DOI:
10.1039/D2SC04962G
(Review Article)
Chem. Sci., 2023,
14, 29-53
Inorganic-based biomaterials for rapid hemostasis and wound healing
Received
6th September 2022
, Accepted 7th November 2022
First published on 30th November 2022
Abstract
The challenge for the treatment of severe traumas poses an urgent clinical need for the development of biomaterials to achieve rapid hemostasis and wound healing. In the past few decades, active inorganic components and their derived composites have become potential clinical products owing to their excellent performances in the process of hemorrhage control and tissue repair. In this review, we provide a current overview of the development of inorganic-based biomaterials used for hemostasis and wound healing. We highlight the methods and strategies for the design of inorganic-based biomaterials, including 3D printing, freeze-drying, electrospinning and vacuum filtration. Importantly, inorganic-based biomaterials for rapid hemostasis and wound healing are presented, and we divide them into several categories according to different chemistry and forms and further discuss their properties, therapeutic mechanisms and applications. Finally, the conclusions and future prospects are suggested for the development of novel inorganic-based biomaterials in the field of rapid hemostasis and wound healing.
1. Introduction
Emergencies are still the number one reason for premature death worldwide; therefore, rapid hemorrhage control and effective wound healing are of great importance in medical care.1–3 Bleeding caused by minor wounds is usually stopped by intrinsic hemostatic mechanisms, and minor wounds can also be self-healed by the body. However, uncontrolled hemorrhage and healing difficulties following severe trauma are associated with high mortality.4,5 Rapid control of bleeding and promotion of wound healing are imperative and have motivated the development of hemostatic biomaterials and wound healing biomaterials. Hemostatic biomaterials with excellent absorption capacity and procoagulant ability can rapidly aggregate blood cells and platelets and accelerate the conversion of fibrinogen to fibrin in blood, thereby forming a physical barrier to prevent further bleeding.6 Wound healing biomaterials contribute to functions including antibacterial, immunomodulatory, fluid absorption, maintaining a moist environment, etc., which promote cell proliferation, angiogenesis and tissue regeneration, leading to a great reduction of the healing time.7 At present, the application of biomaterials in hemostasis and wound healing is mostly separated in clinical cases, and this results in a certain amount of inconvenience and waste. Meanwhile, more and more reports show that there is a tight connection between hemostasis and wound healing, which inspires researchers to prepare biomaterials for both rapid hemostasis and wound healing.8–13 Ideal biomaterials for hemostasis and wound healing should rapidly control bleeding, be easy to apply to different types of complex wounds, be biocompatible and non-cytotoxic, be good for wound healing and be easy to remove or biodegrade. Besides, the preparation process should be simple and efficient, and long-term stability during storage can be achieved.
The two main factors that need to be considered are the active chemical components and material forms when developing biomaterials for hemostasis and wound healing. Active chemical components can be divided into organic components and inorganic components according to chemical composition. Active organic components include natural polymers such as chitosan,14,15 alginic acid,16 gelatin,17 and synthetic polymers such as polyvinyl alcohol18 and GelMA.19 Active inorganic components include natural minerals,20 synthetic silicates,21 phosphates,22etc. Compared with organic biomaterials, inorganic biomaterials with unique advantages or characteristics show better potential in hemostasis and wound healing. On the one hand, inorganics generally exhibit much higher surface energy than organics, which makes them hydrophilic and even super-hydrophilic. Subsequently, inorganic biomaterials can quickly absorb water and achieve ultra-rapid hemostasis. On the other hand, inorganic biomaterials possess various functions like bioactive ion release, photothermal properties, magnetothermal properties, photodynamic properties or conductivity, which endow them with good prospects for future development. Hence, we mainly focus on exploring inorganic-based biomaterials for rapid hemostasis and wound healing in this review. Active inorganic components show the advantages of good biocompatibility, high bioactivity, absorption of blood, promotion of blood coagulation and release of bioactive ions, which have attracted the interest of researchers.7 However, active inorganic components are mostly micro–nano-scale particles whose applications are limited. Preparing inorganic-based biomaterials with more useful characteristics by combining novel methods, strategies and manufacturing techniques still remains a huge challenge. Many forms of inorganic-based biomaterials have been developed, ranging from simple to complex, from 2D to 3D, from dense to porous, from hydrophilic to hydrophobic and from macrosized to nanosized. Importantly, the manufacturing technique is the key to determining the material form that influences the functions and applications of an inorganic-based biomaterial. Representative manufacturing techniques include 3D printing,23–25 freeze-drying,26–28 electrospinning29,30 and vacuum filtration,31,32 which endow inorganic-based biomaterials with the forms of 3D scaffolds, porous bulk, soft membranes or tough hydrogels. The diverse forms of inorganic-based biomaterials are suitable for various and complex wounds—no matter hard or soft tissue wounds, or even superficial, deep, and irregular wounds. Hence, it is important to decide on appropriate active inorganic components and manufacturing techniques.
Generally, inorganic-based biomaterials for rapid hemostasis and wound healing are divided into three categories—inorganic particles, inorganic–polymer composite biomaterials and self-supporting inorganic-based biomaterials. Moreover, different material forms and active inorganic components endow these biomaterials with various properties, such as hemostasis, antibacterial activity, angiogenesis and degradation (Fig. 1). For hemostasis, natural mineral clays act as a molecular sieve to absorb water, thereby concentrating various clotting factors, platelets and blood cells over the site of the wound to form a plug.33 However, the exothermic reaction upon water absorption leads to thermal damage to surrounding tissue and even necrosis, severely limiting the application of natural mineral clays.34 Therefore, researchers have prepared silica-based mesoporous particles, such as mesoporous silica and bioactive glasses, which display good hemostatic properties and biocompatibility.35 Furthermore, various inorganic–polymer composite hemostatic materials and self-supporting inorganic-based hemostatic materials such as sponges,36,37 aerogels,38 hydrogels,39 and membranes40 have been developed to meet the needs of different clinical applications. These novel inorganic-based hemostatic biomaterials show inspiring development prospects because they have better hemostatic activity and can be applied to deep and complex wounds. As for wound healing, biomaterials with forms of basic dressings or scaffolds play the role of fundamental fixing and supporting during the wound healing process.41 On the other hand, multifunctional inorganic-based wound healing biomaterials have been developed to induce specific therapeutic effects on wound healing. These multifunctional biomaterials possess antibacterial, photothermal, immunoregulation, angiogenesis, and hair follicle regeneration functions, which can synergistically accelerate wound healing.42 Recently, intelligent responsive inorganic-based biomaterials have attracted attention because of their great importance in extending therapeutic methods of wound healing. Intelligent response means that the materials can respond to physical stimuli such as electricity, light, pH and temperature.43,44 It helps patients to monitor the wound healing process in time or regulate the appropriate healing microenvironment, so that biomaterials can promote wound healing more effectively.
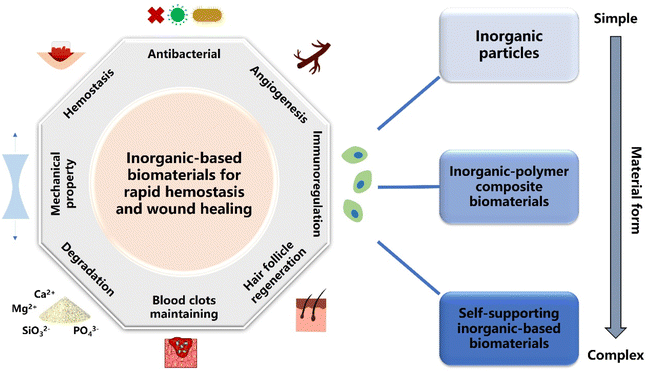 |
| Fig. 1 Schematic illustration of the three types of inorganic-based biomaterials classified by the complexity of the material form for various applications of rapid hemostasis and wound healing. | |
Many studies demonstrate a strong link between hemostasis and wound healing. In brief, the blood clots formed during hemostasis play an essential role in the subsequent wound healing process.45–47 Blood clots are composed mainly of platelets and fibrin, and they are a viable, dynamic matrix of proteins and cells, which not only contributes to hemostasis but also serves as a provisional lattice for incoming inflammatory cells, fibroblasts and growth factors.48 Moreover, blood clots could also regulate the immune microenvironment at the wound site during the process of formation and fibrinolysis.47 Therefore, if biomaterials could stop bleeding rapidly and be removed without causing secondary damage or be retained at the wound site for degradation, the remaining blood clots will further promote wound healing and achieve a better integrated treatment effect. To date, a number of inorganic-based biomaterials for rapid hemostasis and wound healing have been prepared, mainly including surface-modified natural mineral clays and inorganic–polymer composite sponges, which hold the abilities of excellent hemostatic performances, biocompatibility, and bioactive ion release behavior to promote wound healing.49–52 Meanwhile, scientists are developing inorganic-based biomaterials with multifunctional synergistic effects such as hemostasis, antibacterial, immunoregulation, photothermal therapy and wound healing. Therefore, using only one material to meet the therapeutic needs of complex wounds represents the promising development future of inorganic-based biomaterials for rapid hemostasis and wound healing.30,53–55 Nevertheless, there are several challenges of inorganic-based biomaterials for hemostasis and wound healing. Firstly, more and more active inorganic components with better effects of hemostasis and wound healing should be developed to address more complex clinical situations, such as the need for ultra-rapid hemostasis in the battlefield and skin regeneration of extensive cutaneous burns. Secondly, it is critical to fabricate active inorganic components in bulk forms instead of powder forms for extensive clinical application. Thirdly, the molecular mechanisms of inorganic-based biomaterials for rapid hemostasis and wound healing still need to be further investigated and explored.
In this review, our aim is to summarize the recent progress on inorganic-based biomaterials for rapid hemostasis and wound healing, especially focusing on the chemical components, preparation methods and strategies, and functional roles of hemostasis and wound healing. We provide an overview and discuss the development of chemical modification and manufacturing techniques over the past 10 years. Moreover, we describe the different forms of inorganic-based hemostatic biomaterials and wound healing biomaterials, highlighting their advantages, disadvantages and applications. Additionally, the designs, developments and challenges of novel inorganic-based biomaterials for both rapid hemostasis and wound healing and multifunctional synergetic inorganic-based biomaterials are also discussed to inspire more in-depth research in this area.
2. Methods and strategies for the design of novel inorganic-based biomaterials
Clearly, material design is the key process that determines the structures, properties and performances of materials, which has inspired plenty of researchers to explore various methods and strategies for preparing inorganic-based biomaterials. As known, a variety of active inorganic components ranging from nano- to micro-scales have been synthesized by chemical methods such as hydrothermal, sol–gel, solid reaction, precursor-derived method, etc.56–59 Nevertheless, it is difficult to directly apply these active inorganic components in clinics. Therefore, preparing useable inorganic-based biomaterials by utilizing suitable manufacturing techniques is extremely essential. In this section, we provide an overview of several common manufacturing techniques, and subsequently focus on how these manufacturing techniques determine the forms, properties and applications of inorganic-based biomaterials.
2.1. 3D printing
3D printing has become a critical technique to manufacture clinical biomaterials.60 As known, 3D printing is to develop materials with a three-dimensional structure through layer-by-layer stacking. Conventionally, the 3D printing process can be simply described as follows: first, a 3D model is established with computer-aided design (CAD) software, and then the established 3D model is printed along a preset path through a robotic arm, a nozzle or other apparatus.61 Nowadays, the 3D printing technique has also been widely applied in fabricating biomaterials for hemostasis and wound healing. The biomaterials prepared by the 3D printing possess reliable mechanical strength, good biocompatibility and a connected macroporous structure. Hence, they can play a role of support and fixation at the wound site and further provide channels for cell migration and nutrient delivery.
2.1.1. Extrusion-based 3D printing.
Extrusion-based 3D printing is the earliest developed technology of 3D printing. Under the preset CAD program, the nozzle-loaded ink is printed and stacked layer by layer to obtain a three-dimensional scaffold. For inorganic-based 3D printed scaffolds, high temperature treatment could remove the organic binder and densify the particles, thereby greatly improving their mechanical strength.62,63 For instance, a 3D scaffold dressing composed of decellularized small intestinal submucosa, mesoporous bioactive glass and exosome was fabricated by Hu et al. The introduction of mesoporous bioactive glass increased the printability of the slurry and the bioactivity of the scaffolds. Interestingly, this scaffold dressing could accelerate diabetic wound healing through increasing the blood flow of the wound and stimulating the angiogenesis process.64 For a long time, our group have 3D printed a series of inorganic-based scaffolds based on bioceramics and bioglasses. These scaffolds have cross-connected porous structures of hundreds of microns that provide an environment for nutrient delivery and cell migration. Furthermore, the released bioactive ions from these scaffolds can also promote cell proliferation and differentiation.65–68 In another study, Li et al. fabricated a dopamine-modified bioceramic scaffold of which the mussel-inspired surface could stimulate the paracrine effect of adipose-derived MSCs. Interestingly, such a bioceramic scaffold showed abilities to accelerate wound closure and enhance vascularization.69
2.1.2. Digital laser processing-based 3D printing.
Digital laser processing-based 3D printing is a manufacturing technique based on a light-activated polymerization reaction, in which polymers are polymerized through selective laser irradiation to form a three-dimensional structure.70 Combining bioceramics with polymers, scientists can fabricate inorganic-based 3D printed scaffolds with a complex and precise internal structure through digital laser processing-based 3D printing.71 For instance, Sakurai et al. investigated the principal components of hemostasis based on a microengineered vascularized bleeding model. Contributing to the excellent rheological properties and formability of polydimethylsiloxane (PDMS), a bleeding model consisting of three PDMS layers that mimic the structure of a natural blood vessel had been successfully fabricated. The experimental results further showed the essential functions of platelets, von Willebrand factor and endothelial phosphatidylserine in hemostasis. This biomimetic bleeding model held significant promise as a therapeutic-guiding tool for hemostasis.72 In another study, Kim and co-workers developed a methacrylated photocurable silk fibroin bioink with excellent printability, mechanical and rheological properties, and biocompatibility, which could be used broadly including in nerve tissue engineering, trachea tissue engineering and wound healing.73 Inspired by the mechanical properties of natural blood vessels, Bracaglia et al. designed a printable and degradable hybrid scaffold. The experimental results showed that the phenotype of macrophages could be affected by changing the components of this scaffold, thereby regulating inflammation and promoting wound healing.74
2.1.3. 3D bioprinting.
With the development of tissue engineering, the traditional “inanimate” 3D printing scaffolds are difficult to meet the complex clinical needs. Therefore, 3D bioprinting has been proposed and flourished. The most significant feature of 3D bioprinting is the use of “bioink” that contains living cells. Through 3D bioprinting, cells can be pre-grown, differentiated in vitro, and then implanted into the body, which greatly improves the bioactivity and therapeutic effect of the materials.75 For example, Biranje et al. designed a cellulose nanofibril and casein composite bioink that has a viscoelastic behavior, stability, homogeneity and better printability. Furthermore, the 3D printed cell-laden composite scaffold could promote the growth and proliferation of NIH 3T3 fibroblast cells.76 In another study, Turner et al. reported a novel double-peptide functionalized GelMA bioink and directly deposited BMSCs and HUVECs to prepare a core/shell bioprinting scaffold. Encouragingly, this core/shell scaffold showed good physico/chemical characteristics that supported the propagation and early development of vascular cells in a tube-like structure.77 Recently, our group reported a Li–Mg–Si bioceramic-GelMA hydrogel composite bioink, which was utilized to construct a co-culture scaffold containing bone marrow mesenchymal stem cells (hpMSCs) and chondrocytes (RCs). Owing to the introduction of Li–Mg–Si bioceramic, the printability of bioink was improved and the bioactivity of the scaffold was also increased. In vitro and in vivo experiments have verified that the scaffold containing Li–Mg–Si bioceramic exhibited the function of stimulating multiple cells for differentiation towards specific directions, thereby accelerating the repair and regeneration of severe osteochondral defects.78
2.2. Freeze-drying
Freeze-drying, also known as ice templating, is a particularly versatile technique. It has been applied extensively for the fabrication of well-controlled biomimetic porous materials based on ceramics, metals, polymers, biomacromolecules and carbon nanomaterials.79 Briefly, freeze-drying involves the controlled solidification of a solution, suspension, sol or gel, followed by the sublimation of the solvent under reduced pressure.80,81 Moreover, one outstanding advantage of freeze-drying is that the micro/macro-structures of the prepared freeze-drying scaffolds can be easily tailored by adjusting the process parameters.82 Therefore, biomaterials manufactured by freeze-drying have been developed into the most common forms for rapid hemostasis and wound healing benefitting from their porous structure and absorption capacity.
2.2.1. Unidirectional freeze-drying.
In the case of conventional unidirectional freezing-drying, the suspension starts to freeze under a single temperature gradient, causing the nucleation of ice to occur randomly on the cold surface and grow radially. As a result, the solidified suspension media consist commonly of microscale crystallites oriented preferentially along the direction of freezing, and constructing scaffolds with cellular porous structures.79 For instance, Yuan et al. developed a novel hemostatic nanocomposite fabricated by coupling oxidized bacterial cellulose and chitosan with collagen. These three raw materials possessed both good formability and excellent hemostatic properties, and excellent hemostatic efficacy of the composite was confirmed in vivo.83 Huang et al. prepared a series of biodegradable interpenetrating polymer network (IPN) dry cryogel hemostats. Owing to the IPN structure, the hemostat showed good injectability, robust mechanical and shape memory properties.84 Similarly, a series of antibacterial and antioxidant tissue-adhesive cryogels based on chitosan and polydopamine were designed by Li and co-workers, and these cryogels possessed good platelet adhesion, enrichment and activation properties.85 In another study, Lan et al. fabricated a chitosan/gelatin/sodium hyaluronate hemostatic dressing with silver nanoparticles. Additionally, this dressing had high blood absorption and promoted platelet aggregation by the activation of a positively charged surface and stimulation of silver nanoparticles.86
2.2.2. Bidirectional freeze-drying.
Apart from unidirectional freeze-drying, bidirectional freeze-drying is another important manufacturing technique in the preparation of biomaterials with “brick and mortar” (BM) structures.87 In bidirectional freezing, solid phase building blocks assemble into a large-size single-domain aligned lamellar structure, which is attributed to the controlled nucleation of ice crystals that allow the ice to grow both vertically and horizontally.88,89 From this perspective, active inorganic components with the morphology of nanosheets or nanowires are more likely to be fabricated into biomaterials with lamellar structures through bidirectional freeze-drying. For example, Bai and co-workers first reported on a bidirectional freezing technique to successfully assemble ceramic particles into a scaffold with a large-scale aligned, lamellar, porous, nacre-like structure and long-range order on the centimeter scale. Encouragingly, the scaffold possessed extremely high compressive strength.90 In a similar case, Li et al. of our group utilized akermanite and the bidirectional freeze-drying technique to construct hot dog-like biomaterials and “brick-and-mortar” structural biomaterials. These scaffolds displayed excellent properties including the high loading and sustained release of drugs and proteins and the improvement of the migration and differentiation of tissue cells.91,92 In addition to the above, Feng et al. reported a bio-inspired lamellar chitosan scaffold with a long range ordered porous structure. Most interestingly, the chitosan scaffold was found to be capable of inducing macrophage differentiation to the M2 phenotype that played an important role in tissue regeneration.93
2.3. Electrospinning
Electrospinning is the most widely developed manufacturing technique for preparing soft fiber membrane materials. Conventionally, a typical apparatus for electrospinning is cost-effective and straight forward. It consists of a high voltage supplier, a capillary tube with a small diameter needle or pipet, and a grounded fiber collecting screen.94,95 As is known, inorganic-based bio-membranes can be prepared by mixing bioceramic powders and polymers, while the physicochemical properties can be flexibly adjusted. Importantly, inorganic-based bio-membranes possessing excellent flexibility can be wrapped and applied to wounds like bandages. Furthermore, they show the multiple functions of hemostasis, bioactive ion release and wound healing promotion, thereby having great potential in tissue engineering.
