DOI:
10.1039/D4FO05580B
(Paper)
Food Funct., 2025,
16, 3296-3307
Organic acids from ice wine ameliorate fructose-induced disorders of glycolipid metabolism in C57BL/6J mice†
Received
13th November 2024
, Accepted 26th February 2025
First published on 28th February 2025
Abstract
Excessive intake of fructose has been widely reported to cause glycolipid metabolism disorders, and it is unclear whether long-term consumption of ice wine, a sweet wine with high sugar content, is beneficial for health. In this study, 6-week-old male C57BL/6J mice were divided into pure water, ice wine, fructose, fructose + succinic acid, fructose + malic acid and fructose + alcohol groups to study the effects and mechanisms of organic acids on glycolipid metabolism. The results indicated that long-term consumption of ice wine did not lead to disorders of glycolipid metabolism, and organic acids inhibited the negative effects of fructose and reduced hepatic fat synthesis by decreasing the mRNA expression of hepatic ACC1, SREBP-1c, and ChREBP-β, as well as controlling the protein expression of KHK-C. These findings provide a theoretical basis for the healthy consumption of ice wine, helping consumers enjoy wine more scientifically and promoting the high-quality development of the industry.
Introduction
In recent years, fructose has been more widely consumed as a food sweetener due to its high relative sweetness. Low doses of fructose are mostly metabolized by the gut, and excessive intake saturates the fructose absorption and clearance capacity in the intestine, spilling over into the liver and colonic microbiota, with adverse health effects through affecting liver function or microbial composition.1 Large amounts of fructose have been shown to lead to liver damage,2,3 impair glucose tolerance, contribute to the development of non-alcoholic fatty liver disease,4,5 promote whitening of brown adipocytes and cause hypertrophy of white adipocytes in mice, and these changes are associated with disorders of glycolipid metabolism to a similar extent to those caused by a high-fat diet.6
Organic acids are a class of natural compounds containing carboxyl groups, which are considered beneficial for glycolipid metabolism due to their physiologically active functions.7,8 Studies have suggested that supplementing with L-malic acid improves liver lipid accumulation in tilapia,9 while insulin-resistant individuals who ingest apple cider vinegar (containing acetic acid) have reduced serum glycerol, bile, and visceral fat.10 Dietary succinic acid, which is thought to be a substrate for intestinal gluconeogenesis, improves glucose and insulin tolerance in mice.11 Also, animals fed diets supplemented with organic acids exhibit better growth performance and different intestinal metabolic patterns.12
Ice wine is a sweet wine with a high sugar content (residual sugar ≥125 g L−1), which is loved by consumers for its good palatability.13 Meanwhile, organic acids, as the backbone of wine, are also widely present in ice wine and are important flavor substances.14 The organic acids in wine come not only from grapes (tartaric, malic, citric, and gluconic acids), but also from fermentation processes (succinic, fumaric, lactic, and acetic acids).15
In our previous study, we found that short-term consumption of ice wine for a period of 4 weeks did not significantly affect hepatic glycolipid metabolism in C57BL/6J mice.16 We hypothesized that longer-term consumption of ice wine might not lead to disruption of glycolipid metabolism and assumed that the higher content of organic acids such as malic and succinic acids would inhibit glycolipid metabolism disorders associated with high fructose intake. The present study addresses the above hypothesis by conducting experiments using two characteristic organic acids in wine, malic acid and succinic acid, in order to clarify the role of dietary organic acids in ameliorating the negative effects of fructose, to explore whether the longer-term consumption of ice wine may adversely affect glycolipid metabolism, and to guide healthy consumption of ice wine, so as to provide a theoretical basis for the use of organic acids in preventing and ameliorating metabolism-related disorders induced by ice wine or a high-fructose diet.
Materials and methods
Wine samples
Vidal ice wine (2022) was obtained from Liaoning Changyu Ice Wine Winery Co., Ltd (Huanren, China). Some indicators of the ice wine were determined according to the method described by Li with minor modifications.17 A Shimadzu LC-2020C HPLC system (Shimadzu, Kyoto, Japan) coupled with a photo-diode array (PDA) was used for the detection of organic acids at a 210 nm wavelength, and a refractive index detector (RID) was used to analyze glucose, fructose, glycerol and ethanol at 290 nm. Separation of these components in ice wine using an Aminex HPX-87H column (300 mm × 7.8 mm, Bio-Rad, America) was carried out in a 10 mM sulfuric acid solution at a flow rate of 0.6 mL min−1 and a column temperature of 60 °C. The injection volume was 20 μL.
Animal models and experimental design
Fifty-four 6-week-old male C57BL/6J mice, purchased from the Laboratory Animal Center of Northwest A&F University (Yangling, China), were group-housed in cages (three mice per cage) in a controlled environment (22 ± 2 °C and 50 ± 15% relative humidity) with a 12-hour light/12-hour dark cycle and free access to water and diet. All procedures conformed to the ARRIVE guidelines and were conducted in accordance with the Guidelines for the Management of Laboratory Animals issued by the National Science and Technology Commission of China. In addition, the experimental protocols were approved by the Ethics Committee of Northwest A&F University.
The mice were randomly divided into six distinct groups and gavaged with pure water (CK), ice wine (B), fructose (F, 127 g L−1), fructose + succinic acid (FS, 127 g L−1 + 3.5 g L−1), fructose + malic acid (FM, 127 g L−1 + 15.5 g L−1), and fructose + alcohol (FA, 127 g L−1 + 12 g L−1), using dedicated gavage needles attached to syringes with a thumb-forefinger needle-holding technique at a dose of 15 mL per kg bw per d for 10 weeks. All substances were dissolved in pure water.
Oral glucose tolerance test (OGTT) and insulin tolerance test (ITT)
The OGTT was performed at the beginning of the tenth week; mice were fed with glucose (2 g per kg bw) after fasting for 12 h. Glucose tolerance was determined by testing tail-tip blood glucose values at 0, 15, 30, 60 and 120 min using a blood glucose meter (Cofoe Medical Technology Co., Ltd, Hunan, China). At the end of the tenth week, the mice were fasted for 6 h and injected intraperitoneally with insulin (0.75 U kg−1, Tonghua Dongbao Pharmaceutical Co., Ltd, Jilin, China), and the blood glucose values were detected at 0, 15, 30, 60 and 120 min to determine the insulin sensitivity in mice. For the OGTT and ITT, the glucose change curves were plotted and the area under the curve (AUC) was also measured.
Biochemical analysis
Three days after the ITT, the mice were executed to collect samples. Plasma was obtained by centrifugation of blood from eyeball removal in anticoagulated centrifuge tubes at 16
000g for 10 min at 4 °C. Determination of plasma cholesterol (TC), triglyceride (TG), low-density lipoprotein (LDL-C), high-density lipoprotein (HDL-C), alanine aminotransferase (ALT), aspartate aminotransferase (AST), and liver TC, TG, LDL-C, HDL-C, ALT, AST, and total protein (BCA) was performed using kits from Nanjing Jiancheng Bioengineering Institute (Nanjing, China). Kits from Jiangsu Sumeike Biotech Co., Ltd (Yancheng, China) were used for the detection of plasma glucose (GLU), uric acid (UA), insulin (INS) and leptin (LEP).
The liver, spleen, epididymal white fat, inguinal white fat and scapular brown fat obtained from dissection were weighed. Organ coefficient (%) = organ weight (g)/body weight (g) × 100%.
