DOI:
10.1039/C3QI00087G
(Review Article)
Inorg. Chem. Front., 2014,
1, 215-225
Hydroxyapatite nanocrystals: colloidal chemistry, assembly and their biological applications
Received
20th November 2013
, Accepted 17th January 2014
First published on 14th February 2014
Abstract
Hydroxyapatite (HAp) nanocrystals with excellent biocompatibility and bioresorbability are usually used in the fields of tissue engineering, medicine, etc. In this review, recent advances in the tunable synthesis, ion doping, assembly and applications of monodisperse HAp nanocrystals are summarized, which may be helpful for the designed synthesis and surface modification of HAp or other nanocrystals according to practical applications.
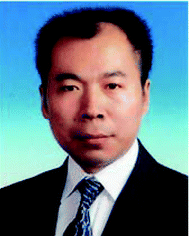 Junfeng Hui | Associate Professor Junfeng Hui received his Ph.D. degree in Biochemical Engineering from Northwest University in 2010, followed by working with Prof. Xun Wang in the Department of Chemistry, Tsinghua University as a postdoctoral fellow. He joined the faculty of Chemical Engineering School, Northwest University after his undergraduate study in 1998, and was promoted to associate professor in 2011. His main awards include the second prize for the National Award for Technological Invention (2013). His current research interests focus on the preparation and application of functional nanometer materials. |
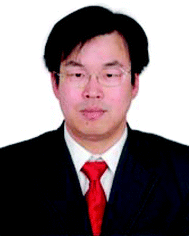 Xun Wang | Professor Xun Wang received his Ph.D. degree from the Department of Chemistry, Tsinghua University in 2004. Then he joined the faculty of the Department of Chemistry, Tsinghua University as a research assistant, and was promoted to associate professor and full professor in 2005 and 2007, respectively. His main awards include National Science and Technology Award for Young Scientist (2009), National Science Fund for Distinguished Young Scholars (2007) and IUPAC Prize for Young Chemists (2005). His current research interests include synthetic methodology, self-assembly and properties of inorganic nanocrystals. |
1. Introduction
As the main constituent of the inorganic phase in the human body, hydroxyapatite (HAp) has excellent biocompatibility, bioresorbability, osteogenesis, osteoconductivity and osteoinductivity, and easily forms direct chemical bonds with living tissue.1–4 Therefore, HAp plays extremely important and irreplaceable roles in the field of tissue engineering. In order to endow other materials with excellent biological and mechanical properties, HAp nanocrystals are often used as coating materials and the inorganic phase of organic/inorganic composites.5–8 HAp has many other applications. HAp spheres can be used as nano-carriers for controlled drug delivery.9 Nanoscale HAp could selectively inhibit the growth of cancer cells and is expected to become a novel green anticancer drug.10–12 In addition, HAp is a good medium to separate and purify sugars, amino acids, peptides, nucleic acids, enzymes, proteins, and other biological macromolecules in the non-acidic system.13–15 HAp powder can be used as a water treatment agent to remove heavy metal ions such as Cd2+, Hg2+, and Pb2+ based on ion-exchange reaction.16,17 HAp is usually used in catalysis as a supporting material. Recently, HAp was found to be an inexpensive and effective phase-pure catalyst for volatile organic compound combustion.18
Up to now, many methods have been reported for the preparation of HAp, such as (microwave-) hydrothermal, homogeneous precipitation, sol–gel, electrophoretic deposition, microemulsion, template methods, solid phase methods, and biomineralization.19–27 HAp with various shapes, sizes, stoichiometries, and levels of crystallinity can be obtained, depending on the synthetic technique. Foreign-ion doping further enriches the kinds and properties of HAp and extends their potential applications. For example, europium ions endow HAp crystals with bright fluorescence properties,28 and silver or zinc ion doping endows HAp crystals with antimicrobial and catalytic properties.29,30
HAp particles in native bone are nanoscale platelets or rods.1,2 However, most of the preparation of HAp crystals is fulfilled in the water phase, and the resulting particle size is too large, and/or the particles are prone to aggregate, limiting their effective application. The controlled synthesis of monodisperse hydroxyapatite nanocrystals is a continuing focus.
Herein, we mainly summarize our recent endeavors on the synthesis, assembly and application of monodisperse HAp nanorods, nano-spindles, nanowires, etc. The whole summary consists of four parts. Firstly, the Liquid-Solid-Solution (LSS) phase transfer and separation strategy31 is interpreted and applied in the preparation of HAp nanorods and fluorine-substituted (FHAp) nanotubes. Secondly, based on this or a modified strategy, synthesis and properties of monodisperse ion-doped HAp nanocrystals are reviewed. Thirdly, we describe the (self-) assembly of several kinds of monodisperse HAp nanocrystals and analyze the related mechanism of assembly. Finally, we introduce surface grafting modification and encapsulation methods, by which hydrophobic HAp nanocrystals are converted into hydrophilic ones. Meanwhile, the corresponding biological application experiment is described.
2. LSS phase transfer and separation strategy
In 2005, Wang et al. first presented a LSS phase transfer synthetic strategy for the synthesis of various functional nanocrystals with different chemistries and properties.31 As shown in Fig. 1, the LSS strategy reveals actually an oil–water interface-controlled reaction system which refers to three phases (liquid, solid, and solution phase) and two interfaces (liquid–solid and solid–solution interface). In this system, water, ethanol, a long chain fatty acid and its metal-salt form a relatively complex three-phase reaction system: a water–ethanol mixed solution acts as the main continuous solution phase dissolving starting inorganic salts, ethanol and a long chain fatty acid (like oleic acid or linoleic acid) serve as a liquid phase, and a fatty acid metal salt (sodium linoleate, sodium oleic acid, and so on) is a solid phase. Under the designated reaction conditions, the Mn+ ions of (RCOO)nM could be reduced to metal nanocrystals by ethanol, or dehydrate to oxides and/or composite oxides, or react with other anion species from the solution phase to yield a large variety of functional nanocrystals.32–39 The as-obtained product can be easily collected because of a spontaneous phase-separation process based on the weight of nanocrystals and/or incompatibility between their hydrophobic surfaces and hydrophilic surroundings. This strategy has been demonstrated to be effective in the synthesis of HAp nanocrystals with controllable sizes and shapes comparable with those of other colloidal nanocrystals.