Electrospinning bio-membranes have excellent flexibility, mechanical strength and absorption capacity, which can be easily used in hemostasis, healing and therapy of soft tissue wounds. Hence, a series of studies have illustrated their applications in hemorrhage control. For instance, Li et al. prepared a curcumin-loaded mesoporous silica incorporated nanofiber mat. The mesoporous silica with an extremely high surface area exhibited excellent hemostatic activity. Interestingly, the hybrid nanofiber mat could rapidly transform into a hydrogel when contacted with blood, and then activate the clotting system to stop bleeding.97 Long and co-workers described an emerging bifunctional hybrid fiber membrane incorporated with ZnO–Fe2O3 and kaolinite nanoclay for the first time, and the results showed that ZnO–Fe2O3 and kaolin possessed hemostatic, antibacterial and anti-inflammatory activities for favorable therapeutic efficacy.98
In addition to hemostasis, here are their extensive developments and applications in skin or other soft tissue wound healing. Inspired by the design strategy and fabrication method of Chinese sesame sticks, our group developed a scaffold through spin coating of CaCuSi4O10 nanoparticles on the surface of electrospinning fibers (As shown in Fig. 2). Notably, the CaCuSi4O10 nanoparticles not only improved the stability of this fiber scaffold, but also endowed the scaffold with high bioactivity. In vivo evaluation proved that the scaffold could release bioactive Cu2+ and SiO44− to promote chronic wound healing.96 In another study, Chen and co-workers prepared a nanobioglass incorporated chitosan-PVA tri-layer nanofibrous membrane. Interestingly, the spatially designed structure optimized the functions of each component. In vivo experiments further showed that this membrane could significantly accelerate wound healing.99 Additionally, Balakrishnan et al. reported an Al2O3-based PVP nanofibrous scaffold. Contributing to the incorporation of Al2O3, the composite nanofibrous scaffold not only possessed improved porosity and degradation but also obtained a sustained release of Al2O3 nanoparticles to promote cell proliferation.100
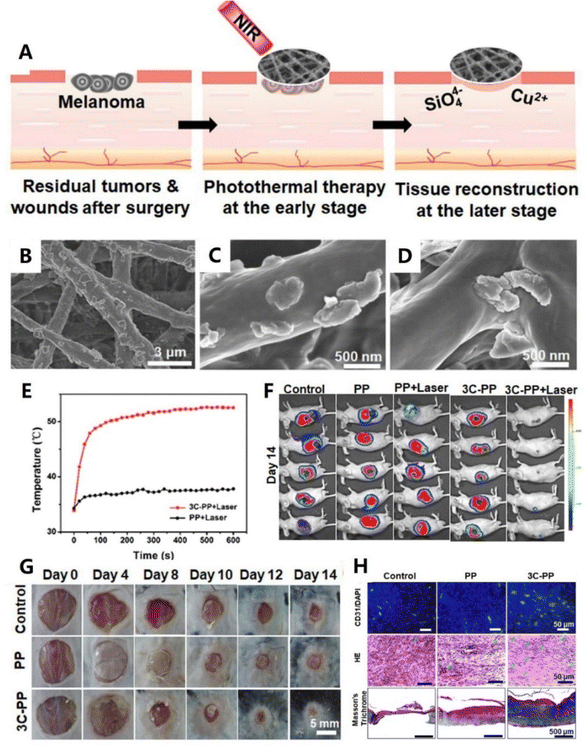 |
| Fig. 2 (A) Schematic illustration of the CaCuSi4O10 nano-sized particle (NP)-coated fibrous scaffolds for photothermal therapy and tissue regeneration. (B–D) SEM images of the CaCuSi4O10 NP-coated fibrous scaffolds. (E) Temperature changes of the tumors treated with laser-irradiated PP and 3C-PP scaffolds (0.45 W cm−2, 15 min). (F) The whole-body fluorescence imaging of tumors on day 14 (n = 5). (G) Wound photographs at chronic wounds of different groups after fibrous scaffold implantation. (H) Immunofluorescence (green: CD31 and blue: nuclei), H&E staining and Masson's Trichrome staining images of skin wounds on day 14. Adapted with permission from ref. 96. Copyright 2018, Elsevier Ltd. | |
2.4. Vacuum filtration and evaporation-induced self-assembly
Vacuum filtration and evaporation-induced self-assembly are also two typical manufacturing techniques to fabricate membrane materials. As is known, these two methods directly utilize building blocks and perform a simple bottom-up and layer-by-layer stacking strategy to fabricate inorganic-based bio-membrane materials.101 Besides, the physicochemical properties of the membranes can be easily controlled by adjusting the concentration of the building block suspension. Consequently, bio-membrane materials with a high inorganic content can be prepared by vacuum filtration or evaporation-induced self-assembly techniques. These bio-membrane materials show great potential in clinical treatment due to their excellent mechanical properties and bioactivities.
Numerous studies have presented inorganic-based bio-membranes with favorable performances by using vacuum filtration or evaporation-induced self-assembly. For instance, Mansoorianfar et al. prepared densely packed cellulose layers impregnated with 58S bioglass nanoparticles. Interestingly, the effective integration of bioglass nanoparticles between cellulose interlayers increased the hemostatic activity of the developed fabric by 50%.102 Inspired by the close relationship between the strength and hierarchical structure of nacre, Xue et al. fabricated a hierarchical and porous graphene oxide–chitosan–calcium silicate membrane biomaterial, in which graphene oxide was the main component, chitosan acted as a binder and calcium silicate provided bioactivity. Moreover, this bioinspired hierarchical material possessed high tensile strength, compatible breathability, water absorption and ideal photothermal performance, which significantly promoted wound healing.103 For evaporation-induced self-assembly, Yu and co-workers demonstrated that a large-sized, three-dimensional bulk artificial nacre could be facilely fabricated via a bottom-up assembly process. Furthermore, this artificial nacre comprehensively mimicked the hierarchical structures and toughening mechanisms of natural nacre based on laminating pre-fabricated two-dimensional nacre-mimetic membranes. Obviously, such three-dimensional bulk artificial nacre would be promising for hemostasis and wound healing owing to its porous and hierarchical structure.104,105
3. Inorganic-based biomaterials for rapid hemostasis
3.1. Hemostasis mechanisms
Hemostasis, or coagulation, is a naturally occurring process that results in forming a stable and insoluble blood clot at an injury site to prevent further bleeding.106 The formation of blood clots is activated within a few seconds of injury and localized only at the wound site.107 Therefore, coagulation is a very accurate process to avoid the risk of thromboembolism.108 Hemostasis primarily consists of two stages, primary and secondary hemostasis. Fig. 3 schematically presents the details of the hemostasis process.
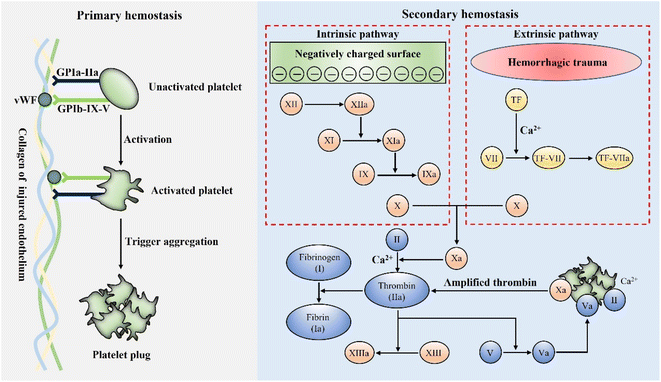 |
| Fig. 3 Schematic illustration of the mechanisms of primary hemostasis and secondary hemostasis. | |
Generally, the primary hemostasis occurs immediately when bleeding occurs. In the process of primary hemostasis, platelets quickly adhere to the injured endothelium and are activated through specific receptors, namely GPIa-IIa and GPIb-IX-V.109,110 The activated platelets then trigger the aggregation and formation of a platelet plug to stop bleeding.111,112 However, the platelet plug is fragile and the secondary hemostasis is essential to further form a stable blood clot. In the process of secondary hemostasis, coagulation factors undergo a complex coagulation cascade, which includes extrinsic and intrinsic pathways. The extrinsic pathway is initiated by blood exposure to tissue factor (TF) and eventually leads to the activation of factor Xa, while the intrinsic pathway is initiated when blood contacts a negatively charged surface, which also results in the activation of factor Xa. Subsequently, the common factor Xa can produce a large amount of prothrombin (II) into thrombin (IIa) under the synergetic effect of phospholipids and Ca2+.113 As is known, thrombin is the key factor, which can convert fibrinogen (I) into fibrin (Ia), and the interwoven fibrin entangles platelets and blood cells to form a firm and stable blood clot at the bleeding site, which can stop bleeding more effectively.109,111
For hemostatic materials, it is possible to accelerate hemostasis by regulating a certain process in the complex coagulation reaction through specific properties. As an example, anionic polysaccharides represented by chitosan possess a positively charged group –NH3+, which can accelerate coagulation by aggregating and activating platelets in hemostasis.114,115 Besides, natural mineral clays such as zeolites and kaolin exhibit a mesoporous structure and Ca2+ release ability to promote hemostasis.33 In the following section, we focus on four major types of inorganic-based hemostatic materials, further discussing their properties and hemostatic mechanisms.
3.2. Natural mineral clays
As is known, natural mineral clays are composed of unreactive oxides of magnesium, aluminum, sodium, silicon, and minute amounts of quartz. These agents can rapidly absorb water to gather platelets, blood cells and coagulation factors at the injury site. They can also activate the secondary hemostasis, thereby accelerating blood clotting.33 Zeolites, kaolin, and montmorillonite are the three most widely used natural mineral clay hemostats. Furthermore, a variety of inorganic-based hemostatic materials based on natural mineral clays have been approved for battlefield trauma, field first aid and clinical hemostasis.
3.2.1. Zeolites.
Zeolites, microporous crystalline aluminosilicate minerals found in nature, are composed of a tetrahedral SiO2 framework to form a three-dimensional structure.116 When in contact with blood, zeolites can absorb a large volume of water to gather platelets, blood cells and coagulation factors. Meanwhile, the released Ca2+ from zeolites activates the intrinsic pathway, further accelerating coagulation. In a recent study, Shang et al. revealed the hemostatic mechanism of Ca-zeolites at the molecular level.117 A prothrombin-to-thrombin conversion on the surface of Ca-zeolite was observed and it displayed a striking thrombin activation pattern, which exhibited an exceptionally high plateau thrombin activity and at least 12-fold enhanced endogenous thrombin compared to natural platelet-based physiological processes. Remarkably, various zeolite-based inorganic hemostatic materials have been marketed. QuikClot granular powder (QC) was approved for application by the FDA in 2002. For instance, Margulis et al. demonstrated that QC induced an immediate and continuous hemostasis.118 Similarly, Rhee et al. reported that QC controlled bleeding in 92% of cases for 103 military and civilian patients.119 However, QC had a serious side effect of causing tissue thermal damage. Wright and co-workers found that QC could cause an increased temperature at both the surface and in the interior of the tissue of about 95 °C and 50 °C.120 To deal with this challenge, Ostomel et al. modified a zeolite-based hemostatic agent by exchanging Ca2+ with Ag+. Hence, they greatly reduced the heat generation and endowed the hemostatic agent with an antibacterial ability.121 In another study, Yu and co-workers constructed an artificial catalyst composed of trypsin and a Ca2+-exchanged Y-type zeolite. This artificial catalyst exhibited strong hemostatic ability in animal models of massive hemorrhage and hemophilia without causing tissue thermal damage.122
3.2.2. Kaolin.
Kaolin is a hydrous aluminum silicate mineral, which exhibits a relatively low specific surface area, low cation exchange capacity and a minimal charge on the layer.123,124 There are several factors including the “glass effect” and negative surface charge that cumulatively contribute to the ability of kaolin in accelerating the body's natural blood clotting.125 Conventionally, the marketed kaolin-based inorganic hemostatic materials are mainly QuikClot Combat Gauze™ (QCG) approved by the FDA in 2013. QCG acts as a procoagulant, and when it comes in contact with blood, the kaolin immediately dissociates from the gauze and activates the intrinsic pathway. However, QCG is a non-absorbable hemostatic dressing that does not offer immediate hemostasis.126 For example, Gegel et al. assessed the efficacy of QCG in vivo and demonstrated that QCG could form a more robust thrombus to significantly reduce blood loss.127 Chavez-Delgado et al. further applied QCG to hemostasis of 230 patients undergoing tonsillectomy, and QCG achieved rapid and complete hemostasis in 84.8% of patients.128 Currently, inspired by a traditional Chinese medicine hematitum which consisted of iron oxide, Long and co-workers developed an emerging kaolin nanoclay composite (α-Fe2O3-kaolinKAc) through a facile precipitation method based on natural hemostatic agents. The results showed that the emerging kaolin nanoclay composite exhibited the properties of absorbing fluid, concentrating coagulation factors and activating the intrinsic coagulation pathway. Besides, α-Fe2O3 in this nanoclay composite could also promote blood cell aggregation and coagulation to further promote hemostasis.129
3.2.3. Montmorillonite.
Montmorillonite is also a hydrous aluminum silicate mineral, which possesses various properties, including a large specific surface area, cation exchange capacity and high viscosity due to its small particle size.124,130 When exposed to blood, the montmorillonite can absorb a large volume of water, thereby aggregating coagulation factors. Furthermore, the negative surface charge of montmorillonite also activates the intrinsic coagulation pathway.130 Montmorillonite has been commercialized as WoundStat™ (WS) approved by the FDA in 2007. WS can rapidly absorb water and swell into a clay paste with high plasticity and strong adhesiveness to form a physical barrier and stop bleeding. Nevertheless, WS is non-biodegradable and must be removed by physical means. What's more, residual WS particles in the body can easily lead to thromboembolism and complications. As an example, Ward et al. reported the application of WS in an arterial hemorrhage swine model, and histological examination revealed residual particles in the arteries, which might lead to thrombosis.131 In another study, Kheirabadi et al. evaluated the safety of WS. The results indicated that the majority of WS-treated vessels were occluded by intravascular thrombus formation, suggesting that WS might lead to thromboembolic risk.132 Although montmorillonite was reported to be an effective hemostatic agent to address massive bleeding, the inflammation and thrombosis led to restricted application.
3.3. Silica-based mesoporous particles
Recently, mesostructured materials such as mesoporous silica, mesoporous bioactive glasses, diatom silica and their composites have opened up a new direction in the field of hemostasis. Similar to natural mineral clays, silica-based mesoporous particles can rapidly absorb water and aggregate platelets, blood cells and coagulation factors at the injury site. On the other hand, the negative surface charge and released Ca2+ from silica-based mesoporous particles also activate the intrinsic pathway.35 Furthermore, silica-based mesoporous particles show much better biosafety than natural mineral clays, because silica or silicate processes excellent biocompatibility and degradability. Therefore, silica-based mesoporous particles displayed greater potential in hemostasis and have been extensively studied and applied.