Histopathology
Small intestines were isolated and measured for length. Parts of the jejunum, colon and liver were fixed in 4% paraformaldehyde solution for 24 hours, respectively; they were paraffin-embedded after ethanol washing and gradient dehydration, sectioned and stained with hematoxylin–eosin (H&E), dehydrated, permeabilized and sealed, and then observed using a light microscope (TONATYW M, Chongqing, China). Dinucleated hepatocytes, lipid droplets, blighted cells, and neutrophils were counted in five 200× fields of view of H&E-stained sections of the liver and bar graphs were plotted. Bar graphs were plotted using Image J (v1.8.0) for 20 measurements of the villus height, crypt depth, and muscle thickness in the jejunum and for the crypt depth and muscle thickness in the colon within the sections, respectively.
Furthermore, a portion of the liver was stained with oil red O after fixed sections, the background was differentiated using isopropyl alcohol, and after hematoxylin restaining, the liver was differentiated using hydrochloric acid solution, and the slices were sealed and observed using a light microscope. ImageJ was used to digitally analyse the images before plotting the bar charts.
16S rRNA
The contents taken from the mice colon were lysed to extract DNA, which was quality checked to determine whether it met the requirements for further experiments, and the target DNA was amplified with universal 16S rRNA gene primers, followed by gel electrophoresis of PCR products, purification and sequencing. Alpha diversity and beta diversity analyses were carried out using mothur (v.1.31.2, USA) and QIIME (v1.80, USA).
Quantitative real-time PCR analysis and western blot
PCR and WB were performed with reference to the published articles by Ma et al.16 The samples were processed using an RNA extraction kit (New Cell & Molecular Biotech Co., Ltd, Suzhou, China), followed by reverse transcription with HiScript IIQ RT SuperMix for Qpcr (+gDNA wiper) (Yeasen Biotech Co., Ltd, Shanghai, China), and amplification of gene expression by qRT-PCR (CFX96TM, Real-Time PCR Detection System, Bio-Rad). Data were analyzed using the 2−ΔΔCT method.
The lysed liver homogenate supernatant was assayed for total protein concentration, and protein denaturation was performed after adjusting the protein concentration to the same. It was separated using SDS-PAGE at a concentration of 7.5% before transferring it to a PVDF membrane, which was blocked with 5% skimmed milk for 2 h at room temperature, and then incubated with the primary antibody overnight at 4 °C, removed and washed, and incubated with the secondary antibody for 1 h at room temperature. Proteins were visualised by ECL luminescence using an imaging system (Champ Chemi 610 Plus, Sage, Beijing, China) and Sage Capture software (Sage, Beijing, China). Protein quantification was done using ImageJ.
Statistical analysis
All the data were expressed as the mean ± standard. The analysis of variance (ANOVA) was performed using the least significant difference test under IBM SPSS Statistics 26. All figures used to present the data were plotted using ORIGIN 2024. A value of p ≤ 0.05 was considered significant.
Results and discussion
Chemical compositions of ice wine
In order to validate the hypothetical role of the two sources of malic acid (from grape berries) and succinic acid (from the fermentation process), which are the most abundant in ice wine, and to exclude the effect of alcohol, animal experiments were carried out with similar concentrations of fructose (127 g L−1), fructose + succinic acid (127 g L−1 + 3.5 g L−1), fructose + malic acid (127 g L−1 + 15.5 g L−1), and fructose + alcohol (127 g L−1 + 12 g L−1). Glucose and glycerol were not considered as no relevant beneficial effects were reported (Table 1).
Table 1 Chemical composition of ice wine
Index |
Content (g L−1) |
Index |
Content (g L−1) |
Glucose |
45.73 ± 0.28 |
Tartaric acid |
0.9 ± 0.05 |
Fructose |
126.97 ± 0.14 |
Malic acid |
15.5 ± 0.05 |
Glycerol |
11.07 ± 0.66 |
Succinic acid |
3.83 ± 0.33 |
Ethanol |
12.17 ± 0.05 |
Lactic acid |
2.03 ± 0.8 |
Citric acid |
0.73 ± 0.00 |
Acetic acid |
0.17 ± 0.05 |
Effects of organic acids on glucose and insulin tolerance in mice
The OGTT and ITT are classical experiments for characterizing the general metabolic phenotype and specifically assessing the changes in glucose metabolism in mice. Among them, the OGTT was used as a tool to detect changes in peripheral disposition and insulin secretion over time with oral glucose loading.18 After ingesting glucose, the mice experienced a rapid increase in blood glucose, which peaked at approximately 15 min, and after 60 min, dropped to basal levels with proper glucose elimination (Fig. 1a). The blood glucose values of group F were the highest compared to the other groups at 15, 30 and 60 min, and significantly higher than the control group at 30 min. Besides, the increase of blood glucose in group F was sharper at 0–15 min, and the decrease was relatively gentler at 15–60 min, suggesting that the glucose tolerance of mice in group F was weakened, which might be caused by insulin resistance.18 While FS, FM, and FA groups exhibited a certain intervention for this adverse effect, particularly at 60 min, the blood glucose values of FM and FA groups were significantly lower than those of the F group. Meanwhile, Fig. 1c demonstrates the AUC of the OGTT, a parameter obtained by calculating the area of the glucose change curve versus time generation over 120 min in the OGTT.19 The AUC was significantly high in group F compared to the control group, which indicates increased glucose intolerance. Comparatively, the AUC values of FS and FM groups were lower than those of the F group, and the difference was significant in the FM group, showing that glucose disappeared faster, which means that the addition of succinic acid and malic acid improved glucose tolerance.
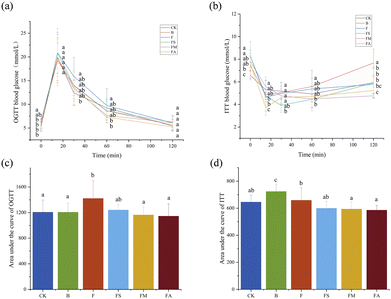 |
| Fig. 1 Effects of organic acids on glucose and insulin tolerance in mice. (a) OGTT blood glucose change curve; (b) ITT blood glucose change curve; (c) area under the OGTT curve; and (d) area under the ITT curve. n = 9. CK, control group; B, ice wine group; F, fructose group; FS, fructose + succinic acid group; FM, fructose + malic acid group; FA, fructose + alcohol group. OGTT: oral glucose tolerance test; ITT: insulin tolerance test. | |
Since insulin promotes glucose uptake by insulin-sensitive tissues and the extent of blood glucose reduction demonstrates systemic insulin action,20 systemic insulin sensitivity could be examined by ITT monitoring of blood glucose changes induced by insulin infusion.18,19 For all treatment groups, the fasting blood glucose of mice was significantly above that of the control group, but the tempo of blood glucose change was also found to be higher than that of the control group, which implies no decrease in insulin sensitivity (Fig. 1b). For the AUC of the ITT (Fig. 1d), it was significantly higher in the ice wine group compared to the other five groups, indicating that ice wine somewhat reduced whole-body insulin sensitivity, whereas those in the FM and FA groups were significantly lower than that in the F group, suggesting that the addition of malic acid and alcohol to fructose enhanced insulin sensitivity.