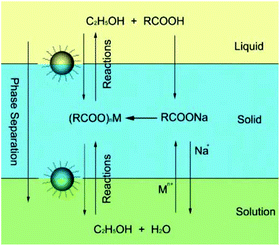 |
| Fig. 1 LSS strategy for nanocrystal synthesis. Adapted with permission from ref. 31, Copyright 2005, Nature Publishing Group. | |
2.1 LSS strategy for the synthesis of monodisperse HAp nanorods
Based on this LSS strategy, Wang et al. successfully fabricated uniform monodisperse HAp nanorods with tunable sizes, aspect ratios, and surface properties at 80–200 °C for about 8–10 h.40 As shown in Fig. 2, sodium linoleate (or sodium oleate), linoleic acid (or oleic acid), and ethanol were mixed together under agitation; then an aqueous solution of Ca(NO3)2 was added to form the liquid (ethanol/linoleic acid), solid (sodium linoleate), and solution (Ca(NO3)2 aqueous solution) phases. After the ion-exchange process of Ca2+ and Na+, a solution of Na3PO4 was added to the solution phase and reacted with the calcium linoleate. The as-obtained Hap nanocrystals have highly uniform sizes and narrow size- and length-distributions. By tuning the reaction temperatures, the surface properties can be further controlled, and the experimental results show that the HAp nanorods possess stable hydrophobic surface properties under higher-temperature conditions based on monolayer complexation of linoleic acid on the outer surface, or unstable hydrophilic ones under lower-temperature conditions which were attributed to the bilayer absorption of linoleic acid on the surfaces.
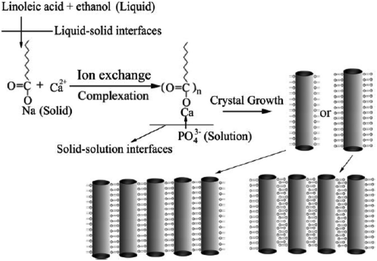 |
| Fig. 2 LSS strategy to HAp nanorods and their assembly. Adapted with permission from ref. 40, Copyright 2006, Wiley Online Library. | |
2.2 Synthesis of FHAp nanotubes based on Kirkendall effect
Compared with nanorods, single-crystal nanotube structures have better mechanical properties.41–45 Based on the LSS process and Kirkendall effect, Hui et al. synthesized monodisperse single-crystal F-substituted HAp (FHAp) nanotubes with different aspect ratios via a hydrothermal-based synthetic method (Fig. 3a–i).46 It was found that uniform nanotubes can be obtained when F− ions are added to the system before PO43− ions; in contrast, only nanorods with small pores on the surfaces can be obtained if PO43− ions are added before F− ions. The possible process was that Ca2+ ions and F− ions first generate CaF2, and then the CaF2 reacts with the absorbed PO43− ions to form CaF2 @ HAp. CaF2 gradually vanished with F− doped into the HAp lattice, so hollow interior space was left with the formation of nanotubes. The TEM images from the as-obtained nanotubes revealed that with the increase of F/Ca molar ratios from 0.033 to 0.25, the aspect ratios of HAp nanotubes would decrease, and the diameters would increase from 10 to 20 nm when the lengths decreased from 200 to 50 nm. Further study showed that the addition of the amphiphilic ligand polyethylene glycol (PEG, higher than 10
000, MW) to the reaction system would lead to formation of nanotubes with amphiphilic surface properties, which was attributed to the co-anchoring of PEG and hydrophobic oleic acid on the outer surface of the nanotubes.
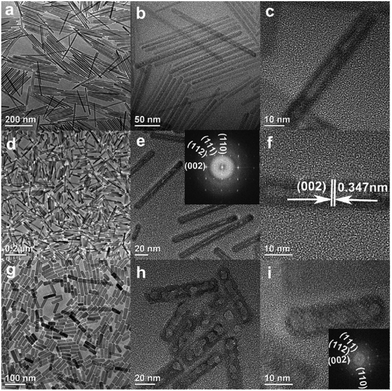 |
| Fig. 3 (a–i) Representative TEM and HRTEM images of FHAp nanotubes with different doping levels: a–c, F/Ca = 0.033, 100 °C; d–f, F/Ca = 0.05, 100 °C; g–i, F/Ca = 0.25, 100 °C. Adapted with permission from ref. 46, Copyright 2009, American Chemical Society. | |
F doping into the lattice of HAp will certainly improve their anti-erosion performance. To investigate the stability of FHAp nanotubes and pure HAp nanorods synthesized in the LSS system, they were immersed in the mixed solution of acetic acid and water for a certain period of time. The results showed that HAp nanorods were quickly destroyed and converted into dicalcium phosphate dihydrate (DCPD) hollow nanospheres with diameters of about 10–60 nm, while the structures of FHAp nanotubes remained nearly the same as that before erosion.46,47 All these indicate that these FHAp nanotubes are quite stable in acidic environments.
3. Doping chemistry of HAp nanocrystals
3.1 Rare-earth doped FHAp nano-spindles and/or nanowires
Rare-earth element doping was proved to be effective in the preparation of novel functional nanomaterials, which not only can effectively control the growth of the nanocrystals, but also endow the product with abundant performance for potential applications, such as optical communication, display devices, catalysis, biological labeling, etc.48–56 For the mixed solvent system of octadecylamine, oleic acid and water, different rare-earth ions (La3+, Ce3+, Nd3+, Eu3+, Gd3+, Tb3+, Er3+, Tm3+, Yb3+, etc.) and F− ions are chosen as doping ions. We found that the reaction temperature, pH value, and volume ratio of the mixed solvent were crucial to synthesize HAp nanostructures with different morphologies and properties. As shown in Fig. 4, when the mixture of 4 mL oleic acid, 16 mL ethanol, about 16 mL water and 0.5 g octadecylamine was used as the reaction system, and Ca(NO3)2·4H2O, Na3PO4, NaF, and rare-earth nitrates were used as starting materials, FHAp:Ln3+ (Ln = Ce, Eu, Tb, etc.) nano-spindles were easily prepared. Increasing the amount of fluorine ions led to smaller length-to-diameter ratios of the nano-spindles, and increasing the amount of rare-earth ions contributed to the preparation of nano-spindles with more sharp heads.57 In our previous work, rice-like FHAp nanocrystals were once reported as the intermediate in the process of crystal growth.46 Herein, it was apparent that the absorption of octadecylamine on the surface and the doping of rare-earth ions suppressed the growth along the c-axis and resulted in the formation of rice-like end-products. The experimental results also showed that the anion surfactant oleic acid could be preferable for the growth along the c-axis and rare-earth ions could further enhance the template function of oleic acid because of their having higher positive charge than calcium ions in the absence of octadecylamine. As shown in Fig. 5, when choosing a mixture of 20 mL of oleic acid, 8 mL of ethanol, 9 mL of water, and the right amount of NaOH as the reaction system, and when Ca(NO3)2·4H2O, Na3PO4, NaF, and rare-earth nitrates were likewise used as starting materials, the end-product could be dramatically tuned from rice-like nanocrystals to single-crystal ultrathin nanowires with diameters 3–4 nm and lengths up to 5–10 μm.57 The ionic radius of the Ln3+ ion is close to that of the Ca2+ ion, which well proved that different rare-earth elements would lead to similar results in the same reaction systems.