3.3.1. Mesoporous silica.
Mesoporous silica (MS) has currently attracted worldwide attention because of its significant features including biocompatibility, tailorable surface charges, large pore sizes and pore volumes.133,134 For hemostasis, MS with a high specific surface area and porosity can promote hemostasis by absorbing a large amount of water and condensing coagulation factors.135 For example, Dai et al. developed a silver exchanged calcium doped ordered mesoporous silica sphere (AgCaMSS) for hemorrhage control. The AgCaMSS showed a pore size of 3.2 nm, BET surface area of 919 m2 g−1 and pore volume of 0.74 m3 g−1. In vivo experiments further demonstrated that the AgCaMSS could significantly promote blood clotting, activate the intrinsic pathway and induce platelet adherence.136 In another study, Wang and co-workers prepared tannic acid-loaded mesoporous silica nanoparticles (TMS). Hemostasis tests proved that the TMS could reduce the hemostatic time by 65% both in vitro and in vivo. TMS also exhibited antibacterial activity against Staphylococcus aureus and Staphylococcus epidermidis.137 Similarly, Bhat et al. developed new gated mesoporous silica nanoparticles (MSN) loaded with an anticoagulant drug. Moreover, the thrombin-dependent response was assessed and a significant increase in the coagulation time from 2.6 min to 5 min was found.138 Sun et al. aimed to improve the hemostatic activity of chitosan through incorporating MS and they eventually developed porous chitosan-silica composite microspheres (CSMS-S). Interestingly, CSMS-S exhibited abundant surface and inner macropores, which were important for rapid hemostasis. The whole blood clotting kinetics further showed that CSMS-S could form larger blood clots and reduce the hemostatic time from 114 s to 97 s.139
3.3.2. Mesoporous bioactive glasses.
A new generation of nanostructured bioceramics, referred to as mesoporous bioactive glasses (MBGs), was first developed by Yan et al. in 2004 via a sol–gel route process.140 It's worth noting that MBGs share a similar mesoporous structure to MS. Moreover, MBGs possess a large surface area, large pore volume and Ca2+ release for good hemostatic activity.141,142 Additionally, Ostomel et al. further investigated the hemostatic activity of MBGs. Encouragingly, they demonstrated that MBGs with a higher Si
:
Ca ratio (80% Si) possessed better pro-coagulation activity.143 Pourshahrestani and co-workers developed MBGs containing various concentrations of Ga2O3. The incorporation of a lower Ga2O3 content (1 mol%) into the MBG system improved structural properties including specific surface area, mesopore size and pore volume, which endowed MBGs with excellent hemostatic and antibacterial effects.144 Similarly, Mendonca et al. reported MBGs containing tantalum along with their potential application as hemostats. It was found that the Ta incorporation in MBGs caused a decreased surface area and pore volume. However, the Ta-MBGs still showed a significant hemostatic ability, revealing that Ta had a special hemostatic activity.145 In another research study, Zheng and co-workers combined MBGs and corn starch porous microspheres (CMSs) to prepare MBG@CMS particles. Notably, the MBG@CMS particles possessed a higher water absorption rate and activated both intrinsic and extrinsic coagulation pathways, thereby significantly shortening the blood clotting time.146
3.3.3. Diatom silica.
The recent advance in biotechnology has accelerated the discovery and development of new materials. For example, diatom silica is a precisely tuned nanostructured silica biomaterial with 3D porous structures.147 The diatom silica possesses various shapes, sizes, hierarchical pores, high porosity and specific surface area.148 Consequently, these features endow diatom silica with abilities of absorbing water, concentrating coagulation factors and promoting hemostasis.149 Lee et al. explored dramatic differences of special surface wettability between natural diatom silica and synthetic silica. The results showed that the surface properties of synthetic silica were hydrophobic and hemophobic, while diatom silica exhibited superhydrophilicity and even superhemophilicity. Apparently, such superhydrophilicity of diatom silica did not solely originate from nanoporous structures but from the synergy of high-density silanol anions and the nanoarchitecture, thus demonstrating the hemostasis potential of diatom silica.150 In another study, Luo et al. isolated the diatom Navicula australoshetlandica sp. to obtain diatom silica. Interestingly, this diatom silica had a specific “porous web” (6–8 nm) substructure in the ordered nanopores (165–350 nm) and they further exhibited a low hemolysis rate and shortened blood clotting time in vivo.151 Feng and co-workers developed a series of chitosan-coated diatoms (CS-diatoms) for hemorrhage control. As a result, the CS-diatoms prepared with 1% chitosan exhibited favorable biocompatibility, great fluid absorption and a desirable hemostasis effect.152 Likewise, Wang prepared chitosan/dopamine/diatom-silica composite beads (CDDs), and found that the porous internal structure of CDDs led to a large amount of water absorption, which contributed to rapid hemostasis.153
3.4. Inorganic–polymer composite hemostatic materials
In order to better take advantage of high hemostatic activities of inorganic hemostatic materials, researchers have prepared inorganic–polymer composite hemostatic materials by combining active inorganic components with polymers.37,154–156 The polymers mainly provide supporting structures and partial hemostatic activities, while the inorganic active components afford the major hemostatic and therapeutic functions. It is a common way to achieve a uniform and stable inorganic–polymer composite by combining active inorganic components and polymers. When applied to wounds, inorganic particles in the composites will not fall off or come into direct contact with the wound site to cause damage. Moreover, the gradual release properties of active inorganic components in the composites are beneficial to the wound healing process, which will not cause the sharp change of ion concentration and pH in the microenvironment. Therefore, inorganic–polymer composite biomaterials possess better biosafety and biocompatibility compared to powders. Additionally, the most obvious feature of inorganic–polymer composite hemostatic materials is their various material forms including sponges, membranes and hydrogels, endowing the composites with extensive applications.
3.4.1. Sponge composites.
As is known, sponge materials with high hydrophilicity and absorption capacity have been proven to be effective for promoting coagulation, owing to their ability of concentrating platelets, blood cells and coagulation factors. Conventionally, a sponge composite hemostat is fabricated through a specific molding technique (typically freeze-drying). Furthermore, these composite materials consisting of several active components can enable synergetic activation of the coagulation cascade and achieve better hemostasis. For instance, Jiang et al. used loose corn stalk and silver particles to adjust the solo chitin sponge. The prepared sponge showed a higher blood absorption ratio and lower blood clotting index.37 Pourshahrestani and co-workers constructed a series of 1% Ga2O3-containing mesoporous bioactive glass–chitosan composite sponges. The hydrophilicity and blood absorption rate of this sponge were significantly improved due to the high surface area of mesoporous bioactive glass. The results further revealed that the composite sponge exhibited increased capability to enhance thrombus generation and blood clotting.154 Similarly, Li et al. developed a hemostatic chitosan/diatom-biosilica-based aerogel. It's worth noting that the strong interface effect between dilation-biosilica and blood was favorable for promoting platelet aggregation and activating intrinsic pathway.155 In a recent study, Yu et al. tightly bound a mesoporous single-crystal zeolite onto the surface of cotton fibers to prepare a flexible zeolite-cotton hybrid hemostat (Fig. 4). On the one hand, zeolite particles were firmly anchored onto the cotton surface, thereby preventing tissue thermal damage. On the other hand, this hemostatic device had superior hemostatic performance over most other clay or zeolite-based inorganic hemostats.156 In another study, Zhang and co-workers synthesized mesoporous silica particles with large mesopores and fixed them on cotton fibers. Notably, this modified-cotton showed a shorter blood clotting time (86.00 s) and less blood loss (0.02 g) in vivo.157
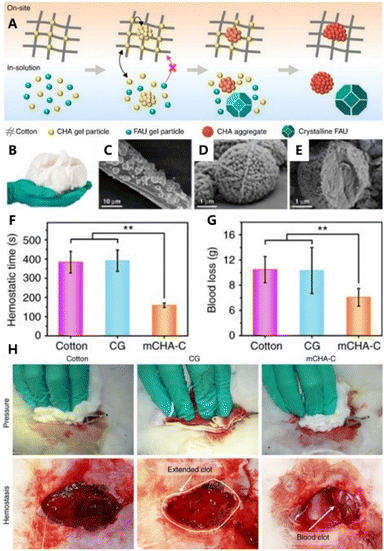 |
| Fig. 4 (A) Schematic representation of the tentative formation process mechanism of mCHA-C of on-site and in-solution products. (B) Photograph of mCHA-C. FE-SEM images of (C) mCHA-C, (D) mCHA zeolite on cotton, and (E) mCHA zeolite after removing cotton fiber by calcination. Quantitative analysis of hemostatic time (F) and blood loss (G) in a rabbit femoral artery injury model. (H) Hemostasis was assessed upon manual pressure on a rabbit lethal femoral artery injury with cotton, CG, or mCHA-C. Adapted with permission from ref. 156. Copyright 2019, CC BY 4.0. | |
3.4.2 Membrane composite.
Inspired by battlefield bandage, many inorganic–polymer composite membrane materials have also been developed. Different from sponge materials, the membrane hemostatic materials can be either hydrophilic158 or hydrophobic.159 On the one hand, the hydrophilic membranes can absorb water to aggregate coagulation factors. On the other hand, the hydrophobic membranes can act as a physical barrier to stop further bleeding. For example, Cui et al. reported a rapid effective nanoclay-based hemostatic membrane (NEM). The NEM with 60 wt% kaolinite (KEM) showed excellent hemostatic performance in vitro and in vivo benefiting from its enriched hemostatic functional site, robust fluffy framework, and hydrophilic surface. Furthermore, these results indicated that NEM acute hemostatic bandages were promising candidate materials for compressible hemorrhage control applications.40 Likewise, Delyanee M. and co-workers fabricated an electrospinning poly (lactic acid) and amino-modified halloysite nanotube (PLA/HNT) biomembrane. Interestingly, the negative surface charge of this biomembrane was greatly increased owing to the incorporation of HNTs and the intrinsic pathway of the coagulation cascade was activated faster. Hence, the blood clotting formation time decreased from 9 to 4 min.160 Chen et al. synthesized urushiol-functionalized mesoporous silica nanoparticles (MSN@U), which could form an amphipathic Janus membrane by interfacial self-assembly. The results showed that the Janus membrane possessed a large specific surface area, a rich porous structure and an ability of accelerating clotting cascade reactions.161 In another study, Jia et al. prepared a chitosan/mesoporous bioactive glass membrane for rapid hemostasis. The bioactive glasses with a mesoporous structure and high surface area endowed this membrane with better hydrophilicity and hemostatic activity. The results showed that the porous membrane possessed a good water absorption character and procoagulant activities in hemostasis.162
3.4.3 Hydrogel composite.
In addition to sponge and membrane composites, hydrogel-based hemostatic materials have also been extensively investigated and developed in recent years. In hemostasis, the hydrogel composite with good water absorption capacity can form a physical barrier at the wound site to stop bleeding after swelling and provide a moist ECM.163–165 For instance, Li et al. manufactured a maltose-like injectable hemostatic nanocomposite via combining chitosan polysaccharide and clay rectorite. Contributing to the hemostatic properties of rectorite, the viscous nanocomposite decreased the in vitro clotting time by 43%. Besides, an in vivo porcine skin model further confirmed the hemostatic performances of the viscous nanocomposite.166 Inspired by the great adhesive behavior of mussels and Arion subfuscus, Fan and co-workers prepared a novel polyacrylamide-tannic acid-kaolin hydrogel. Notably, the kaolin nanoparticles served as not only a physical crosslinking agent, but also an activator of the blood clotting factor FXII for accelerating coagulation.167 In another study, an injectable antibacterial conductive cryogel hemostat was reported by Zhao. This carbon nanotube incorporated cryogel displayed robust mechanical strength, rapid blood-triggered shape recovery and absorption speed together with high blood uptake capacity. Moreover, carbon nanotubes were discovered to be an activator of platelets and accelerate primary hemostasis.168 Mesoporous particles were also utilized to compound with polymer hydrogels. In two studies presented by Sundaram et al. and Shen et al., MBG or MS and chitosan were composited into injectable hydrogels. Owing to the incorporation of mesoporous particles, these two kinds of hydrogels exhibited remarkably increased hemostatic effects.169,170
3.5 Self-supporting inorganic-based hemostatic materials
Generally, the content of active inorganic components in inorganic–polymer composites is relatively low (usually less than 30%),37,156,167,171 which motivated researchers to investigate hemostatic materials with both a high inorganic content and self-supporting structure. However, the major factor of materials to maintain the self-supporting structure is the interaction forces between the inorganic components (such as van der Waals forces, electrostatic interactions and intertwining). Hence, there are two main categories currently being studied—carbon materials and fiber materials.
3.5.1 Carbon materials.
Graphene and carbon nanotubes are two self-supporting inorganic carbon materials. As is known, graphene has been demonstrated to exhibit the function of activating platelets, thereby promoting blood coagulation and thrombosis formation.172,173 Graphene oxide (GO), a derivative of graphene, can form self-supporting sponges through electrostatic attraction and chemical bonding.174 Furthermore, graphene oxide possesses better hydrophilicity due to the oxygen-containing groups on its surface.175 For example, Liang et al. designed a zeolite/cross-linked graphene sponge (Z-CGS) which could control the heat release of the zeolite and maintain a stable low temperature. Thus, the Z-CGS sponge showed more significant hemostatic activities under the synergistic effects of thermal stimulation, electric charge stimulation and physical absorption capacity.34 Liang also developed a graphene-kaolin composite sponge (GKCS). Notably, the GKCS sponge could absorbed plasma and promoted the coagulation process without causing any harm such as cytotoxicity and hemolysis.176 Li and co-workers fixed montmorillonite (MMT) powder into cross-linked graphene sheets under a hydrothermal reaction and prepared a graphene-MMT composite sponge (GMCS). Encouragingly, the GMCS sponge with a 3D porous structure could absorb plasma, enrich coagulation factors and activate the coagulation cascade to achieve rapid hemostasis.36
Similar to graphene, carbon nanotubes (CNTs) have also been applied in hemostasis. CNTs show great advantages in hemorrhage control due to their excellent physicochemical properties including light weight, large specific surface area, high mechanical strength and platelet activation ability. From this perspective, Zhang et al. developed an effective three-dimensional hemostatic sponge (JWCNT/HBC) by chemical modification of a joint-welded carbon nanotube (JWCNT) sponge with hydroxybutyl chitosan (HBC). Encouragingly, the JWCNT/HBC sponge exhibited high elasticity, a porous structure, suitable blood-absorption and blood-maintaining performance.177
3.5.2 Inorganic fibers.
Another type of inorganic self-supporting material is inorganic fiber. Different from traditional inorganic powders with intrinsic rigidity and brittleness, inorganic fibers possess a high aspect ratio structure, flexibility and bendability.178 As we know, a variety of inorganic fibers (such as SiO2, Al2O3, and TiO2) have been used to prepare inorganic self-supporting sponges, aerogels and membranes. Furthermore, these inorganic self-supporting materials have been applied in the fields of thermal insulation, catalysis, oil–water separation, etc. However, there are still few studies about inorganic fiber materials for rapid hemostasis reported yet. Recently, our group fabricated an inorganic hemostatic aerogel mainly based on ultralong hydroxyapatite (HAP) nanowires (80% content) with polyvinyl alcohol (PVA) as the organic binder (as shown in Fig. 5). Interestingly, this HAP aerogel exhibited super-hydrophilicity and super-hematophilicity, which were favorable for promoting hemostasis. When applied in rat liver and rabbit femoral artery bleeding models, the hemostatic time and blood loss of this HAP aerogel were significantly decreased.38 Additionally, Li et al. fabricated biomimetic ‘‘cotton-like” hollow Al2O3 fibers (HFs) to address the challenges of hemostasis. The HFs possessed a hollow porous 3D structure, large specific surface area and negative Zeta potential. Moreover, the HFs could not only concentrate blood components and activate the intrinsic pathway, but also form HF-based clots with similar morphological characteristics to fibrin-based clots.179
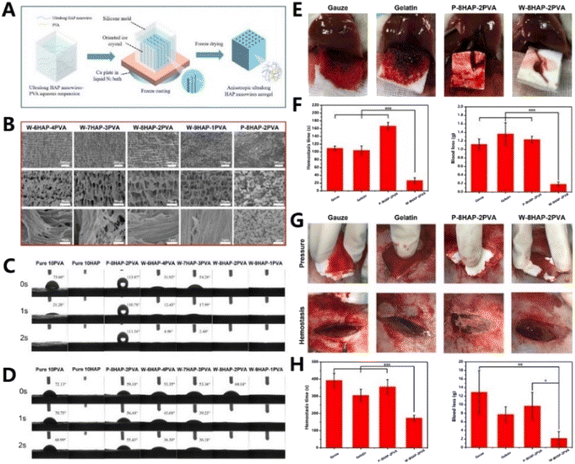 |
| Fig. 5 (A) Schematic illustration of the fabrication process of the W-HAP-PVA aerogel (composed of ultralong HAP nanowires and PVA). (B) SEM images of W-HAP-PVA aerogels with different compositions and P-8HAP-2PVA aerogel (consisting of 80 wt% HAP nanopowders and 20 wt% PVA). Comparison of the water contact angle (C) and blood contact angle (D) of W-HAP-PVA aerogels with different compositions, P-8HAP-2PVA, pure 10HAP and pure 10PVA aerogels. (E) Digital photos of hemostasis in rat liver puncture bleeding of W-8HAP-2PVA, P-8HAP-2PVA, gauze and gelatin sponge, and hemostatic capability of the materials: (F) hemostasis time and blood loss. (G) Digital photos of pressure and hemostasis in rabbit femoral artery injury bleeding of W-8HAP-2PVA, P-8HAP-2PVA, gauze and gelatin sponge. Hemostatic capability of the materials: (H) hemostasis time and blood loss. Adapted with permission from ref. 38. Copyright 2021, Elsevier B.V. | |
4. Inorganic-based biomaterials for wound healing
In the process of wound healing, non-healing chronic wound or delayed wound healing always occurs due to various intrinsic and extrinsic factors.180 Autograft or allografts is considered as the most effective treatment option for wound healing, but it still suffers from inadequacies including limited donor source, risk of immune rejection and heavy financial burden.181 Recently, inorganic-based biomaterial are found to be promising candidates for wound healing.182,183 On the one hand, many inorganic materials with a small size can cooperate with polymers, resulting in forming hydrogels with skin-like structures.184 On the other hand, inorganic materials release many functional bioactive ions, which facilitate cell proliferation, migration or differentiation.185 Furthermore, the shelf availability and affordable price of inorganic materials will promote their development and application.186 Therefore, inorganic-based biomaterials hold great promise in acute and chronic wound healing.
4.1 Different composite forms of inorganic-based biomaterials for wound healing
As we know, inorganic biomaterials mainly exist in the form of particles and fibres.187 Considering that cutaneous damage is often large and varied in shape, the way of utilizing inorganic biomaterials alone for wound healing is limited.188 In order to meet the needs of different types of wounds, it is an important direction to incorporate inorganic biomaterials into other materials. Consequently, the composite materials are suitable for various wound types, greatly enhancing the application prospects of inorganic biomaterials.189
4.1.1 Dressing.
Generally, a wound dressing can be prepared by incorporating inorganic biomaterials into natural polymers and synthetic polymers.190 Natural polymer-based wound dressings have been extensively investigated including proteins (gelatin and collagen), polysaccharides (alginates and chitosan) and proteoglycans.191 Benefiting from controllable physical and chemical properties, synthetic polymers has also been used as wound dressings like poly(vinyl alcohol) (PVA), poly(ε-caprolactone) (PCL), and poly-(lactic acid) (PLA).192 However, the insufficient mechanical strength of natural materials and the poor biocompatibility of synthetic materials limit their development as wound dressings respectively.193 Inspiringly, the addition of inorganic biomaterials addressed these problems due to their favourable effect on stiffness, viscosity and bioactive environments.194 For example, Wang et al. designed a composite hydrogel dressing by adding CaO–SiO2–TiO2 (CST5) into alginate, and therefore the gelling ability and healing microenvironment were adjusted by using calcium and silicon ions respectively.195 Therefore, inorganic biomaterial-based wound dressings are one of the most widely used forms of wound healing materials.