Effects of organic acids on the growth performance of mice
During the ten-week experiment, food intake was recorded daily and body weights were recorded at the end of each week. To exclude the effect of initial body weight on the growth results, the weekly weight gain of the mice over the initial body weight was measured (Fig. 2a). As observed, the mice showed sustained body weight growth, and the FS, B and CK groups consistently showed higher growth with no significant difference, which may be related to the relatively high feed intake (Fig. 2b). In contrast, the FM group experienced the lowest and significantly less sustained growth than the highest growth groups B or FS; accordingly, the dietary intake was significantly lower in the FM group compared to groups B and F. Although the fructose group consistently grew less than the control group, it might be related to the lower level of food intake.
 |
| Fig. 2 Effects of organic acids on the growth performance of mice. (a) Mice growth from initial body weight; (b) weight of food intake per day; (c) liver weight; (d) liver coefficient; (e) spleen weight; (f) spleen coefficient; (g) epididymal white fat weight; (h) groin white fat weight; and (i) scapular brown fat weight. n = 9. CK, control group; B, ice wine group; F, fructose group; FS, fructose + succinic acid group; FM, fructose + malic acid group; FA, fructose + alcohol group. | |
Meanwhile, the liver weights of mice in the B and FS groups were all slightly increased compared to the control group, and a slight decrease was observed in the F, FM, and FA groups, but none of them showed significant changes (Fig. 2c). Supplementation of malic acid with fructose intake resulted in significantly lower liver weight than that with succinic acid, probably due to the variability of the different types of acids. Regarding the statistics of liver coefficients, only group B showed a slight increase compared to the control group, and there was a non-significant decrease in the other four groups. The difference between the B and FA was significant (Fig. 2d). Normally, the ratio between organs and body weight remains relatively constant, and thus certain components within the ice wine might influence the relative homeostasis of the liver coefficient, succinic acid, malic acid, alcohol or even fructose, possibly constraining this effect. Fig. 2e and f demonstrate that there were no significant differences between groups for either splenic weight or splenic coefficient, but both the FS and FA groups were the highest and lowest value groups for these two parameters.
Also, adiposity plays an essential role in assessing the growth status of mice. Mammalian adipose tissue can be divided into two primary categories: white adipose tissue (WAT) and brown adipose tissue (BAT).21 WAT takes primary responsibility for lipid mobilization and storage, which stores the body's excess energy in the form of triglycerides, and also participates in immune, hormone secretion, and other activities. Excess WAT accumulation causes metabolic damage and presents a major risk factor for the development of cardiovascular disease, insulin resistance, and type 2 diabetes. Meanwhile, the BAT acts as a major thermogenic organ, both in terms of storing nutrients as lipids and consuming lipids as heat during non-shivering thermogenesis, and as a powerful metabolic tissue, with the ability to control glucose levels and triglyceride metabolism, which allows for the release or extraction of substances through the circulatory system.22,23
Sampling and weighing of epididymal white fat (Fig. 2g), inguinal white fat (Fig. 2h) and scapular brown adipose tissue (Fig. 2i) were carried out in mice. Significantly higher weights of both white fats were noted in mice gavaged with fructose than in controls, and ice wine gavage led to a non-significant increase in the weights of both white fats. For epididymal white fat, the addition of succinic acid and malic acid to fructose markedly enhanced it but resulted in a non-significant improvement over the gavage of fructose alone, and the addition of alcohol dramatically reduced it, bringing it closer to the control group. A significant increase in weight was achieved for inguinal white fat due to the co-existence of alcohol and fructose, while succinic acid and malic acid did not contribute effectively. Simultaneously, the weight of brown fat was less in all treatment groups than that of the control group, with significant differences in groups B and F. In this case, the presence of organic acids seems to be evident in somewhat inhibiting the undesirable accumulation of white fats due to fructose intake alone, as well as fostering healthy fat gain, which positively affects the healthy activation and profitable transformation of body fats.
Effects of organic acids on the morphology of mice organ tissues
H&E staining was performed on the liver, jejunum and colon samples in order to identify various tissue types and morphological changes. Typically, the nuclei were stained blue, and the cytoplasm and extracellular matrix showed varying degrees of pink coloration.24
Fig. 3a shows the relatively disorganized structure of the hepatocytes in group F, with tiny fat vacuoles and an increased number of binucleated hepatocytes accompanied by Kupffer cells and neutrophils, which could be an indication of the occurrence of mild steatosis and inflammation, whereas the overall structure of the cells in the treatment group with the simultaneous addition of succinic acid or malic acid was normal. Statistical analyses more accurately reflected these characteristics (Fig. 3c), while the binucleated hepatocytes, Kupffer cells and neutrophils in the ice wine and FA groups were significantly more than those in the control group but significantly lower than those in the fructose group. The FS group showed a significantly lower number of each index than the F group, whereas in the FM group, only the number of Kupffer cells was non-significantly different from that in the F group, but remained lower. It indicated that the presence of other components in ice wine suppressed the adverse phenomena brought about by fructose, and organic acids improved the inflammatory response of hepatocytes associated with fructose.
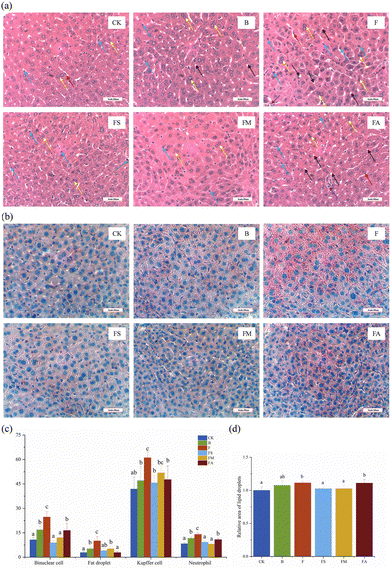 |
| Fig. 3 Effects of organic acids on the histomorphology of mice livers. (a) H&E-stained section of the liver (400× microscope field of view); (b) oil red O-stained sections of the liver (400× microscope field of view); (c) functional cell count after HE staining of the liver; and (d) relative area of a lipid droplet. CK, control group; B, ice wine group; F, fructose group; FS, fructose + succinic acid group; FM, fructose + malic acid group; FA, fructose + alcohol group. Yellow arrows: binuclear hepatocytes; black arrows: tiny fat droplets; blue arrows: Kupffer cells; green arrows: neutrophils; red arrows: epithelial cells; purple arrows: necrotic cells. | |
However, the observation of H&E staining results is subjective and relatively difficult for detecting lipid microvesicles,25 whereas there may be a large amount of microsteatosis in the liver. It has been noted in the literature that H&E staining underestimates hepatic steatosis by roughly 75%, and the combination of oil red O staining and digital image analysis allows for a more accurate assessment of it.26 As such, this methodology used in the present study has complemented the description of lipoatrophy, where lipid-containing areas were shown to be characteristically red in color under a microscope (Fig. 3b). The results of image analysis suggested that the relative area of lipid droplets was significantly higher in the F and FA groups than in the other treatment groups except for the ice wine group (Fig. 3d), which suggested that the addition of succinic acid and malic acid significantly reduced the increase in the lipid area brought about by the intake of fructose alone.