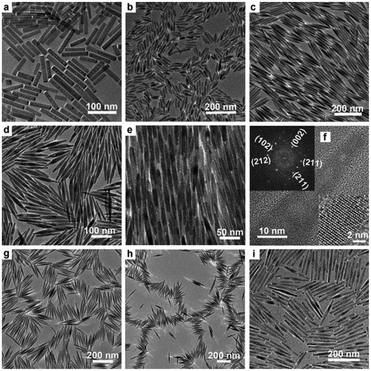 |
| Fig. 4 Representative TEM and HRTEM images of FHAp nanoparticles with different doping levels (molar ratio) in the reaction system composed of octadecylamine, oleic acid, ethanol, and water (F/Ca = 0.2, 150 °C, 12 h for all samples): (a) Eu/Ca = 0, (b) Eu/Ca = 2.5 : 97.5, (c–f) Eu/Ca = 5 : 95, (g) Tb/Ca = 5 : 95, (h) Ce/Ca = 5 : 95, and (i) Er/Ca = 20 : 80. The inset in (f) shows a local enlargement of the HRTEM image (bottom right corner) and the corresponding Fourier-transform diffractogram (top left corner). Adapted with permission from ref. 57, Copyright 2011, Wiley Online Library. | |
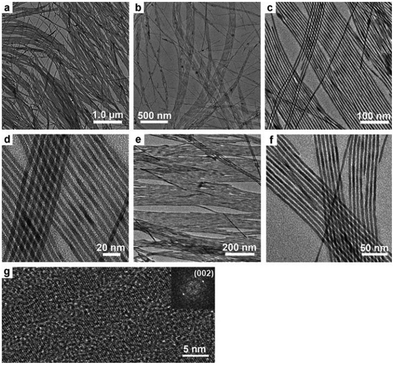 |
| Fig. 5 Representative TEM and HRTEM images of FHAp nanowires with different doping levels (molar ratio) at 180 °C for 36 h in the reaction system composed of NaOH, oleic acid, ethanol, and water: (a–d) 10% Yb3+, (e) 10% Er3+, and (f) 10% Tm3+ (F/Ca = 0.2, Ln3+ doping percent based on the total molar number of Ca and Ln3+). (g) HRTEM image and the corresponding Fourier-transform diffractogram (inset) of the nanowires shown in (a)–(d). Adapted with permission from ref. 57. Copyright 2011, Wiley Online Library. | |
Studies have shown that the doping of Eu3+ (or Tb3+) ions usually endow nanocrystals with novel optical properties.58,59 In the doping experiments as described above, FHAp:Eu3+ (or Tb3+) nano-spindles were endowed with bright red (or green) luminescence properties (Fig. 6).57 It was clear that the replacement of –OH groups in the lattice by F− ions could lessen the hydroxyl-quenching effects on the photoluminescence properties and significantly enhance the luminescence properties of the nanocrystals. In addition, the reaction temperature and the doping amount of Eu3+ (or Tb3+) ions strongly affected the fluorescence properties of the as-obtained materials. However, it was worth noting that the HRTEM images of nano-spindles and ultrathin nanowires show that they all grow along the c-axis corresponding with the (002) lattice plane and were highly crystalline (Fig. 4f and 5g), and the former possessed better fluorescence intensity than the latter, when adopting the same conditions of temperature, reaction time, and the molar ratio of raw materials.
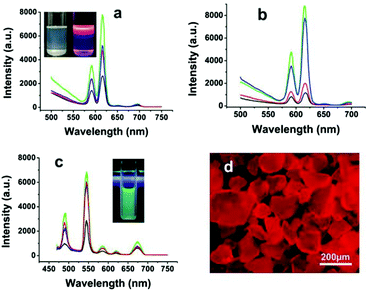 |
| Fig. 6 Luminescent spectra of rice-like FHAp-Eu3+ nanoparticles under different doping conditions: (a) 5% Eu3+ at 220 °C (green line), 180 °C (blue line), 150 °C (red line), and 120 °C (black line); (b) 10% Eu3+ (green line), 5% Eu3+ (blue line), 2.5% Eu3+ (red line), and 1% Eu3+ (black line). The inset in (a) shows the ordinary (left) and luminescent (right) photographs of FHAp-Eu3+ (5%) nanoparticles (180 °C, 12 h) dissolved in cyclohexane. (c) Luminescent spectra of rice-like FHAp-Tb3+ nanoparticles with different Tb3+-doping levels: 10% (green line), 5% (red line), 2.5% (blue line), and 1% (black line). The inset in (c) shows the fluorescence photograph of FHAp-Tb3+ (5%) nanoparticles (180 °C, 12 h) dissolved in cyclohexane. (d) The fluorescence micrograph of FHAp-Eu3+ powder shown in the inset in (a). Adapted with permission from ref. 57. Copyright 2011, Wiley Online Library. | |
3.2 Ultrathin CHAp nanowires and their full color emission by rare earth doping
In the natural bone mineral, HAp nanocrystals are not pure and contain about 4–8 wt% of carbonate and other ionic substitutions like Na+, Mg2+, K+, F−, Cl−, and so on. According to the hydroxyapatite structure, the carbonate group can easily substitute both the hydroxyl and the phosphate ions of the HAp crystal lattice, and form A-type and B-type carbonated hydroxyapatite (CHAp), respectively.60–62 CHAp nanocrystals are characterized by low stability and high reactivity which meet the needs of the organism.62
To simulate natural biological minerals in the structural characteristics and further investigate the mutual influence between PO43− and CO32− groups on the synthesis of nanocrystals, abundant carbonate ions were introduced into the reaction system in the preparation of HAp nanoparticles.63 The results displayed that the reaction temperature and P/C (PO43−/CO32−) molar ratios of precursors were the main factors that influenced the structures of the final products. With the same reaction temperature (90–220 °C), time and Ca/C (Ca2+/CO32−) molar ratio of 1, increasing the P/C molar ratios in the reaction system would gradually tune the diameter from ∼1 to 4 nm and length from 200 to 800 nm of the as-obtained CHAp solid-solution ultrathin nanowires (Fig. 7).
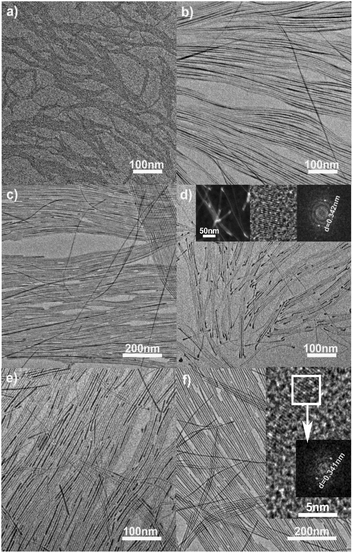 |
| Fig. 7 TEM images of the ultrathin CHAp solid-solution nanowires under different conditions. (a, b) 90 °C, 12 h, the P/C molar ratios of 9 and 36%; (c) 150 °C, 12 h, the P/C molar ratio of 30%; (d–f) 220 °C, 12 h, the P/C molar ratios were 6, 12 and 30%, respectively. Inset in (d), STEM image of nanowires (left), HRTEM image of the bulky nanowire end (middle), and the corresponding Fourier-transform diffractogram (right). Inset in (f), HRTEM image of the nanowires and the corresponding Fourier-transform diffractogram. Adapted with permission from ref. 63. Copyright 2012, Wiley Online Library. | |
Perhaps because of the existence of abundant HAp unit cells, in which the PO43− and OH− ions were replaced by CO32−, the ultrathin CHAp solid-solution nanowires obtained in the suitable P/C molar ratio could produce bright self-activated blue luminescence (Fig. 8a).63–66 If the ultrathin CHAp nanowires were chosen as the matrix and doped with Tb3+ or Eu3+ ions by the second hydrothermal synthesis process, the as-prepared CHAp:Eu3+ (or Tb3+) nanowires with unchanged morphology could emit bright green (red) luminescence (Fig. 8b and c). Using the above tricolors of ultrathin nanowires, recombination luminescence over a full-color spectrum range was easily obtained (Fig. 8d and e).63Fig. 9 shows TEM images and the corresponding EDS analysis of the ultrathin CHAp:Eu3+ (or Tb3+) nanowires prepared by a two-step process.
