 3169–3175 RSC
.
- L. Wang, R. Ma, J. Xia, X. Liu, J. Sun, G. Zheng and Q. Zhang, DBU-Mediated Isomerization/6-pi Electro-Cyclization/Oxidation Cascade of Sulfonyl-Substituted Allenyl Ketones for the Construction of Hetero-1,3,5-Trisubstituted Benzene, Chem. – Eur. J., 2022, 29, e202203309 CrossRef PubMed
.
- C.-Y. Tan, M. Kim and S. Hong, Photoinduced Electron Transfer from Xanthates to Acyl Azoliums: Divergent Ketone Synthesis via N-Heterocyclic Carbene Catalysis, Angew. Chem., Int. Ed., 2023, 62, e202306191 CrossRef CAS PubMed
.
- J. Ye and S. Ma, Palladium-Catalyzed Cyclization Reactions of Allenes in the Presence of Unsaturated Carbon–Carbon Bonds, Acc. Chem. Res., 2014, 47, 989–1000 CrossRef CAS PubMed
.
- L. Liu, R. M. Ward and J. M. Schomaker, Mechanistic Aspects and Synthetic Applications of Radical Additions to Allenes, Chem. Rev., 2019, 119, 12422–12490 CrossRef CAS PubMed
.
- L. Wang, J. Sun, J. Xia, R. Ma, G. Zheng and Q. Zhang, Visible light-mediated NHC and photoredox co-catalyzed 1,2-sulfonylacylation of allenes via acyl and allyl radical cross-coupling, Org. Chem. Front., 2023, 10, 1047–1055 RSC
.
|
This journal is © the Partner Organisations 2023 |