The intestine, which is a digestive and absorptive organ, could be affected by any morphological alteration. The villus height, crypt depth and villus-crypt ratio are believed to directly reflect growth and development and are closely related to the digestive and absorptive capacity of the small intestine.27,28 In Fig. 4c, only the FA group showed a significantly shorter villus length than that of the control group, while those in the other treatment groups were slightly shortened but the difference was not significant. The shortened intestinal villi contributed directly to the reduced intestinal surface area, triggering impaired absorption and a negative effect on material exchange. Meanwhile, whereas the jejunal crypts were significantly deepened in the F and FA groups of mice, the villus-crypt ratios were markedly lower than those in the control, FS and FM groups. The villus height/crypt depth provides an invaluable criterion for assessing the possible digestive capacity of the small intestine. Reduction of the villus height/crypt depth is considered to be detrimental to digestion and absorption.27,29 Also, the thickness of the jejunal muscularis propria was reduced significantly in mice ingesting fructose, while the simultaneous ingestion of succinate or malic acid significantly ameliorated the extent of its reduction, even though the final thickness was still significantly lower than that of the control group, which implies an alteration in the thickness of the intestinal barrier. In addition, for small bowel length measurements (Fig. 4d), ingestion of ice wine, pure fructose, fructose and succinate, fructose and alcohol all resulted in significant reductions in the length of the small bowel, demonstrating poor growth of the small bowel, which is usually associated with morphological alterations of the intestinal epithelium and affects intestinal hydrolysis and absorptive function.27
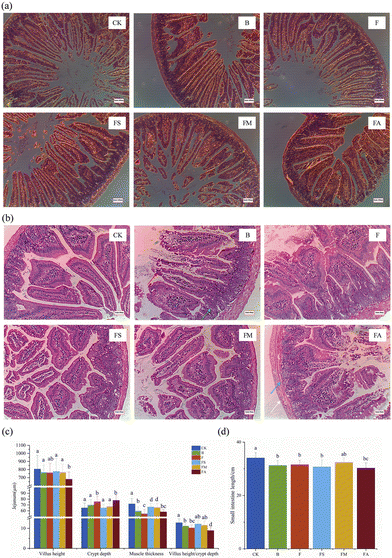 |
| Fig. 4 Effects of organic acids on the histomorphology of the mice jejunum. (a) H&E-stained section of the jejunum (100× microscope field of view); (b) H&E-stained section of the jejunum (200× microscope field of view); (c) effects of organic acids on the villus height, crypt depth and muscle thickness of the mice jejunum; and (d) length of the small intestine. CK, control group; B, ice wine group; F, fructose group; FS, fructose + succinic acid group; FM, fructose + malic acid group; FA, fructose + alcohol group. Red arrows: villus; green arrows: crypt; blue arrows: muscularis; yellow arrows: serous coat. | |
While the colons of mice ingesting pure water, ice wine, fructose-succinic acid, and fructose-malic acid presented no morphological signs of damage or inflammation, significant proliferation of immune tissues was observed in group F. Extensive damage was caused to the colonic mucosa of mice in groups F and A (Fig. 5a). Simultaneously, the depth of crypts was significantly increased and the muscle thickness was significantly reduced in group F compared to controls. There was a deepening of the depth of the crypts of the colon and a decrease in the thickness of the muscularis propria in the mice of groups B and FS. Overall, the presence of organic acids suppressed negative histomorphological changes in mice, such as the development of an inflammatory response in the liver, the increase in lipid areas, the shortening of the small intestine, the increase in the crypt depth of the jejunum and colon, and the thinning of the muscularis propria.
 |
| Fig. 5 Effects of organic acids on the histomorphology of the mice colon. (a) H&E-stained section of the colon (100× microscope field of view); (b) H&E-stained section of the colon (200× microscope field of view); (c) effects of organic acids on the crypt depth and muscle thickness of the mice colon. CK, control group; B, ice wine group; F, fructose group; FS, fructose + succinic acid group; FM, fructose + malic acid group; FA, fructose + alcohol group. Yellow arrows: serous coat; red arrows: lymphatic tissue; blue arrows: muscularis; black arrows: lamina propria; green arrows: crypt; white arrows: goblet cell. | |
Effects of organic acids on plasma and hepatic biochemical indices in mice
Fig. 6a–g show the indicators related to lipid metabolism in mice plasma and Fig. 6h–k show those related to glucose metabolism. As essential clinical parameters, plasma TC and TG were slightly higher in group F compared to those in the control group. Ice wine also resulted in increased TG but slightly decreased TC levels. The presence of succinic acid, malic acid and alcohol decreased TC and TG levels due to fructose, and succinic acid was significant in decreasing TC and alcohol was significant in decreasing TG, respectively (Fig. 6a and b). This indicated that succinic acid, malic acid and alcohol contributed to the amelioration of the elevated levels of plasma TC and TG in mice introduced by the ingestion of fructose. However, consumption of fructose alone resulted in a significant increase in plasma HDL-C levels, which may be an adaptive response of the organism to the stress of a high-fructose diet alone (Fig. 6c and d). AST and ALT are sensitive indicators of liver injury. There was no significant difference in plasma ALT levels between treatment groups. Significantly higher AST levels were found in F and FM groups. Calculations for AST/ALT demonstrated that the ratio was obviously higher in the F group, which confirmed the occurrence of hepatic injury, whereas succinic acid, malic acid, and alcohol could alleviate the injury to a certain extent (Fig. 6e–g).
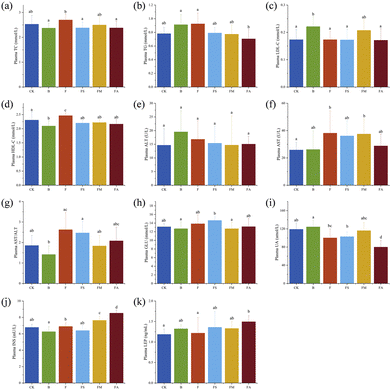 |
| Fig. 6 Effects of organic acids on plasma biochemical indices in mice. (a) Plasma TC level; (b) plasma TG level; (c) plasma LDL-C level; (d) plasma HDL-C level; (e) plasma ALT activity; (f) plasma AST activity; (g) plasma AST/ALT level; (h) fasting plasma glucose level; (i) plasma UA level; (j) fasting plasma insulin level; and (k) plasma LEP level. n = 9. CK, control group; B, ice wine group; F, fructose group; FS, fructose + succinic acid group; FM, fructose + malic acid group; FA, fructose + alcohol group. | |
Fasting blood glucose, uric acid, insulin and leptin in mice were changed by the various treatments (Fig. 6h–k). The slight elevation of blood glucose and insulin levels in group F indicates that high-dose fructose intake does not significantly affect fasting blood glucose in normally fed animals, which is consistent with previous findings.16 But fasting blood glucose in the FS group and fasting insulin levels in the FM and FA groups were remarkably higher than those in the control group, suggesting that succinic acid suppressed the function of pancreatic islets to a certain extent, while malic acid and alcohol promoted insulin secretion compared with pure fructose solutions of the same concentration. The tests for uric acid suggested that a 10-week period of ice wine consumption did not significantly elevate uric acid levels, whereas pure fructose, fructose-succinate, and fructose-alcohol even significantly reduced uric acid levels, and that hypouricaemia probably developed, which requires further study.
