DOI:
10.1039/D3SC04597H
(Review Article)
Chem. Sci., 2023,
14, 13325-13345
Advancing cell surface modification in mammalian cells with synthetic molecules
Received
31st August 2023
, Accepted 30th October 2023
First published on 10th November 2023
Abstract
Biological cells, being the fundamental entities of life, are widely acknowledged as intricate living machines. The manipulation of cell surfaces has emerged as a progressively significant domain of investigation and advancement in recent times. Particularly, the alteration of cell surfaces using meticulously crafted and thoroughly characterized synthesized molecules has proven to be an efficacious means of introducing innovative functionalities or manipulating cells. Within this realm, a diverse array of elegant and robust strategies have been recently devised, including the bioorthogonal strategy, which enables selective modification. This review offers a comprehensive survey of recent advancements in the modification of mammalian cell surfaces through the use of synthetic molecules. It explores a range of strategies, encompassing chemical covalent modifications, physical alterations, and bioorthogonal approaches. The review concludes by addressing the present challenges and potential future opportunities in this rapidly expanding field.
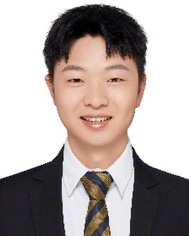 He Yang | He Yang received his BS degree from the Soochow University in 2019, and currently is pursuing his PhD degree under the supervision of Prof. Hong Chen and Prof. Gaojian Chen at the College of Chemistry, Chemical Engineering and Materials Science, Soochow University. His research interests are in cell surface modification and polymer biomimetic materials. |
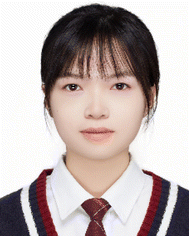 Lihua Yao | Lihua Yao became a master's student at the College of Chemistry, Chemical Engineering and Materials Science of Soochow University in 2022, studying under the guidance of Prof. Hong Chen and Prof. Gaojian Chen. Her research interests center on click-chemistry and cell surface modification. |
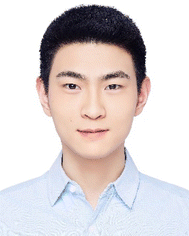 Yichen Wang | Yichen Wang received his BS degree from Soochow University in 2022, and is currently pursuing his PhD degree under the supervision of Prof. Hong Chen at the College of Chemistry, Chemical Engineering and Materials Science, Soochow University. His research interests are in cell modification and intracellular macromolecule delivery. |
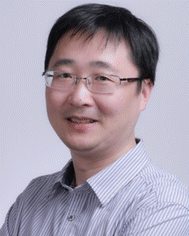 Gaojian Chen | Gaojian Chen is a professor at Soochow University. He began his MSc research in Prof. Xiulin Zhu's group at Soochow University in 2000, and later pursued his PhD degree in Prof. David Haddleton's group at the University of Warwick in 2004. Following that, he worked as a postdoctoral research fellow with Prof. Martina Stenzel at the University of New South Wales and Prof. Chi Wu at the Chinese University of Hong Kong. In 2017, he was elected as a Fellow of the Royal Society of Chemistry (FRSC). Currently, his research focuses on the synthesis and application of glycopolymer-based materials. |
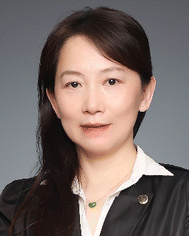 Hong Chen | Hong Chen is a professor at Soochow University. She earned her PhD degree from Nanjing University in 2001 and then worked at McMaster University as a postdoctoral fellow (2001–2004). She became a professor at Wuhan University of Technology (2004–2009), and joined Soochow University in 2010. She was the winner of the National Science Fund for Distinguished Young Scholars (2011). She was admitted as a Fellow of the Royal Society of Chemistry (FRSC) in 2014 and a Fellow of the International Union of Societies for Biomaterials Science and Engineering (IUSBSE) in 2019. Her research interests are in macromolecules and biointerfaces. |
1. Introduction
Mammalian cells (hereinafter referred to as “cells”), as natural constituents of organisms, have been propelled into the spotlight in the biomedical field, primarily due to their unique characteristics, such as biosynthesis ability, communication and interaction ability, and migration ability, among others.1–4 Over the past few decades, the manipulation of cells has provided a powerful tool to enhance our understanding of the underlying mechanisms governing various biological behaviors in basic research and has also promoted the development in biomedical applications, such as medical diagnosis and cell-based therapy.5 For instance, mesenchymal stem cells (MSCs), red blood cells (RBCs), and macrophages exhibit promising capabilities as delivery vehicles to transport diagnostic molecules or therapeutic agents.6–8 Immune cells, including T cells and natural killer (NK) cells, have emerged as highly prominent candidates for tumor immunotherapy owing to their specific cytotoxicity against tumor cells while sparing normal cells.9,10
Despite these exciting achievements, the functions of natural cells themselves are limited. The cell surface, also known as the cell membrane, is a highly heterogeneous and dynamic milieu comprising lipids, proteins, carbohydrates, and their complexes, which governs numerous intracellular and extracellular processes.11 Simultaneously, the complex cell surface provides plenty of opportunities for further modification aimed at achieving particular functionalities, a process referred to as cell surface modification. This process serves as a powerful means to facilitate the biomedical application of natural cells.12 One notable example is the universal blood, which involves the modification of red blood cell surfaces to impede the recognition of antigenic sites by antibodies, thereby preventing immune responses caused by blood type incompatibility.13–15 Chemical manipulation of cell behavior and function through modification of cell surfaces using precisely synthesized and well-characterized synthetic molecules, such as polymers, is a captivating area of research.16 Various strategies have been devised for this purpose. While several reviews have provided summaries of certain strategies, there is a noticeable dearth of comprehensive discussions specifically centered on the strategy of cell surface modification using synthetic molecules.16–18
Hence, this paper provides a comprehensive review of the latest advancements in strategies related to the modification of cell surfaces accompanied by a discussion on their biomedical applications, with a particular emphasis on developments from 2017 onwards. These strategies encompass a range of approaches, including chemical covalent approaches, physical techniques, and bioorthogonal methods for synthetic molecules (Fig. 1). The synthetic molecules discussed include specific functional groups, synthetic functional small molecules, synthetic polymers, synthetic nanoparticles, synthetic cell coatings, and synthetic DNA, among others. Lastly, the paper discusses the existing challenges and potential future prospects in this rapidly expanding field.
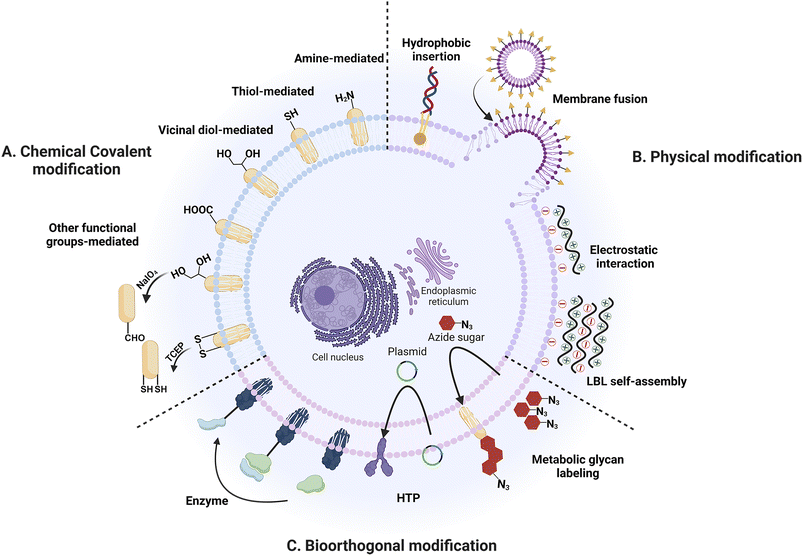 |
| Fig. 1 Mammalian cell surface modification strategies. (A) Chemical covalent modification strategies. (B) Physical modification strategies. (C) Bioorthogonal modification strategies. | |
2. Chemical covalent modification
Chemical covalent modification is a strategic approach that entails the utilization of the chemically reactive functionalities found on the surface of the cell membrane. The cell membrane, a multifaceted chemical structure composed of lipids, proteins, carbohydrates and other components, offers a diverse array of functional groups that can be employed for chemical covalent binding.19 Previous researches have predominantly focused on employing amine, thiol, and vicinal diol groups present on amino acid residues within proteins or sugar residues as the most frequently utilized groups (Fig. 2).20 The stable attachment of synthetic molecules and the absence of cell pretreatment are the primary benefits of this approach, making it a simple yet effective method for modifying cell membranes. However, it is widely recognized that directly modifying cell membranes with reactive functional groups through covalent bonds can potentially impair the functionality of membrane proteins and subsequently impair cellular functions. Consequently, when employing this strategy, careful attention must be paid to both cell viability and effector functions.
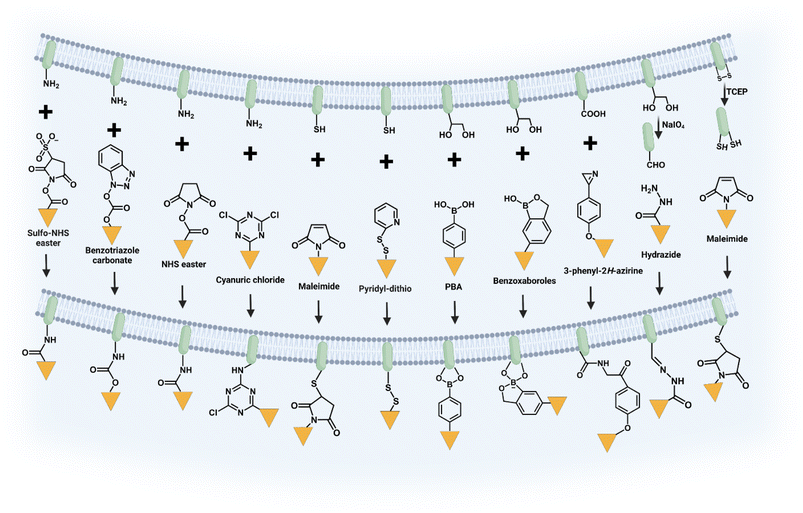 |
| Fig. 2 Illustration of chemical covalent modification through the reactions between functional groups on cells and synthetic molecules. | |
2.1 Amine-mediated covalent modification strategy
Amine groups (–NH2) are mainly present at the lysine residues of proteins and the N-terminus of polypeptide chains. They are widely used for chemical modification of cell membrane surfaces due to their ease of chemical covalent modification and mild reaction conditions. The amine-mediated covalent binding strategy can be achieved through two primary pathways: acylation or alkylation. Generally, these reactions exhibit rapidity and selectivity, resulting in the formation of stable bonds (such as amide or secondary amine bonds) and high yields.
Among various kinds of reagents, N-hydroxysuccinimide (NHS) ester is the most frequently used to covalently bind to –NH2 on cell membranes. Recently, Cai et al. modified human umbilical vein endothelial cells (HUVECs) and human skin fibroblasts (HSFs) with succinimide ester-methoxy polyethylene glycol (NHS-mPEG), resulting in a significant enhancement of cell migration ability and motility through reduction of the focal adhesion area.21 In a separate study shown in Fig. 3A, Wang et al. employed acrylic acid NHS ester (NHS-AA) to immobilize vinyl onto cell membranes, followed by free radical polymerization to covalently attach polymers to the membrane.22 After subsequent ion exchange and electroless deposition (ELD), the polymer-functionalized cells could be converted into metallic biocomposites, which can be applied in the fields of biosensors, electronics, and energy. Sulfo-NHS ester is a more suitable reagent for covalent reactions with –NH2 groups on the cell membrane due to its enhanced water solubility and negative charge, which reduces the transmembrane permeability of the sulfo-NHS ester. For instance, Jasiewicz et al. employed sulfosuccinimidyl 4-(N-maleimidomethyl) cyclohexane-1-carboxylate (sulfo SMCC), a heterobifunctional crosslinker, to modify MSCs by covalently binding to the amines on the cell membrane and subsequently decorating them with heterodimerizing leucine zippers.23 In addition, sulfo-NHS-biotin was also commonly utilized to modify the cell membrane where it serves as a “bridge” and facilitates the decoration of the cell membrane with cargoes through streptavidin–biotin interaction.24–29 In addition to NHS ester derivatives, other types of reagents have been developed for covalently binding to –NH2 including cyanuric chloride and benzotriazole carbonate.30,31
2.2 Thiol-mediated covalent modification strategy
Thiol groups (–SH), mainly located on the cysteine residues of amino acids in proteins, are one of the most potent nucleophiles, stronger than amino groups. Thiol groups are frequently employed for the covalent modification of cell membranes. Maleimide derivatives, which form stable thioether bonds with thiol groups through an energetically favorable Michael addition reaction, are the most widely used reaction reagents because they exhibit high stability and chemoselectivity with thiol groups. The significant advantage of this strategy lies in the extensive availability of commercially accessible reagents and linkers.