Apparently, inorganic biomaterial-based wound dressings are quite suitable for continuous large wound defects owing to the characteristics of a small thickness, large area and full coverage for wounds.196 Correspondingly, the types of dressings mainly include hydrogel membranes, electrospinning films, and microneedle patches. Inorganic biomaterial-based hydrogels are widely implemented in wound dressings thanks to their soft, hydrophilic and biocompatible properties.197 Wang et al. prepared hydrogel membranes consisting of a series of black bioceramics with a chitosan matrix, which enhanced skin regenerative activity in vivo.198 In contrast, inorganic biomaterial-based electrospinning films have better flexibility and mechanical properties, serving as an important complement to wound dressings. Zhang et al. reported a kind of electrospinning film with Zn-doped hollow mesoporous silica nanospheres (HMZS) and polycaprolactone (PCL) that exhibited the ability to promote angiogenesis and wound healing.199 In recent years, inorganic biomaterial-based microneedle patches, a new category of innovative materials, have been increasingly brought into focus for wound dressings. They can penetrate through the epithelial basement membrane to access subcutaneous tissues and get a tighter fit with the wound, thereby preventing falling down and accelerating the healing rate.200
4.1.2 Spray.
In addition to continuous large wound defects, discontinuous wound defects or wounds with unfavourable topography are also common types of cutaneous damage. It has attracted great interest in clinical practice in recent years. Consequently, skin spray is utilized in the treatment of such wounds, owing to the advantages such as convenience of operation, adaptability to complex shapes and controllability of dressing thickness.201
Furthermore, inorganic biomaterial-based spray with therapeutic function has been greatly developed. Recently, our group developed a sprayable β-FeSi2-incorporated sodium alginate (FS/SA) hydrogel.202 It achieved timely healing of wounds with an irregular morphology induced by tumor. With the help of Fe ions and ˙OH generated under the tumor microenvironment, photothermal and chemodynamic therapies were allied to suppress tumors. Besides, favourable migration and differentiation of endothelial cells were induced by the bioactive Fe and Si ions in spray, contributing to the angiogenesis of skin wounds and efficient healing. In another study, Ouyang et al. designed a smart black phosphorus (BP)-based gel spray for diabetic ulcer treatment.203 On the one hand, such chronic wound healing was accelerated by promoting the proliferation of endothelial cells and vascularization. On the other hand, BP in the spray could response to NIR laser irradiation to generate local heat, thereby accelerating the microcirculatory blood flow and releasing the loaded drugs.
4.1.3 Scaffold.
Compared to dressing and spray, the scaffold has a porous structure that provides a favourable space and microenvironment to promote cell growth, adhesion and migration. Therefore, more and more 3D-printed scaffolds have been developed to repair injured skin.
Siebert et al. 3D printed a hydrogel scaffold encapsulating vascular endothelial growth factor (VEGF) decorated with tetrapodal zinc oxide (t-ZnO) microparticles. The scaffold had controlled the elastic modulus and degradation behavior by adjusting t-ZnO contents, and the in vivo wound healing was enhanced with the help of the scaffold.205 Considering the therapeutic effect of bioactive ions released from inorganic biomaterials, a CaSiO3-containing hydrogel scaffold was prepared by Ma et al. Such a hydrogel scaffold promoted the proliferation and migration of human umbilical vein endothelial cells and human dermal fibroblasts in vitro and enhanced angiogenesis and wound regeneration in vivo.206 However, for the regeneration of large-scale skin defects, a large number of cells are required, which is difficult to be satisfied through autologous cell proliferation. It is worth noting that cell-laden scaffolds are an emerging solution to this problem.207 Recently, our group synthesized strontium silicate (SS) microcylinders, based on which a novel multicellular scaffold was developed.204 On the one hand, the bilayered spatial distribution of vascular endothelial cells and fibroblasts in the scaffolds offered a vascularized skin-mimicking structure. On the other hand, the SS-based inorganic scaffold acted as a cell-induced factor to induce angiogenic vitality. Importantly, the positive effect of the bioprinted scaffolds on wound healing was verified in chronic wound and acute wound respectively (Fig. 6).
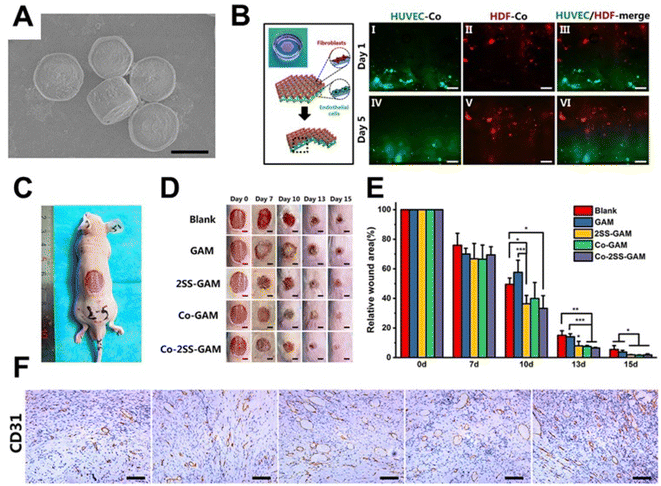 |
| Fig. 6 3D bioprinted scaffolds containing strontium silicate for wound healing. (A) SEM images of strontium silicate microparticles. (B) Schematic illustration and fluorescence images of the spatial distribution of HUVECs and HDFs in the scaffold. (C) Full-thickness skin defect model of nude mice. (D and E) Photos of wounds at different points of time and the corresponding statistics of wound closure rates. (F) Images of CD31 antibody immunohistochemical staining. Adapted with permission from ref. 204. Copyright 2021, Wiley-VCH GmbH. | |
4.2 Therapeutic effects of inorganic-based biomaterials for wound healing
As is known, the process of wound healing is divided into four stages: hemostasis, inflammation, proliferation, and remodeling.208 Each stage has its own characteristics. For instance, in the inflammation stage, neutrophils infiltrate the injury site to kill bacteria and degrade damaged matrix proteins.209 Wound healing enters the proliferative stage 2–3 days after injury, in which the endothelial cells will across the basement membrane and further grow into capillaries or microvessels.210 Subsequently, some skin appendages such as hair follicles, sweat glands and sebaceous glands can regenerate during the remodelling stage.211 As a result, the development of inorganic-based biomaterials with specific therapeutic functions has become an effective way to promote wound healing.
4.2.1 Antimicrobial properties.
Metal oxides are common inorganic biomaterials with distinctive antibacterial performance, owing to their abilities to target cell wall, membrane, and cytoplasmic contents so as to disrupt cellular homeostasis.212 Various types of metal oxide-based composites have been developed in recent studies. Razali et al. prepared a titanium dioxide nanotube (TiO2-NT)-based bionanocomposite film.213 In this study, TiO2-NTs endowed the film with concentration-dependent antimicrobial properties, and the film containing 20% TiO2-NTs (w/w%) showed the best antibacterial performance, thereby significantly accelerating the wound healing rate in animal studies. Similarly, Ahmed et al. designed a ZnO-incorporated composite nanofiber membrane, and the antimicrobial properties conferred by ZnO nanoparticles promoted the wound healing in diabetic rabbits.214 Apart from metal oxides, silicate-based composites also exhibited antimicrobial properties during wound healing. For instance, CaCuSi4O10-containing nanofibrous membranes demonstrated that the Cu component could produce ROS to kill bacteria by destroying their cell walls or membranes.215 Likewise, another study reported a kind of wound dressing based on Cu-containing bioactive glass and eggshell membranes, in which a sustained release of Cu2+ ions distinctly inhibited the viability of bacteria.216
4.2.2 Promoting angiogenesis.
In normal tissues, the vasculature delivers adequate nutrients and oxygen to cells and remove carbon dioxide and waste products. Consequently, fluid accumulation, inflammation and hypoxia occur if angiogenesis is blocked during the wound healing.217 Therefore, developing inorganic biomaterials with angiogenesis performance is an attractive way of promoting wound healing. Bao et al. designed a four-layer composite bioglass-containing dressing by electrospinning and hot pressing. The results showed that the bioactive ions released from bioglass transferred to the wound bed for the stimulation of angiogenesis.218 As reported by Wu et al., a bioscaffold with hollow manganese silicate nanospheres could enhance the expressions of angiogenic factors in endothelial cells by stimulating macrophages.190 The mechanism of inorganic-based biomaterials promoting angiogenesis has attracted more and more attention. For instance, Cu2+, Mg2+ and Sr2+ all promote vascular regeneration through activating eNOS and VEGF pathways.219–221
4.2.3 Skin appendage remolding.
Skin appendages, including hair follicles, sweat glands and sebaceous glands, play a vital role in the functions of normal tissue.222 Among them, hair follicles serve multiple functions including thermal insulation, physical protection, sensory perception, sebaceous dispersion and decorative purposes for social interactions, being the key to skin appendage remolding in wound healing.223 Excitedly, the studies of inorganic biomaterials for hair follicle regeneration have been under investigation for a long time. Zhang et al. synthesized Zn-doped hollow mesoporous silica nanospheres (HMZSs), which were incorporated into polycaprolactone (PCL) electrospun fibers to form a novel wound healing dressing. The Zn ions enhanced the recruitment and proliferation of hair follicle stem cells. Furthermore, Zhang et al. designed a biofluid-absorbing bioactive sandwich-structured Zn–Si bioceramic composite wound dressing. The results showed that the synergistic effect of the Zn2+/SiO32−combination enhanced the gene expressions of hair follicle anagen markers including PDGF-A, PDGF-B, and C-Myc in vitro, and in vivo results further indicated that the synergistic activity activated the recruitment of hair follicle stem cells and promoted the regeneration of hair follicles.224 In addition, Zhang et al. also prepared a new type of composite composed of curcumin loaded Fe–SiO2 nanoparticles, and demonstrated that the synergistic effect of SiO32−, Fe3+ and Fe-Cur chelates obviously inhibited scar hyperplasia and promoted hair follicle regeneration.225
4.3 Intelligently responsive inorganic-based biomaterials for wound healing
To date, the conventional wound dressings including bandages, hydrogels and foams have been widely explored.226 However, wound healing is a continuously changing dynamic process in which various chemical and physical changes take place. Therefore, external and internal stimuli such as light, pH, heat, and strain can influence the microenvironment of wound healing.227–229 Inorganic biomaterial-based wound dressings that can flexibly respond to these changes are one of the key therapeutants of the intelligent wound healing field.
4.3.1 Light-responsive inorganic-based biomaterials for wound healing.
Light, a particularly promising tool, holds the advantages of noninvasive nature, ease of application and spatiotemporal control. Effects derived from the reaction of inorganic biomaterials to light like photothermal therapy (PTT), photodynamic therapy (PDT), and a combination of PTT and PDT have been applied in the treatment of wound healing.227 Li et al. fabricated a NIR light-responsive hydrogel consisting of 3-(trimethoxysilyl) propyl methacrylate (MPS) and mesoporous silica (mSiO2) modified CuS nanoparticles for wound healing. The produced local heat under NIR light irradiation endowed the composite with controlled Cu2+ release behavior, leading to enhanced antibacterial efficacy and a faster wound healing rate.230 In addition to the antibacterial effect, the local heat generated by PTT plays an important role in tissue cells in wound healing as well. For example, Sheng et al. prepared a novel fayalite-based hydrogel with the photothermal effect and the heat stimulated angiogenesis through the VEGF and HSP90/eNOS signaling in endothelial cells. Subsequently, obvious enhancement of angiogenesis and chronic wound healing could be observed in vivo.231 PDT means generating (ROS) by using photosensitizers and appropriate excitation sources, thereby oxidatively damaging surrounding biomolecules and killing bacteria.232 Zhang et al. synthesized an efficient NIR-triggered antimicrobial photodynamic therapy system with lanthanide-doped upconversion nanoparticles (UCNPs, LiYF4: Yb/Er). The results demonstrated the high efficacy of the nanosystem for the inhibition of deep-tissue multi-drug resistant (MDR) bacteria in vitro and in vivo.233 However, single PTT or single PDT needs a higher temperature or abundant ROS respectively to achieve effective antibacterial properties, which may cause side effects to normal tissues.227 Therefore, the alternative strategy of employing synergistic photothermal/photodynamic therapy is practically needed for wound healing. Zhang et al. designed an effective antibacterial hydrogel embedded with Ag3PO4/MoS2 composites, which produced more ROS and more heat under dual light irradiation compared to PTT or PDT alone. As a result, the composite hydrogel exhibited excellent wound healing efficiency.
4.3.2 pH-responsive inorganic-based biomaterials for wound healing.
According to a previous study, the wound pH varies continuously during the different wound healing stages. A slightly acidic pH is beneficial to wound healing thanks to the increased fibroblast activity and limited bacterial proliferation.234 Hence, it is a good new idea for wound healing to design inorganic materials that respond to the wound pH and in turn act on the wound microenvironment. Recently, Wu et al. designed an injectable and pH-responsive nanocomposite hydrogel based on amine-modified silica nanoparticles. The results showed that the mechanical, drug release and hydrolytic degradation behaviours of this hydrogel could be adjusted in the mildly acidic range.235 Junior et al. successfully synthesized a series of poly (acid methacrylic)/LAPONITE® RDS nanocomposite hydrogels with different amounts of Lap RDS by free-radical polymerization. The variety of pH could adjust the hydrophilic properties of the nanocomposites, leading to a controlled release system in wound healing.236 However, these hydrogels have not been experimentally verified for the wound healing process. Excitedly, Guo et al. prepared a nanofibrous mat containing sodium bicarbonate by coaxial electrospinning, realizing pH-controlled dual-drug release according to the specific needs of various wound healing periods.237 It is believed that with the update of material preparation technology and the development of material properties, more and more pH-responsive inorganic materials will be applied in the treatment of cutaneous wounds.
4.3.3 Other stimuli-responsive inorganic-based biomaterials for wound healing.
In addition to the two most common stimuli mentioned above, some inorganic biomaterials that respond to other stimuli have also been developed for wound healing. Zhang et al. designed a novel wound dressing based on magnetothermally responsive microfibers composed of Fe3O4@SiO2. The smart constructs released antibiotics as needed to prevent infection. Subsequently, the controlled drug delivery significantly accelerates the healing process.238 Mao et al. developed a hydrogel composed of MXene (Ti3C2Tx) which could electrically modulate cell behaviors. Benefiting from the unique conductivity of Ti3C2Tx-MXene, the composite hydrogel actively enhanced the proliferation of NIH3T3 cells in vitro and significantly accelerated the wound healing rate in vivo under electrical stimulation.239 Mechano-responsive inorganic-based biomaterials are also a class of promising wound healing materials. Bhang et al. developed a zinc oxide nanorod-based piezoelectric patch, which generated electric potential difference-derived electrical fields (EFs) upon mechanical deformation caused by animal motion and induced piezoelectric potentials at the wound bed to facilitate wound healing. It was attributed to the promotion of cell migration, angiogenesis, and collagen synthesis under the EFs. With the concept of intelligent and refined wound management gradually recognized by the public, it is believed that stimuli-responsive inorganic materials will be gradually translated into the clinic and become the next generation of efficient wound repair tools.
In recent years, most of studies on inorganic-based biomaterials for wound healing have been focused on skin regeneration, because the skin is the body's largest organ and vulnerable to injury due to direct contact with the environment. However, there are also some typical examples for healing other soft tissues like cornea, oral mucosal or muscle with inorganic-based biomaterials. For instance, Wu et al. developed a wireless-powered electrical bandage contact lens (EBCL) by depositing a Cu film on a flexible polyimide substrate. This equipment generated an external electric field in situ to stimulate the corneal epithelial cells, thereby accelerating the corneal wound healing.240 Chen et al. prepared a porous polydroxyalkanoate (PHA) scaffold containing zinc oxide nanoparticles (ZnO NPs) for oral soft tissue regeneration. The presence of ZnO NPs resulted in the release of ROS to prevent bacterial growth. Subsequently, the rat oral soft tissue defect after infection was repaired better under the treatment of the scaffold.241 Similarly, Elshazly et al. utilized bioactive glass nanofibers (BGnf) of composition B2O3, SiO2, and CaO to enhance oral mucosal wound regeneration in diabetes mellitus.242 Interestingly, Zhang et al. designed injectable conductive microcryogels based on reduced graphene oxide (rGO) and gelatin (GT). The addition of rGO significantly improved myogenic proliferation, differentiation and in situ muscle regeneration.243 All in all, inorganic-based biomaterials have been widely developed and used for wound healing, no matter whether for skin or other soft tissues.
5. Inorganic-based biomaterials for bifunctional rapid hemostasis and wound healing
As mentioned above, wound healing is a complex multiphase physiological process that includes four overlapping phases. On the one hand, most of the inorganic-based biomaterials for hemostasis only act in the hemostasis phase, that is, to stop bleeding in the early stage of wound formation. When bleeding stops, the inorganic-based biomaterial for hemostasis is removed along with adhering blood clots and does not participate in the subsequent phases of wound healing. On the other hand, inorganic-based biomaterials for wound healing mainly act in the phases of inflammation, proliferation and remodeling. They are fixed and maintained at the wound site to provide the necessary moist environment and bioactive ions over a period of time. Hence, there is almost no connection between single-functional inorganic-based biomaterials for hemostasis and single-functional inorganic-based biomaterials for wound healing because they act in different phases of wound healing. It had led to a bottleneck in the application of inorganic-based biomaterials, because single functional biomaterials were increasingly difficult to meet the complex and diverse clinical needs. In recent years, there has been increasing evidence that hemostasis is closely related to wound healing,46,48,244–246 thereby further driving research interest in the design of inorganic-based biomaterials for both rapid hemostasis and wound healing. For instance, blood clots are the final structure formed during the hemostatic phase and were composed mainly of platelets and fibrin, and numerous studies have shown that blood clots play decisive roles in promoting wound healing.247 Furthermore, blood clots not only play the role of fixing and connecting nearby tissues, but also provide a good immune microenvironment for the invasion of inflammatory cells and the migration and proliferation of fibroblasts.248 Therefore, if blood clots formed after hemostasis are stably fixed and maintained, the wound healing process can be better promoted. Inorganic-based biomaterials for bifunctional rapid hemostasis and wound healing can be achieved through the following aspects. One is that inorganic-based biomaterials exhibit dual functions of rapid hemostasis and wound healing, and these inorganic-based biomaterials can be applied for either the rapid hemostasis stage or the wound healing stage. Another is that inorganic-based biomaterials possess the ability of maintaining clots, which means that biomaterials can absorb blood, form clots, and play a role along with blood clots in the wound healing process without being removed or peeled off. For example, inorganic-based biomaterials that not only promote blood coagulation but also exhibit functions such as antibacterial and bioactive ion release to accelerate wound healing are the former category. Meanwhile inorganic-based biomaterials that quickly absorb blood to form blood clots and gradually degrade at the wound site to provide the necessary microenvironment and active ingredients for wound healing are the latter category.