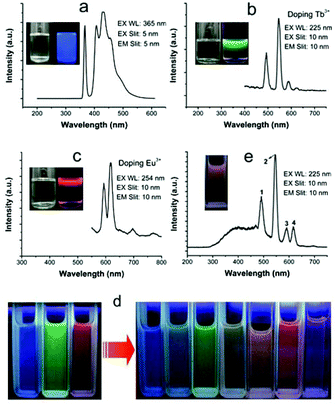 |
| Fig. 8 (a)–(c) Fluorescence spectra of three ultrathin CHAp nanowires formed under different doping conditions (Ln/Ca molar ratio: none, 10% Tb3+, and 10% Eu3+, respectively); insets: the nanowire solution in cyclohexane under visible light (left) and under UV light (right). (d) Full-color images of the three fluorescent solutions (left) and their mixtures (right). (e) Fluorescence spectra and photograph (inset) of a mixture of the green and red fluorescent solutions. Fluorescent photographs were taken under λ = 254 nm UV light by using a camera. Adapted with permission from ref. 63. Copyright 2011, Wiley Online Library. | |
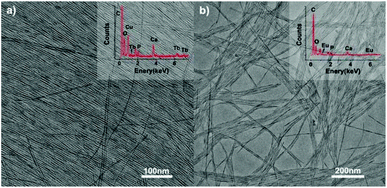 |
| Fig. 9 TEM images of ultrathin CHAp solid-solution nanowires doped with (a) Tb3+ ions and (b) Eu3+ ions. Inset: the corresponding EDS analysis. Adapted with permission from ref. 63. Copyright 2012, Wiley Online Library. | |
3.3 Ultrathin CHAp/Ln3+ solid-solution nanowires
In the same system containing oleic acid, octadecylamine, ethanol and deionized water, we succeeded in synthesizing ultrathin CHAp:Ln3+ (Ln = Ce, Eu, Tb) nanowires of about 1 nm diameter and 10–300 nm length by suitably tuning the doping amount of the rare-earth ions via a one-step approach, which further pushed the size of the CHAp nanobuilding blocks to the one unit cell region (Fig. 10).63 FTIR spectra demonstrated that the rare-earth-doped ultrathin nanowires also have the same characteristic peaks similar to the CHAp nanocrystals reported in the literature (Fig. 11A).60 It showed that the co-doping synergy of different foreign ions could easily cause the growth malformation of the original matrix and lead to the formation of novel ultrathin nanostructures.
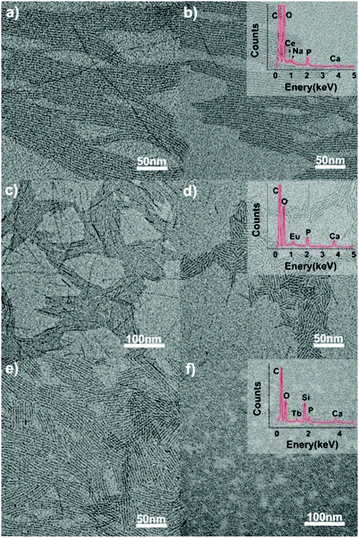 |
| Fig. 10 TEM images of the ultrathin CHAp/Ln3+ solid-solution nanowires synthesized through a one-step hydrothermal process at 120 °C for 12 h. Precursor: CO32− (7 mL), Ca2+ (7 − x) mL, PO43− 0.35 mL, x is the doping ions, as follows: (a) Ce3+ (0.7 mL), (b) Ce3+ (0.8 mL), (c) Eu3+ (0.35 mL), (d) Eu3+ (0.7 mL), (e) Tb3+ (0.14 mL), and (f) Tb3+ (0.35 mL). Adapted with permission from ref. 63. Copyright 2012, Wiley Online Library. | |
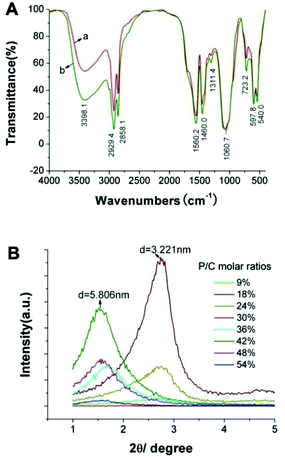 |
| Fig. 11 (A) FTIR spectrum of the as-prepared ultrafine CHAp solid-solution nanowires doped with (a) Ce3+ or (b) Tb3+; (B) SXRD patterns of the ultrathin CHAp solid-solution nanowires obtained with different P/C molar ratios at 90 °C for 12 h. Adapted with permission from ref. 63, Copyright 2012, Wiley Online Library. | |
4. Assembly of monodisperse HAp nanocrystals
The in vivo complex hierarchical macrostructures with multifunctions like bone, muscle fiber, lipid bilayer, etc. are all perfectly assembled by different levels of tiny units.67 The rapidly developing electron microscopy technique is revealing how the fascinating magic structures happen. Inspired by these assembly phenomena of micro/nanoscale units, scientists have been especially keen on researching the assembly and performances of various elementary building blocks.68–70 Due to interesting shape- and size-dependent phenomena and properties, the self-assembly and potential applications of nanocrystals have been extensively investigated in catalysis, sensing, energy storage, electronics, tissue engineering, medicine, environment, etc.71–79 The bottom-up strategy is usually implemented by the assembly of basic nanoscale blocks (such as nanorods, nanowires, nanotubes, and nanopolyhedra) into expected macro/nanostructures. Compared with single atoms or molecules and the bulk counterparts, individual nanocrystals show different properties and become excellent building blocks. But this usually requires precise control over the nanocrystals themselves in terms of size, shape, concentration, monodispersity and the medium in which they are suspended.70
The LSS strategy is not only a general method of monodisperse nanocrystal synthesis, but also of surface modification of nanocrystals prone to self-assembly. Using universal LSS methods, Wang and his colleagues have synthesized different kinds of nanocrystals and found that specific shapes and long-chain ligand modified surfaces are two key factors to direct the self-assembly of functional nanocrystals into higher-order superstructures, and the interactions between the long-chain ligands on the crystal surface would induce the crystal to assemble into certain patterns.80,81 For example, Hu et al. availed a mixture of oleic acid, oleylamine, ethanol and water to synthesize new kinds of one-dimensional ultrathin nanostructures of rare earth hydroxide.80 Through the reduction of size and the increased ratio of ligand to inorganic backbone, the as-synthesized ultrathin nanoribbons with thicknesses less than 1 nm could self-assemble into series of interesting space conformations (nanoloops, periodic frameworks, and various coiling conformations) based on the interaction and energy balance among organic ligands, inorganic structure, and solvent, with reduced flexural rigidity of ultrathin nanowires and/or nanoribbons.