Early research was conducted by Irvine's team which focused on surface modification of various cell types containing thiols. They investigated the application of liposomes and liposome-like nanoparticles containing maleimide terminal groups for T cells, hematopoietic stem cells, and other cell surface modifications.32–36 Recently, Wang et al. developed PEGylated solid lipid nanoparticles functionalized with maleimide end groups (SLN-PEG-Mal).36 As is shown in Fig. 3B, by exploiting the reaction between maleimide and sulfhydryl groups on the surface of RBCs, the researchers successfully enhanced the adsorption of modified nanoparticles onto RBCs, leading to significant alterations in the properties and morphology of RBCs. Moreover, these nanoparticle-loaded RBCs exhibited a remarkable ability to be engulfed by macrophages, thereby demonstrating promising potential for targeted drug delivery to macrophages. Wang et al. utilized 2-iminothiolane (Traut's agent), a thiolation reagent, to introduce extra free thiol groups by capping primary amines with thiol groups. This allows them to modify platelets with PD-L1 antibody, thereby reducing post-surgical tumor recurrence and metastasis.35
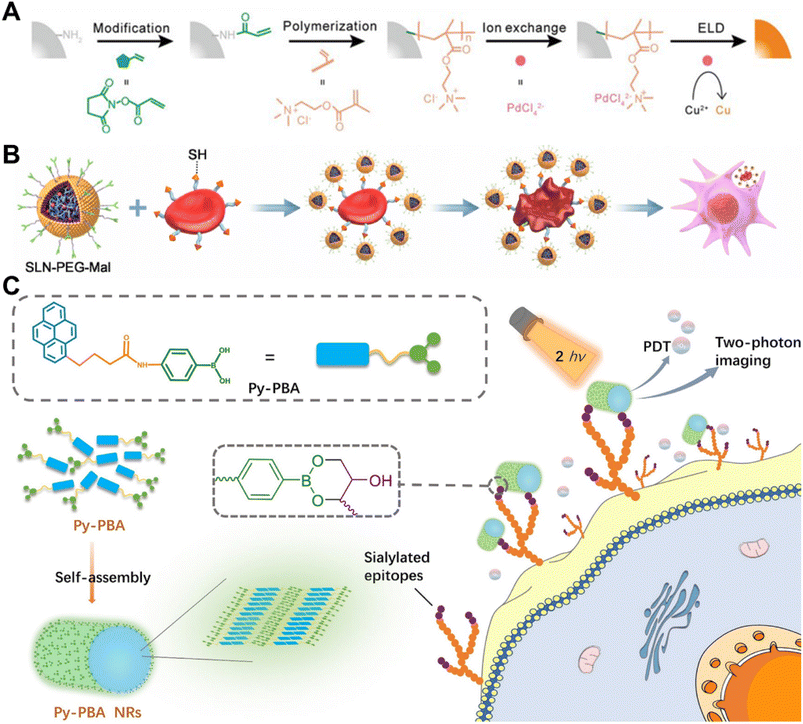 |
| Fig. 3 Methods based on chemical covalent modification. (A) Schematic illustration of realizing polymer-assisted cell metallization by the use of reactions between amino groups on cell membranes and NHS-AA.22 Copyright 2021, John Wiley & Sons, Inc. (B) Schematic illustration of modifying RBCs with SLN-PEG-Mal through reactions between maleimide and thiol groups, leading to their engulfment by macrophages.36 Copyright 2023, Elsevier. (C) Schematic illustration of combining Py-phenylboronic acid (PBA) NRs to the cell membrane by vicinal diol groups for two-photon imaging of cell surface sialic acids and PDT.37 Copyright 2021, American Chemical Society. | |
Research has been conducted to combine maleimide with other functional components in order to develop multifunctional nanoparticles.38 For instance, Luo et al. synthesized double-bound magnetic nanoparticles (DBMN) containing PEG-Mal, hyaluronic acid (HA), and Fe3O4.38 Following a simple incubation, DBMN was able to anchor onto the cell membrane through a Michael addition reaction between the Mal component and sulfhydryl groups on the T cell surface, resulting in magnetized T cells (DBMN-T). Under external magnetic field guidance, DBMN-T exhibited excellent targeting ability. Additionally, HA could bind to highly expressed CD44 on tumor cells, promoting recognition and killing of tumor cells.
In addition to the reaction between maleimides and thiols, cell surface modification could also be achieved through the exchange between disulfide bonds and thiols.39–43 Wayteck et al. incorporated thiol-reactive phospholipids into liposome bilayers with a pyridyldithiopropionate (PDP) head group, which was capable of forming reducible disulfide bonds with thiol groups exposed on the cell surface, thereby enabling the covalent coupling of liposomes.39
2.3 Vicinal diol-mediated covalent modification strategy
Vicinal diol groups are abundant on the cell membrane and primarily originate from sialic acid (SA), mannose, and galactose residues within glycoproteins and the extracellular matrix. Phenylboronic acid (PBA) derivatives form unique dynamic covalent bonds with vicinal diol groups of the cell membrane and are affected by pH, making them of substantial interest. However, only sialic acids can be efficiently coupled to PBA under physiological conditions, while other diol groups require alkaline reaction conditions with a pH value higher than the pKa of PBA. Therefore, most studies have focused on the reaction between PBA derivatives and SA on the cell membrane surface. Tao and colleagues reported a novel fluorescent polymer containing PBA through the combination of multicomponent reactions (MCRs) with reversible addition–fragmentation chain transfer (RAFT) polymerization.44,45 Specifically, the Hantzsch reaction, a classical four-component reaction, was carried out simultaneously with RAFT polymerization to create innately fluorescent 1,4-dihydropyridine (1,4-DHP). The formed fluorescent polymer is suitable for cell membrane conjugation and imaging through the interaction between phenylboronic acid and sialic acid on the cell membrane. In our laboratory, we utilized a copolymer containing PBA groups to modify silicon nanowire arrays which exhibited a high capture capacity for cells overexpressing SA on the membrane. This modification also allowed for high efficiency of intracellular delivery of diverse biomacromolecules.46 Additionally, overexpression of SA has been demonstrated in various tumors, including lung, melanoma, colon, and breast cancers.47 Consequently, the SA-PBA reaction was commonly employed for tumor cell imaging, targeting, and capturing.37,48–54 Li et al. developed self-assembled nanorods of PBA-functionalized pyrene (Py-PBA NRs), which possess a highly efficient and specific imaging feature of SA on the cell membrane. Three cell lines with different expression levels of SA were utilized to demonstrate this imaging ability. Additionally, the nanorods exhibited efficient generation of 1O2 under two-photon irradiation, providing potential possibilities for tumor therapy (Fig. 3C).37 Furthermore, PBA derivatives with low pKa values have been developed to enhance the applicability of this strategy to cell species characterized by low expression levels of sialic acids. A series of PBAs with different substituents were synthesized, and it was demonstrated that the introduction of electron-withdrawing groups, such as fluoro and nitro, effectively decreased the pKa even to 4.2.55 However, it should be noted that the optimal binding pH may not always exceed the pKa of PBAs, particularly in complex multicomponent systems.56
In addition to PBA derivatives, benzoxaborole (BA), a cyclic hemi-ester of boronic acid, can also be utilized for cell surface modification via the covalent reaction with vicinal diol.57 Morgese et al. have successfully modified supramolecular polymers containing BAs onto the surface of human RBCs via the covalent reaction between BAs and SA.58 The specific interactions between functional copolymers and the cell surface were further visualized in real time using total internal reflection fluorescence microscopy.
2.4 Other functional groups-mediated covalent modification strategy
Carboxyl groups are abundantly present on the cell membrane, mainly distributed at the residues of aspartic acid (Asp) and glutamic acid (Glu) within membrane proteins, as well as at the C-terminus of polypeptide chains. However, the modification of membranes using carboxyl groups necessitates pre-activation of these groups, typically employing an activator known as 3-(ethyliminomethylideneamino)-N,N-dimethylpropan-1-amine (EDC), which causes significant harm to mammalian cell viability. Consequently, the utilization of carboxyl groups for cell modification is often limited. Recently, Ma et al. developed a novel probe (3-phenyl-2H-azirine) that effectively labeled carboxyl groups on the surface of living cells. This presented new possibilities for chemical modification utilizing carboxyl groups present on the cell membrane.59
In addition to utilizing existing groups for direct modification of the cell membrane, strategies have been devised to convert commonly present but difficult-to-modify functional groups into easily modifiable ones through mild oxidation or reduction reactions on the cell surface under gentle conditions. For example, researchers have found that a mild oxidation reaction using NaIO4 can convert diols on cell surfaces into aldehydes, which can be treated as active sites for subsequent cell modification.60–65 Recently, Liu et al. proposed a novel cell surface engineering platform using classical thiazolidine chemistry to combine small molecules containing aminothiol moieties with cells pretreated with aldehyde groups on their surface by NaIO4.64
Efforts have also been devoted to converting disulfide bonds (S–S) on cell membranes to thiol groups and tris(2-carboxyethyl) phosphine (TCEP) is a widely used mild reducing agent in this strategy. Researchers have utilized the strategy to modify synthetic materials including chondroitin sulfate (CS), PEG, mesoporous silica nanoparticles, and silver nanoclusters on the cell membrane for a diverse range of applications.66–70
3. Physical modification
In addition to the chemical covalent binding strategy, physical approaches such as hydrophobic insertion, membrane fusion, electrostatic interaction, and layer-by-layer self-assembly offer versatile and easy ways to introduce synthetic molecules to the cell membrane while maintaining cellular physiology.
3.1 Hydrophobic insertion
The cell membrane skeleton is a phospholipid bilayer structure allowing the spontaneous insertion of synthetic materials with hydrophobic anchors or “tails” driven by the hydrophobic effect.71–73 The commonly used hydrophobic anchors include lipids (phospholipids21,26,73–80 and cholesterols81–92), alkane chains93–98 and oleyl chains.99–105 They can be categorized into single and multiple anchors based on the number of hydrophobic anchors. Shi et al. constructed a polyvalent antibody mimic (PAM) for engineering NK cells with highly efficient targeting, adhesion and killing effects for tumor cells.71 The DNA initiator (DI) with a single anchor was displayed on the NK cell membrane by the hydrophobic insertion approach. Subsequently, a DNA scaffold was synthesized and hybridized with multiple aptamers in situ forming PAM-engineered NK cells. Sun et al. reported a DNA-assisted bottom-up self-assembly approach for achieving precise control over the lateral and vertical distributions of T cell activation ligands on RBCs and constructing RBCs-based artificial antigen presenting cells (aAPCs) which could effectively activate and expand T cells.107 DNA strands with a cholesterol end group were inserted into the membranes by hydrophobic interaction and then bound with T cell activation ligands through specific DNA hybridization as well as biotin–avidin interaction. The vertical distributions of T cell activation ligands can be easily manipulated by adjusting the length of DNA strands, while the lateral distributions were achieved through biotin–avidin interaction. The subsequent study shown in Fig. 4 employed the approach to construct lymphocyte-based aAPCs exhibiting homologous targeting functionality for personalized cancer immunotherapy.106 Zhao et al. developed a surface-anchored framework for sheltering the epitopes on Rhesus D (RhD)-positive RBCs.102 RBCs were modified with horseradish peroxidase containing a single oleyl chain via hydrophobic insertion, thereby catalyzing the reaction of H2O2 to construct a polysialic acid (PSA)-tyramine framework on the RBC membrane. The crosslinking framework successfully achieved transfusion of the modified RBCs to RhD-negative recipients without eliciting immunogenicity, by effectively balancing the modified fluidity of RBC membranes and shielding of RhD antigens.
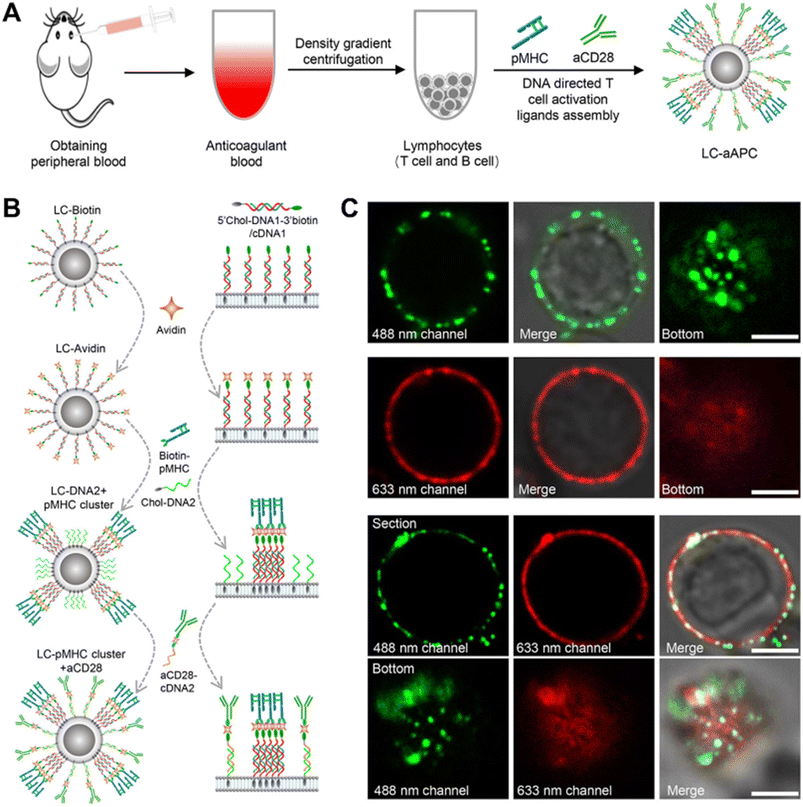 |
| Fig. 4 Construction of LC-aAPCs with lymphocytes from peripheral blood.106 (A) Schematic diagram of constructing lymphocyte-based aAPCs from peripheral blood for personalized tumor immunotherapy. (B) Schematic illustration of DNA-mediated bottom-up assembly of pMHC-I and aCD28 on lymphocytes, including hydrophobic insertion and specific DNA hybridization as well as biotin–avidin interaction. (C) Confocal microscope images showing distribution of pMHC-I and aCD28 on the surface of lymphocytes. Copyright 2022, John Wiley & Sons, Inc. | |
In addition to the single anchor, hydrophobic insertion moieties with more anchors were developed. Niu et al. reported the first effort for cytocompatible controlled radical polymerization (CRP) techniques.72 Chain-transfer agents with a two-tailed hydrophobic anchor, 1,2-distearoyl-sn-glycero-3-phosphoethanolamine (DSEP), were successfully modified on the cell membranes with the hydrophobic insertion strategy, thereby realizing polymerization to be initiated directly in the live cell surface while maintaining high cell viability. The strategy effectively enhanced the efficiency of grafting polymers compared to the traditional grafting-to methods and offered novel possibilities for modulating cellular interactions.