5.1 Dual functional inorganic-based biomaterials
Many studies have reported the preparation and development of inorganic-based biomaterials with dual functions of rapid hemostasis and wound healing. From this perspective, silver nanoparticles (AgNPs) and their derivatives with both hemostatic and antibacterial activities are the most commonly used active inorganic components, and they can act in the hemostasis phase and inflammation phase (reducing inflammation by suppressing bacterial infections) to achieve better therapeutic effects. For example, Wu et al. used thiol-modified chitosan and AgNPs to prepare a composite sponge (TMC/AgNPs) for stemming the bleeding and preventing infection. The TMC/AgNP sponge had a complex interlaced tubular porous structure with high porosity (99.42%). Moreover, the TMC/AgNP sponge exhibited excellent antibacterial activity against Staphylococcus aureus, Escherichia coli, and Pseudomonas aeruginosa. In vivo experiments further confirmed its efficient hemostatic and wound healing performances.249 Similarly, Lan and co-workers designed a chitosan hemostatic dressing with AgNPs (CGSH/Ag50), and found that CGSH/Ag50 could promote platelet aggregation by the activation of the positively charged surface and stimulation of AgNPs. Besides, this dressing showed high liquid absorption capacity, good hemostatic activity and antibacterial activity in vivo, thereby significantly accelerating full-thickness wound healing.86 To overcome the challenges in controlling the size and colloidal stability of AgNPs, Shakya et al. developed a template-guided synthesis of ultrafine AgNPs around 2 nm using water-soluble and biocompatible γ-cyclodextrin metal–organic frameworks (CD-MOFs). The ultrafine AgNPs could be easily dispersed in aqueous media and exhibit effective bacterial inhibition. Furthermore, the synthesized AgNPs@CD-MOFs showed a boosted hemostatic effect that further enhanced wound healing in synergy with the antibacterial effect.250 In another study, Zhang et al. reported a green strategy for in situ biomimetic syntheses of silver nanoparticles@organic frameworks/graphene oxide in a sericin/chitosan/polyvinyl alcohol hydrogel. Contributing to AgNPs, this composite dressing showed excellent lasting antibacterial properties against drug-sensitive and drug-resistant pathogenic bacteria. Interestingly, the hydrogel possessed a coagulation effect because it aggregated and acted on blood cells and platelets. In vivo evaluation further demonstrated that the composite dressing could achieve rapid hemostasis, and accelerate wound healing and re-epithelialization.251 Xu and co-workers demonstrated a multifunctional cryogel to block acute hemorrhage and promote wound healing, and this composite cryogel was composed of chitosan, silver and tannic acid (CS/Ag/TC). Due to the porous structure and positive charge of CS, the prepared cryogel exhibited good hemostatic capability with a hemostasis time less than 20 s. Besides, the CS/Ag/TC cryogel showed good antibacterial activity and effective oxidation resistance attributed to Ag and TA molecules. Under the synergetic effects of hemostasis, antibacterial activity and oxidation resistance, this cryogel could significantly promote wound repair in the skin incision model.252
In addition to AgNPs, other active inorganic components have also been investigated to fabricate composite biomaterials for both rapid hemostasis and wound healing. For instance, Ren et al. successfully prepared a kind of nanofiber dressing blended with silk fibroin, chitosan and drug-loaded halloysite nanotubes (HNTs). The addition of HNTs had a significant effect on the hemostatic activity of nanofibers and led to rapid coagulation of blood in vitro. Furthermore, this medical dressing presented stable antibacterial activity for wound healing owing to its drug release ability.254 To enhance the therapeutic effects of chitosan, Zhou and co-workers simply doped CaCO3 into acetate chitosan to form a wound dressing. Interestingly, the H+ reacted with CaCO3 to produce a mass of Ca2+ after absorbing water, resulting in activating secondary hemostasis. The released Ca2+ and residual CaCO3 further cross-linked with chitosan to form a tough and adhesive hydrogel to prevent further bleeding. Additionally, the wound dressing exhibited excellent hemostatic efficacy and accelerated wound healing through promoting re-epithelization and collagen deposition in vivo.255 In another study, Rao et al. synthesized a hemostatic and antibacterial biomaterial, namely a ZnO nanocomposite using mushroom carboxymethyl chitosan as a natural polymer stabilizing agent. As a result, the ZnO nanocomposite showed enhanced antibacterial activity toward S. aureus ascribed to the synergetic activities of mushroom carboxymethyl chitosan and ZnO. Moreover, this nanocomposite had acceptable hemostatic properties for use in wound dressing and cosmetic applications.256 Inspired by the mechanisms of blood clot formation and secondary coagulation cascade activation in the natural hemostasis process, Wang et al. prepared an injectable hydrogel sponge (QHM) consisting of hydroxyethyl cellulose and mesocellular silica foam. The QHM exhibited instant water-triggered expansion and superabsorbent capacity, thereby synergistically promoting hemostasis. Moreover, the remarkable antibacterial activity and excellent cytocompatibility of the QHM were also observed in vitro. With efficient hemostatic efficacy and excellent antibacterial behavior, the QHM dramatically facilitated the wound healing in a full-thickness skin defect model.53
5.2 Clot maintaining inorganic-based biomaterials
Considering the key role of blood clots in the wound healing process, utilizing inorganic-based biomaterials for fixing blood clots and acting in the wound site still remains a challenge. From this perspective, it requires inorganic-based biomaterials to possess good tissue adhesion, excellent biocompatibility and hemostatic activity, so as to stably fix blood clots. Furthermore, inorganic-based biomaterials should slowly degrade and release bioactive ions at the wound site to promote wound healing. For example, Nie et al. prepared silver nanoparticle-incorporated mesoporous silica granules (AgNP-MSG) for hemostasis and repair of internal organs. In vitro experiments demonstrated that the as-prepared composite showed high absorption capacity, hemostasis efficacy, good biocompatibility and sustained antibacterial activity. Besides, AgNP-MSG was applied in a rat liver injury model for hemorrhage control and organ healing. Encouragingly, bleeding was effectively controlled within 7 s and AgNP-MSG was retained with blood clots in the injured liver. After two weeks, the rat survived and the liver was almost fully repaired with no trace of AgNP-MSG being found.257 In another study, Li et al. developed biphasic Janus self-propelled hemostatic particles loaded with thrombin (MSS@CaCO3T). Notably, these Janus particles were implanted in a rabbit back subcutaneous muscle injury model after bleeding. Contributing to the release of thrombin and Ca2+, MSS@CaCO3T could significantly accelerate blood clotting in vivo for activating the coagulation cascade. After the implantation of Janus MSS@CaCO3T for 14 days, histological analysis further confirmed that MSS@CaCO3T particles could gradually degrade and promote tissue repair. Hence, MSS@CaCO3T particles with excellent hemostatic efficacy and biodegradation displayed great potential in the therapy of complex traumas (Fig. 7).253 To achieve rapid, strong hemostasis as well as closure and healing in deep wounds, Meddahi-Pelle and co-workers prepared aqueous solutions of Stober silica or iron oxide nanoparticles that exhibited nanobridging function. It was shown that aqueous solutions of nanoparticles could stop bleeding within a minute in a rat liver organ injury and promote healing of the organ injury due to its instant hemostasis, tight closure and rapid degradation.258 Currently, Liu et al. developed a portable electrospinning device (150 g in weight) for outdoor hemostasis and further utilized this device to green-synthesize CuS composite nanofibers. Interestingly, the CuS composite nanofibers could be deposited in situ and possessed better compactness onto the rough wound surface than conventional nanofiber mats. Furthermore, they are favorable to simultaneously achieve rapid hemostasis outdoors and ablate superbacteria, resulting in promoting wound healing. Thus, the CuS composite nanofibers not only accelerate the blood coagulation (<6 s) but also shorten the healing time of the wound with superbacterial infection (18 days).29 Although many inorganic-based biomaterials for bifunctional rapid hemostasis and wound healing have been successfully prepared, some current bottlenecks and problems need to be addressed. Firstly, components and forms of inorganic-based biomaterials for bifunctional rapid hemostasis and wound healing are mostly powders, represented by AgNPs. However, powders for wounds could lead to a sharp release of ions and a large change in pH, which were negative for tissue healing. Moreover, residual particles in the body might increase the risk of thromboembolism. Combining active inorganic components with polymers is a development trend to address this problem. Secondly, most inorganic-based biomaterials for bifunctional rapid hemostasis and wound healing only integrate hemostatic and antimicrobial effects. Nevertheless, the process of wound healing also needs the avoidance of excessive inflammatory response, a suitable immune microenvironment and a large number of extra tissue cells. It means that inorganic-based biomaterials are required to combine hemostasis with more functions including anti-inflammation, immunoregulation, promotion of cell proliferation and differentiation, and tissue remolding. It is still a challenge to connect different phases of wound healing with inorganic-based biomaterials and have a cascade effect to achieve better therapeutic outcomes. Hence, it is important to develop more inorganic-based biomaterials with multifunctional properties, which requires scientists to further carry out more innovations and attempts.
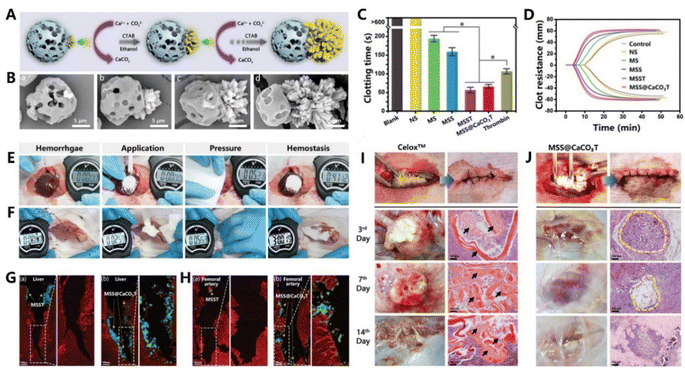 |
| Fig. 7 (A) Schematic showing the growth of flower-like CaCO3 crystals on negative-potential-charged microporous starch (MSS) during the synthesis of Janus MSS@CaCO3 particles. (B) SEM images of MSS@CaCO3 Janus particles with different aspect ratios of [MSS]/[CaCO3]: (a) 1 : 0.1, (b) 1 : 0.5, (c) 1 : 1, and (d) 1 : 2. (C) Whole blood in vitro clotting time. (D) Representative TEG parameters of whole blood treated with particles. Images of hemostasis in liver (E) and femoral artery (F) bleeding models. Histological sections from (G) liver bleeding models and (H) femoral artery bleeding models treated with (a) MSST and (b) Janus MSS@CaCO3T. Histological sections from in vivo biodegradation models treated with Celox (I) and Janus MSS@CaCO3T (J). Adapted with permission from ref. 253. Copyright 2020, Wiley-VCH GmbH. | |
As discussed above, there are various inorganic-based biomaterials with different biofunctions that have been extensively developed. In order to facilitate the reading and understanding for readers, we have sorted out and summarized the above contents. Table 1 lists some representative types of inorganic-based biomaterials that can be applied to rapid hemostasis and wound healing.
Table 1 Inorganic-based biomaterials for rapid hemostasis and wound healing
Application field |
Material type |
Active inorganic component |
Biofunctions |
Ref. |
Rapid hemostasis |
Natural mineral clays |
Zeolite, kaolin, montmorillonite |
Blood absorption, Ca2+ release, negative surface charge, mechanical strength enhancement, tissue adhesive |
121 and 128 |
Silica-based mesoporous particles |
AgCaMSS, CSMS-S, Ga2O3-MBGs, Ta-MBGs, diatom silica |
Blood absorption, high surface aera and pore volume, Ca2+ release, negative surface charge |
135, 138, 143 and 150 |
Inorganic–polymer composites |
Mesoporous single-crystal zeolite, NEM, HNTs, rectorite |
Blood absorption, concentrating coagulation factors, negative surface charge, tissue adhesive, physical barrier |
40, 155, 159 and 165 |
Self-supporting inorganic-based biomaterials |
Graphene, GO, CNTs, ultralong HAP nanowires, hollow Al2O3 fibers |
Blood absorption, super hydrophilicity and hemophilicity, high surface aera, activation of platelets |
36, 38, 150–153, 176 and 178 |
Wound healing |
Basic dressings |
CST5, FS, BP, t-ZnO, CaSiO3, SS |
Bioactive ion release, angiogenesis, PTT, CDT, skin defects regeneration |
194, 201, 203 and 205 |
Therapeutic functional inorganic-based biomaterials |
TiO2-NTs, CaCuSi4O10, HMZS, hollow manganese silicate nanospheres |
Bioactive ion release, antibacterial activity, angiogenesis, skin appendage remolding, hair follicle regeneration |
38, 179, 189, 212 and 215 |
Intelligently responsive inorganic-based biomaterials |
Ag3PO4/MoS2, LAPONITE® RDS, Ti3C2Tx, Fe3O4@SiO2, MoS2 nanosheet |
Antibacterial activity, angiogenesis, pH-responsive, PTT, PDT, controlled drug delivery, electrical stimulation |
232–236
|
Rapid hemostasis and wound healing |
Dual functional inorganic-based biomaterials |
AgNPs, HNTs, CaCO3, ZnO mesocellular silica foam |
Blood absorption, negative surface charge, hemostasis drug release, antibacterial activity, reduce inflammation, promote re-epithelization and collagen deposition |
53, 243, 245 and 246 |
Clot maintaining inorganic-based biomaterials |
AgNP-MSG, MSS@CaCO3T, CuS, Stober silica and iron oxide |
Blood absorption, negative surface charge, tissue adhesive, clot maintaining, antibacterial activity, degradability, bioactive ion release, biocompatibility |
29, 248–250 |
6. Conclusions and prospects
Inorganic-based biomaterials for rapid hemostasis and wound healing are essential in wound treatment, especially in the cases of severe bleeding when the inherent hemostatic system fails to stop the bleeding. In this article, we systematically review inorganic-based biomaterials for hemostasis and wound healing from different perspectives. Components and material forms are two key factors to determine the properties and functions of inorganic-based biomaterials. The components with hemostasis and wound healing functions have been well studied over the past 20 years, leading to the identification of new active inorganic components. Meanwhile, researchers provide deep insight into their mechanisms of stopping bleeding and promoting healing. The forms of materials also affect hemostasis and healing processes, and various forms of inorganic-based biomaterials have been developed. However, each form of inorganic-based biomaterials has its own limitations, which require additional investigations to identify innovation solutions. Hence, it is an important issue to discuss the challenges and prospects in the material design, functions and applications of inorganic-based biomaterials for rapid hemostasis and wound healing.
Material design determines the composition and structure of biomaterials. However, most of current design routes to inorganic-based biomaterials are complicated and time-consuming. The principles of green chemistry and the cost of the ingredients should also be considered before developing a new synthetic method. It is worth noting that inorganic-based biomaterials prepared in recent years mostly lack pertinence. This means that researchers need to pay more attention to the intrinsic mechanisms of hemostasis and wound healing, thereby designing targeted biomaterials. For instance, a potential research direction is to design inorganic-based biomaterials that participate in or promote physiological hemostasis and wound healing processes, and this strategy has not yet been widely targeted in existing studies. The design routes in current studies mainly depend on the final performances of inorganic-based biomaterials, while ignoring how the biomaterials participate in physiological hemostasis and wound healing processes. Hence, designing inorganic-based biomaterials to mimic native components and processes of hemostasis and wound healing is an appealing future trend.259,260 For example, the design of smart biomaterials such as platelet-like particles and fibrin-structured nanofibers could provide great exciting opportunities in the development of inorganic-based biomaterials. Although numerous types of inorganic-based biomaterials for rapid hemostasis and wound healing have been developed, only a few of them have reached the clinical trial stage of development. Nowadays, only several inorganic-based biomaterials containing natural mineral clays261 or silica-based mesoporous particles262 have been applied in clinical application, because they have easy-to-use material forms and active components approved by regulatory authorities. In general, inorganic-based biomaterials comprising simple chemicals, components and material forms are more likely to have access to clinical trials.
Although inorganic-based biomaterials with a single function have been widely studied, they are unable to meet the increasing clinical needs. Multifunctional inorganic-based biomaterials that integrate hemostatic ability with one or more other characteristics or functions are particularly desirable for clinic applications. As discussed above, inorganic-based biomaterials with multiple functions including hemostasis, antibacterial, immunoregulation, angiogenesis, etc. show great potential for wound treatment. Such situations have attracted more attention and more novel inorganic-based biomaterials are expected to be fabricated for better clinical therapeutic effects. The wound healing process is complicated and most of the inorganic-based therapeutic biomaterials only focus on skin repair. Unfortunately, the biomaterials that can promote the repair of internal organs are very rare although they belong to an important area that requires increased research attention.263 Besides, inorganic-based biomaterials with diagnostic and monitoring abilities have advantages over traditional materials. The pH, temperature and electrical properties of a wound site always change during hemostasis and wound healing processes. Therefore, it is highly promising to develop materials with the ability of monitoring these physiochemical changes at the wound sites so as to determine wound's conditions without peeling off the dressing. It also enables doctors and patients to better track the wound healing process. Another challenge is to design inorganic-based biomaterials that are easy to remove without peeling off blood clots and causing secondary bleeding or biomaterials that can be maintained with blood clots in the wound and gradually degrade. Hence, the biocompatibility of degradation biomaterials and the risk of an in vivo thrombo-inflammatory response should be taken into consideration. Determining how to control the degradation of inorganic-based biomaterials is another important issue, as the degradation rate should match the tissue repair process and the degradation products should be absorbed by around histiocytes.
The ultimate goal of biomaterials is to be applied in clinical practice for reliable therapeutic effects. It means that inorganic-based biomaterials for rapid hemostasis and wound healing should not only exhibit good therapeutic function, but also show easy operation in practical applications. However, most research in the lab rarely focuses on the application of biomaterials, which is not conducive to their clinical trials and industrialization. There are various practical properties including long-term stability and portability that need to be considered in developing inorganic-based biomaterials for rapid hemostasis and wound healing. For example, portable inorganic-based biomaterials that can be stored for a long time even under extreme conditions are particularly important for military and first-aid applications. The cost of inorganic-based biomaterials is also a key indicator, determining whether they can be mass-produced and developed for civilian use. Furthermore, many inorganic-based biomaterials for rapid hemostasis and wound healing are applied externally, so their aesthetics should be carefully considered.
In summary, further studies on inorganic-based biomaterials for rapid hemostasis and wound healing need to focus on more than effective hemorrhage control and wound healing. Numerous studies reported various inorganic-based biomaterials with different components, forms, properties or functions, and they showed excellent therapeutic activities in rapid hemostasis and wound healing. Nevertheless, there are still some obvious deficiencies, such as complicated synthesis and fabrication processes (time-consuming and costly) and a narrow application field (mostly in skin wounds). It is believed that the advancement of chemistry design and fabrication technology will greatly promote the development of inorganic-based biomaterials with hemostasis and wound healing properties, thereby accelerating their clinical application in the near future.
Author contributions
C. Wu and Y. Zhu supervised the project and reviewed and revised the manuscript. Y. Zheng and J. Wu co-wrote the manuscript.
Conflicts of interest
There are no conflicts to declare.