In the process of HAp nanocrystals synthesized via the LSS strategy, the self-assembly phenomenon of the different morphologies of nanocrystals was also very common. On the carbon-coated copper grids, HAp nanorods, FHAp nanotubes, FAp:Ln3+ nanospindles (FAp refers to FHAp with doping levels of F/Ca = 0.2) and ultrathin nanowires tended to form parallel arranged bundles with adjacent nanocrystals along with increasing concentration (Fig. 2–5, 7, 8 and 10).40,46,57,63 This phenomenon should be attributed to the fact that the as-prepared nanocrystals were all enshrouded in a layer of oleic acid, and the interactions between abundant ligands in the surface resulted in the regular arrangement of nanocrystals with even intervals. Fig. 11B shows small angle X-ray diffraction (SXRD) patterns of the ultrathin CHAp solid-solution nanowires obtained with different P/C molar ratios at 90 °C for 12 h.63 The SXRD patterns had different response peaks corresponding to the layer spacing and relative evenness of the samples, which entirely reflected the uniformity of the obtained samples and the thickness of the hydrophobic capping layer (oleic acid and/or octadecylamine) with about 0.9–1.1 nm.
Gradual concentration of monodisperse nanocrystals at the interface is the most basic process for generation of reference assemblies. Various techniques aiming at the interfaces are developed, such as drop casting evaporation, sedimentation, and substrate-induced evaporation, etc. which are convenient for assembly of nanocrystals.82–85 About 1 nm CHAp:Ce3+ solid-solution nanowires could easily form transparent films even through the simple centrifugal sedimentation and natural dry process (Fig. 12).83 And when FAp:Eu3+ nanospindles cyclohexane solutions were dropped on the glass slide, the nanospindles self-assembled into neat stripe patterns by cyclohexane evaporation (Fig. 13a and b).57
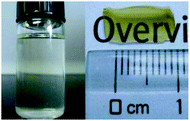 |
| Fig. 12 Ce3+-doped nanowires in cyclohexane (left) and a film formed from Ce3+-doped nanowires (right). Adapted with permission from ref. 63, Copyright 2012, Wiley Online Library. | |
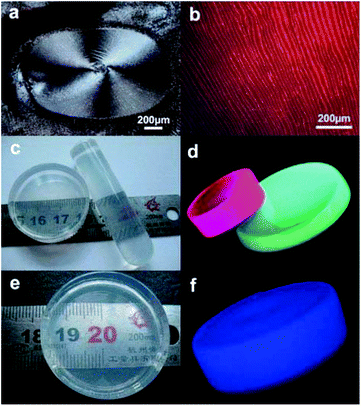 |
| Fig. 13 (a) and (b) show micrographs of dark-field and green-light excitation of a thin layer formed on glass slides from droplets of FHAp:Eu3+ nanoparticles dissolved in cyclohexane; (c) photograph that shows the physical dimensions and the transparency of the nanoparticle/poly(dimethylsiloxane) PDMS composite materials composed of FHAp:Eu3+ (5%, left) and FHAp:Tb3+ (5%, right) nanoparticles; (d) fluorescence photograph of the PDMS samples obtained by a camera through excitation with UV light at 254 nm; (e) photograph showing physical dimensions and transparency of the nanowire/PDMS composite material; (f) fluorescence photograph of the nanowire/PDMS sample taken under λ = 254 nm UV light by using a camera. Adapted with permission from ref. 57 and 63, Copyright 2011, 2012, Wiley Online Library. | |
In addition, all kinds of as-obtained HAp nanocrystals have excellent hydrophobic surface, uniform sizes and shapes, and could perfectly assemble with hydrophobic polymers. As shown in Fig. 4, FAp:Ln3+ (Ln = Eu or Tb) nanospindles, CHAp or CHAp:Ln3+ nanowires could be successfully assembled into the PDMS with different shapes, which did not influence the polymerization of polymers and endowed the new materials with perfect luminescent properties (Fig. 13c–f).57,63 Undoubtedly, the above co-assembly of PDMS-HAp means the realization of more complexities with more functions and flexibilities.
5. Biological applications of HAp nanocrystals
HAp nanocrystals are usually used in the field of tissue engineering, medicine and health, industrial catalysis, environmental protection and other important areas.11,15–18,59,61 HAp nanocrystals are good host materials and are usually doped with diverse ions like Mg2+, Zn2+, Sr2+, Fe2+, Eu3+, Tb3+, F−, Cl−, CO32−, etc. The ion-doped HAp nanoparticles are often endowed with many new changes such as in the shape and size, optical and/or magnetic properties, catalysis, and so on. In general, the synthesis of hydroxyapatite is implemented in the water phase system, and it is difficult to control the shape and size of particles to a certain extent, limiting their application because of the large particle scale. Our research group adopted the LSS strategy to synthesize pure or ion-doped HAp nanocrystals with hydrophobic surfaces, which greatly enriched the types, features and applications of nanometer HAp, such as the preparation of PDMS-HAp composite materials with luminescent properties. But the super-hydrophobic surfaces of HAp nanocrystals also hamper their potential application in the hydrophilic environment.
In order to achieve the hydrophobic/hydrophilic conversion, several strategies were frequently adopted such as the following: oxidation of the oleic acid capping ligand into azelaic acids (HOOC(CH2)7COOH) by Lemieux–von Rudloff reagent,86–88 the complete exchange of the oleic acid molecule with a hydrophilic ligand like poly(acrylicacid) (PAA),88 and polymer encapsulation.78 Hui et al. successfully converted hydrophobic nanocrystals into hydrophilic ones via surface grafting modification using a surfactant (Pluronic F127).89Fig. 14 shows the representative surfactant-mediated hydrophobic/hydrophilic transformation of fusiform FAp:Eu3+ nanocrystals for the purpose of cell markers. The as-prepared uniform hydrophilic FAp:Eu3+ nanospindles with good crystallinity could be very well dispersed in aqueous solution, as well as in cyclohexane solution before modification, as shown in Fig. 15a–c. The hydrophilic FAp:Eu3+ (or Tb3+) nanocrystals would not significantly change the luminescence emission of them (Fig. 15d and e). The results of A549 cells and HeLa cells cultured in media containing different concentrations of FAp:Eu3+ (or Tb3+) nanocrystals demonstrated that these nanoparticles had excellent biocompatibility.