Additionally, the method was also utilized for T cell modification with liposomal nanoparticles, as described in Hao et al.'s investigation.73 The tetrazine (Tre) groups with two-tailed lipids (DSPE) were inserted into T cells and subsequently drug liposomes with bicyclo nonyne (BCN) were modified on the cell membranes of T cells via click reaction while preserving the intact functionality of T cells. A platform (Fig. 5A) for cell membrane engineering with modular polymers was developed by our group.91 The study employed cholesteryl-methacrylate as one of the monomers for constructing modular polymers with multiple anchors through one-pot RAFT copolymerization, along with deoxy-2-(methacrylamido)glucopyranose (MAG) as a hydrophilic monomer and adamantane carbonyl methacrylate (Ada) as a guest monomer. In addition to the introduction of functional molecules through host–guest units, the residence time of the modular polymers could also be regulated on the cell membrane by adjusting the content of cholesterol modules.
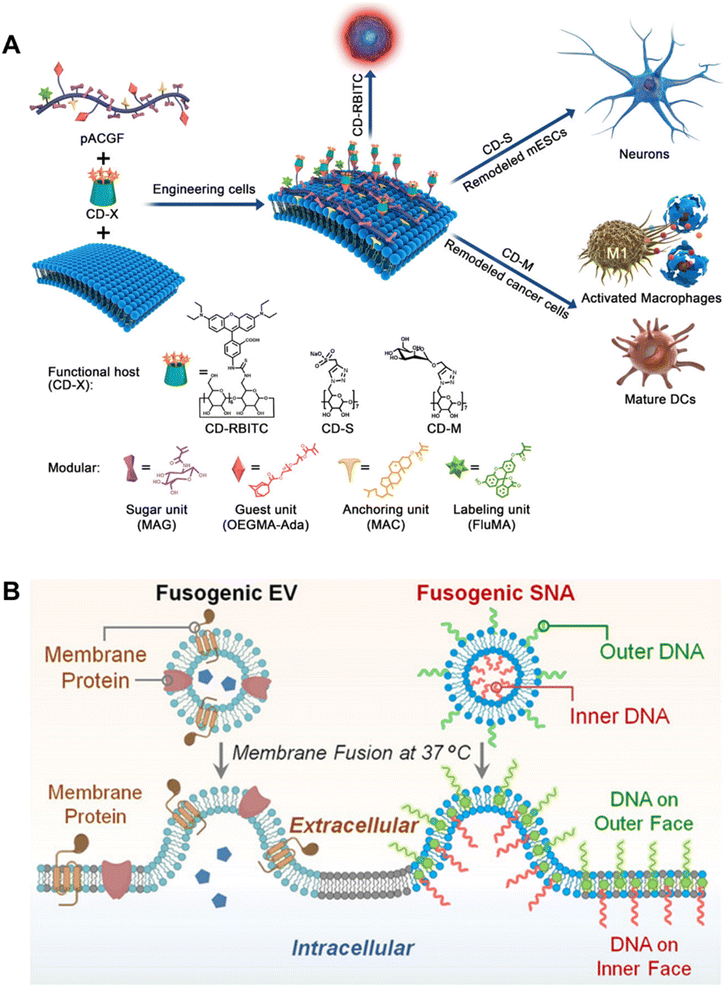 |
| Fig. 5 Representative examples based on hydrophobic insertion and membrane fusion strategies. (A) Schematic illustration of the construction of a platform for the manipulation of cell behaviors, by using hydrophobic insertion to bind modular polymers to the cell surface.91 Copyright 2019, American Chemical Society. (B) Schematic showing the biomimetic LiFT approach to engineer the plasma membrane by membrane fusion.108 Copyright 2022, John Wiley & Sons, Inc. | |
The hydrophobic insertion strategy is considered a simple, powerful, and less invasive approach for cell surface modification. However, functional synthetic materials introduced to the cell membrane surface through hydrophobic insertion are prone to loss during membrane flow and endocytosis, thereby limiting their long-term presence on the cell membrane. The hydrophobic insertion moieties with multiple anchors may offer a promising strategy for achieving relatively stable and long-time modification.
3.2 Membrane fusion
Unlike the strategy of hydrophobic insertion into the cell membrane through hydrophobic anchors, cell surface modification is achieved through liposomes loaded with synthetic materials or functional groups diffusing and mixing with the cell membrane in the membrane fusion strategy.
Sarkar et al. developed a versatile platform technology for the modification of cell membranes.109 Biotinylated lipid vesicles were utilized for the incubation with MSCs, leading to the attachment of biotin on the cell surface via vesicle fusion. The biotin moieties serve as binding sites for subsequent ligands. Yousaf and colleagues conducted a series of studies that utilized the membrane fusion strategy to introduce chemical functional groups onto the cell membrane.110–118 The prepared lipid, containing either ketone or oxyamine molecules, underwent spontaneous insertion and fusion into the cell membrane, resulting in the modification of cells with either ketone or oxyamine molecules for subsequent bio-orthogonal ligation reactions.110 Additionally, the bioorthogonal molecules, ketone or oxyamine, could also be modified onto different populations of cells using the same method to regulate the cell–cell interaction and generate 3D tissue-like structures.111 Following this, the researchers employed a membrane fusion approach to create and modify cell membrane surfaces with bioorthogonal chemical molecules possessing diverse characteristics, including photoresponsive and redox-responsive cleavage. The primary emphasis of their investigation was on the utilization of these modified cells in the field of three-dimensional tissue engineering. Membrane fusion strategies have recently been extensively used as a potent tool for modifying cell membranes in various investigations.
Zheng et al. designed core–shell membrane-fusing liposome (MFL) containing NK cell-activating glycans, Lewis X trisaccharide (LeX), and loaded it into a thermosensitive hydrogel which could be released responsively through the tumor microenvironment. Subsequently, the released MFL was fused with tumor cell membranes, realizing the modification of tumor membranes with Lex which could enhance the anti-tumor effects.119 Shi et al. designed T-cell-targeting fusogenic liposomes by conjugating ROS-scavenging groups, 2,2,6,6-tetramethylpiperidine (TEMP) and T-cell-targeting anti-CD3 F(ab′)2 fragments to the surface of liposomes, in which TEMP groups were designed for neutralizing ROS and protecting T cells from an oxidation-induced loss of activity. In the meantime, the procedure would result in paramagnetic transition of TEMP to TEMPO molecules, allowing for the measurement of the in situ activity of T cells, enabling a better understanding of engineering T cells for cancer treatment.120 Lin et al. reported a liposomal fusion-based transport (LiFT) strategy to anchor functional DNA strands on the inner face of the cell membrane, addressing the previous lack of suitable synthetic tools to engineer the intracellular interior (Fig. 5B).108 In subsequent studies, the group combined membrane-anchored catalysts with the previously reported LiFT strategy, through which they were able to prepare the corresponding fusion liposome catalyst through a simple strategy, achieving precise position control of the catalyst on the cell membrane.121 The drug molecules generated by this method may have higher drug delivery efficiency than traditional methods using drug delivery vehicles. Furthermore, by integrating targeting motifs into the outer surface of liposomes, cell-specific membrane engineering can be achieved for potential targeted drug delivery.
3.3 Electrostatic interaction
Cell surface modification through electrostatic interactions is an appealing strategy that capitalizes on the negative charge conferred mainly by sialic acid residues in the carbohydrate layer, along with the phosphatidylserine on the plasma membrane. Cationic polymers such as polyethyleneimine (PEI), poly-L-lysine (PLL) and chitosan (CS) are often utilized in the strategy.122–126 For instance, Choi and colleagues achieved the development of silica coating on mammalian cells by modifying PEI on the cell membrane through electrostatic interactions, serving as a catalytic template for silicification.126 In a subsequent study, TiO2 shells were developed for the cytoprotective encapsulation of Jurkat T cells.122 This method could effectively protect the T cells in the shell while simultaneously preserving their functionality, including cell division, juxtacrine interactions and cytokine secretion. Upon administration into the organism, the lymphocytes' therapeutic capabilities are effectively reinstated through the rupture of the protective shell. The TiO2-inducing peptide, (RKK)4D8 (R: arginine, K: lysine, D: aspartic acid), was deposited on the surface of Jurkat cells via electrostatic interactions to facilitate the formation of bioinspired TiO2 using titanium bis(ammonium lactato)dihydroxide (TiBALDH) as a precursor. However, interactions with most cationic polymers readily lead to the destruction of the cell membrane, resulting in pronounced cytotoxicity and cellular damage. To address this issue, cationic polymers can be modified with biocompatible molecules, such as grafting PEG or alginate, to mitigate the detrimental effects on cell viability.127
Despite the overall negative charge of the cell membrane surface, a few cationic sites on the plasmalemma still exist which can be modified with negatively charged materials.130,131 For instance, Thomsen et al. modified T cells with negatively charged degradable poly(lactic acid) (PLA) nanoparticles with electrostatic adsorption.125 Furthermore, the modification of negatively charged materials can be achieved through a synergistic combination of electrostatic interactions, hydrogen bonding and hydrophobic interactions.132–134 It is important to note that the presence of a negatively charged cell membrane hinders the uptake of negatively charged nanoparticles by cells.
3.4 Layer-by-layer (LBL) self-assembly
LBL self-assembly strategies have been developed on the basis of electrostatic interaction and widely employed for the construction of cell coatings, in which oppositely charged materials are sequentially deposited onto the cell membrane through electrostatic interaction along with hydrogen bonding, van der Waals forces, etc.21,127,128,135–147 As shown in Fig. 6A, Hong and colleagues developed LBL self-assembled nanofilms for cell surface modification of viable MSCs. Positively charged PLL was layer-by-layer assembled with negatively charged hyaluronic acid (HA) and arginine-glycine-aspartic acid (RGD) to fabricate nanofilms, which not only provided biochemical signals but also offered mechanical support for MSCs without interfering with the stemness of MSCs.128 Subsequent studies have demonstrated the successful construction of nanofilms on the surface of human induced pluripotent stem cells (iPSCs) and immune cells such as AML-12 cells and peripheral blood mononuclear cells (PBMCs) via the LBL self-assembly strategy.140,148 Gels can be formed via LBL to coat or encapsulate cells. Chen and colleagues proposed a gentle approach (Fig. 6B) to achieve the nanoencapsulation of individual mammalian cells.129 The gelatin coatings, which mimic the extracellular matrix (ECM), are formed through LBL self-assembly between positively charged gelatin type A (GA) and negatively charged gelatin type B (GB) on the cell membrane surface. Additionally, the outer layer of PEG was further constructed using thiol-maleimide click chemistry which could be degraded on-demand by the addition of the reducing agent glutathione (GSH). Subsequent studies involved the development of an enzyme-responsive nano-coating for encapsulating individual living cells, which was prepared through layer-by-layer self-assembly of oppositely charged gelatin-poly(ethylene glycol)maleimide and the incorporation of cysteine-terminated peptide sequences (CGGPLGLAGGC) via click reaction.135 Moreover, the peptide chain could undergo enzymolysis upon exposure to high concentrations of matrix metalloproteinase-7 (MMP-7), which is frequently overexpressed in tumors, leading to the release of encapsulated cells.
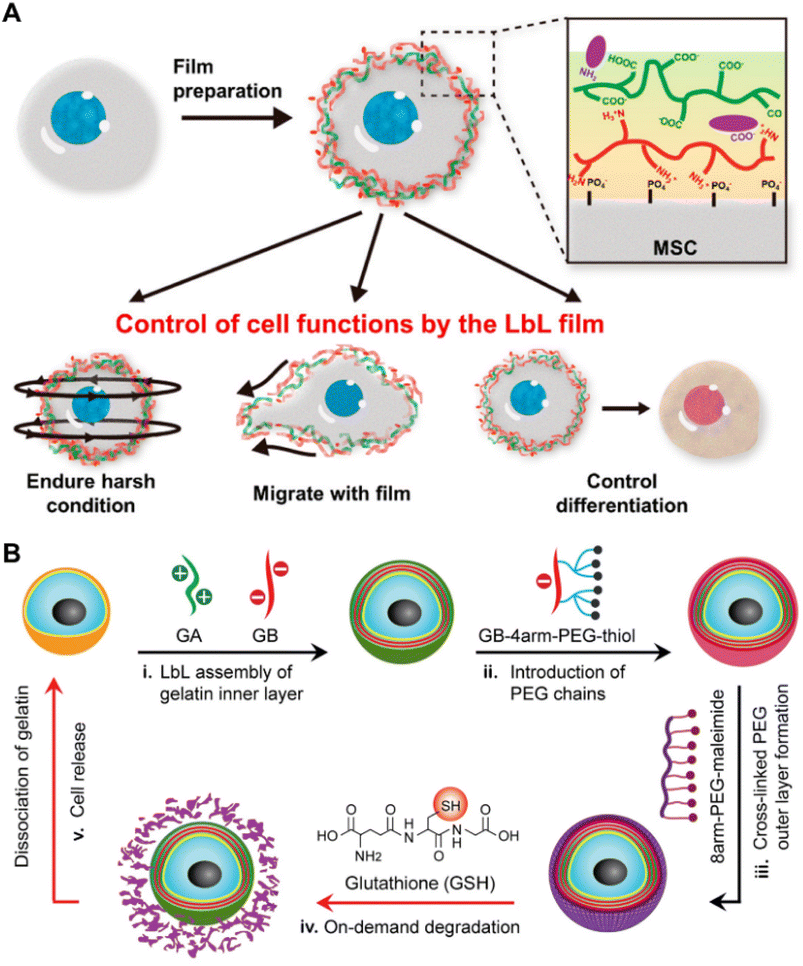 |
| Fig. 6 Methods based on layer-by-layer self-assembly. (A) Schematic illustration of MSCs nanofilms prepared using positively charged PLL, negatively charged HA and RGD, and the functions exhibited by the modified cells.128 Copyright 2017, American Chemical Society. (B) Schematic illustration of mammalian cell nanoencapsulation conducted by LBL self-assembly between GA and GB, and subsequent thiol-maleimide reaction. GSH could be added for on-demand release.129 Copyright 2017, Elsevier. | |
4. Bioorthogonal modification
Despite the abundance of functional groups on the surface of the cell membrane that are amenable to chemical covalent modification, the utilization of non-specific covalent modification strategies may have detrimental effects on the viability and functionality of normal cells. Additionally, physical strategies are limited by the short residence time of synthetic molecules. In contrast, bioorthogonal chemistry offers a highly efficient and selective approach that takes place within a mild physiological environment, without disrupting intrinsic biochemical processes. This strategy represents a substantial advancement in terms of both cell viability and the stability of modifications. Here, we provide an overview of recent developments in the integration of metabolism with copper-free click chemistry, Halo-Tag proteins, and enzyme-mediated approaches.