Acknowledgements
This work was supported by the Natural Science Foundation of China (32130062 and 32225028), Technology Commission of Shanghai Municipality (21DZ1205600), CAS Project for Young Scientists in Basic Research Grant (No. YSRB073) and Shanghai Pilot Program for Basic Research – Chinese Academy of Science, Shanghai Branch (JCYJ-SHFY-2022-003).
References
- Y. Hong, F. Zhou, Y. Hua, X. Zhang, C. Ni, D. Pan, Y. Zhang, D. Jiang, L. Yang, Q. Lin, Y. Zou, D. Yu, D. E. Arnot, X. Zou, L. Zhu, S. Zhang and H. Ouyang, Nat. Commun., 2019, 10, 2060 CrossRef PubMed.
- Z. Li, A. Milionis, Y. Zheng, M. Yee, L. Codispoti, F. Tan, D. Poulikakos and C. H. Yap, Nat. Commun., 2019, 10, 5562 CrossRef CAS.
- S. Y. Ong, J. Wu, S. M. Moochhala, M. H. Tan and J. Lu, Biomaterials, 2008, 29, 4323–4332 CrossRef CAS PubMed.
- A. M. Behrens, M. J. Sikorski and P. Kofinas, J. Biomed. Mater. Res., Part A, 2014, 102, 4182–4194 CrossRef PubMed.
- J. Zia, J. Kimball, C. Rolfes, J. O. Hahn and O. T. Inan, Sci. Adv., 2020, 6, 11 Search PubMed.
- B. Guo, R. Dong, Y. Liang and M. Li, Nat. Rev. Chem., 2021, 5, 773–791 CrossRef CAS.
- B. Dalisson and J. Barralet, Adv. Healthcare Mater., 2019, 8, e1900764 CrossRef.
- S. Hou, Y. Liu, F. Feng, J. Zhou, X. Feng and Y. Fan, Adv. Healthcare Mater., 2020, 9, e1901041 CrossRef PubMed.
- W. Han, B. Zhou, K. Yang, X. Xiong, S. Luan, Y. Wang, Z. Xu, P. Lei, Z. Luo, J. Gao, Y. Zhan, G. Chen, L. Liang, R. Wang, S. Li and H. Xu, Bioact. Mater., 2020, 5, 768–778 CrossRef PubMed.
- J. Qu, X. Zhao, Y. Liang, T. Zhang, P. X. Ma and B. Guo, Biomaterials, 2018, 183, 185–199 CrossRef CAS PubMed.
- C. Liu, C. Liu, S. Yu, N. Wang, W. Yao, X. Liu, G. Sun, Q. Song and W. Qiao, Int. J. Biol. Macromol., 2020, 160, 1130–1143 CrossRef CAS.
- G. Hu, B. Song, A. Jiang, B. Chu, X. Shen, J. Tang, Y. Su and Y. He, Small, 2019, 15, e1803200 CrossRef PubMed.
- Y. Liang, X. Zhao, T. Hu, B. Chen, Z. Yin, P. X. Ma and B. Guo, Small, 2019, 15, e1900046 CrossRef PubMed.
- E. E. Leonhardt, N. Kang, M. A. Hamad, K. L. Wooley and M. Elsabahy, Nat. Commun., 2019, 10, 2307 CrossRef PubMed.
- A. L. Harkins, S. Duri, L. C. Kloth and C. D. Tran, J. Biomed. Mater. Res., Part B, 2014, 102, 1199–1206 CrossRef PubMed.
- Z. Zhai, K. Xu, L. Mei, C. Wu, J. Liu, Z. Liu, L. Wan and W. Zhong, Soft Matter, 2019, 15, 8603–8610 RSC.
- G. Lan, B. Lu, T. Wang, L. Wang, J. Chen, K. Yu, J. Liu, F. Dai and D. Wu, Colloids Surf., B, 2015, 136, 1026–1034 CrossRef CAS PubMed.
- Y. F. Zhao, J. Y. Zhao, W. Z. Hu, K. Ma, Y. Chao, P. J. Sun, X. B. Fu and H. Zhang, J. Mater. Chem. B, 2019, 7, 1855–1866 RSC.
- N. Annabi, D. Rana, E. Shirzaei Sani, R. Portillo-Lara, J. L. Gifford, M. M. Fares, S. M. Mithieux and A. S. Weiss, Biomaterials, 2017, 139, 229–243 CrossRef CAS PubMed.
- L. Bacakova, M. Vandrovcova, I. Kopova and I. Jirka, Biomater. Sci., 2018, 6, 974–989 RSC.
- F. Lv, J. Wang, P. Xu, Y. Han, H. Ma, H. Xu, S. Chen, J. Chang, Q. Ke, M. Liu, Z. Yi and C. Wu, Acta Biomater., 2017, 60, 128–143 CrossRef CAS PubMed.
- N. Ribeiro, A. Sousa, C. Cunha-Reis, A. L. Oliveira, P. L. Granja, F. J. Monteiro and S. R. Sousa, Nanomedicine, 2021, 33, 102353 CrossRef CAS PubMed.
- L. Chen, C. Deng, J. Li, Q. Yao, J. Chang, L. Wang and C. Wu, Biomaterials, 2019, 196, 138–150 CrossRef CAS PubMed.
- M. Nowicki, W. Zhu, K. Sarkar, R. Rao and L. G. Zhang, Bioprinting, 2020, 17, e00066 CrossRef.
- X. Zhou, T. Esworthy, S. J. Lee, S. Miao, H. Cui, M. Plesiniak, H. Fenniri, T. Webster, R. D. Rao and L. G. Zhang, Nanomedicine, 2019, 19, 58–70 CrossRef CAS PubMed.
- H. Maleki, M. A. Shahbazi, S. Montes, S. H. Hosseini, M. R. Eskandari, S. Zaunschirm, T. Verwanger, S. Mathur, B. Milow, B. Krammer and N. Husing, ACS Appl. Mater. Interfaces, 2019, 11, 17256–17269 CrossRef CAS PubMed.
- N. Zhao, M. Yang, Q. Zhao, W. Gao, T. Xie and H. Bai, ACS Nano, 2017, 11, 4777–4784 CrossRef CAS PubMed.
- J. Zhu, R. Xiong, F. Zhao, T. Peng, J. Hu, L. Xie, H. Xie, K. Wang and C. Jiang, ACS Sustainable Chem. Eng., 2019, 8, 71–83 CrossRef.
- X.-F. Liu, J. Zhang, J.-J. Liu, Q.-H. Zhou, Z. Liu, P.-Y. Hu, Z. Yuan, S. Ramakrishna, D.-P. Yang and Y.-Z. Long, Chem. Eng. J., 2020, 401, 126096 CrossRef CAS.
- R. Ahmed, M. Tariq, I. Ali, R. Asghar, P. Noorunnisa Khanam, R. Augustine and A. Hasan, Int. J. Biol. Macromol., 2018, 120, 385–393 CrossRef CAS PubMed.
- W. T. Cao, F. F. Chen, Y. J. Zhu, Y. G. Zhang, Y. Y. Jiang, M. G. Ma and F. Chen, ACS Nano, 2018, 12, 4583–4593 CrossRef CAS PubMed.
- L.-Y. Dong and Y.-J. Zhu, ACS Sustainable Chem. Eng., 2018, 6, 17239–17251 CrossRef CAS.
- S. Pourshahrestani, E. Zeimaran, I. Djordjevic, N. A. Kadri and M. R. Towler, Mater. Sci. Eng., C, 2016, 58, 1255–1268 CrossRef CAS PubMed.
- Y. Liang, C. Xu, F. Liu, S. Du, G. Li and X. Wang, ACS Appl. Mater. Interfaces, 2019, 11, 23848–23857 CrossRef CAS PubMed.
- S. Pourshahrestani, N. A. Kadri, E. Zeimaran and M. R. Towler, Biomater. Sci., 2018, 7, 31–50 RSC.
- G. Li, K. Quan, Y. Liang, T. Li, Q. Yuan, L. Tao, Q. Xie and X. Wang, ACS Appl. Mater. Interfaces, 2016, 8, 35071–35080 CrossRef CAS.
- Q. Jiang, B. Luo, Z. Wu, B. Gu, C. Xu, X. Li and X. Wang, Chem. Eng. J., 2021, 421, 129815 CrossRef CAS.
- Y. Zheng, W. Ma, Z. Yang, H. Zhang, J. Ma, T. Li, H. Niu, Y. Zhou, Q. Yao, J. Chang, Y. Zhu and C. Wu, Chem. Eng. J., 2022, 430, 132912 CrossRef CAS.
- C. Cui, T. Wu, F. Gao, C. Fan, Z. Xu, H. Wang, B. Liu and W. Liu, Adv. Funct. Mater., 2018, 28, 1804925 CrossRef.
- Y. Cui, Z. Huang, L. Lei, Q. Li, J. Jiang, Q. Zeng, A. Tang, H. Yang and Y. Zhang, Nat. Commun., 2021, 12, 5922 CrossRef CAS PubMed.
- I. V. Fadeeva, E. S. Trofimchuk, A. A. Forysenkova, A. I. Ahmed, O. I. Gnezdilov, G. A. Davydova, S. G. Kozlova, A. Antoniac and J. V. Rau, Polymers, 2021, 13, 3989 CrossRef CAS PubMed.
- L. Zhou, X. Zhao, M. Li, L. Yan, Y. Lu, C. Jiang, Y. Liu, Z. Pan and J. Shi, Int. J. Biol. Macromol., 2021, 181, 1183–1195 CrossRef CAS PubMed.
- F. Mariani, M. Serafini, I. Gualandi, D. Arcangeli, F. Decataldo, L. Possanzini, M. Tessarolo, D. Tonelli, B. Fraboni and E. Scavetta, ACS Sens., 2021, 6, 2366–2377 CrossRef CAS PubMed.
- Z. Tu, M. Chen, M. Wang, Z. Shao, X. Jiang, K. Wang, Z. Yao, S. Yang, X. Zhang, W. Gao, C. Lin, B. Lei and C. Mao, Adv. Funct. Mater., 2021, 31, 2100924 CrossRef CAS.
- S. Y. Cho and M. Hur, Ann. Lab. Med., 2019, 39, 343–344 CrossRef PubMed.
- C. L. Baum and C. J. Arpey, Dermatol. Surg., 2005, 31, 674–686 CrossRef CAS.
- L. Xiao, Y. Ma, R. Crawford, J. Mendhi, Y. Zhang, H. Lu, Q. Zhao, J. Cao, C. Wu, X. Wang and Y. Xiao, Mater. Today, 2022, 54, 202–224 CrossRef CAS.
- N. Laurens, P. Koolwijk and M. P. M. De Maat, J. Thromb. Haemostasis, 2006, 4, 932–939 CrossRef CAS PubMed.
- K. Sasaki, M. Tenjimbayashi, K. Manabe and S. Shiratori, ACS Appl. Mater. Interfaces, 2016, 8, 651–659 CrossRef CAS.
- G. Sandri, C. Aguzzi, S. Rossi, M. C. Bonferoni, G. Bruni, C. Boselli, A. I. Cornaglia, F. Riva, C. Viseras, C. Caramella and F. Ferrari, Acta Biomater., 2017, 57, 216–224 CrossRef CAS PubMed.
- C. A. Vaiana, M. K. Leonard, L. F. Drummy, K. M. Singh, A. Bubulya, R. A. Vaia, R. R. Naik and M. P. Kadakia, Biomacromolecules, 2011, 12, 3139–3146 CrossRef CAS.
- O. Ziv-Polat, M. Topaz, T. Brosh and S. Margel, Biomaterials, 2010, 31, 741–747 CrossRef CAS PubMed.
- C. Wang, H. Niu, X. Ma, H. Hong, Y. Yuan and C. Liu, ACS Appl. Mater. Interfaces, 2019, 11, 34595–34608 CrossRef CAS PubMed.
- L. Zhang, B. Casey, D. K. Galanakis, C. Marmorat, S. Skoog, K. Vorvolakos, M. Simon and M. H. Rafailovich, Acta Biomater., 2017, 54, 164–174 CrossRef CAS PubMed.
- X. Ma, Y. Cheng, H. Jian, Y. Feng, Y. Chang, R. Zheng, X. Wu, L. Wang, X. Li and H. Zhang, Adv. Healthcare Mater., 2019, 8, e1900256 CrossRef.
- D. Y. Zhao, J. L. Feng, Q. S. Huo, N. Melosh, G. H. Fredrickson, B. F. Chmelka and G. D. Stucky, Science, 1998, 279, 548–552 CrossRef CAS PubMed.
- F. Chen and Y.-J. Zhu, ACS Nano, 2016, 10, 11483–11495 CrossRef CAS PubMed.
- C. X. Li, A. K. Born, T. Schweizer, M. Zenobi-Wong, M. Cerruti and R. Mezzenga, Adv. Mater., 2014, 26, 3207–3212 CrossRef CAS PubMed.
- C. Wu, J. Chang, S. Ni and J. Wang, J. Biomed. Mater. Res., Part A, 2006, 76, 73–80 CrossRef PubMed.
- E. A. Guzzi and M. W. Tibbitt, Adv. Mater., 2020, 32, e1901994 CrossRef PubMed.
- A. J. Boydston, B. Cao, A. Nelson, R. J. Ono, A. Saha, J. J. Schwartz and C. J. Thrasher, J. Mater. Chem. A, 2018, 6, 20621–20645 RSC.
- N. Paxton, W. Smolan, T. Bock, F. Melchels, J. Groll and T. Jungst, Biofabrication, 2017, 9, 044107 CrossRef PubMed.
- P. T. Smith, A. Basu, A. Saha and A. Nelson, Polymer, 2018, 152, 42–50 CrossRef CAS.
- Y. Hu, B. Wu, Y. Xiong, R. Tao, A. C. Panayi, L. Chen, W. Tian, H. Xue, L. Shi, X. Zhang, L. Xiong, B. Mi and G. Liu, Chem. Eng. J., 2021, 426, 130634 CrossRef CAS.
- C. Feng, W. Zhang, C. Deng, G. Li, J. Chang, Z. Zhang, X. Jiang and C. Wu, Adv. Sci., 2017, 4, 1700401 CrossRef PubMed.
- H. Ma, C. Jiang, D. Zhai, Y. Luo, Y. Chen, F. Lv, Z. Yi, Y. Deng, J. Wang, J. Chang and C. Wu, Adv. Funct. Mater., 2016, 26, 1197–1208 CrossRef CAS.
- W. Zhang, C. Feng, G. Yang, G. Li, X. Ding, S. Wang, Y. Dou, Z. Zhang, J. Chang, C. Wu and X. Jiang, Biomaterials, 2017, 135, 85–95 CrossRef CAS PubMed.
- Y. Zhang, L. Xia, D. Zhai, M. Shi, Y. Luo, C. Feng, B. Fang, J. Yin, J. Chang and C. Wu, Nanoscale, 2015, 7, 19207–19221 RSC.
- T. Li, H. Ma, H. Ma, Z. Ma, L. Qiang, Z. Yang, X. Yang, X. Zhou, K. Dai and J. Wang, ACS Appl. Mater. Interfaces, 2019, 11, 17134–17146 CrossRef CAS.
- J. W. Stansbury and M. J. Idacavage, Dent. Mater., 2016, 32, 54–64 CrossRef CAS PubMed.
- S. C. Ligon, R. Liska, J. Stampfl, M. Gurr and R. Mulhaupt, Chem. Rev., 2017, 117, 10212–10290 CrossRef CAS PubMed.
- Y. Sakurai, E. T. Hardy, B. Ahn, R. Tran, M. E. Fay, J. C. Ciciliano, R. G. Mannino, D. R. Myers, Y. Qiu, M. A. Carden, W. H. Baldwin, S. L. Meeks, G. E. Gilbert, S. M. Jobe and W. A. Lam, Nat. Commun., 2018, 9, 509 CrossRef PubMed.
- S. H. Kim, H. Hong, O. Ajiteru, M. T. Sultan, Y. J. Lee, J. S. Lee, O. J. Lee, H. Lee, H. S. Park, K. Y. Choi, J. S. Lee, H. W. Ju, I. S. Hong and C. H. Park, Nat. Protoc., 2021, 16, 5484–5532 CrossRef CAS.
- L. G. Bracaglia, M. Messina, S. Winston, C. Y. Kuo, M. Lerman and J. P. Fisher, Biomacromolecules, 2017, 18, 3802–3811 CrossRef CAS.
- P. He, J. Zhao, J. Zhang, B. Li, Z. Gou, M. Gou and X. Li, Burns Trauma, 2018, 6, 5 Search PubMed.
- S. S. Biranje, J. Sun, L. Cheng, Y. Cheng, Y. Shi, S. Yu, H. Jiao, M. Zhang, X. Lu, W. Han, Q. Wang, Z. Zhang and J. Liu, ACS Appl. Mater. Interfaces, 2022, 14, 3792–3808 CrossRef CAS PubMed.
- P. R. Turner, E. Murray, C. J. McAdam, M. A. McConnell and J. D. Cabral, ACS Appl. Mater. Interfaces, 2020, 12, 32328–32339 CrossRef CAS.
- C. Qin, J. Ma, L. Chen, H. Ma, H. Zhuang, M. Zhang, Z. Huan, J. Chang, N. Ma and C. Wu, Mater. Today, 2021, 49, 68–84 CrossRef CAS.
- G. Shao, D. A. H. Hanaor, X. Shen and A. Gurlo, Adv. Mater., 2020, 32, e1907176 CrossRef PubMed.
- U. G. Wegst, H. Bai, E. Saiz, A. P. Tomsia and R. O. Ritchie, Nat. Mater., 2015, 14, 23–36 CrossRef CAS PubMed.
- H. Zhang and A. I. Cooper, Adv. Mater., 2007, 19, 1529–1533 CrossRef CAS.
- S. Deville, Scr. Mater., 2018, 147, 119–124 CrossRef CAS.
- H. Yuan, L. Chen and F. F. Hong, ACS Appl. Mater. Interfaces, 2020, 12, 3382–3392 CrossRef CAS PubMed.
- Y. Huang, X. Zhao, Z. Zhang, Y. Liang, Z. Yin, B. Chen, L. Bai, Y. Han and B. Guo, Chem. Mater., 2020, 32, 6595–6610 CrossRef CAS.
- M. Li, Z. Zhang, Y. Liang, J. He and B. Guo, ACS Appl. Mater. Interfaces, 2020, 12, 35856–35872 CrossRef CAS PubMed.
- G. Lan, Q. Li, F. Lu, K. Yu, B. Lu, R. Bao and F. Dai, Cellulose, 2019, 27, 385–400 CrossRef.
- H. Bai, F. Walsh, B. Gludovatz, B. Delattre, C. Huang, Y. Chen, A. P. Tomsia and R. O. Ritchie, Adv. Mater., 2016, 28, 50–56 CrossRef CAS PubMed.