Fig. 7a–e present the indicators related to lipid metabolism in mouse plasma, and Fig. 7f–h present those related to liver injury. Addition of succinic acid significantly reduced hepatic TC levels and the addition of malic acid and alcohol significantly reduced hepatic TG levels in the associated mice compared to fructose intake alone. Meanwhile, fructose-succinic acid intake significantly increased hepatic LDL-C levels, which was consistent with the highest body weight gain in the FS group of mice. With multiple beneficial functions, HDL-C is an inverse predictor of many metabolism-related diseases,30 and significantly higher levels of HDL-C in the FS and FM groups implied the positive significance of the consumption of succinic acid and malic acid. In Fig. 7g, AST and ALT ratios were found to be notably increased by fructose treatment, while no significant changes were observed in any of the other treatment groups, suggesting that a 10-week period of ice wine intake did not lead to liver injury and that succinic acid, malic acid, and alcohol significantly improved the liver injury associated with pure fructose, which is consistent with the results of serum analyses.
 |
| Fig. 7 Effects of organic acids on hepatic biochemical indices in mice. (a) Hepatic TC level; (b) hepatic TG level; (c) hepatic LDL-C level; (d) hepatic HDL-C level; (e) hepatic ALT activity; (f) hepatic AST activity; (g) hepatic AST/ALT level; and (h) hepatic BCA level. n = 9. CK, control group; B, ice wine group; F, fructose group; FS, fructose + succinic acid group; FM, fructose + malic acid group; FA, fructose + alcohol group. | |
Effects of organic acids on the intestinal flora of mice
As the core part of intestinal microecology, the intestinal immune system matures with the development of intestinal flora, and dynamic and complex interactions exist between host health and the intestinal microbiota.28 A variety of environmental factors, including diet, affect the composition of the intestinal flora.31 Shannon index and coverage index dilution curves were plotted to assess the quality and quantity of sequenced 16S rRNA genes in the colonic contents of mice in different treatment groups. With the increasing number of samples sequenced, the Shannon index reached a plateau (Fig. 8a), denoting good sequencing coverage. All coverage indices were higher than 0.99 (Fig. 8b), which means that the determination results could realistically reflect the microbial communities in the samples.
 |
| Fig. 8 Effects of organic acids on the intestinal flora of mice. (a) Alpha sample dilution curve (Shannon); (b) alpha sample dilution curve (coverage); (c) differences between alpha diversity groups (chao); (d) differences between beta diversity groups; (e) species composition (class); (f) species composition (genus): species not annotated at this taxonomic level and with an abundance of less than 0.5% of the sample were combined into others; (g) comparison of key species differences (class); and (h) comparison of key species differences (genus). *: p < 0.05, **: p < 0.01, and ***: p < 0.001. n = 6. CK, control group; B, ice wine group; F, fructose group; FS, fructose + succinic acid group; FM, fructose + malic acid group; FA, fructose + alcohol group. | |
The Chao index, which captures the abundance of microbial communities in a sample, showed that all treatment groups increased the alpha diversity of the mouse intestinal flora compared to the control group, with the highest increase observed in the FM group, followed by the B group (Fig. 8c). Also, all treatments reduced the beta diversity of the intestinal flora of mice compared to the control group, with the highest reduction observed with the addition of succinic acid (Fig. 8d).
As shown in Fig. 8e and g, the dominant intestinal flora at the phylum level in mice across all studied groups were Bacillota, Bacteroidota, Verrucomicrobiota, and Pseudomonadota. Significant differences in the composition of the flora in groups F and B were noted compared to the CK group. The relative abundance of Bacillota was markedly increased (p < 0.01), and that of Bacteroidota (p < 0.01) and Verrucomicrobiota was decreased. Bacillota abundance was also significantly increased (p < 0.01) and there was a substantial reduction in Bacteroidota (p < 0.01) due to chronic consumption of fructose-alcohol, fructose-malate, and fructose-succinate as compared to the CK group. As the primary constituents of intestinal flora, the Bacillota and Bacteroidota ratio, formally referred to as Firmicutes and Bacteroidetes, respectively, are the key indicators of flora balance.32 The ratio is normally maintained within a relatively stable range under normal physiological conditions to ensure a balanced and healthy intestinal microbial environment. It was higher than the control group in all other treatment groups and in descending order CK (0.50), B (0.80), F (0.92), FS (1.35), FM (1.70) and FA (1.99), implicating the presence of disturbed intestinal flora.
At the generic level, Duncaniella, Muribaculum, Prevotellamassilia, Akkermansia and Allobaculum were the major flora existing in the intestinal tract in this study (Fig. 8f and h). The CK group showed remarkable differences in Duncaniella (p < 0.001), Muribaculum (p < 0.01), and Allobaculum (p < 0.01) when compared to the other groups. In particular, only Allobaculum abundance was enhanced in the F, FA and FM groups in comparison with the CK group, while the abundance of the other four genera was reduced. While prolonged consumption of ice wine had no significant effects on most genera, the biggest difference was recorded in Akkermansia.
Effects of organic acids on the relative expression levels of genes associated with hepatic glucolipid metabolism
The liver is a major target organ for the regulation of glycolipid metabolism of the body.33 In this study, PCR was performed for the mRNA expression levels of seven major genes related to glycolipid metabolism (Fig. 9), including acetyl-CoA-carboxylase 1 (ACC1), sterol regulatory element-binding protein 1 (SREBP-1c), carnitine palmitoyltransferase-1α (CPT-1α), phosphoenolpyruvate carboxylase (PEPCK), carbohydrate-responsive element–binding protein-β (ChREBP-β), peroxisome proliferator–activated receptor-α (PPAR-α) and ketohexokinase-C (KHK-C).
 |
| Fig. 9 Effects of organic acids on the relative expression levels of genes associated with hepatic glucolipid metabolism. (a) ACC1; (b) SREBP-1c; (c) CPT-1α; (d) PEPCK; (e) ChREBP-β; (f) PPAR-α; and (g) KHK-C. n = 9. CK, control group; B, ice wine group; F, fructose group; FS, fructose + succinic acid group; FM, fructose + malic acid group; FA, fructose + alcohol group. | |
Among them, ACC1, a protein in the cytoplasm, is the rate-limiting enzyme in the fatty acid de novo synthesis pathway and serves as the hub of the metabolic network related to fatty acid synthesis.34 In Fig. 9a, both high fructose and high fructose-alcohol intake significantly enhanced the relative mRNA expression level of hepatic ACC1, whereas the intake of high fructose along with succinate or malate significantly suppressed the increase in expression, while chronic consumption of ice wine did not result in hepatic overexpression of ACC1. ACC1 stimulates very low-density hepatic lipoprotein secretion and causes elevated TG levels, triggering dyslipidaemia by activating SREBP-1c.35 SREBP-1c is a key transcriptional activator regulating hepatic lipogenesis, which is involved in cellular lipid homeostasis and cholesterol biosynthesis, and the aberrant expression of SREBP-1c is associated with the development of fatty liver.36 Ten weeks of ice wine intake resulted in a significant increase in the relative mRNA expression of SREBP-1c (Fig. 9b), which was significantly reduced by succinate, malate or alcohol, whereas fructose intake alone only slightly increased the expression compared to drinking pure water. In comparison with the results of plasma and liver TC and TG content measurements, changes in the mRNA expression of SREBP-1c did not affect lipid accumulation accordingly, and it was speculated that the complexity of the organism and the expression of other indices also affected lipid accumulation.
ChREBP is another transcriptional activator of hepatic lipogenesis with two primary isoforms, ChREBP-α and ChREBP-β. Fructose metabolism in the liver is mainly regulated by ChREBP-β. A significant increase in the relative mRNA expression level of ChREBP-β was observed in this study in groups F and FA; a slight increase in group B and a slight decrease in groups FS and FM compared to group CK (Fig. 9e) were observed, indicating that fructose consumption upregulated ChREBP-β, which is in agreement with previous findings.37 Simultaneous exposure to succinic acid, malic acid, or alcohol suppressed the expression increase brought about by fructose intake alone.