4.1 Metabolic glycan labeling strategy
Since the pioneering work of Bertozzi and colleagues, who introduced exogenous glycans into the cell membrane glycocalyx, there has been a gradual development of strategies for metabolic glycan labeling to modify the cell membrane.149,150 In this strategy, unnatural sugars containing functional groups such as azide, alkyne, thiol and alkene are internalized by cells and the chemically reactive functional groups are “installed” on the glycan residues on the cell membrane by metabolic pathways.150–156 In recent years, glycans containing azide groups represented by N-azidoacetylmannosamine-tetraacetate (Ac4ManNAz) have gained the most widespread adoption with the development of copper-free “click” azide–alkyne reactions due to their high selectivity, synthetic simplicity and commercial availability. Tomas and colleagues have conducted a series of investigations on the cell surface modification with polymers via the metabolic glycan labeling strategy.157–159 In a recent study, Tomas et al. who proposed the “engineering cells to capture polymers” strategy incubated tumor cells with Ac4ManNAz for 96 hours to obtain azido-modified cancer cells which could capture chemotherapeutic polymers covalently and this strategy significantly augmented the concentration specifically targeted towards the tumor cell membrane whilst optimizing therapeutic efficacy by reducing systemic toxicity and enhancing selectivity.158 In addition to polymers, the metabolic glycan labeling method effectively facilitates the modification of nanoparticles on the live cell membrane which is an attractive strategy for drug delivery.160–162 Zhou et al. successfully modified an oligomeric proanthocyanidin loaded liposome on the membrane of MSCs (MSC-Lipo-OPC) via metabolic labeling combined with the click chemistry strategy (Fig. 7A).160 The MSC-Lipo-OPC could control the progression of inflammation due to the excellent abilities to scavenge free radicals and effectively prevent the formation of radiation-induced pulmonary fibrosis. Chen et al. developed polyvalent spherical aptamer (PSA) engineered macrophages which could effectively recognize tumor cells and inhibit tumor growth.161 PSA which has superior affinity and specificity to tumor cells was constructed through covalent reaction of gold nanoparticles (AuNPs) with AS1411 aptamer and DBCO groups, and was subsequently modified on macrophage membranes via metabolic labeling. Moreover, Lamoot et al. developed a 2-step click strategy for achieving highly specific cell surface conjugation of nanoparticles. In the study, cells were incubated with N-azidoacetylmannosamine-tetraacetylated (Ac4ManN3) to present azido groups on cell membrane (Fig. 7B).162 Subsequently, sulfo-6-methyl-tetrazine-dibenzyl cyclooctyne (Tz-DBCO) was exploited as a “bridge” between azide-modified cells and trans-cyclooctene (TCO) functionalized nanoparticles to realize nanoparticle-engineered cells exhibiting extremely low non-specific background binding.
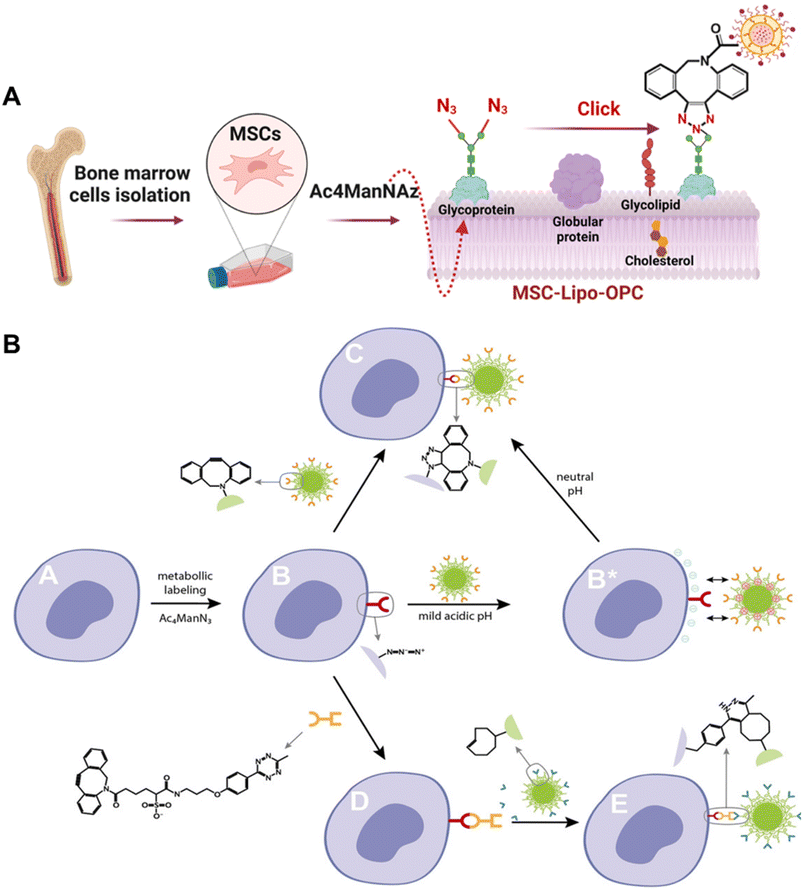 |
| Fig. 7 Methods based on the metabolic glycan labeling strategy. (A) Schematic illustration of binding Lipo-OPC to MSCs which were pre-incubated with Ac4ManNAz and presented azido groups on the cell membrane.160 Copyright 2023, Elsevier. (B) Overview of the 2-step click strategy for achieving highly specific cell surface conjugation of nanoparticles.162 Copyright 2020, John Wiley & Sons, Inc. | |
In addition to in vitro applications for modifying cell membranes, researchers have also conducted studies to achieve this process in vivo.163,164 Wang et al. conducted an interesting study by labeling and modulating DCs and regulating DC–T cell interactions in vivo.163 They synthesized Ac4ManAz nanoparticles overcame the limitations of Ac4ManAz utilized in vivo such as poor encapsulation and water solubility. The Ac4ManAz nanoparticles and granulocyte-macrophage colony-stimulating factor (GM-CSF) were loaded into an injectable alginate gel for the purpose of realizing in situ recruitment and azide labeling of dendritic cells. Dibenzocyclooctyne (DBCO)-labelled immunomodulatory agents, such as tumor antigens, adjuvants, and cytokines could be modified on the DC membrane via click chemistry in vivo thereby effectively enhancing the subsequent T cell activation and tumor killing process. Additionally, Tu et al. employed Ac4ManAz nanoparticles for in situ labeling of tumor cell membranes with azido groups, followed by the binding of chlorin e6 (Ce6), a commonly used photosensitizer, via click chemistry.164 This approach effectively enhanced the therapeutic efficiency of photodynamic therapy. Recently, Chen and colleagues reported a cell-type-specific labeling approach in vivo.165 In this study, the cardiomyocyte was specifically labeled without any interference from other cardiac cell types, which provided a powerful tool for cell-type selective modification. Gong et al. accomplished in situ PEGylation of CAR-T cells through the utilization of the metabolic glycan labeling strategy.166 When the molecular weight of PEG reached 600000, it effectively hindered the intercellular interactions among CAR-T cells, tumor cells, and monocytes, thereby attenuating the secretion of cytotoxic cytokines and ameliorating the symptoms associated with cytokine release syndrome (CRS).
Compared to the direct covalent binding with functional groups on the cell membrane, the metabolic glycan labeling strategy effectively enhances the density of reactive sites on cells, but it is a time-consuming process that can take several days. The significant advantage of metabolic glycan labeling combined with bioorthogonal reactions is that it transits cell surface modification from nonspecific to specific, enabling cell surface modification in situ and in vivo. This is still an emerging field, with immense potential for further development and expansion.
4.2 Halo-Tag protein
Halo-Tag protein (HTP) is an engineered protein derived from the bacterial haloalkane dehalogenase, which selectively reacts with alkanes containing a terminal chloride group (chloroalkanes) forming a covalent bond.167,168 Similar protein recognition tags, such as SNAP tags169 and ACP tags,170 could also be utilized in cell surface modification, but they will not be extensively discussed in this section. A two-step approach was utilized in the HTP strategy: the expression of HTP on the cellular membrane is achieved via genetic engineering methods and further combined with cargoes containing the chloroalkane. HTP is commonly used in protein isolation and purification, molecular imaging, molecular interactions etc. in most reported studies and was first utilized for cell surface modification by Pulsipher et al.171 They proposed a long-lived cell membrane engineering strategy utilizing HTP as an anchor for modifying embryonic stem cells (ESCs) with heparan sulfate (HS), which was covalently modified on the ESC membrane and stayed for more than one week.
Subsequently, our group developed a series of studies via the HTP strategy.172,175,176 Tumor cells were modified with specific glycopolymers via the HTP fusion technique combined with RAFT polymerization (Fig. 8A). The glycopolymers that were modified on tumor cells could bind to lectins on dendritic cells or macrophages which effectively enhanced the tumor immune response.172 An interesting discovery was that the migration of the tumor cells modified with glycopolymers could be affected. Specifically, compared with the unmodified tumor cells, the migration direction was altered and diffusion slowed down which offered novel insights pertaining to the management of cancer metastasis.175 A following study was carried out and we constructed glycopolymers modified DCs via the HTP strategy. Enhanced interactions were discovered between glycopolymer modified DCs and T cells which effectively promoted the T cell activation and proliferation, providing a novel approach to designing more efficient DC vaccines.176
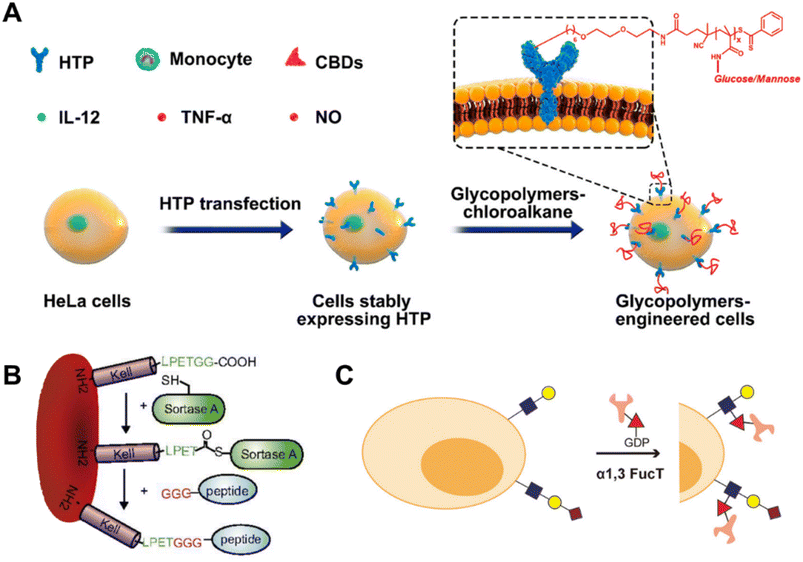 |
| Fig. 8 Methods based on other bioorthogonal strategies. (A) Schematic illustration of displaying synthetic glycopolymers on HeLa cell membranes using HTP anchors.172 Copyright 2019, American Chemical Society. (B) Schematic illustration of Kell C-terminal sortase labeling with GGG-carrying antigen peptides.173 Copyright 2017, National Academy of Sciences. (C) Schematic illustration of transferring biomacromolecules to glycocalyx on the surface of living cells with fucosyltransferase.174 Copyright 2018, American Chemical Society. | |
The HTP strategy for cell surface modification is still in its infancy. It is noteworthy due to the strong stability of binding between HTP expressed on the cell membrane and its corresponding ligand, thereby enabling sustained modifications that persist for over a week, offering a suitable method for long-time and stable cell surface modification. However, the implementation of HTP expression necessitates the manipulation of gene transfection, a process that is intricate and time-consuming, thereby inapplicable to certain challenging-to-transfect cell types such as primary cells.