- G. Du, A. Mao, J. Yu, J. Hou, N. Zhao, J. Han, Q. Zhao, W. Gao, T. Xie and H. Bai, Nat. Commun., 2019, 10, 800 CrossRef PubMed.
- J. K. Han, G. L. Du, W. W. Gao and H. Bai, Adv. Funct. Mater., 2019, 29, 9 Search PubMed.
- H. Bai, Y. Chen, B. Delattre, A. P. Tomsia and R. O. Ritchie, Sci. Adv., 2015, 1, 8 Search PubMed.
- T. Li, D. Zhai, B. Ma, J. Xue, P. Zhao, J. Chang, M. Gelinsky and C. Wu, Adv. Sci., 2019, 6, 1901146 CrossRef CAS PubMed.
- T. Li, B. Ma, J. Xue, D. Zhai, P. Zhao, J. Chang and C. Wu, Adv. Healthcare Mater., 2020, 9, e1901211 CrossRef.
- Y. Feng, H. L. Gao, D. Wu, Y. T. Weng, Z. Y. Wang, S. H. Yu and Z. Wang, Adv. Funct. Mater., 2021, 31, 2105348 CrossRef CAS.
- T. Jiang, E. J. Carbone, K. W. H. Lo and C. T. Laurencin, Prog. Polym. Sci., 2015, 46, 1–24 CrossRef CAS.
- R. Zamani, S. F. Aval, Y. Pilehvar-Soltanahmadi, K. Nejati-Koshki and N. Zarghami, Drug Res., 2018, 68, 425–435 CrossRef CAS PubMed.
- Q. Yu, Y. Han, T. Tian, Q. Zhou, Z. Yi, J. Chang and C. Wu, Biomaterials, 2019, 194, 25–35 CrossRef CAS PubMed.
- D. Li, W. Nie, L. Chen, Y. Miao, X. Zhang, F. Chen, B. Yu, R. Ao, B. Yu and C. He, RSC Adv., 2017, 7, 7973–7982 RSC.
- M. Long, Q. Liu, D. Wang, J. Wang, Y. Zhang, A. Tang, N. Liu, B. Bui, W. Chen and H. Yang, Mater. Today Adv., 2021, 12, 100190 CrossRef CAS.
- Q. Chen, J. Wu, Y. Liu, Y. Li, C. Zhang, W. Qi, K. W. K. Yeung, T. M. Wong, X. Zhao and H. Pan, Mater. Sci. Eng., C, 2019, 105, 110083 CrossRef CAS PubMed.
- S. B. Balakrishnan, S. Kuppu and S. Thambusamy, J. Mol. Struct., 2021, 1246, 131195 CrossRef CAS.
- T. W. Sun, Y. J. Zhu and F. Chen, Chemistry, 2017, 23, 3850–3862 CrossRef CAS PubMed.
- M. Mansoorianfar, K. Shahin, M. M. Mirström and D. Li, Ceram. Int., 2021, 47, 416–423 CrossRef CAS.
- J. Xue, X. Wang, E. Wang, T. Li, J. Chang and C. Wu, Acta Biomater., 2019, 100, 270–279 CrossRef CAS PubMed.
- S.-M. Chen, H.-L. Gao, X.-H. Sun, Z.-Y. Ma, T. Ma, J. Xia, Y.-B. Zhu, R. Zhao, H.-B. Yao, H.-A. Wu and S.-H. Yu, Matter, 2019, 1, 412–427 CrossRef.
- H.-L. Gao, S.-M. Chen, L.-B. Mao, Z.-Q. Song, H.-B. Yao, H. Cölfen, X.-S. Luo, F. Zhang, Z. Pan, Y.-F. Meng, Y. Ni and S.-H. Yu, Nat. Commun., 2017, 8, 1–8 CrossRef CAS.
- A. Malik, F. U. Rehman, K. U. Shah, S. S. Naz and S. Qaisar, J. Biomed. Mater. Res., Part B, 2021, 109, 1465–1477 CrossRef CAS PubMed.
- A. J. Gale, Toxicol. Pathol., 2011, 39, 273–280 CrossRef PubMed.
- S. Palta, R. Saroa and A. Palta, Indian J. Anaesth., 2014, 58, 515–523 CrossRef CAS PubMed.
- B. Dahlbäck, Lancet, 2000, 355, 1627–1632 CrossRef PubMed.
- H. H. Versteeg, J. W. Heemskerk, M. Levi and P. H. Reitsma, Physiol. Rev., 2013, 93, 327–358 CrossRef CAS PubMed.
- D. A. Hickman, C. L. Pawlowski, U. D. S. Sekhon, J. Marks and A. S. Gupta, Adv. Mater., 2018, 30, 1700859 CrossRef PubMed.
- Y. Sang, M. Roest, B. de Laat, P. G. de Groot and D. Huskens, Blood Rev., 2021, 46, 100733 CrossRef CAS PubMed.
- B. B. Hsu, W. Conway, C. M. Tschabrunn, M. Mehta, M. B. Perez-Cuevas, S. G. Zhang and P. T. Hammond, ACS Nano, 2015, 9, 9394–9406 CrossRef CAS PubMed.
- Q. Tang, C. Chen, Y. Jiang, J. Huang, Y. Liu, P. M. Nthumba, G. Gu, X. Wu, Y. Zhao and J. Ren, J. Mater. Chem. B, 2020, 8, 5756–5764 RSC.
- S. An, E. J. Jeon, J. Jeon and S. W. Cho, Mater. Horiz., 2019, 6, 1169–1178 RSC.
- T. Maesen, Stud. Surf. Sci. Catal., 2007, 168, 1–12 CrossRef.
- X. Q. Shang, H. Chen, V. Castagnola, K. Liu, L. Boselli, V. Petseva, L. S. Yu, L. P. Xiao, M. He, F. J. Wang, K. A. Dawson and J. Fan, Nat. Catal., 2021, 4, 607–614 CrossRef CAS.
- V. Margulis, E. D. Matsumoto, R. Svatek, W. Kabbani, J. A. Cadeddu and Y. Lotan, J. Urol., 2005, 174, 761–764 CrossRef PubMed.
- P. Rhee, C. Brown, M. Martin, A. Salim, D. Plurad, D. Green, L. Chambers, D. Demetriades, G. Velmahos and H. Alam, J. Trauma: Inj., Infect., Crit. Care, 2008, 64, 1093–1099 CrossRef PubMed.
- J. K. Wright, J. Kalns, E. A. Wolf, F. Traweek, S. Schwarz, C. K. Loeffler, W. Snyder, L. D. Yantis and J. Eggers, J. Trauma: Inj., Infect., Crit. Care, 2004, 57, 224–230 CrossRef PubMed.
- T. A. Ostomel, P. K. Stoimenov, P. A. Holden, H. B. Alam and G. D. Stucky, J. Thromb. Thrombolysis, 2006, 22, 55–67 CrossRef CAS PubMed.
- L. S. Yu, B. Yu, H. Chen, X. Q. Shang, M. He, M. C. Lin, D. Li, W. Z. Zhang, Z. Z. Kang, J. C. Li, F. J. Wang, L. P. Xiao, Q. Wang and J. Fan, Nano Res., 2021, 14, 3309–3318 CrossRef CAS.
- A. Jmal Ayadi, S. Baklouti, A. Kammoun and J. Soro, Int. J. Appl. Ceram. Technol., 2021, 19, 1477–1489 CrossRef.
- H. H. Murray, Clay Miner., 1999, 34, 39–49 CrossRef CAS.
- T. A. Ostomel, Q. Shi, P. K. Stoimenov and G. D. Stucky, Langmuir, 2007, 23, 11233–11238 CrossRef CAS PubMed.
- M. J. Sena, G. Douglas, T. Gerlach, J. K. Grayson, K. O. Pichakron and D. Zierold, J. Surg. Res., 2013, 183, 704–709 CrossRef CAS PubMed.
- B. Gegel, J. Burgert, J. Gasko, C. Campbell, M. Martens, J. Keck, H. Reynolds, M. Loughren and D. Johnson, Mil. Med., 2012, 177, 1543–1547 CrossRef PubMed.
- M. E. Chavez-Delgado, C. V. Kishi-Sutto, X. N. Albores de la-Riva, M. Rosales-Cortes and P. Gamboa-Sanchez, J. Surg. Res., 2014, 192, 678–685 CrossRef CAS PubMed.
- M. Long, Y. Zhang, P. Huang, S. Chang, Y. Hu, Q. Yang, L. Mao and H. Yang, Adv. Funct. Mater., 2018, 28, 1704452 CrossRef.
- L. L. Hench, J. Am. Ceram. Soc., 1991, 74, 1487–1510 CrossRef CAS.
- K. R. Ward, M. H. Tiba, W. H. Holbert, C. R. Blocher, G. T. Draucker, E. K. Proffitt, G. L. Bowlin, R. R. Ivatury and R. F. Diegelmann, J. Trauma: Inj., Infect., Crit. Care, 2007, 63, 276–283 CrossRef PubMed.
- B. S. Kheirabadi, J. E. Mace, I. B. Terrazas, C. G. Fedyk, J. S. Estep, M. A. Dubick and L. H. Blackbourne, J. Trauma: Inj., Infect., Crit. Care, 2010, 68, 269–277 CrossRef CAS PubMed.
- M. Manzano and M. Vallet-Regí, J. Mater. Chem., 2010, 20, 5593–5604 RSC.
- N. Rahmat, A. Z. Abdullah and A. R. Mohamed, Am. J. Appl. Sci., 2010, 7, 1579–1586 CrossRef CAS.
- T. A. Ostomel, Q. H. Shi and G. D. Stucky, J. Am. Chem. Soc., 2006, 128, 8384–8385 CrossRef CAS PubMed.
- C. Dai, Y. Yuan, C. Liu, J. Wei, H. Hong, X. Li and X. Pan, Biomaterials, 2009, 30, 5364–5375 CrossRef CAS PubMed.
- C. Wang, H. Zhou, H. Niu, X. Ma, Y. Yuan, H. Hong and C. Liu, Biomater. Sci., 2018, 6, 3318–3331 RSC.
- R. Bhat, A. Ribes, N. Mas, E. Aznar, F. Sancenon, M. D. Marcos, J. R. Murguia, A. Venkataraman and R. Martinez-Manez, Langmuir, 2016, 32, 1195–1200 CrossRef CAS.
- X. Sun, Y. Fang, Z. Tang, Z. Wang, X. Liu and H. Liu, Int. J. Biol. Macromol., 2019, 127, 311–319 CrossRef CAS PubMed.
- X. Yan, C. Yu, X. Zhou, J. Tang and D. Zhao, Angew. Chem., Int. Ed. Engl., 2004, 43, 5980–5984 CrossRef CAS PubMed.
- I. Izquierdo-Barba, A. J. Salinas and M. Vallet-Regí, Int. J. Appl. Glass Sci., 2013, 4, 149–161 CrossRef CAS.
- M. Vallet-Regi, I. Izquierdo-Barba and M. Colilla, Philos. Trans. R. Soc., A, 2012, 370, 1400–1421 CrossRef CAS PubMed.
- T. A. Ostomel, Q. Shi, C. K. Tsung, H. Liang and G. D. Stucky, Small, 2006, 2, 1261–1265 CrossRef CAS PubMed.
- S. Pourshahrestani, E. Zeimaran, N. Adib Kadri, N. Gargiulo, S. Samuel, S. V. Naveen, T. Kamarul and M. R. Towler, J. Mater. Chem. B, 2016, 4, 71–86 RSC.
- A. Mendonca, M. S. Rahman, A. Alhalawani, O. Rodriguez, R. C. Gallant, H. Ni, O. M. Clarkin and M. R. Towler, J. Biomed. Mater. Res., Part B, 2019, 107, 2229–2237 CrossRef CAS PubMed.
- C. Zheng, Q. Bai, W. Wu, K. Han, Q. Zeng, K. Dong, Y. Zhang and T. Lu, Int. J. Biol. Macromol., 2021, 179, 507–518 CrossRef CAS.
- E. V. Armbrust, Nature, 2009, 459, 185–192 CrossRef CAS.
- B. Delalat, V. C. Sheppard, S. Rasi Ghaemi, S. Rao, C. A. Prestidge, G. McPhee, M. L. Rogers, J. F. Donoghue, V. Pillay, T. G. Johns, N. Kroger and N. H. Voelcker, Nat. Commun., 2015, 6, 8791 CrossRef CAS.
- S. Maher, T. Kumeria, M. S. Aw and D. Losic, Adv. Healthcare Mater., 2018, 7, e1800552 CrossRef.
- J. Lee, H. A. Lee, M. Shin, L. J. Juang, C. J. Kastrup, G. M. Go and H. Lee, ACS Nano, 2020, 14, 4755–4766 CrossRef CAS.
- Y. Luo, S. Li, K. Shen, Y. Song, J. Zhang, W. Su and X. Yang, Materials, 2021, 14, 3752 CrossRef CAS PubMed.
- C. Feng, J. Li, G. S. Wu, Y. Z. Mu, M. Kong, C. Q. Jiang, X. J. Cheng, Y. Liu and X. G. Chen, ACS Appl. Mater. Interfaces, 2016, 8, 34234–34243 CrossRef CAS.
- Y. Wang, Y. Fu, J. Li, Y. Mu, X. Zhang, K. Zhang, M. Liang, C. Feng and X. Chen, Carbohydr. Polym., 2018, 200, 6–14 CrossRef CAS.
- S. Pourshahrestani, E. Zeimaran, N. A. Kadri, N. Gargiulo, H. M. Jindal, S. V. Naveen, S. D. Sekaran, T. Kamarul and M. R. Towler, ACS Appl. Mater. Interfaces, 2017, 9, 31381–31392 CrossRef CAS PubMed.
- J. Li, X. J. Sun, K. C. Zhang, G. N. Yang, Y. Z. Mu, C. Su, J. H. Pang, T. T. Chen, X. G. Chen and C. Feng, Adv. Healthcare Mater., 2020, 9, 13 Search PubMed.
- L. Yu, X. Shang, H. Chen, L. Xiao, Y. Zhu and J. Fan, Nat. Commun., 2019, 10, 1932 CrossRef PubMed.
- Z. Zhang, T. Liu, Z. Qi, F. Li, K. Yang, S. Ding, S. Lin and F. Tian, Silicon, 2022, 14, 10521–10534 CrossRef CAS.
- M. Yin, Y. Wang, Y. Zhang, X. Ren, Y. Qiu and T. S. Huang, Carbohydr. Polym., 2020, 232, 115823 CrossRef CAS PubMed.
- M. B. Dowling, R. Kumar, M. A. Keibler, J. R. Hess, G. V. Bochicchio and S. R. Raghavan, Biomaterials, 2011, 32, 3351–3357 CrossRef CAS PubMed.
- M. Delyanee, A. Solouk, S. Akbari and M. Daliri Joupari, Polym. Adv. Technol., 2021, 32, 3934–3947 CrossRef CAS.
- J. Chen, W. Cheng, S. Chen, W. Xu, J. Lin, H. Liu and Q. Chen, Nanoscale, 2018, 10, 22818–22829 RSC.
- T. Jia, J. Chen, X. Feng and J. Chang, J. Clin. Rehabil. Tissue Eng. Res., 2011, 15, 7877–7880 CAS.
- M. H. Kim, J. Lee, J. N. Lee, H. Lee and W. H. Park, Acta Biomater., 2021, 123, 254–262 CrossRef CAS PubMed.
- S. Liang, Y. Zhang, H. Wang, Z. Xu, J. Chen, R. Bao, B. Tan, Y. Cui, G. Fan, W. Wang, W. Wang and W. Liu, Adv. Mater., 2018, 30, e1704235 CrossRef.
- M. Lo Presti, G. Rizzo, G. M. Farinola and F. G. Omenetto, Adv. Sci., 2021, 8, e2004786 CrossRef.
- X. Li, Y. C. Li, M. Chen, Q. Shi, R. Sun and X. Wang, J. Mater. Chem. B, 2018, 6, 6544–6549 RSC.
- X. Fan, S. Wang, Y. Fang, P. Li, W. Zhou, Z. Wang, M. Chen and H. Liu, Mater. Sci. Eng., C, 2020, 109, 110649 CrossRef CAS PubMed.
- X. Zhao, B. Guo, H. Wu, Y. Liang and P. X. Ma, Nat. Commun., 2018, 9, 2784 CrossRef.
- Y. F. Shen, J. H. Huang, Z. E. Wu, K. Y. Wang, J. Zheng, L. Cai, X. L. Li, H. Gao, X. Y. Jin and J. F. Li, Mater. Sci. Eng., C, 2020, 111, 110841 CrossRef CAS.
- M. N. Sundaram, S. Amirthalingam, U. Mony, P. K. Varma and R. Jayakumar, Int. J. Biol. Macromol., 2019, 129, 936–943 CrossRef CAS PubMed.
- P. Fathi, M. Sikorski, K. Christodoulides, K. Langan, Y. S. Choi, M. Titcomb, A. Ghodasara, O. Wonodi, H. Thaker, M. Vural, A. Behrens and P. Kofinas, J. Biomed. Mater. Res., Part B, 2018, 106, 1662–1671 CrossRef CAS PubMed.
- S. K. Singh, M. K. Singh, M. K. Nayak, S. Kumari, S. Shrivastava, J. J. A. Gracio and D. Dash, ACS Nano, 2011, 5, 4987–4996 CrossRef CAS PubMed.
- G. Reina, J. M. Gonzalez-Dominguez, A. Criado, E. Vazquez, A. Bianco and M. Prato, Chem. Soc. Rev., 2017, 46, 4400–4416 RSC.
- C. Zhang, Z.-L. Hou, B.-X. Zhang, H.-M. Fang and S. Bi, Carbon, 2018, 137, 467–474 CrossRef CAS.
- R. R. Nair, H. A. Wu, P. N. Jayaram, I. V. Grigorieva and A. K. Geim, Science, 2012, 335, 442–444 CrossRef CAS.
- Y. Liang, C. Xu, G. Li, T. Liu, J. F. Liang and X. Wang, Colloids Surf., B, 2018, 169, 168–175 CrossRef CAS PubMed.
- W. Zhang, L. Zhao, C. Gao, J. Huang, Q. Li and Z. Zhang, J. Mater. Chem. B, 2021, 9, 9754–9763 RSC.
- X. Xu, S. Fu, J. Guo, H. Li, Y. Huang and X. Duan, Mater. Today, 2021, 42, 162–177 CrossRef CAS.
- C. Li, F. Li, J. Chen, H. Wu, Y. Lin, C. Chen, P. Zhang, Q. Wang, J. Liu and G. Deng, Mater. Des., 2022, 213, 110365 CrossRef CAS.