The inhibition of fatty acid oxidation by ChREBP could be achieved by down-regulating enzyme activities such as CPT-1α.38 In Fig. 9c, group F with significantly higher relative expression levels of ChREBP-β showed significantly lower CPT-1α, but no consistent negative correlation was observed across all groups; for example, the FS group expressed the lowest mRNA level of CPT-1α but the lowest ChREBP-β. The suppression of fatty acid oxidation by ChREBP also occurs via antagonism of PPAR-α,38 which is highly expressed in hepatocytes and acts as one of the major participants in hepatic lipid homeostasis, essential for mitochondrial biosynthesis and fatty acid oxidation.39Fig. 9f presents the relative mRNA expression levels of PPAR-α in various groups. It can be seen that the level of PPAR-α notably increased in the ice wine-treated group, did not change markedly in the F group, and reduced significantly in the FS, FM and FA groups. It suggests that other components in ice wine, except fructose, succinate, malate and alcohol, promote the mRNA expression of PPARα. Meanwhile, activated PPAR-α up-regulates the expression of the rate-limiting enzyme CPT-1α, which promotes β-oxidation and degradation of fatty acids.40 But the actual expression levels did not positively correlate with CPT-1α in equal proportions, nor negatively correlate with ChREBP-β in equal proportions, which once again reflects the complexity of the organism.
In addition, an inverse relationship was also demonstrated between KHK-C and CPT-1α.41 KHK-C, expressed in metabolic tissues such as the liver, kidney and intestine, is believed to have high affinity for fructose and metabolises fructose to fructose-1-phosphate. Excess fructose enhances adipogenesis by promoting KHK-C to form a substrate for fatty acid synthesis.38 The expression of KHK-C in group FS was notably higher than that in CK, B and F. There was no significant difference among the latter three groups.
The FA group exhibited markedly higher levels than the FS and FM groups. Elevated KHK-C mediates endoplasmic reticulum stress, leading to metabolic dysfunction,42 and thus, concomitant consumption of fructose and alcohol contributes to metabolic abnormalities.
Additionally, the level of PEPCK, which plays a crucial role in gluconeogenesis, glycolysis, and the tricarboxylic acid cycle, is exemplified in Fig. 9d. PEPCK is widely known to be a key and rate-limiting enzyme in the process of gluconeogenesis.43 The livers of mice in the treatment group ingesting fructose with concomitant addition of succinic acid, malic acid and alcohol showed a significant increase in the relative mRNA expression level of PEPCK, suggesting the promotion of gluconeogenesis.
Overall, long-term continuous consumption of ice wine did not cause obvious modifications in the mRNA expression of ACC1, PEPCK, ChREBP-β and KHK-C in C57BL/6J male mice, but significantly increased the mRNA expression of PPAR-α. PPAR-α is able to attenuate hepatic lipid deposition and hepatocyte lipid accumulation by regulating the levels of fibroblast growth factor 21 (FGF21), a regulator of glycolipid metabolism, and hypoxia-inducible factor 1 alpha (HIF-1α), and also activates the bile acid-regulating receptor farnesol X receptor (FXR), which decreases triglyceride synthesis and reduces hepatic lipid accumulation. The presence of organic acids significantly reduced the relative mRNA expression of ACC1, SREBP-1c, and ChREBP-β in mouse livers. Thus, at the transcriptional level, ice wine probably impacts PPAR-α to promote fatty acid β oxidation and degradation, while organic acids significantly ameliorate hepatic lipogenesis from fructose intake.
Effects of organic acids on the relative expression of proteins related to glucolipid metabolism in the liver
Furthermore, the effects of organic acids on the expression of proteins related to hepatic glycolipid metabolism were investigated (Fig. 10). Ice wine, fructose-alcohol and fructose consumption alone did not induce significant effects on ACC1 protein expression, but it was significantly reduced in the FS group and increased in the FM group (Fig. 10a). Also, the hepatic SREBP-1c and PPAR-α protein expression were lower in the FS and FA groups of mice compared to the control group (p < 0.05, Fig. 10b and f), with a consistent trend of PPAR-α protein expression and relative mRNA expression levels. FM and FA groups exhibited significantly higher CPT-1α and PEPCK protein expression, respectively, compared with the other groups (Fig. 10c and d). The protein expression of ChREBP-β was found to be significantly lower in the F and FS groups compared to that in the other groups (Fig. 10e). KHK-C protein expression was significantly higher in the group F compared to the group CK and lower in the group FA. The trends of ACC1, SREBP-1c and ChREBP-β in relation to hepatic TC and TG levels were similar between the groups.
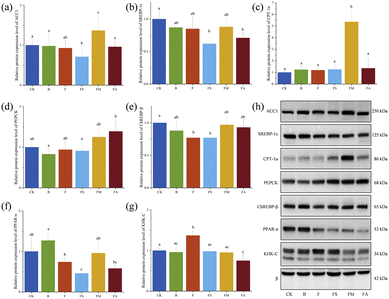 |
| Fig. 10 Effects of organic acids on relative expression of proteins related to glucolipid metabolism in the liver. (a) ACC1; (b) SREBP-1c; (c) CPT-1α; (d) PEPCK; (e) ChREBP-β; (f) PPAR-α; (g) KHK-C; and (h) western blotting of proteins related to glucolipid metabolism in the liver. n = 9. CK, control group; B, ice wine group; F, fructose group; FS, fructose + succinic acid group; FM, fructose + malic acid group; FA, fructose + alcohol group. | |
In general, long-term continuous consumption of ice wine did not bring significant differences in protein expression of the seven genes associated with glycolipid metabolism, and fructose intake alone significantly increased the KHK-C protein expression and significantly decreased ChREBP-β and PPAR-α protein expression. Succinic acid supplementation reduced the protein expression of PPAR-α and KHK-C (p < 0.05). Malic acid addition, on the other hand, significantly increased ACC1 and CPT-1α expression while significantly decreasing the KHK-C protein expression. Additional alcohol supplementation markedly reduced KHK-C protein expression only. Thus, at the protein level, the adverse events associated with prolonged exposure to fructose mainly occur through a possible increase in gluconeogenesis and inhibition of fatty acid degradation. The management of the adverse effects of fructose by organic acids was demonstrated by the reduction of hepatic fat synthesis by controlling the protein expression of KHK-C, and malic acid also contributed to the β-oxidation and degradation of fatty acids.
Conclusions
In conclusion, a 10-week continuous intervention confirmed that ice wine did not cause significant lipid accumulation and organ damage in mice and could promote fatty acid β-oxidation and degradation by affecting PPAR-α, but it somewhat reduced systemic insulin sensitivity and triggered mild liver inflammation and small intestinal shortening. Chronic fructose exposure caused undesirable accumulation of WAT, significant reduction of BAT and the development of hepatic inflammation and injury, an increase in lipid areas, shortening of the small intestine, an increase in the depth of the crypts of the jejunum and colon, thinning of the muscularis propria, and elevation of undesirable biochemical markers (e.g., hepatic TG), which were ameliorated by the organic acids. Also, ingestion of these substances increased alpha diversity, decreased beta diversity, and altered the dominant intestinal flora in mice. The side effects of fructose might be caused by increased gluconeogenesis and glycolysis and inhibition of fatty acid degradation, whereas the improvement of organic acids was achieved by decreasing the mRNA expression of hepatic ACC1, SREBP-1c, and ChREBP-β, as well as controlling the protein expression of KHK-C to reduce hepatic fat synthesis. In conclusion, it was confirmed that long-term consumption of ice wine did not induce disorders of glycolipid metabolism and that the high content of organic acids such as malic and succinic acids in ice wine inhibited the negative effects of fructose to a certain extent.