4.3 Enzyme-mediated strategy
Enzyme-mediated modification of cell membranes represents a novel approach for in situ modification of candidate materials, reacting with pre-existing structures on the cell surface under the specific catalysis of enzymes. Specifically, certain enzymes such as oxidoreductases (galactose oxidases177), glycosyltransferases (sialyltransferases,178,179 galactosyltransferases, N-acetyl-glucosaminyl transferases and fucosyltransferases174,180), transpeptidases (butelases and sortases173,181,182), transglutaminases (TGases183) etc. have been utilized for the modification of cell membranes, representing an appealing approach due to their remarkable specificity and high yield. For example, galactose oxidases can specifically convert the endogenous terminal galactoses or N-acetylgalactosamine residues on the cell surface into aldehyde groups, facilitating subsequent reactions between aldehyde groups and aminooxy-functional molecules.177 Glycosyltransferases are primarily utilized for the modification of pre-existing sugars on cell membranes, thereby facilitating the introduction of non-natural sugars. Moreover, it's worth noting that in comparison to metabolic engineering approaches, glycosyltransferases, particularly sialyltransferases and fucosyltransferases, offer a novel method for introducing greater kinds and intricacy of sugars on the cell membrane surface. In the presence of transpeptidases, molecules bearing recognition motifs can be directly conjugated to either the N or C termini of membrane proteins. For example, as shown in Fig. 8B, Pishesha et al. reported a strategy for inducing antigen-specific tolerance by utilizing the transpeptidase sortase to covalently conjugate disease-associated autoantigens onto red blood cells (RBCs), thereby attenuating the contribution of major subsets of immune effector cells to immunity in an antigen-specific manner.173 Li et al. focused on fucosyltransferase and transferred bio-macromolecules to the glycocalyx on the surface of living cells, which represented faster speed, better biocompatibility, and less interference to cells (Fig. 8C).174 Through this method, they constructed two antibody–cell conjugates, which exhibited significant improvements in the process of targeting and killing anti-cancer immune responses.
5. Conclusion and outlook
Modifying cell surfaces with tailor-made and well-characterized synthesized molecules can effectively introduce novel functionalities or manipulate cells. This offers a powerful tool to overcome challenges encountered in cell-based biomedical applications. In this review, we present a comprehensive overview of the latest advances in cell surface modification using synthetic molecules. We summarize the typical strategies, including chemical covalent modifications, physical alterations, and bioorthogonal approaches (Table 1), along with the advantages, disadvantages, and applicable conditions of each strategy. The chemical covalent strategy offers a straightforward and versatile approach for achieving stable and long-lasting surface modification.184 However, the strategy has the potential to adversely impact cell activity and functionality. The physical modification strategy provides a non-invasive and cytocompatible approach. However, modifications achieved through physical interactions, such as electricity and hydrophobicity, are relatively short-term and unstable. It is important to note that the two methods mentioned above are non-specific, lacking precision in cell surface modification and potentially increasing the risk of adverse effects during practical applications. Therefore, bioorthogonal chemistry provides a valuable strategy for the selective and highly biocompatible incorporation of synthetic molecules onto cell surfaces, even enabling cell surface modification in vivo – a remarkable development. However, the approaches used to introduce bioorthogonal groups, whether via genetic engineering or metabolic engineering, are time-consuming.
Table 1 A summary of the advancements in strategies for the cell surface modification with synthetic molecules in this review
|
Strategy |
Cell typea |
Synthetic molecules modified on the cell surface |
Abbreviation for the full cell name or cell species: HUVECs: human umbilical vein endothelial cells; C2C12 cells: murine myoblasts; MSCs: mesenchymal stem cells; RBCs: red blood cells; Jurkat cells: acute T-cell leukemia cells; NK cells: natural killer cells; DCs: dendritic cells; B16 cells: murine melanoma cells; L929 cells: mouse fibroblast cells; MCF-7 cells: human breast adenocarcinoma cells; HepG2 cells: human liver cancer cells; HeLa cells: human cervical cancer cells; HDFs: human dermal fibroblasts; AB22 cells: mouse mesothelioma cells; ZL34 cells: human mesothelioma cells; PC-3 and DU145 cells: human prostate cancer cells; iPSCs: induced pluripotent stem cells; HT-29: human colon cancer cells; MDA-MB-231 cells: human breast cancer cells; NEs: neutrophils; HSFs: human skin fibroblasts; CCRF-CEM cells: human acute lymphoblastic leukemia cells; RAW 264.7 cells: mouse leukemia cells of monocyte macrophages; L-O2 cells: human normal liver cells; HEK293T cells: human embryonic kidney cells; A549 cell: human lung cancer cells; LUDLU-1 cells: human Caucasian lung squamous carcinoma cells; SubT1 cells: human CD4 expressing T-lymphoblastoid cells; U937: histiocytic lymphoma cells; Th9 cells: helper T cell 9; 4T1 cells: mouse breast cancer cells; 3T3 cells: mouse embryonic fibroblasts; RIN cells: mouse insulinoma cells; hASCs: human adipose-derived mesenchymal stem cells; DPCs: dermal papilla cells; MIN6 cells: pancreatic β-cells; AML-12 cells: human lung cancer cells; PBMCs: peripheral blood mononuclear cells; CHO cells: Chinese hamster ovary cells.
|
Chemical covalent modification strategies |
Amine groups (–NH2) |
HUVECs |
PEG,21 biotin–streptavidin22 |
C2C12 cells |
Poly(methacryloyloxy)ethyltrimethylammonium chloride (PMETAC)22 |
MSCs |
Heterodimerizing leucine,23 chitosan nanoparticles,24 sialyl Lewis X29 |
RBCs |
Biotin–streptavidin,26 PEG30,31 |
T cells |
Nanoparticles32 |
Jurkat and NK cells |
Biotin–streptavidin27 |
Thiol groups (–SH) |
RBCs |
Nanoparticles36 |
DCs |
Bovine serum albumin (BSA) protein shells41 |
B16 cells |
BSA protein shells41,43 |
T cells |
Nanoparticles33,34,38,39 |
Vicinal diol groups |
L929 cells |
Fluorescent polymer44 |
MCF-7, HepG2 and HeLa cells |
Pyrene derivative37 |
B16 cells |
Gadolinium DO3A amide48 |
HDFs |
DEGMA or NVP polymers49 |
HepG2 cells |
BSA nanoparticles50 |
B16, AB22 and ZL34 cells |
Contrast agents (Gd- and 68Ga-DOTA-EN)52 |
PC-3, DU145 and Jurkat cells |
Sialic acid-imprinted fluorescent core–shell SiO2 particles53 |
MCF-7, HeLa and PC-3 cells |
Polymer nanoparticles54 |
RBCs |
Supramolecular polymers58 |
Carboxyl groups |
MCF-7 cells |
Reactive probe59 |
Convert diols into aldehydes |
HDFs |
Biotin–streptavidin60 |
HT29 and MDA-MB-231 cells |
Maltol hydrazide61 |
HepG2 cells |
Dendrimer hydrazides62 |
PEI conjugated with multiple hydrazide groups63 |
MCF-7 cells |
Peptide and protein64 |
HeLa cells |
p-Benzoquinone/ethylenediamine polymer65 |
Convert disulfide bonds (S–S) to thiol groups |
Human iPSC-derived-MSCs |
ECM coating66 |
HepG2 cells |
DNA bridge complex-templated silver nanoclusters (DNA bridge-AgNCs)67 |
HeLa cells |
Fluorescent dye, polymer, and nanoparticles69 |
NEs |
Liposomal stimulator of interferon genes (STING) agonists70 |
Physical modification strategies |
Hydrophobic insertion |
HUVECs and HSFs |
PEG21 |
T cells |
Tetrazine73 |
DCs and PC-3 cells |
Cucurbit[7]uril-based supramolecular polymer74 |
CCRF-CEM cells, splenocytes, melanoma, human MSCs and beta cells |
Synthetic peptide76 |
RAW264.7 cells and L-O2 cells |
β-Cyclodextrin (β-CD) and adamantane (ADA)77 |
Hydrophobic insertion |
Human T cells and B cells, erythrocytes, hepatocytes, L929 and HEK293T cells |
Cell-penetrating peptide79 |
Hydrophobic insertion |
MCF7, A549, LUDLU-1 cells |
Aptamer80 |
HeLa cells |
DNAzyme,82 DNA,89 multicomponent polymer,91 aptamer,80 core–shell upconversion nanoparticles92 |
MCF-7 cells |
Fluorescein isothiocyanate (FITC),83 core–shell upconversion nanoparticles92 |
SubT1 cells |
Biotin–PEG84 |
MDA-MB-231 cells |
Nitrilotriacetic acid (NTA)85 |
NK cells,81 Jurkat, NK, and Ramos cells,86 CCRF-CEM cells,87 U937 cells,88 RBCs,107 lymphocytes106 |
DNA |
MSCs |
Hyperbranched polyglycerol (HPG) covalently modified with vasculature binding peptides (VBPs)93 |
Th9 cells, 4T1 cells |
Peptides94,95 |
Jurkat cells |
ssDNA97 |
3T3 cells |
Poly(oxanorbornene) block copolymers,98 PEG104 |
HepG2 cells |
Peptides,99 PEG100 |
RIN cells |
PEG101 |
Fibroblast cells |
Ketone and oxyamine,110,116,118 dialdehyde,112 hydroquinone117 |
NK cells |
Lewis X trisaccharide119 |
T cells |
2,2,6,6-Tetramethylpiperidine groups120 |
HeLa cells |
DNA108 |
Electrostatic interaction |
HUVEC |
Poly-L-lysine (PLL)21 |
Jurkat cells |
TiO2 coating122 |
hASCs |
Chitosan derivative124 |
HeLa, 3T3, and Jurkat cells |
Poly(ethyleneimine) (PEI)126 |
T cells |
PLA nanoparticles125 |
Layer-by-layer (LBL) self-assembly |
HeLa cells |
Cationic gelatin and anionic gelatin129,135 |
MSCs |
PLL, hyaluronic acid (HA), and arginine-glycine-aspartic acid (RGD),128 gelatin,135 silk fibroin polyelectrolyte139 |
RBCs |
Chitosan-graft-phosphorylcholine, HA and PLL-PEG136 |
DPCs |
Gelatin and alginate137 |
MIN6 cells |
Gelatin and fibronectin (FN)138 |
L929 cells |
Silk fibroin polyelectrolyte139 |
AML-12 cells and PBMCs |
PLL and HA140 |
T cells |
Chitosan and alginate147 |
iPSCs |
FN, heparin (Hep), and chondroitin sulfate (CS)148 |
Bioorthogonal strategies |
Metabolic glycan labeling strategy |
Jurkat cells |
Probes,151 lipid nanoparticles,162 polymer–antibody conjugates156 |
HEK 293T cells |
Probes154 |
MCF-7 cells |
β-CD and aptamer155 |
Metabolic glycan labeling strategy |
A549 cells |
Poly(hydroxyethyl acrylamide),157 polycation,158 poly(hydroxyethyl acrylamide) (pHEA)159 |
MCF-7 cells |
Polycation158 |
MSCs |
Antioxidant liposome160 |
Macrophages |
Polyvalent spherical aptamer161 |
DCs |
Antigens, adjuvants and cytokines163 |
4T1 cells |
Chlorin 6 (ref. 164) |
HeLa cells |
Probes183 |
Halo-Tag protein |
B16 cells,175 DCs,176 Hela cells172 |
Glycopolymers |
Enzyme-mediated strategy |
B cells |
Biotin177 |
NK cells |
Bio-macromolecules,174 Sialyl Lewis X and CD22-specific ligands185 |
T cells |
Bio-macromolecules174 |
Mouse lung endothelial cells |
Synthetic ligands and biotin178 |
CHO cells |
Probes,181 bio-macromolecules174 |
RBCs |
Probes,182 peptide173 |
HEK 293T cells |
Probes181 |
HeLa cells |
Probes183 |
Despite notable advancements in the utilization of synthetic compounds for cell surface modification, there remain unresolved challenges and prospects for further investigation. One such challenge pertains to the inherent detrimental impact of exogenous synthetic compounds bound to the cell surface on cellular functionality, albeit with varying degrees of severity. Hence, it is of utmost importance to meticulously choose a suitable strategy for modifying cells, taking into consideration the particular cell type and application scenarios. Subsequently, it becomes imperative to assess and describe the condition of the modified cells, encompassing cell viability, phenotype, and associated functionalities. It is noteworthy that the cell surface constitutes a dynamic membrane structure, wherein synthetic molecules may undergo endocytosis or excretion by the cell. Consequently, it is crucial to monitor the destiny of synthesized molecules during and post cell surface modification. In practical scenarios, the presence of synthetic molecules on cellular surfaces carries the potential for immune activation and subsequent clearance by the immune system, thereby considerably restricting their capacity to modify cell surfaces in vivo. Consequently, it becomes crucial to implement suitable adjustments to synthetic molecules to ensure their compatibility with in vivo applications. Additionally, a significant hurdle lies in selecting and designing molecules that possess both biocompatibility and augmented functionality for specific applications. Determining the optimal chemical group, structure, and sequence becomes essential in this regard. Therefore, the availability of databases serving as a toolbox for researchers to facilitate informed molecule selection is highly desirable.
There are several possible avenues for future research. One potential area of exploration is the development of more precise and targeted methods for modifying cell surfaces. There is a need to develop more proficient and potent methodologies for the selective and highly biocompatible integration of synthetic molecules onto cell surfaces. Furthermore, additional research is necessary to gain a better understanding of the influence of synthetic molecules on cellular functionality and to optimize modification strategies tailored to specific cell types and applications. An additional area of research that holds promise for the future is the advancement of synthetic molecules that possess improved biocompatibility and biofunctionality, enabling their application in the modification of cell surfaces. Notable examples of these molecules encompass functional nucleic acids, targeting aptamers, and polymers characterized by well-defined structures and chain sequences. The utilization of artificial intelligence (AI) can be facilitated by the establishment of databases containing comprehensive information regarding ligand–receptor interactions specific to cells, as well as the attributes associated with each modification technique. This integration of AI can aid in the design of optimal, customized molecules and the selection of appropriate methods for modification.