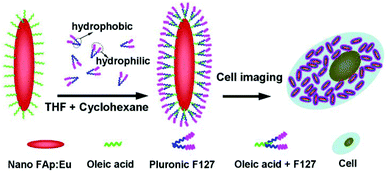 |
| Fig. 14 Schematic diagram showing transformation of oleic acid coated fluoridated HAp:Eu3+ nanoparticles to hydrophilic fluorescent nanocrystals with Pluronic F127 and their use as cell imaging probes. Adapted with permission from ref. 89, Copyright 2012, the Royal Society of Chemistry. | |
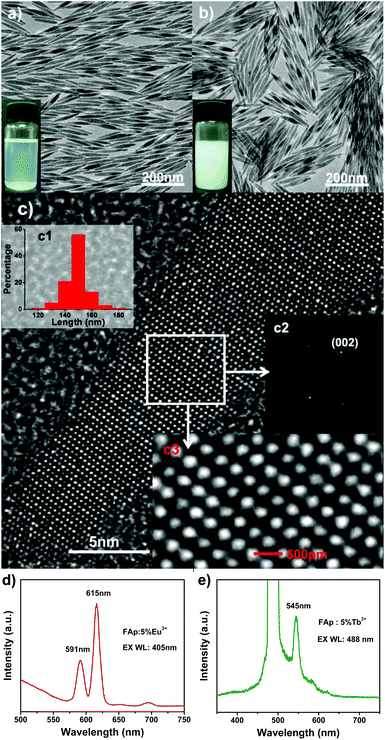 |
| Fig. 15 (a) TEM image of the hydrophobic FAp:5%Eu3+ nanoparticles; the inset shows nanoparticles dissolved in cyclohexane under room light. (b) TEM image of the hydrophilic FAp:5%Eu3+ nanoparticles; the inset shows nanoparticles dissolved in water under room light. (c) HRTEM image of the FAp:5%Eu3+ nanoparticles in (a); insets in (c) are the corresponding length distribution (c1) of nanoparticles, Fourier-transform diffractogram (c2) and local enlargement (c3) of HRTEM images, respectively. (d) Luminescent spectrum of the FAp:5%Eu3+ nanoparticles under excitation at 405 nm. (e) Luminescent spectrum of the FAp:5% Tb3+ nanoparticles under excitation at 488 nm. Adapted with permission from ref. 89, Copyright 2011, the Royal Society of Chemistry. | |
In addition, Zhang et al. proposed a novel PEGylation strategy for surface modification of FAp:Eu3+ (or Tb3+) nanorods with diblock synthetic polymers.90 Choosing stearyl methacrylate (SMA) and poly(ethylene glycol)methacrylate (PEGMA) as monomers, Zhang et al. synthesized amphiphilic polymers through reversible addition-fragmentation chain transfer (RAFT) polymerization. When the aqueous solution of amphiphilic polymers and the cyclohexane solution of FAp:Eu3+ (or Tb3+) nanorods were added to a suitable amount of tetrahydrofuran, the hydrophobic segments (SMA) readily interacted with the oleic acid on the nanorods. After removing the organic media by rotary evaporation, hydrophilic FAp:Eu3+ (or Tb3+) nanorods with PEG coating could be prepared. These PEGylated FAp:Eu3+ (or Tb3+) nanorods maintained the original morphology, and possessed excellent biocompatibility and strong red or green fluorescence, which was successfully utilized for living cell imaging (Fig. 16).
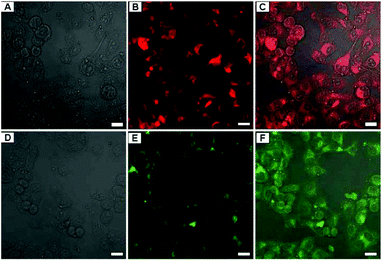 |
| Fig. 16 LCSM images of A549 cells incubated with 150 μg mL−1 of FAp-PEG nanorods for 3 h. (A–C) FAp:Eu3+-PEG nanorods, (D–F) FAp:Tb3+-PEG nanorods; (A and D) bright field, (B and E) fluorescent images excited with a 405 nm (Eu) and a 488 nm (Tb) laser, and (C and F) merged images. Scale bar = 20 μm. Adapted with permission from ref. 90, Copyright 2013, the Royal Society of Chemistry. | |
In order to achieve effective transformation from hydrophobic to hydrophilic properties of HAp:Eu3+ nanocrystals and simultaneously enhance their potential applications, Pan et al. proposed a different scheme which fabricated novel functional nanomaterials.91 According to this strategy (Fig. 17A), HAp:Eu3+ nanocrystals were encapsulated into biocompatible polymer (poly D,L-lactide-co-gly-colide, PLGA) nanospheres with the surface carboxyl groups via a solvent extraction/evaporation method, and then the surface of polymer NPs encapsulating HAp:Eu3+ nanocrystals were grafted with the targeting molecule (folate). The as-prepared HAp:Eu3+-loaded PLGA nanoparticles with folate decoration can be observed in Fig. 17B and E. Experimental results showed that the new type of functional NPs had low toxicity, high sensitivity, and good photostability, and they could be used for specific targeted imaging of MCF-7 breast cancer cells with overexpression of folate receptors by folate receptor-medicated endocytosis.
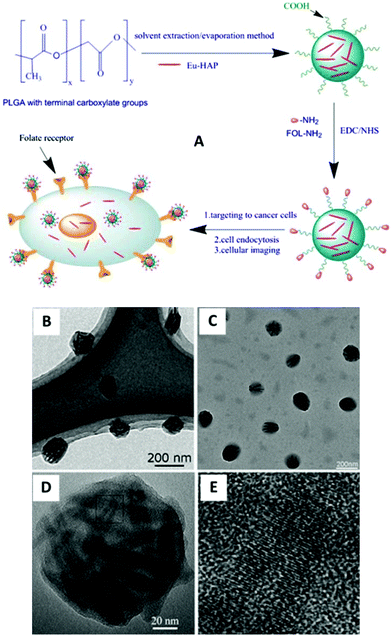 |
| Fig. 17 (A) Detailed scheme for the preparation of HAp:Eu3+-loaded nanoparticles with folate decoration for cellular imaging; (B, C) TEM images of HAp:Eu3+-loaded nanoparticles with folate decoration; (D) HRTEM images of a single HAp:Eu3+ polymer nanoparticle; (E) enlarged HRTEM image of the square part in (D). Adapted with permission from ref. 91, Copyright 2013, American Institute of Chemical Engineers. | |
6. Conclusions
Over the years, based on the excellent physical/chemical properties and biological performance of HAp nanocrystals, their synthesis methods and application ways have been pursued by many researchers. In this article, we summarize our recent developments in tunable synthesis, ion doping, assembly and applications of monodisperse HAp nanocrystals. Through the design of reasonable ligands, types of dopants and doping ways, series of HAp nanocrystals with different shapes/sizes and features were successfully prepared and applied. However, there are still many challenges, such as the following: how to achieve a more precise control of synthesis, how to synthesize hydrophilic ultrathin nanocrystals, how to simulate biological assembly utilizing different nano-HAp building blocks, how to fabricate novel/new HAp nanocrystals by multiple-ion synergistic effect, and so on. We believe that these issues are significant to expand potential applications of HAp nanocrystals in biotechnology, catalysis, medicine, etc., and hope that the relevant research process and results can provide reference for the development of other materials.