Author contributions
Yang Liu: data curation, formal analysis, investigation, methodology, validation, visualization, and writing – original draft. Fuliang Han: conceptualization, funding acquisition, supervision, and writing – review & editing. Xinyuan Ma: methodology. Luye Yang: methodology and data curation. Zhenan Shi: methodology.
Data availability
The data supporting this article have been included as part of the ESI.†
Conflicts of interest
There are no conflicts of interest to declare.
Acknowledgements
This work was supported by the Science and Technology Program of Tumxuk City, Third Division, Xinjiang Uygur Autonomous Region, China, through grant number KY2024JBGS06 and the Key Research and Development Projects of Shaanxi Province, China, through grant number 2023-GHZD-39.
References
- C. Jang, S. Hui, W. Lu, A. J. Cowan, R. J. Morscher, G. Lee, W. Liu, G. J. Tesz, M. J. Birnbaum and J. D. Rabinowitz, The small intestine converts dietary fructose into glucose and organic acids, Cell Metab., 2018, 27, 351–361 CrossRef CAS PubMed.
- A. Alim, T. Li, T. Nisar, Z. Ali, D. Ren, Y. Liu and X. Yang, Polyphenols and pectin enriched golden kiwifruit (actinidia chinensis) alleviates high fructose-induced glucolipid disorders and hepatic oxidative damage in rats: in association with improvement of fatty acids metabolism, Food Sci. Hum. Wellness, 2023, 12, 1872–1884 CrossRef CAS.
- Y. Lu, Y. Wu, X. Chen, X. Yang and H. Xiao, Water extract of shepherd's purse prevents high-fructose induced-liver injury by regulating glucolipid metabolism and gut microbiota, Food Chem., 2021, 342, 128536 CrossRef CAS PubMed.
- H. Hattori, Y. Hanai, Y. Oshima, H. Kataoka and N. Eto, Excessive intake of high-fructose corn syrup drinks induces impaired glucose tolerance, Biomedicines, 2021, 9, 541 CrossRef CAS PubMed.
- S. T. Im, H. Mun, S. Park, H. Kang, W. C. Kim, S. Heo and S. Lee, Ishige okamurae Celluclast extract ameliorates non-alcoholic fatty liver in high-fructose diet-fed mice by modulation of lipid metabolism and gut microbiota composition, Food Chem. Toxicol., 2023, 177, 113864 CrossRef CAS PubMed.
- C. S. Miranda, F. M. Silva-Veiga, D. A. Santana-Oliveira, A. Fernandes-Da-Silva, G. C. Brito, F. F. Martins and V. Souza-Mello, Chronic excessive fructose intake maximizes brown adipocyte whitening but causes similar white adipocyte hypertrophy than a high-fat diet in C57BL/6 mice, J. Am. Nutr. Assoc., 2023, 42, 435–444 CAS.
- J. F. de Carvalho and A. Lerner, Malic acid for the treatment of rheumatic diseases, Mediterr. J. Rheumatol., 2023, 34, 592–593 CrossRef PubMed.
- M. N. Mia, S. Z. Smrity, M. H. Bappi, H. Kamli, T. Islam, A. A. S. Prottay, M. S. Akbor, M. A. Latif, S. Islam, K. Bhakta, M. C. Shill, F. C. P. de Sousa, G. de Luna, H. D. M. Coutinho and M. T. Islam, Anxiolytic-like effect of succinic acid: a possible GABAergic intervention, Food Biosci., 2023, 55, 103044 CrossRef CAS.
- Y. J. Chen, T. Y. Zhang, L. Luo, Y. Q. Shi, F. J. Bai and D. N. Jiang, Impact of dietary l–malic acid supplementation on growth, feed utilization, ash deposition, and hepatic lipid metabolism of juvenile genetically improved farmed tilapia, Oreochromis niloticus, J. World Aquacult. Soc., 2017, 48, 563–573 CrossRef CAS.
- H. O. Santos, W. M. A. M. de Moraes, G. A. R. Da Silva, J. Prestes and B. J. Schoenfeld, Vinegar (acetic acid) intake on glucose metabolism: a narrative review, Clin. Nutr. ESPEN, 2019, 32, 1–7 CrossRef PubMed.
- F. de Vadder, P. Kovatcheva-Datchary, C. Zitoun, A. Duchampt, F. Bäckhed and G. Mithieux, Microbiota-produced succinate improves glucose homeostasis via intestinal gluconeogenesis, Cell Metab., 2016, 24, 151–157 CrossRef CAS PubMed.
- E. Grilli, M. R. Messina, M. Tedeschi and A. Piva, Feeding a microencapsulated blend of organic acids and nature identical compounds to weaning pigs improved growth performance and intestinal metabolism, Livest. Sci., 2010, 133, 173–175 CrossRef.
- F. Sinesio, E. Moneta, S. D. Marzo, G. P. Zoboli and S. Abbà, Influence of wine traits and context on liking, intention to consume, wine-evoked emotions and perceived sensory sensations, Food Qual. Prefer., 2021, 93, 104268 CrossRef.
- K. Tang, J. Li, B. Wang, L. Ma and Y. Xu, Evaluation of nonvolatile flavor compounds in vidal icewine from China, Am. J. Enol. Vitic., 2013, 64, 110–117 CrossRef CAS.
- A. Robles, M. Fabjanowicz, T. Chmiel and J. Płotka-Wasylka, Determination and identification of organic acids in wine samples. Problems and challenges, TrAC, Trends Anal. Chem., 2019, 120, 115630 CrossRef CAS.
- X. Ma, Y. Liu, F. Han, T. Cheng, K. Wang and Y. Xu, Effect of short-term moderate intake of ice wine on hepatic glycolipid metabolism in C57BL/6J mice, Food Funct., 2024, 15, 5063–5072 RSC.
- P. Li, Y. Gao, C. Wang, C. Zhang, X. Guo and D. Xiao, Effect of ILV6 deletion and expression of aldB from Lactobacillus plantarum in Saccharomyces uvarum on diacetyl production and wine flavor, J. Agric. Food Chem., 2018, 66, 8556–8565 CrossRef CAS PubMed.
- C. Nagy and E. Einwallner, Study of in vivo glucose metabolism in high-fat diet-fed mice using oral glucose tolerance test (OGTT) and insulin tolerance test (ITT), J. Visualized Exp., 2018, 131, e56672 Search PubMed.
-
Á. Vinué and H. González-Navarro, Glucose and insulin tolerance tests in the mouse, in Methods in molecular biology, Springer, New York: USA, 2015, vol. 1339, pp. 247–254 Search PubMed.
- A. Dudele, G. M. Rasmussen, D. Mayntz, H. Malte, S. Lund and T. Wang, Effects of ambient temperature on glucose tolerance and insulin sensitivity test outcomes in normal and obese C57 male mice, Physiol. Rev., 2015, 3, e12396 Search PubMed.
- L. Cheng, J. Wang, H. Dai, Y. Duan, Y. An, L. Shi, Y. Lv, H. Li, C. Wang, Q. Ma, Y. Q. Li, P. F. Li, H. F. Du and B. S. Zhao, Brown and beige adipose tissue: a novel therapeutic strategy for obesity and type 2 diabetes mellitus, Adipocyte, 2021, 10, 48–65 CrossRef CAS PubMed.