Author contributions
Conceptualization: H. Chen, G. Chen and H. Yang; writing the original draft: H. Yang, L. Yao and Y. Wang; validation: H. Yang, L. Yao and Y. Wang; review and editing: H. Chen and G. Chen.
Conflicts of interest
There are no conflicts to declare.
Acknowledgements
The study was supported by the National Natural Science Foundation of China (No. 21935008 and 22171203) and the Priority Academic Program Development of Jiangsu Higher Education Institutions (PAPD). The authors also thank the website https://app.biorender.com/ for the assistance in creating the illustration figures.
References
- L. Tang, Y. Zheng, M. B. Melo, L. Mabardi, A. P. Castaño, Y.-Q. Xie, N. Li, S. B. Kudchodkar, H. C. Wong, E. K. Jeng, M. V. Maus and D. J. Irvine, Nat. Biotechnol., 2018, 36, 707–716 CrossRef CAS PubMed
.
- C. W. Shields, M. A. Evans, L. L.-W. Wang, N. Baugh, S. Iyer, D. Wu, Z. Zhao, A. Pusuluri, A. Ukidve, D. C. Pan and S. Mitragotri, Sci. Adv., 2020, 6, eaaz6579 CrossRef CAS PubMed
.
- G. Q. Daley, Cell Stem Cell, 2012, 10, 740–749 CrossRef CAS PubMed
.
- M. A. Fischbach, J. A. Bluestone and W. A. Lim, Sci. Transl. Med., 2013, 5, 179ps177 Search PubMed
.
- H.-R. Jia, Y.-X. Zhu, Q.-Y. Duan and F.-G. Wu, Chem. Soc. Rev., 2021, 50, 6240–6277 RSC
.
- L. Zheng, X. Hu, H. Wu, L. Mo, S. Xie, J. Li, C. Peng, S. Xu, L. Qiu and W. Tan, J. Am. Chem. Soc., 2020, 142, 382–391 CrossRef CAS PubMed
.
- Z. Wu, B. Esteban-Fernández de Ávila, A. Martín, C. Christianson, W. Gao, S. K. Thamphiwatana, A. Escarpa, Q. He, L. Zhang and J. Wang, Nanoscale, 2015, 7, 13680–13686 RSC
.
- D. Dapkute, M. Pleckaitis, D. Bulotiene, D. Daunoravicius, R. Rotomskis and V. Karabanovas, ACS Appl. Mater. Interfaces, 2021, 13, 43937–43951 CrossRef CAS PubMed
.
- M. H. Kershaw, J. A. Westwood and P. K. Darcy, Nat. Rev. Cancer, 2013, 13, 525–541 CrossRef CAS PubMed
.
- D. Zhang, Y. Zheng, Z. Lin, X. Liu, J. Li, H. Yang and W. Tan, Angew. Chem., Int. Ed. Engl., 2020, 59, 12022–12028 CrossRef CAS PubMed
.
- H. Watson, Essays Biochem., 2015, 59, 43–69 CrossRef PubMed
.
- K. Adebowale, R. Liao, V. C. Suja, N. Kapate, A. Lu, Y. Gao and S. Mitragotri, Adv. Mater., 2023, 2210059 CrossRef PubMed
.
- S. Yang, H. Choi, D. T. Nguyen, N. Kim, S. Y. Rhee, S. Y. Han, H. Lee and I. S. Choi, Adv. Ther., 2023, 6, 2300037 CrossRef CAS
.
- S. Mansouri, Y. Merhi, F. M. Winnik and M. Tabrizian, Biomacromolecules, 2011, 12, 585–592 CrossRef CAS PubMed
.
- N. A. A. Rossi, I. Constantinescu, R. K. Kainthan, D. E. Brooks, M. D. Scott and J. N. Kizhakkedathu, Biomaterials, 2010, 31, 4167–4178 CrossRef CAS PubMed
.
- M. C. Arno, Macromol. Rapid Commun., 2020, 41, e2000302 CrossRef PubMed
.
- D. Wu, K. Yang, Z. Zhang, Y. Feng, L. Rao, X. Chen and G. Yu, Chem. Soc. Rev., 2022, 51, 1336–1376 RSC
.
- S. Roy, J. N. Cha and A. P. Goodwin, Bioconjugate Chem., 2020, 31, 2465–2475 CrossRef CAS PubMed
.
- B. Kellam, P. A. De Bank and K. M. Shakesheff, Chem. Soc. Rev., 2003, 32, 327–337 RSC
.
- J. Park, B. Andrade, Y. Seo, M. J. Kim, S. C. Zimmerman and H. Kong, Chem. Rev., 2018, 118, 1664–1690 CrossRef CAS PubMed
.
- F. Cai, Y. Ren, J. Dai, J. Yang and X. Shi, Macromol. Biosci., 2023, 23, e2200379 CrossRef PubMed
.
- W. Wang, Q. Gan, Y. Zhang, X. Lu, H. Wang, Y. Zhang, H. Hu, L. Chen, L. Shi, S. Wang and Z. Zheng, Adv. Mater., 2021, 33, e2102348 CrossRef PubMed
.
- N. E. Jasiewicz, K. C. Mei, H. M. Oh, P. Chansoria, D. A. Hendy, E. E. Bonacquisti, E. M. Bachelder, K. M. Ainslie, H. Yin, L. Qian, B. C. Jensen and J. Nguyen, Adv. Healthcare Mater., 2023, 12, e2201094 CrossRef PubMed
.
- M. Xu, S. Asghar, S. Dai, Y. Wang, S. Feng, L. Jin, F. Shao and Y. Xiao, Int. J. Biol. Macromol., 2019, 134, 1002–1012 CrossRef CAS PubMed
.
- C. M. Csizmar, J. R. Petersburg and C. R. Wagner, Cell Chem. Biol., 2018, 25, 931–940 CrossRef CAS PubMed
.
- X. Sun, X. Han, L. Xu, M. Gao, J. Xu, R. Yang and Z. Liu, Small, 2017, 13, 1701864 CrossRef PubMed
.
- B. Wang, J. Song, H. Yuan, C. Nie, F. Lv, L. Liu and S. Wang, Adv. Mater., 2014, 26, 2371–2375 CrossRef CAS PubMed
.
- P. Gong, W. Zheng, Z. Huang, W. Zhang, D. Xiao and X. Jiang, Adv. Funct. Mater., 2013, 23, 42–46 CrossRef CAS
.
- D. Sarkar, P. K. Vemula, G. S. L. Teo, D. Spelke, R. Karnik, L. Y. Wee and J. M. Karp, Bioconjugate Chem., 2008, 19, 2105–2109 CrossRef CAS PubMed
.
- A. J. Bradley, K. L. Murad, K. L. Regan and M. D. Scott, Biochim. Biophys. Acta, 2002, 1561, 147–158 CrossRef CAS PubMed
.
- M. D. Scott, K. L. Murad, F. Koumpouras, M. Talbot and J. W. Eaton, Proc. Natl. Acad. Sci. U. S. A., 1997, 94, 7566–7571 CrossRef CAS PubMed
.
- B. Huang, W. D. Abraham, Y. Zheng, S. C. Bustamante López, S. S. Luo and D. J. Irvine, Sci. Transl. Med., 2015, 7, 291ra294 Search PubMed
.
- M. T. Stephan, S. B. Stephan, P. Bak, J. Chen and D. J. Irvine, Biomaterials, 2012, 33, 5776–5787 CrossRef CAS PubMed
.
- M. T. Stephan, J. J. Moon, S. H. Um, A. Bershteyn and D. J. Irvine, Nat. Med., 2010, 16, 1035–1041 CrossRef CAS PubMed
.
- C. Wang, W. Sun, Y. Ye, Q. Hu, H. N. Bomba and Z. Gu, Nat. Biomed. Eng., 2017, 1, 0011 CrossRef CAS
.
- X. Wang, X. Meng, K. Mao, H. Chen, X. Cong, F. Liu, J. Wang, S. Liu, Y. Xin, G. Zhu, H. Tan, Y. G. Yang and T. Sun, Biomaterials, 2023, 300, 122187 CrossRef CAS PubMed
.
- T. Li and Y. Liu, Anal. Chem., 2021, 93, 7029–7036 CrossRef CAS PubMed
.
- Z. Luo, L. Luo, Y. Lu, C. Zhu, B. Qin, M. Jiang, X. Li, Y. Shi, J. Zhang, Y. Liu, X. Shan, H. Yin, G. Guan, Y. Du, N. Cheng and J. You, J. Nanobiotechnol., 2022, 20, 261 CrossRef CAS PubMed
.
- L. Wayteck, H. Dewitte, L. De Backer, K. Breckpot, J. Demeester, S. C. De Smedt and K. Raemdonck, Biomaterials, 2016, 77, 243–254 CrossRef CAS PubMed
.
- T. Li, W. Gao, J. Liang, M. Zha, Y. Chen, Y. Zhao and C. Wu, Anal. Chem., 2017, 89, 8501–8508 CrossRef CAS PubMed
.
- L. He, H. Wang, Y. Han, K. Wang, H. Dong, Y. Li, D. Shi and Y. Li, ACS Appl. Mater. Interfaces, 2019, 11, 47750–47761 CrossRef CAS PubMed
.
- R. Bej, A. Ghosh, J. Sarkar, B. B. Das and S. Ghosh, Chembiochem, 2020, 21, 2921–2926 CrossRef CAS PubMed
.
- Y. Wen, Y. Liu, F. Guo, Y. Han, Q. Qiu, Y. Li, H. Dong, T. Ren and Y. Li, Biomater. Sci., 2021, 9, 973–984 RSC
.
- Q. Sun, G. Liu, H. Wu, H. Xue, Y. Zhao, Z. Wang, Y. Wei, Z. Wang and L. Tao, ACS Macro Lett., 2017, 6, 550–555 CrossRef CAS PubMed
.
- Q. Zhang, Y. Zhang, Y. Zhao, B. Yang, C. Fu, Y. Wei and L. Tao, ACS Macro Lett., 2015, 4, 128–132 CrossRef CAS PubMed
.
- Y. Qu, Y. Zheng, L. Yu, Y. Zhou, Y. Wang, Y. Zhang, Q. Yu and H. Chen, Adv. Funct. Mater., 2020, 30, 1906362 CrossRef CAS
.
- M. N. Christiansen, J. Chik, L. Lee, M. Anugraham, J. L. Abrahams and N. H. Packer, Proteomics, 2014, 14, 525–546 CrossRef CAS PubMed
.
- C. Rajan, J. Seema, Y. W. Chen, T. C. Chen, M. H. Lin, C. H. Lin and D. W. Hwang, Biomedicines, 2021, 9, 1459 CrossRef CAS PubMed
.
- A. J. Amaral and G. Pasparakis, Chem. Commun., 2015, 51, 17556–17559 RSC
.
- J. Wang, W. Wu, Y. Zhang, X. Wang, H. Qian, B. Liu and X. Jiang, Biomaterials, 2014, 35, 866–878 CrossRef CAS PubMed
.
- H. Liu, Y. Li, K. Sun, J. Fan, P. Zhang, J. Meng, S. Wang and L. Jiang, J. Am. Chem. Soc., 2013, 135, 7603–7609 CrossRef CAS PubMed
.
- J. Martinelli, L. Tei, S. Geninatti Crich, D. Alberti and K. Djanashvili, Molecules, 2021, 26, 1730 CrossRef CAS PubMed
.
- S. Shinde, Z. El-Schich, A. Malakpour, W. Wan, N. Dizeyi, R. Mohammadi, K. Rurack, A. Gjorloff Wingren and B. Sellergren, J. Am. Chem. Soc., 2015, 137, 13908–13912 CrossRef CAS PubMed
.
- J. Kim, Y. M. Lee, H. Kim, D. Park, J. Kim and W. J. Kim, Biomaterials, 2016, 75, 102–111 CrossRef CAS PubMed
.
- J. Yan, G. Springsteen, S. Deeter and B. Wang, Tetrahedron, 2004, 60, 11205–11209 CrossRef CAS
.
- W. Chen, X. Zhen, W. Wu and X. Jiang, Sci. China: Chem., 2020, 63, 648–664 CrossRef CAS
.
- B. Dhawan, G. Akhter, H. Hamid, P. Kesharwani and M. S. Alam, J. Mol. Struct., 2022, 1252, 132057 CrossRef CAS
.
- G. Morgese, B. F. M. de Waal, S. Varela-Aramburu, A. R. A. Palmans, L. Albertazzi and E. W. Meijer, Angew. Chem., Int. Ed. Engl., 2020, 59, 17229–17233 CrossRef CAS PubMed
.
- N. Ma, J. Hu, Z. M. Zhang, W. Liu, M. Huang, Y. Fan, X. Yin, J. Wang, K. Ding, W. Ye and Z. Li, J. Am. Chem. Soc., 2020, 142, 6051–6059 CrossRef CAS PubMed
.
- J. Sinclair and A. K. Salem, Biomaterials, 2006, 27, 2090–2094 CrossRef CAS PubMed
.
- A. Ciupa, P. A. De Bank and L. Caggiano, Chem. Commun., 2013, 49, 10148–10150 RSC
.
- D. Zhao, S. M. Ong, Z. Yue, Z. Jiang, Y. C. Toh, M. Khan, J. Shi, C. H. Tan, J. P. Chen and H. Yu, Biomaterials, 2008, 29, 3693–3702 CrossRef CAS PubMed
.