Acknowledgements
This work was supported by the National Natural Science Foundation of China (21376190, 21221062 and 91127040), the State Key Project of Fundamental Research for Nanoscience and Nanotechnology (2011CB932402), Shaanxi Provincial Scientific Technology Research and Development Program (2012JM2014), and China Postdoctoral Science Foundation (2013M542378).
Notes and references
- M. A. Meyers, P. Y. Chen, A. Y. M. Lin and Y. Seki, Prog. Mater. Sci., 2008, 53, 1 CrossRef CAS PubMed
.
- F. Z. Cui, Y. Li and J. Ge, Mater. Sci. Eng., R, 2007, 57, 1 CrossRef PubMed
.
- L. C. Palmer, C. J. Newcomb, S. R. Kaltz, E. D. Spoerke and S. I. Stupp, Chem. Rev., 2008, 108, 4754 CrossRef CAS PubMed
.
- X. Su, K. Sun, F. Z. Cui and W. J. Landis, Bone, 2003, 32, 150 CrossRef CAS
.
- D. M. Liu, Q. Z. Yang and T. Troczynski, Biomaterials, 2002, 23, 691 CrossRef CAS
.
- Y. Yang, K. H. Kim and J. L. Ong, Biomaterials, 2005, 26, 327 CrossRef CAS PubMed
.
- L. Zhang, P. Tang, M. Xu, W. Zhang, W. Chai and Y. Wang, Acta Biomater., 2010, 6, 2189 CrossRef CAS PubMed
.
- H. Zhou and J. Lee, Acta Biomater., 2011, 7, 2769 CrossRef CAS PubMed
.
- T. Y. Liu, S. Y. Chen, D. M. Liu and S. C. Liou, J. Control. Release, 2005, 107, 112 CrossRef CAS PubMed
.
- M. Corti and F. J. Rohlf, Biol. J. Linn. Soc., 2001, 73, 99 CrossRef
.
- M. Iafisco, J. M. Delgado-Lopez, E. M. Varoni, A. Tampieri, L. Rimondini, J. Gomez-Morales and M. Prat, Small, 2013, 9, 3834 CrossRef CAS PubMed
.
- M. Z. Yin, Y. C. Han, I. W. Bauer, P. Chen and S. P. Li, Biomed. Mater., 2006, 1, 38 CrossRef CAS PubMed
.
- W. Paul and C. P. Sharma, J. Colloid Interface Sci., 1995, 174, 224 CrossRef CAS
.
- A. Barroug, J. Fastrez, J. Lemaitre and P. Rouxhet, J. Colloid Interface Sci., 1997, 189, 37 CrossRef CAS
.
- Y. Hou, C. J. Morrison and S. M. Cramer, Anal. Chem., 2011, 83, 3709 CrossRef CAS PubMed
.
- S. Saxena and S. F. D'Souza, Environ. Int., 2006, 32, 199 CrossRef CAS PubMed
.
- J. A. Gómez del Rio, P. J. Morando and D. S. Cicerone, J. Environ. Manage., 2004, 71, 169 CrossRef PubMed
.
- J. Xu, T. White, P. Li, C. He and Y. F. Han, J. Am. Chem. Soc., 2010, 132, 13172 CrossRef CAS PubMed
.
- I. Mobasherpour, M. S. Heshajin, A. Kazemzadeh and M. Zakeri, J. Alloys Compd., 2007, 430, 330 CrossRef CAS PubMed
.
- Z. C. Wang, F. Chen, L. M. Huang and C. J. Lin, J. Mater. Sci., 2005, 40, 4955 CrossRef CAS
.
- N. Nassif, F. Martineau, O. Syzgantseva, F. Gobeaux, M. Willinger, T. Coradin, S. Cassaignon, T. Azaïs and M. M. Giraud-Guille, Chem. Mater., 2010, 22, 3653 CrossRef CAS
.
- D. M. Liu, Q. Yang, T. Troczynski and W. J. Tseng, Biomaterials, 2002, 23, 1679 CrossRef CAS
.
- J. K. Han, H. Y. Song, F. Saito and B. T. Lee, Mater. Chem. Phys., 2006, 99, 235 CrossRef CAS PubMed
.
- G. C. Koumoulidis, A. P. Katsoulidis, A. K. Ladavos, P. J. Pomonis, C. C. Trapalis, A. T. Sdoukos and T. C. Vaimakis, J. Colloid Interf. Sci., 2003, 259, 254 CrossRef CAS
.
- G. Guo, Y. Sun, Z. Wang and H. Guo, Ceram. Int., 2005, 31, 869 CrossRef CAS PubMed
.
- Q. Ren, H. J. Luo and L. Zhou, Rare Metal Mat. Eng., 2004, 33, 115 CAS
.
- K. Kandori, T. Kuroda, S. Togashi and E. Katayama, J. Phys. Chem. B, 2011, 115, 653 CrossRef CAS PubMed
.
- O. A. Graeve, R. Kanakala, A. Madadi, B. C. Williams and K. C. Glass, Biomaterials, 2010, 31, 4259 CrossRef CAS PubMed
.
- V. Stanić, D. Janaćković, S. Dimitrijević, S. B. Tanasković, M. Mitrić, M. S. Pavlović, A. Krstić, D. Jovanović and S. Raičević, Appl. Surf. Sci., 2011, 257, 4510 CrossRef PubMed
.
- G. Liu, J. W. Talley, C. Na, S. L. Larson and L. G. Wolfe, Environ. Sci. Technol., 2010, 44, 1366 CrossRef CAS PubMed
.
- X. Wang, J. Zhuang, Q. Peng and Y. Li, Nature, 2005, 437, 121 CrossRef CAS PubMed
.
- X. Wang, J. Zhuang, Z. Huo, S. Hu and Y. Li, Inorg. Chem., 2008, 47, 543 CrossRef CAS PubMed
.
- X. Wang, J. Zhuang, Q. Peng and Y. Li, Langmuir, 2006, 22, 7364 CrossRef CAS PubMed
.
- X. Liang, X. Wang, J. Zhuang, Y. Chen, D. Wang and Y. Li, Adv. Funct. Mater., 2006, 16, 1805 CrossRef CAS
.
- Z. Zhuang, Q. Peng, J. Liu, X. Wang and Y. Li, Inorg. Chem., 2007, 46, 5179 CrossRef CAS PubMed
.
- X. Wang, J. Zhuang, Q. Peng and Y. Li, Inorg. Chem., 2006, 45, 6661 CrossRef CAS PubMed
.
- Z. Huo, C. Chen, D. Chu, H. Li and Y. Li, Chem.–Eur. J., 2007, 13, 7708 CrossRef CAS PubMed
.
- X. Liang, X. Wang, J. Zhuang, Q. Peng and Y. Li, Adv. Funct. Mater., 2007, 17, 2757 CrossRef CAS
.