- B. Cannon and J. Nedergaard, Brown adipose tissue: function and physiological significance, Physiol. Rev., 2004, 84, 277–359 CrossRef CAS PubMed.
- A. Bartelt and J. Heeren, Adipose tissue browning and metabolic health, Nat. Rev. Endocrinol., 2014, 10, 24–36 CrossRef CAS PubMed.
- A. H. Fischer, K. A. Jacobson, J. Rose and R. Zeller, Hematoxylin and eosin staining of tissue and cell sections, Cold Spring Harb. Protoc., 2008, 6, 4986 CrossRef PubMed.
- M. Catta-Preta, L. S. Mendonca, J. Fraulob-Aquino, M. B. Aguila and C. A. Mandarim-De-Lacerda, A critical analysis of three quantitative methods of assessment of hepatic steatosis in liver biopsies, Virchows Arch., 2011, 459, 477–485 CrossRef PubMed.
- A. P. Levene, H. Kudo, M. R. Thursz, Q. M. Anstee and R. D. Goldin, Is oil red-O staining and digital image analysis the gold standard for quantifying steatosis in the liver?, Hepatology, 2010, 51, 1859 CrossRef PubMed.
- L. Montagne, J. R. Pluske and D. J. Hampson, A review of interactions between dietary fibre and the intestinal mucosa, and their consequences on digestive health in young non-ruminant animals, Anim. Feed Sci. Technol., 2003, 108, 95–117 CrossRef.
- Y. Liu, I. Fernandes, N. Mateus, H. Oliveira and F. Han, The role of anthocyanins in alleviating intestinal diseases: A mini review, J. Agric. Food Chem., 2024, 72, 5491–5502 CrossRef CAS PubMed.
- E. A. Jensen, J. A. Young, J. Kuhn, M. Onusko, J. Busken, E. O. List, J. J. Kopchick and D. E. Berryman, Growth hormone alters gross anatomy and morphology of the small and large intestines in age- and sex-dependent manners, Pituitary, 2022, 25, 116–130 CrossRef CAS PubMed.
- B. J. Ansell, K. E. Watson, A. M. Fogelman, M. Navab and G. C. Fonarow, High-density lipoprotein function, J. Am. Coll. Cardiol., 2005, 46, 1792–1798 CrossRef CAS PubMed.
- M. Horie, T. Miura, S. Hirakata, A. Hosoyama, S. Sugino, A. Umeno, K. Murotomi, Y. Yoshida and T. Koike, Comparative analysis of the intestinal flora in type 2 diabetes and nondiabetic mice, Exp. Anim., 2017, 66, 405–416 CrossRef CAS PubMed.
- A. Oren and G. M. Garrity, Valid publication of the names of forty-two phyla of prokaryotes, Int. J. Syst. Evol. Microbiol., 2021, 71, 005056 Search PubMed.
- Z. Shan, H. Zhang, C. He, Y. An, Y. Huang, W. Fu, M. Wang, Y. Du, J. Xie, Y. Yang and B. S. Zhao, High-protein mulberry leaves improve glucose and lipid metabolism via activation of the PI3k/akt/PPARα/CPT-1 pathway, Int. J. Mol. Sci., 2024, 25, 8726 CrossRef CAS PubMed.
- Y. Wang, W. Yu, S. Li, D. Guo, J. He and Y. Wang, Acetyl-CoA carboxylases and diseases, Front. Oncol., 2022, 12, 836058 CrossRef CAS PubMed.
- J. Jiang, S. Meng, L. Li, X. Duan, H. Xu and S. Li, Correlation of acetyl–coenzyme a carboxylase 1 with Th17 and Th1 cells, serving as a potential prognostic biomarker for acute ischemic stroke patients, J. Clin. Lab. Anal., 2022, 36, e24607 CrossRef CAS PubMed.
- E. H. Lee, J. H. Lee, D. Y. Kim, Y. S. Lee, Y. Jo, T. Dao, K. E. Kim, D. K. Song, J. H. Seo, Y. K. Seo, J. K. Seong, C. Moon, E. Han, M. K. Kim, S. Ryu, M. Shin, G. S. Roh, H. R. Jung, T. F. Osborne, D. Ryu, T. I. Jeon and S. S. Im, Loss of SREBP-1c ameliorates iron-induced liver fibrosis by decreasing lipocalin-2, Exp. Mol. Med., 2024, 56, 1001–1012 CrossRef CAS PubMed.
- A. Bier, E. Shapira, R. Khasbab, Y. Sharabi, E. Grossman and A. Leibowitz, High-fructose diet increases renal ChREBPβ expression, leading to intrarenal fat accumulation in a rat model with metabolic syndrome, Biology, 2022, 11, 618 CrossRef CAS PubMed.
- S. A. Hannou, D. E. Haslam, N. M. Mckeown and M. A. Herman, Fructose metabolism and metabolic disease, J. Clin. Invest., 2018, 128, 545–555 CrossRef PubMed.
- R. Kumari, A. G. Ray, D. Mukherjee, V. Chander, D. Kar, U. S. Kumar, P. V. P. D. Bharadwaj, S. K. Banerjee, A. Konar and A. Bandyopadhyay, Downregulation of PTEN promotes autophagy via concurrent reduction in apoptosis in cardiac hypertrophy in PPAR α−/− mice, Front. Cardiovasc. Med., 2022, 9, 798639 CrossRef CAS PubMed.
- X. Chu, Y. Zhou, S. Zhang, S. Liu, G. Li and Y. Xin, Chaetomorpha linum polysaccharides alleviate NAFLD in mice by enhancing the PPARα/CPT-1/MCAD signaling, Lipids Health Dis., 2022, 21, 140 CrossRef CAS PubMed.
- R. N. Helsley, S. Park, H. J. Vekaria, P. G. Sullivan, L. R. Conroy, R. C. Sun, M. D. M. Romero, L. Herrero, J. Bons, C. D. King, J. Rose, J. G. Meyer, B. Schilling, C. R. Kahn and S. Softic, Ketohexokinase-c regulates global protein acetylation to decrease carnitine palmitoyltransferase 1a-mediated fatty acid oxidation, J. Hepatol., 2023, 79, 25–42 CrossRef CAS PubMed.
- S. H. Park, R. N. Helsley, T. Fadhul, J. Willoughby, L. Noetzli, H. C. Tu, M. H. Solheim, S. Fujisaka, H. Pan, J. M. Dreyfuss, J. Bons, J. Rose, C. D. King, B. Schilling, A. J. Lusis, C. Pan, M. Gupta, R. N. Kulkarni, N. Rohit, K. Fitzgerald, P. A. Kern, S. Divanovic, C. R. Kahn and S. Softic, Fructose induced KHK-c increases ER stress and modulates hepatic transcriptome to drive liver disease in diet-induced and genetic models of NAFLD, BioRxiv, 2023 Search PubMed.
- S. Xue, Y. Cai, J. Liu, K. Ji, P. Yi, H. Long, X. Zhang, P. Li and Y. Song, Dysregulation of phosphoenolpyruvate carboxykinase in cancers: A comprehensive analysis, Cell. Signalling, 2024, 120, 111198 CrossRef CAS PubMed.
|
This journal is © The Royal Society of Chemistry 2025 |
Click here to see how this site uses Cookies. View our privacy policy here.