- S. M. Ong, L. He, N. T. Thuy Linh, Y. H. Tee, T. Arooz, G. Tang, C. H. Tan and H. Yu, Biomaterials, 2007, 28, 3656–3667 CrossRef CAS PubMed
.
- X. Liu, Y. Wang, B. Ye and X. Bi, Chem. Sci., 2023, 14, 7334–7345 RSC
.
- B. B. Chen, X. Y. Wang and R. C. Qian, Chem. Commun., 2019, 55, 9681–9684 RSC
.
- I. Koh, I. Yong, B. Kim, D. Choi, J. Hong, Y. M. Han and P. Kim, ACS Biomater. Sci. Eng., 2020, 6, 813–821 CrossRef CAS PubMed
.
- L. Li, B. Han, Y. Wang, J. Zhao and Y. Cao, Biosens. Bioelectron., 2019, 145, 111714 CrossRef CAS PubMed
.
- J. Cha, H. Kim, N. S. Hwang and P. Kim, ACS Appl. Mater. Interfaces, 2018, 10, 35676–35680 CrossRef CAS PubMed
.
- H. Kim, K. Shin, O. K. Park, D. Choi, H. D. Kim, S. Baik, S. H. Lee, S. H. Kwon, K. J. Yarema, J. Hong, T. Hyeon and N. S. Hwang, J. Am. Chem. Soc., 2018, 140, 1199–1202 CrossRef CAS PubMed
.
- M. Hao, L. Zhu, S. Hou, S. Chen, X. Li, K. Li, N. Zhu, S. Chen, L. Xue, C. Ju and C. Zhang, ACS Nano, 2023, 17, 1663–1680 CrossRef CAS PubMed
.
- P. Shi, X. Wang, B. Davis, J. Coyne, C. Dong, J. Reynolds and Y. Wang, Angew. Chem., Int. Ed. Engl., 2020, 59, 11892–11897 CrossRef CAS PubMed
.
- J. Niu, D. J. Lunn, A. Pusuluri, J. I. Yoo, M. A. O'Malley, S. Mitragotri, H. T. Soh and C. J. Hawker, Nat. Chem., 2017, 9, 537–545 CrossRef CAS PubMed
.
- M. Hao, S. Hou, W. Li, K. Li, L. Xue, Q. Hu, L. Zhu, Y. Chen, H. Sun, C. Ju and C. Zhang, Sci. Transl. Med., 2020, 12, eaaz6667 CrossRef CAS PubMed
.
- F. Huang, J. Liu and Y. Liu, Chem. Sci., 2022, 13, 8885–8894 RSC
.
- C. Gao, Q. Cheng, J. Li, J. Chen, Q. Wang, J. Wei, Q. Huang, S. M. Y. Lee, D. Gu and R. Wang, Adv. Funct. Mater., 2021, 31, 2102440 CrossRef CAS
.
- A. Yoshihara, S. Watanabe, I. Goel, K. Ishihara, K. N. Ekdahl, B. Nilsson and Y. Teramura, Biomaterials, 2020, 253, 120113 CrossRef CAS PubMed
.
- C. Gao, Q. Cheng, J. Wei, C. Sun, S. Lu, C. H. T. Kwong, S. M. Y. Lee, Z. Zhong and R. Wang, Mater. Today, 2020, 40, 9–17 CrossRef CAS
.
- E. Kim, J. C. Kim, K. Min, M. Goh and G. Tae, ACS Appl. Mater. Interfaces, 2018, 10, 24431–24439 CrossRef CAS PubMed
.
- Y. Teramura, S. Asif, K. N. Ekdahl, E. Gustafson and B. Nilsson, ACS Appl. Mater. Interfaces, 2017, 9, 244–254 CrossRef CAS PubMed
.
- M. O. Altman, Y. M. Chang, X. Xiong and W. Tan, Sci. Rep., 2013, 3, 3343 CrossRef PubMed
.
- X. Wang, C. Wen, B. Davis, P. Shi, L. Abune, K. Lee, C. Dong and Y. Wang, ACS Appl. Mater. Interfaces, 2022, 14, 3900–3909 CrossRef CAS PubMed
.
- R. C. Qian, Z. R. Zhou, W. Guo, Y. Wu, Z. Yang and Y. Lu, J. Am. Chem. Soc., 2021, 143, 5737–5744 CrossRef CAS PubMed
.
- X. Chen, X. Zhang, H. Y. Wang, Z. Chen and F. G. Wu, Langmuir, 2016, 32, 10126–10135 CrossRef CAS PubMed
.
- W. Meier, Langmuir, 2000, 16, 1457–1459 CrossRef CAS
.
- Y. Zheng, T. Wegner, D. Di Iorio, M. Pierau, F. Glorius and S. V. Wegner, ACS Chem. Biol., 2023, 18, 1435–1443 CrossRef CAS PubMed
.
- C. Lv, Y. Li, M. Zhang, Y. Cheng, D. Han and W. Tan, Nano Lett., 2023, 23, 1167–1174 CrossRef CAS PubMed
.
- B. Davis, K. Lee, X. Wang and Y. Wang, Biomacromolecules, 2023, 24, 3193–3202 CrossRef CAS PubMed
.
- Y. Fang, Y. Yan, S. Bi, Y. Wang, Y. Chen, P. Xu, H. Ju and Y. Liu, Anal. Chem., 2022, 94, 13205–13214 CrossRef CAS PubMed
.
- S. F. Jones, H. Joshi, S. J. Terry, J. R. Burns, A. Aksimentiev, U. S. Eggert and S. Howorka, J. Am. Chem. Soc., 2021, 143, 8305–8313 CrossRef CAS PubMed
.
- L. Zhang, F. Wang, Q. Li, L. Wang, C. Fan, J. Li and Y. Zhu, Nano Lett., 2020, 20, 3521–3527 CrossRef CAS PubMed
.
- Y. Gu, B. Liu, Q. Liu, Y. Hang, L. Wang, J. L. Brash, G. Chen and H. Chen, ACS Appl. Mater. Interfaces, 2019, 11, 47720–47729 CrossRef CAS PubMed
.
- Y. Kong, F. Yuan, F. Yang, C. Zhang and Y. Xian, Anal. Chem., 2022, 94, 12024–12032 CrossRef CAS PubMed
.
- J. H. Jeong, J. J. Schmidt, R. E. Kohman, A. T. Zill, R. J. DeVolder, C. E. Smith, M. H. Lai, A. Shkumatov, T. W. Jensen, L. G. Schook, S. C. Zimmerman and H. Kong, J. Am. Chem. Soc., 2013, 135, 8770–8773 CrossRef CAS PubMed
.
- T. Chen, Y. Xue, S. Wang, J. Lu, H. Zhou, W. Zhang, Z. Zhou, B. Li, Y. Li, Z. Wang, C. Li, Y. Eloy, H. Sun, Y. Shen, M. D. Diarra, C. Ge, X. Chai, H. Mou, P. Lin, X. Yu and Z. Ye, Bioact. Mater., 2023, 23, 508–523 CAS
.
- L.-H. Liu, W.-X. Qiu, Y.-H. Zhang, B. Li, C. Zhang, F. Gao, L. Zhang and X.-Z. Zhang, Adv. Funct. Mater., 2017, 27, 1700220 CrossRef
.
- C. Y. Lo, B. R. Weil, B. A. Palka, A. Momeni, J. M. Canty Jr and S. Neelamegham, Biomaterials, 2016, 74, 19–30 CrossRef CAS PubMed
.
- R. J. Weber, S. I. Liang, N. S. Selden, T. A. Desai and Z. J. Gartner, Biomacromolecules, 2014, 15, 4621–4626 CrossRef CAS PubMed
.
- D. C. Church and J. K. Pokorski, Angew. Chem., Int. Ed. Engl., 2020, 59, 11379–11383 CrossRef CAS PubMed
.
- Z. Rao, M. Sasaki and T. Taguchi, Colloids Surf., B, 2013, 101, 223–227 CrossRef CAS PubMed
.
- T. Taguchi, Z. Rao, M. Ito and M. Matsuda, J. Mater. Sci.: Mater. Med., 2011, 22, 2357–2363 CrossRef CAS PubMed
.
- M. Ito and T. Taguchi, Acta Biomater., 2009, 5, 2945–2952 CrossRef CAS PubMed
.
- Y. Zhao, M. Fan, Y. Chen, Z. Liu, C. Shao, B. Jin, X. Wang, L. Hui, S. Wang, Z. Liao, D. Ling, R. Tang and B. Wang, Sci. Adv., 2020, 6, eaaw9679 CrossRef CAS PubMed
.
- S. Sakai and M. Taya, ACS Macro Lett., 2014, 3, 972–975 CrossRef CAS PubMed
.
- K. Kato, C. Itoh, T. Yasukouchi and T. Nagamune, Biotechnol. Prog., 2004, 20, 897–904 CrossRef CAS PubMed
.
- L. Zhang, G. Liu, K. Lv, J. Xin, Y. Wang, J. Zhao, W. Hu, C. Xiao, K. Zhu, L. Zhu, J. Nan, Y. Feng, H. Zhu, W. Chen, W. Zhu, J. Zhang, J. Wang, B. Wang and X. Hu, Adv. Sci., 2021, 8, 2003348 CrossRef CAS PubMed
.
- L. Sun, F. Shen, Z. Xiong, H. Yang, Z. Dong, J. Xiang, Q. Gu, Q. Ji, C. Fan and Z. Liu, J. Am. Chem. Soc., 2022, 144, 7634–7645 CrossRef CAS PubMed
.
- L. Sun, F. Shen, J. Xu, X. Han, C. Fan and Z. Liu, Angew. Chem., Int. Ed. Engl., 2020, 59, 14842–14853 CrossRef CAS PubMed
.
- M. Lin, Y. Chen, S. Zhao, R. Tang, Z. Nie and H. Xing, Angew. Chem., Int. Ed. Engl., 2022, 61, e202111647 CrossRef CAS PubMed
.
- D. Sarkar, P. K. Vemula, W. Zhao, A. Gupta, R. Karnik and J. M. Karp, Biomaterials, 2010, 31, 5266–5274 CrossRef CAS PubMed
.
- D. Dutta, A. Pulsipher, W. Luo, H. Mak and M. N. Yousaf, Bioconjugate Chem., 2011, 22, 2423–2433 CrossRef CAS PubMed
.
- D. Dutta, A. Pulsipher, W. Luo and M. N. Yousaf, J. Am. Chem. Soc., 2011, 133, 8704–8713 CrossRef CAS PubMed
.
- S. Elahipanah, P. J. O'Brien, D. Rogozhnikov and M. N. Yousaf, Bioconjugate Chem., 2017, 28, 1422–1433 CrossRef CAS PubMed
.
- W. Luo, A. Pulsipher, D. Dutta, B. M. Lamb and M. N. Yousaf, Sci. Rep., 2014, 4, 6313 CrossRef CAS PubMed
.
- W. Luo, N. Westcott, D. Dutta, A. Pulsipher, D. Rogozhnikov, J. Chen and M. N. Yousaf, ACS Chem. Biol., 2015, 10, 2219–2226 CrossRef CAS PubMed
.
- P. J. O'Brien, S. Elahipanah, D. Rogozhnikov and M. N. Yousaf, ACS Cent. Sci., 2017, 3, 489–500 CrossRef PubMed
.
- P. J. O'Brien, W. Luo, D. Rogozhnikov, J. Chen and M. N. Yousaf, Bioconjugate Chem., 2015, 26, 1939–1949 CrossRef PubMed
.
- A. Pulsipher, D. Dutta, W. Luo and M. N. Yousaf, Angew. Chem., Int. Ed. Engl., 2014, 53, 9487–9492 CrossRef CAS PubMed
.
- D. Rogozhnikov, P. J. O'Brien, S. Elahipanah and M. N. Yousaf, Sci. Rep., 2016, 6, 39806 CrossRef CAS PubMed
.
- C. Zheng, Q. Zhong, W. Song, K. Yi, H. Kong, H. Wang, Y. Tao, M. Li and X. Chen, Adv. Mater., 2023, 35, 2206989 CrossRef CAS PubMed
.
- C. Shi, Q. Zhang, Y. Yao, F. Zeng, C. Du, S. Nijiati, X. Wen, X. Zhang, H. Yang, H. Chen, Z. Guo, X. Zhang, J. Gao, W. Guo, X. Chen and Z. Zhou, Nat. Nanotechnol., 2023, 18, 86–97 CrossRef CAS PubMed
.
- Y. Chen, T. Wu, S. Xie, Y. Bai and H. Xing, Sci. Adv., 2023, 9, eadg2583 CrossRef CAS PubMed
.
- W. Youn, E. H. Ko, M. H. Kim, M. Park, D. Hong, G. A. Seisenbaeva, V. G. Kessler and I. S. Choi, Angew. Chem., Int. Ed. Engl., 2017, 56, 10702–10706 CrossRef CAS PubMed
.
- T. Thomsen and H. A. Klok, ACS Appl. Bio Mater., 2021, 4, 2293–2306 CrossRef CAS PubMed
.
- M. M. Maciel, T. R. Correia, V. M. Gaspar, J. M. M. Rodrigues, I. S. Choi and J. F. Mano, Adv. Funct. Mater., 2021, 31, 2009619 CrossRef CAS
.
- T. Thomsen, R. Reissmann, E. Kaba, B. Engelhardt and H. A. Klok, Biomacromolecules, 2021, 22, 3416–3430 CrossRef CAS PubMed
.
- J. Lee, J. Choi, J. H. Park, M. H. Kim, D. Hong, H. Cho, S. H. Yang and I. S. Choi, Angew. Chem., Int. Ed. Engl., 2014, 53, 8056–8059 CrossRef CAS PubMed
.