- X. C. Wang, J. Chang and C. T. Wu, Appl. Mater. Today, 2018, 11, 308–319 CrossRef.
- F. Groeber, M. Holeiter, M. Hampel, S. Hinderer and K. Schenke-Layland, Adv. Drug Delivery Rev., 2011, 63, 352–366 CrossRef CAS PubMed.
- J. Ma and C. Wu, Exploration, 2022, 20210083 CrossRef.
- Q. Q. Yu, J. Chang and C. T. Wu, J. Mater. Chem. B, 2019, 7, 5449–5460 RSC.
- Q. Xu, F. Jiang, G. Y. Guo, E. D. Wang, M. R. Younis, Z. W. B. Zhang, F. Y. Zhang, Z. G. Huan, C. Fan, C. Yang, H. Shen and J. Chang, Nano Today, 2021, 41, 101330 CrossRef CAS.
- Q. Q. Yu, Y. M. Han, T. Tian, Q. Zhou, Z. F. Yi, J. Chang and C. T. Wu, Biomaterials, 2019, 194, 25–35 CrossRef CAS PubMed.
- C. Qin and C. Wu, View, 2022, 20210018 CrossRef.
- H. P. Lee and A. K. Gaharwar, Adv. Sci., 2020, 7, 2000863 CrossRef CAS PubMed.
- D. Stan, C. Tanase, M. Avram, R. Apetrei, N. B. Mincu, A. L. Mateescu and D. Stan, Exp. Dermatol., 2021, 30, 1218–1232 CrossRef PubMed.
- N. Ninan, M. Muthiah, I. K. Park, T. W. Wong, S. Thomas and Y. Grohens, Polym. Rev., 2015, 55, 453–490 CrossRef CAS.
- J. F. Wu, C. Qin, J. G. Ma, H. J. Zhang, J. Chang, L. X. Mao and C. T. Wu, Appl. Mater. Today, 2021, 23, 101015 CrossRef.
- S. P. Zhong, Y. Z. Zhang and C. T. Lim, Wiley Interdiscip. Rev.: Nanomed. Nanobiotechnol., 2010, 2, 510–525 CAS.
- E. A. Kamoun, E. R. S. Kenawy and X. Chen, J. Adv. Res., 2017, 8, 217–233 CrossRef CAS PubMed.
- G. D. Mogoşanu and A. M. Grumezescu, Int. J. Pharm., 2014, 463, 127–136 CrossRef PubMed.
- A. M. Brokesh and A. K. Gaharwar, ACS Appl. Mater. Interfaces, 2020, 12, 5319–5344 CrossRef CAS PubMed.
- E. Wang, X. Li, Y. Zhang, L. Ma, Q. Xu, Y. Yue, W. Wang, Q. Li, J. Yu and J. Chang, Adv. Funct. Mater., 2021, 31, 2101505 CrossRef CAS.
- L. Zhou, Y. Xi, Y. Xue, M. Wang, Y. Liu, Y. Guo and B. Lei, Adv. Funct. Mater., 2019, 29, 1806883 CrossRef.
- Y. Zhou, C. J. Wan, Y. S. Yang, H. Yang, S. C. Wang, Z. D. Dai, K. J. Ji, H. Jiang, X. D. Chen and Y. Long, Adv. Funct. Mater., 2019, 29, 1806220 CrossRef.
- X. C. Wang, J. M. Xue, B. Ma, J. F. Wu, J. Chang, M. Gelinsky and C. T. Wu, Adv. Mater., 2020, 32, 2005140 CrossRef CAS PubMed.
- Y. Zhang, M. L. Chang, F. Bao, M. Xing, E. Wang, Q. Xu, Z. G. Huan, F. Guo and J. Chang, Nanoscale, 2019, 11, 6315–6333 RSC.
- Q. Lei, D. F. He, L. P. Ding, F. H. Kong, P. Y. He, J. D. Huang, J. M. Guo, C. J. Brinker, G. X. Luo, W. Zhu and Y. L. Yu, Adv. Funct. Mater., 2022, 32, 2113269 CrossRef CAS.
- P. Pleguezuelos-Beltrán, P. Gálvez-Martín, D. Nieto-García, J. A. Marchal and E. López-Ruiz, Bioact. Mater., 2022, 16, 187–203 CrossRef PubMed.
- W. P. Ma, H. S. Ma, P. F. Qiu, H. J. Zhang, Z. B. Yang, B. Ma, J. Chang, X. Shi and C. T. Wu, Biomaterials, 2021, 279, 121225 CrossRef CAS PubMed.
- J. Ouyang, X. Ji, X. Zhang, C. Feng, Z. Tang, N. Kong, A. Xie, J. Wang, X. Sui, L. Deng, Y. Liu, S. Kim Jong, Y. Cao and W. Tao, Proc. Natl. Acad. Sci. U. S. A., 2020, 117, 28667–28677 CrossRef CAS PubMed.
- J. G. Ma, C. Qin, J. F. Wu, H. J. Zhang, H. Zhuang, M. Zhang, Z. W. B. Zhang, L. L. Ma, X. Wang, B. Ma, J. Chang and C. T. Wu, Adv. Healthcare Mater., 2021, 10, 2100523 CrossRef CAS PubMed.
- L. Siebert, E. Luna-Ceron, L. E. Garcia-Rivera, J. Oh, J. Jang, D. A. Rosas-Gomez, M. D. Perez-Gomez, G. Maschkowitz, H. Fickenscher, D. Oceguera-Cuevas, C. G. Holguin-Leon, B. Byambaa, M. A. Hussain, E. Enciso-Martinez, M. Cho, Y. Lee, N. Sobahi, A. Hasan, D. P. Orgill, Y. K. Mishra, R. Adelung, E. Lee and S. R. Shin, Adv. Funct. Mater., 2021, 31, 2007555 CrossRef CAS PubMed.
- H. S. Ma, C. Feng, J. Chang and C. T. Wu, Acta Biomater., 2018, 79, 37–59 CrossRef CAS PubMed.
- A. C. Daly, M. E. Prendergast, A. J. Hughes and J. A. Burdick, Cell, 2021, 184, 18–32 CrossRef CAS PubMed.
- B. K. Sun, Z. Siprashvili and P. A. Khavari, Science, 2014, 346, 941–945 CrossRef CAS PubMed.
- R. Ross and G. Odland, J. Cell Biol., 1968, 39, 152–168 CrossRef CAS PubMed.
- Y. Liang, J. He and B. Guo, ACS Nano, 2021, 15, 12687–12722 CrossRef CAS PubMed.
- W. C. Chen, D. Thiboutot and C. C. Zouboulis, J. Invest. Dermatol., 2002, 119, 992–1007 CrossRef CAS PubMed.
- E. Ren, C. Zhang, D. Li, X. Pang and G. Liu, Viex, 2020, 1, 20200052 Search PubMed.
- M. H. Razali, N. A. Ismail and K. A. Mat Amin, Int. J. Biol. Macromol., 2020, 153, 1117–1135 CrossRef CAS PubMed.
- R. Ahmed, M. Tariq, I. Ali, R. Asghar, P. Noorunnisa Khanam, R. Augustine and A. Hasan, Int. J. Biol. Macromol., 2018, 120, 385–393 CrossRef CAS PubMed.
- G. Guo, Q. Xu, C. Zhu, J. Yu, Q. Wang, J. Tang, Z. Huan, H. Shen, J. Chang and X. Zhang, Appl. Mater. Today, 2021, 22, 100888 CrossRef.
- J. Y. Li, D. Zhai, F. Lv, Q. Q. Yu, H. S. Ma, J. B. Yin, Z. F. Yi, M. Y. Liu, J. Chang and C. T. Wu, Acta Biomater., 2016, 36, 254–266 CrossRef CAS PubMed.
- A. P. Veith, K. Henderson, A. Spencer, A. D. Sligar and A. B. Baker, Adv. Drug Delivery Rev., 2019, 146, 97–125 CrossRef CAS PubMed.
- F. Bao, G. Pei, Z. C. Wu, H. Zhuang, Z. W. B. Zhang, Z. G. Huan, C. T. Wu and J. Chang, Adv. Funct. Mater., 2020, 30, 2005422 CrossRef CAS.
- M. Xing, Y. Jiang, W. Bi, L. Gao, Y.-L. Zhou, S.-L. Rao, L.-L. Ma, Z.-W. Zhang, H.-T. Yang and J. Chang, Adv. Sci., 2021, 7, eabe0726 CrossRef CAS PubMed.
- Q. Yu, Y. Han, X. Wang, C. Qin, D. Zhai, Z. Yi, J. Chang, Y. Xiao and C. Wu, ACS Nano, 2018, 12, 2695–2707 CrossRef CAS PubMed.
- Z. Y. Zhao, G. Li, H. T. Ruan, K. Y. Chen, Z. W. Cai, G. H. Lu, R. M. Li, L. F. Deng, M. Cai and W. G. Cui, ACS Nano, 2021, 15, 13041–13054 CrossRef CAS PubMed.
- T. T. Weng, P. Wu, W. Zhang, Y. R. Zheng, Q. Li, R. H. Jin, H. J. Chen, C. G. You, S. X. Guo, C. M. Han and X. G. Wang, J. Transl. Med., 2020, 18, 53 CrossRef PubMed.
- W. Rahmani, S. Sinha and J. Biernaskie, npj Regener. Med., 2020, 5, 9 CrossRef PubMed.
- Z. W. B. Zhang, W. B. Li, Y. Liu, Z. G. Yang, L. L. Ma, H. Zhuang, E. D. Wang, C. T. Wu, Z. G. Huan, F. Guo and J. Chang, Bioact. Mater., 2021, 6, 1910–1920 CrossRef CAS PubMed.
- Z. W. B. Zhang, Y. Zhang, W. B. Li, L. L. Ma, E. D. Wang, M. Xing, Y. L. Zhou, Z. G. Huan, F. Guo and J. Chang, Appl. Mater. Today, 2021, 23, 101065 CrossRef.
- Y. Liang, B. Chen, M. Li, J. He, Z. Yin and B. Guo, Biomacromolecules, 2020, 21, 1841–1852 CrossRef CAS PubMed.
- A. Maleki, J. He, S. Bochani, V. Nosrati, M.-A. Shahbazi and B. Guo, ACS Nano, 2021, 15, 18895–18930 CrossRef CAS PubMed.
- J. Wang, X.-Y. Chen, Y. Zhao, Y. Yang, W. Wang, C. Wu, B. Yang, Z. Zhang, L. Zhang, Y. Liu, X. Du, W. Li, L. Qiu, P. Jiang, X.-Z. Mou and Y.-Q. Li, ACS Nano, 2019, 13, 11686–11697 CrossRef CAS PubMed.
- A. S. Montaser, M. Rehan and M. E. El-Naggar, Int. J. Biol. Macromol., 2019, 124, 1016–1024 CrossRef CAS PubMed.
- M. Li, X. Liu, L. Tan, Z. Cui, X. Yang, Z. Li, Y. Zheng, K. W. K. Yeung, P. K. Chu and S. Wu, Biomater. Sci., 2018, 6, 2110–2121 RSC.
- L. Sheng, Z. Zhang, Y. Zhang, E. Wang, B. Ma, Q. Xu, L. Ma, M. Zhang, G. Pei and J. Chang, Biomaterials, 2021, 264, 120414 CrossRef CAS PubMed.
- Q. Jia, Q. Song, P. Li and W. Huang, Adv. Healthcare Mater., 2019, 8, 1900608 CrossRef PubMed.
- Y. Zhang, P. Huang, D. Wang, J. Chen, W. Liu, P. Hu, M. Huang, X. Chen and Z. Chen, Nanoscale, 2018, 10, 15485–15495 RSC.
- F. Mariani, M. Serafini, I. Gualandi, D. Arcangeli, F. Decataldo, L. Possanzini, M. Tessarolo, D. Tonelli, B. Fraboni and E. Scavetta, ACS Sens., 2021, 6, 2366–2377 CrossRef CAS PubMed.
- M. Wu, J. S. Chen, W. J. Huang, B. Yan, Q. Y. Peng, J. F. Liu, L. Y. Chen and H. B. Zeng, Biomacromolecules, 2020, 21, 2409–2420 CrossRef CAS PubMed.
- C. R. F. Junior, R. d. S. Fernandes, M. R. de Moura and F. A. Aouada, Mater. Today Commun., 2020, 23, 100936 CrossRef CAS.
- H. Guo, S. Tan, J. Gao and L. Wang, J. Mater. Chem. B, 2020, 8, 1759–1770 RSC.
- X. Z. Zhang, C. H. Tian, Z. R. Chen and G. Zhao, Adv. Ther., 2020, 3, 2000001 CrossRef CAS.
- L. Mao, S. M. Hu, Y. H. Gao, L. Wang, W. W. Zhao, L. N. Fu, H. Y. Cheng, L. Xia, S. X. Xie, W. L. Ye, Z. J. Shi and G. Yang, Adv. Healthcare Mater., 2020, 9, 2000872 CrossRef CAS PubMed.
- Q. N. Wu, C. Yang, W. Chen, K. X. Chen, H. J. Chen, F. M. Liu, D. Liu, H. T. Lin, X. Xie and W. R. Chen, Adv. Sci., 2022, 9, 2202506 CrossRef CAS PubMed.
- F. Chen, X. Liu, X. Ge, Y. Wang, Z. Zhao, X. Zhang, G.-Q. Chen and Y. Sun, Chem. Eng. J., 2023, 451, 138899 CrossRef CAS.
- N. Elshazly, A. Khalil, M. Saad, M. Patruno, J. Chakraborty and M. Marei, Materials, 2020, 13, 2603 CrossRef CAS PubMed.
- Z. Zhang, X. Zhao, C. Wang, Y. Huang, Y. Han and B. Guo, Acta Biomater., 2022, 151, 197–209 CrossRef CAS PubMed.
- P. Kolar, K. Schmidt-Bleek, H. Schell, T. Gaber, D. Toben, G. Schmidmaier, C. Perka, F. Buttgereit and G. N. Duda, Tissue Eng., Part B, 2010, 16, 427–434 CrossRef PubMed.
- S. S. Smyth, R. P. McEver, A. S. Weyrich, C. N. Morrell, M. R. Hoffman, G. M. Arepally, P. A. French, H. L. Dauerman, R. C. Becker and P. Platelet Colloquium, J. Thromb. Haemostasis, 2009, 7, 1759–1766 CrossRef CAS PubMed.
- J. Ware, A. Corken and R. Khetpal, Curr. Opin. Hematol., 2013, 20, 451–456 CrossRef CAS PubMed.
- M. A. Burkhardt, I. Gerber, C. Moshfegh, M. S. Lucas, J. Waser, M. Y. Emmert, S. P. Hoerstrup, F. Schlottig and V. Vogel, Biomater. Sci., 2017, 5, 2009–2023 RSC.
-
A. L. Fogelson and K. B. Neeves, in Annual Review of Fluid Mechanics, Vol. 47, ed. S. H. Davis and P. Moin, Palo Alto, 2015, vol. 47, pp. 377–403 Search PubMed.
- Z. Wu, W. Zhou, W. Deng, C. Xu, Y. Cai and X. Wang, ACS Appl. Mater. Interfaces, 2020, 12, 20307–20320 CrossRef CAS PubMed.
- S. Shakya, Y. He, X. Ren, T. Guo, A. Maharjan, T. Luo, T. Wang, R. Dhakhwa, B. Regmi, H. Li, R. Gref and J. Zhang, Small, 2019, 15, e1901065 CrossRef PubMed.
- M. Zhang, D. Wang, N. Ji, S. Lee, G. Wang, Y. Zheng, X. Zhang, L. Yang, Z. Qin and Y. Yang, Polymers, 2021, 13, 2812 CrossRef CAS PubMed.
- G. Xu, N. Xu, T. Ren, C. Chen, J. Li, L. Ding, Y. Chen, G. Chen, Z. Li and Y. Yu, Int. J. Biol. Macromol., 2022, 208, 760–771 CrossRef CAS PubMed.
- Q. Li, E. Hu, K. Yu, R. Xie, F. Lu, B. Lu, R. Bao, T. Zhao, F. Dai and G. Lan, Adv. Funct. Mater., 2020, 30, 2004153 CrossRef CAS.
- X. Ren, Z. Xu, L. Wang, K. Meng, H. Wang and H. Zhao, Mater. Res. Express, 2019, 6, 125409 CrossRef CAS.
- Y. Zhou, H. Li, J. Liu, Y. Xu, Y. Wang, H. Ren and X. Li, Polym. Adv. Technol., 2019, 30, 143–152 CrossRef CAS.
- K. M. Rao, M. Suneetha, G. T. Park, A. G. Babu and S. S. Han, Int. J. Biol. Macromol., 2020, 155, 71–80 CrossRef CAS PubMed.
- W. Nie, X. Dai, D. Li, D. McCoul, G. J. Gillispie, Y. Zhang, B. Yu and C. He, ACS Biomater. Sci. Eng., 2018, 4, 3588–3599 CrossRef CAS PubMed.
- A. Meddahi-Pelle, A. Legrand, A. Marcellan, L. Louedec, D. Letourneur and L. Leibler, Angew. Chem., Int. Ed. Engl., 2014, 53, 6369–6373 CrossRef CAS PubMed.
- A. C. Brown, S. E. Stabenfeldt, B. Ahn, R. T. Hannan, K. S. Dhada, E. S. Herman, V. Stefanelli, N. Guzzetta, A. Alexeev, W. A. Lam, L. A. Lyon and T. H. Barker, Nat. Mater., 2014, 13, 1108–1114 CrossRef CAS PubMed.
- S. Nandi, E. P. Sproul, K. Nellenbach, M. Erb, L. Gaffney, D. O. Freytes and A. C. Brown, Biomater. Sci., 2019, 7, 669–682 RSC.
- H. Chen, X. Q. Shang, L. S. Yu, L. P. Xiao and J. Fan, J. Biomater. Appl., 2020, 34, 988–997 CrossRef CAS PubMed.
- P. Li, Q. Bu, G. Cao, P. Liu, W. Fang, J. Liu, J. Liu and X. Li, Med. J. Chin. People's Liberation Army, 2021, 46, 175–180 Search PubMed.
- J. He, Z. Zhang, Y. Yang, F. Ren, J. Li, S. Zhu, F. Ma, R. Wu, Y. Lv, G. He, B. Guo and D. Chu, Nano-Micro Lett., 2021, 13, 80 CrossRef CAS PubMed.
Footnote |
† These authors contributed equally to this work. |
|
This journal is © The Royal Society of Chemistry 2023 |
Click here to see how this site uses Cookies. View our privacy policy here.