- J. T. Wilson, W. Cui, V. Kozlovskaya, E. Kharlampieva, D. Pan, Z. Qu, V. R. Krishnamurthy, J. Mets, V. Kumar, J. Wen, Y. Song, V. V. Tsukruk and E. L. Chaikof, J. Am. Chem. Soc., 2011, 133, 7054–7064 CrossRef CAS PubMed
.
- D. Choi, H. Lee, H.-B. Kim, M. Yang, J. Heo, Y. Won, S. S. Jang, J. K. Park, Y. Son, T. I. Oh, E. Lee and J. Hong, Chem. Mater., 2017, 29, 2055–2065 CrossRef CAS
.
- J. Yang, J. Li, X. Li, X. Wang, Y. Yang, N. Kawazoe and G. Chen, Biomaterials, 2017, 133, 253–262 CrossRef CAS PubMed
.
- P. P. Wibroe, A. C. Anselmo, P. H. Nilsson, A. Sarode, V. Gupta, R. Urbanics, J. Szebeni, A. C. Hunter, S. Mitragotri, T. E. Mollnes and S. M. Moghimi, Nat. Nanotechnol., 2017, 12, 589–594 CrossRef CAS PubMed
.
- A. Barbul, K. Singh, L. Horev–Azaria, S. Dasgupta, T. Auth, R. Korenstein and G. Gompper, ACS Appl. Nano Mater., 2018, 1, 3785–3799 CrossRef CAS
.
- A. C. Anselmo, V. Gupta, B. J. Zern, D. Pan, M. Zakrewsky, V. Muzykantov and S. Mitragotri, ACS Nano, 2013, 7, 11129–11137 CrossRef CAS PubMed
.
- T. Thomsen, A. B. Ayoub, D. Psaltis and H. A. Klok, Biomacromolecules, 2021, 22, 190–200 CrossRef CAS PubMed
.
- S. Y. Han, H. Lee, D. T. Nguyen, G. Yun, S. Kim, J. H. Park and I. S. Choi, ACS Appl. Bio Mater., 2021, 1, 2000037 CAS
.
- J. Yang, Y. Yang, N. Kawazoe and G. Chen, Biomaterials, 2019, 197, 317–326 CrossRef CAS PubMed
.
- S. Mansouri, Y. Merhi, F. M. Winnik and M. Tabrizian, Biomacromolecules, 2011, 12, 585–592 CrossRef CAS PubMed
.
- J. Wang, Y. Miao, Y. Huang, B. Lin, X. Liu, S. Xiao, L. Du, Z. Hu and M. Xing, Adv. Healthcare Mater., 2018, 7, 1700447 CrossRef PubMed
.
- Y. Fukuda, T. Akagi, T. Asaoka, H. Eguchi, K. Sasaki, Y. Iwagami, D. Yamada, T. Noda, K. Kawamoto, K. Gotoh, S. Kobayashi, M. Mori, Y. Doki and M. Akashi, Biomaterials, 2018, 160, 82–91 CrossRef CAS PubMed
.
- O. Hasturk, J. K. Sahoo and D. L. Kaplan, Biomacromolecules, 2020, 21, 2829–2843 CrossRef CAS PubMed
.
- J. Hwang, D. Choi, M. Choi, Y. Seo, J. Son, J. Hong and J. Choi, ACS Appl. Mater. Interfaces, 2018, 10, 17685–17692 CrossRef CAS PubMed
.
- L. P. B. Guerzoni, Y. Tsukamoto, D. B. Gehlen, D. Rommel, T. Haraszti, M. Akashi and L. De Laporte, Biomacromolecules, 2019, 20, 3746–3754 CrossRef CAS PubMed
.
- A. Matsuzawa, M. Matsusaki and M. Akashi, Langmuir, 2013, 29, 7362–7368 CrossRef CAS PubMed
.
- J. Sun, Y. Ren, W. Wang, H. Hao, M. Tang, Z. Zhang, J. Yang, Y. Zheng and X. Shi, ACS Biomater. Sci. Eng., 2020, 6, 2336–2345 CrossRef CAS PubMed
.
- K. Sasaki, T. Akagi, T. Asaoka, H. Eguchi, Y. Fukuda, Y. Iwagami, D. Yamada, T. Noda, H. Wada, K. Gotoh, K. Kawamoto, Y. Doki, M. Mori and M. Akashi, Biomaterials, 2017, 133, 263–274 CrossRef CAS PubMed
.
- W. Li, T. Guan, X. Zhang, Z. Wang, M. Wang, W. Zhong, H. Feng, M. Xing and J. Kong, ACS Appl. Mater. Interfaces, 2015, 7, 3018–3029 CrossRef CAS PubMed
.
- H. Lee, N. Kim, H. B. Rheem, B. J. Kim, J. H. Park and I. S. Choi, Adv. Healthcare Mater., 2021, 10, 2100347 CrossRef CAS PubMed
.
- S. Zhao, L. Zhang, J. Han, J. Chu, H. Wang, X. Chen, Y. Wang, N. Tun, L. Lu, X. F. Bai, M. Yearsley, S. Devine, X. He and J. Yu, ACS Nano, 2016, 10, 6189–6200 CrossRef CAS PubMed
.
- U. Han, Y. J. Kim, W. Kim, J. H. Park and J. Hong, Nanoscale, 2019, 11, 13541–13551 RSC
.
- L. K. Mahal, K. J. Yarema and C. R. Bertozzi, Science, 1997, 276, 1125–1128 CrossRef CAS PubMed
.
- R. M. F. Tomas and M. I. Gibson, ACS Macro Lett., 2020, 9, 991–1003 CrossRef CAS PubMed
.
- T. L. Hsu, S. R. Hanson, K. Kishikawa, S. Wang, M. Sawa and C. H. Wong, Proc. Natl. Acad. Sci. U. S. A., 2007, 104, 2614–2619 CrossRef CAS PubMed
.
- S. G. Sampathkumar, A. V. Li, M. B. Jones, Z. Sun and K. J. Yarema, Nat. Chem. Biol., 2006, 2, 149–152 CrossRef CAS PubMed
.
- E. Saxon and C. R. Bertozzi, Science, 2000, 287, 2007–2010 CrossRef CAS PubMed
.
- A. K. Spate, J. E. Dold, E. Batroff, V. F. Schart, D. E. Wieland, O. R. Baudendistel and V. Wittmann, Chembiochem, 2016, 17, 1374–1383 CrossRef PubMed
.
- P. Shi, E. Ju, Z. Yan, N. Gao, J. Wang, J. Hou, Y. Zhang, J. Ren and X. Qu, Nat. Commun., 2016, 7, 13088 CrossRef CAS PubMed
.
- A. Uvyn, R. De Coen, O. De Wever, K. Deswarte, B. N. Lambrecht and B. G. De Geest, Chem. Commun., 2019, 55, 10952–10955 RSC
.
- R. M. F. Tomas and M. I. Gibson, Biomacromolecules, 2019, 20, 2726–2736 CrossRef CAS PubMed
.
- R. M. F. Tomas and M. I. Gibson, Chem. Sci., 2021, 12, 4557–4569 RSC
.
- R. M. F. Tomas, B. Martyn, T. L. Bailey and M. I. Gibson, ACS Macro Lett., 2018, 7, 1289–1294 CrossRef CAS PubMed
.
- H. Zhou, Y. Zhang, P. Pei, W. Shen, X. Yi and K. Yang, Biomaterials, 2023, 300, 122202 CrossRef CAS PubMed
.
- Y. Chen, P. Gao, W. Pan, M. Shi, S. Liu, N. Li and B. Tang, Chem. Sci., 2021, 12, 13817–13824 RSC
.
- A. Lamoot, A. Uvyn, S. Kasmi and B. G. De Geest, Angew. Chem., Int. Ed. Engl., 2021, 60, 6320–6325 CrossRef CAS PubMed
.
- H. Wang, M. C. Sobral, D. K. Y. Zhang, A. N. Cartwright, A. W. Li, M. O. Dellacherie, C. M. Tringides, S. T. Koshy, K. W. Wucherpfennig and D. J. Mooney, Nat. Mater., 2020, 19, 1244–1252 CrossRef CAS PubMed
.
- Y. Tu, Y. Dong, K. Wang, S. Shen, Y. Yuan and J. Wang, Biomaterials, 2020, 259, 120298 CrossRef CAS PubMed
.
- X. Fan, Q. Song, D.-e. Sun, Y. Hao, J. Wang, C. Wang and X. Chen, Nat. Chem. Biol., 2022, 18, 625–633 CrossRef CAS PubMed
.
- N. Gong, X. Han, L. Xue, R. El-Mayta, A. E. Metzloff, M. M. Billingsley, A. G. Hamilton and M. J. Mitchell, Nat. Mater., 2023 DOI:10.1038/s41563-023-01646-6
.
- F. Chai, D. Cheng, Y. Nasu, T. Terai and R. E. Campbell, Biochem. Soc. Trans., 2023, 51, 1585–1595 CrossRef CAS PubMed
.
- C. G. England, H. Luo and W. Cai, Bioconjugate Chem., 2015, 26, 975–986 CrossRef CAS PubMed
.
- A. Keppler, S. Gendreizig, T. Gronemeyer, H. Pick, H. Vogel and K. Johnsson, Nat. Biotechnol., 2003, 21, 86–89 CrossRef CAS PubMed
.
- N. George, H. Pick, H. Vogel, N. Johnsson and K. Johnsson, J. Am. Chem. Soc., 2004, 126, 8896–8897 CrossRef CAS PubMed
.
- A. Pulsipher, M. E. Griffin, S. E. Stone and L. C. Hsieh-Wilson, Angew. Chem., Int. Ed. Engl., 2015, 54, 1466–1470 CrossRef CAS PubMed
.
- Q. Liu, S. Jiang, B. Liu, Y. Yu, Z. A. Zhao, C. Wang, Z. Liu, G. Chen and H. Chen, ACS Macro Lett., 2019, 8, 337–344 CrossRef CAS PubMed
.
- N. Pishesha, A. M. Bilate, M. C. Wibowo, N.-J. Huang, Z. Li, R. Deshycka, D. Bousbaine, H. Li, H. C. Patterson, S. K. Dougan, T. Maruyama, H. F. Lodish and H. L. Ploegh, Proc. Natl. Acad. Sci. U. S. A., 2017, 114, 3157–3162 CrossRef CAS PubMed
.
- J. Li, M. Chen, Z. Liu, L. Zhang, B. H. Felding, K. W. Moremen, G. Lauvau, M. Abadier, K. Ley and P. Wu, ACS Cent. Sci., 2018, 4, 1633–1641 CrossRef CAS PubMed
.
- L. Zhu, R. Feng, G. Chen, C. Wang, Z. Liu, Z. Zhang and H. Chen, ACS Appl. Mater. Interfaces, 2022, 14, 4921–4930 CrossRef CAS PubMed
.
- L. Yu, R. Feng, L. Zhu, Q. Hao, J. Chu, Y. Gu, Y. Luo, Z. Zhang, G. Chen and H. Chen, Sci. Adv., 2020, 6, eabb6595 CrossRef PubMed
.
- T. N. C. Ramya, E. Weerapana, B. F. Cravatt and J. C. Paulson, Glycobiology, 2013, 23, 211–221 CrossRef CAS PubMed
.
- C. J. Capicciotti, C. Zong, M. O. Sheikh, T. Sun, L. Wells and G. J. Boons, J. Am. Chem. Soc., 2017, 139, 13342–13348 CrossRef CAS PubMed
.
- S. Hong, C. Yu, P. Wang, Y. Shi, W. Cao, B. Cheng, D. G. Chapla, Y. Ma, J. Li, E. Rodrigues, Y. Narimatsu, J. R. Yates III, X. Chen, H. Clausen, K. W. Moremen, M. S. Macauley, J. C. Paulson and P. Wu, Angew. Chem., Int. Ed. Engl., 2021, 60, 3603–3610 CrossRef CAS PubMed
.
- T. Zheng, H. Jiang, M. Gros, D. Soriano del Amo, S. Sundaram, G. Lauvau, F. Marlow, Y. Liu, P. Stanley and P. Wu, Angew. Chem., Int. Ed. Engl., 2011, 50, 4113–4118 CrossRef CAS PubMed
.
- T. Tanaka, T. Yamamoto, S. Tsukiji and T. Nagamune, Chembiochem, 2008, 9, 802–807 CrossRef CAS PubMed
.
- J. Shi, L. Kundrat, N. Pishesha, A. Bilate, C. Theile, T. Maruyama, S. K. Dougan, H. L. Ploegh and H. F. Lodish, Proc. Natl. Acad. Sci. U. S. A., 2014, 111, 10131–10136 CrossRef CAS PubMed
.
- C. Lin and A. Y. Ting, J. Am. Chem. Soc., 2006, 128, 4542–4543 CrossRef CAS PubMed
.
- U. S. Patil, H. Qu, D. Caruntu, C. J. O'Connor, A. Sharma, Y. Cai and M. A. Tarr, Bioconjugate Chem., 2013, 24, 1562–1569 CrossRef CAS PubMed
.
- S. Hong, C. Yu, P. Wang, Y. Shi, W. Cao, B. Cheng, D. G. Chapla, Y. Ma, J. Li, E. Rodrigues, Y. Narimatsu, J. R. Yates III, X. Chen, H. Clausen, K. W. Moremen, M. S. Macauley, J. C. Paulson and P. Wu, Angew. Chem., Int. Ed. Engl., 2021, 60, 3603–3610 CrossRef CAS PubMed
.
|
This journal is © The Royal Society of Chemistry 2023 |
Click here to see how this site uses Cookies. View our privacy policy here.