- S. Hu, H. Liu, P. Wang and X. Wang, J. Am. Chem. Soc., 2013, 135, 11115 CrossRef CAS PubMed
.
- X. Wang, J. Zhuang, Q. Peng and Y. Li, Adv. Mater., 2006, 18, 2031 CrossRef CAS
.
- L. Sun, F. Banhart, A. V. Krasheninnikov, J. A. Rodriguez-Manzo, M. Terrones and P. M. Ajayan, Science, 2006, 312, 1199 CrossRef CAS PubMed
.
- O. H. Kwon and A. H. Zewail, Science, 2010, 25, 1668 CrossRef PubMed
.
- J. Goldberger, R. Fan and P. Yang, Acc. Chem. Res., 2006, 39, 239 CrossRef CAS PubMed
.
- M. R. Falvo, G. J. Clary, R. M. Taylor Li, V. Chi, F. P. Brooks Jr., S. Washburn and R. Superfine, Nature, 1997, 389, 582 CrossRef CAS PubMed
.
- S. Hu and X. Wang, J. Am. Chem. Soc., 2008, 130, 8126 CrossRef CAS PubMed
.
- J. Hui, G. Xiang, X. Xu, J. Zhuang and X. Wang, Inorg. Chem., 2009, 48, 5614 CrossRef CAS PubMed
.
- J. Hui and D. Fan, J. Mater. Res., 2009, 24, 2499 CrossRef CAS
.
- F. Wang, Y. Han, C. S. Lim, Y. Lu, J. Wang, J. Xu, H. Chen, C. Zhang, M. Hong and X. Liu, Nature, 2010, 463, 1061 CrossRef CAS PubMed
.
- F. Zhang, J. Li, J. Shan, L. Xu and D. Zhao, Chem.–Eur. J., 2009, 15, 11010 CrossRef CAS PubMed
.
- Y. Liu, D. Wang, J. Shi, Q. Peng and Y. Li, Angew. Chem., Int. Ed., 2013, 52, 4366 CrossRef CAS PubMed
.
- M. A. Katkova and M. N. Bochkarev, Dalton Trans., 2010, 39, 6599 RSC
.
- W. Qin, D. Zhang, D. Zhao, L. Wang and K. Zheng, Chem. Commun., 2010, 46, 2304 RSC
.
- H. Q. Wang, M. Batentschuk, A. Osvet, L. Pinna and C. J. Brabec, Adv. Mater., 2011, 23, 2675 CrossRef CAS
.
- J. C. Zhou, L. D. Sun, J. Shen, J. Q. Gu and C. H. Yan, Nanoscale, 2011, 3, 1977 RSC
.
- D. J. Naczynski, M. C. Tan, M. Zevon, B. Wall, J. Kohl, A. Kulesa, S. Chen, C. M. Roth, R. E. Riman and P. V. Moghe, Nat. Commun., 2013, 4, 2199 CAS
.
- V. Kachkanov, M. J. Wallace, G. van der Laan, S. S. Dhesi, S. A. Cavill, Y. Fujiwara and K. P. O'Donnell, Sci. Rep., 2012, 2, 969 CAS
.
- J. Hui and X. Wang, Chem.–Eur. J., 2011, 17, 6926 CrossRef CAS PubMed
.
- P. Yang, Z. Quan, C. Li, X. Kang, H. Lian and J. Lin, Biomaterials, 2008, 29, 4341 CrossRef CAS PubMed
.
- F. Chen, P. Huang, Y. J. Zhu, J. Wu and D. X. Cui, Biomaterials, 2012, 33, 6447 CrossRef CAS PubMed
.
- J. Barralet, S. Best and W. Bonfield, J. Biomed. Mater. Res., 1998, 41, 79 CrossRef CAS
.
- E. Landi, G. Celotti, G. Logroscino and A. Tampieri, J. Eur. Ceram. Soc., 2003, 23, 2931 CrossRef CAS
.
- S. Padilla, I. Izquierdo-Barba and M. Vallet-Regí, Chem. Mater., 2008, 20, 5942 CrossRef CAS
.
- J. Hui, Q. Yu, Y. Long, Z. Zhang, Y. Yang, P. Wang, B. Xu and X. Wang, Chem.–Eur. J., 2012, 18, 13702 CrossRef CAS PubMed
.
- A. Pifferi, P. Taroni, A. Torricelli, G. Valentini, P. Mutti, G. Ghislotti and L. Zanghieri, Appl. Phys. Lett., 1997, 70, 348 CrossRef CAS PubMed
.
- M. Fujimaki, Y. Ohki and H. Nishikawa, J. Appl. Phys., 1997, 81, 1042 CrossRef CAS PubMed
.
- C. Zhang, J. Yang, Z. Quan, P. Yang, C. Li, Z. Hou and J. Lin, Cryst. Growth Des., 2009, 9, 2725 CAS
.
- T. Dvir, B. P. Timko, D. S. Kohane and R. Langer, Nat. Nanotechnol., 2011, 6, 13 CrossRef CAS PubMed
.
- E. S. Place, N. D. Evans and M. M. Stevens, Nat. Mater., 2009, 8, 457 CrossRef CAS PubMed
.
- H. B. Yao, H. Y. Fang, X. H. Wang and S. H. Yu, Chem. Soc. Rev., 2011, 40, 3764 RSC
.
- J. X. Gong, G. D. Li and Z. Y. Tang, Nano Today, 2012, 7, 564 CrossRef CAS PubMed
.
- S. Sacanna, W. T. M. Irvine, P. M. Chaikin and D. J. Pine, Nature, 2010, 464, 575 CrossRef CAS PubMed
.
- M. R. Jones, R. J. Macfarlane, B. Lee, J. Zhang, K. L. Young, A. J. Senesi and C. A. Mirkin, Nat. Mater., 2010, 9, 913 CrossRef CAS PubMed
.
- D. Zerrouki, J. Baudry, D. Pine, P. Chaikin and J. Bibette, Nature, 2008, 455, 380 CrossRef CAS PubMed
.
- A. Gautieri, S. Vesentini, A. Redaelli and M. J. Buehler, Nano Lett., 2011, 11, 757 CrossRef CAS PubMed
.
- S. Sacanna, L. Rossi and D. J. Pine, J. Am. Chem. Soc., 2012, 134, 6112 CrossRef CAS PubMed
.
- P. Wang, Y. Yang, J. Zhuang and X. Wang, J. Am. Chem. Soc., 2013, 135, 6834 CrossRef CAS PubMed
.
- Z. Tang, S. Shen, J. Zhuang and X. Wang, Angew. Chem., 2010, 122, 4707 CrossRef
.
- A. Sánchez-Iglesias, M. Grzelczak, T. Altantzis and B. Goris, ACS nano, 2012, 6, 11059 Search PubMed
.
- M. E. Leunissen, R. Dreyfus, R. Sha, T. Wang, N. C. Seeman, D. J. Pine and P. M. Chaikin, Soft Matter, 2009, 5, 2422 RSC
.
- S. Hu, H. Liu, P. Wang and X. Wang, J. Am. Chem. Soc., 2013, 135, 11115 CrossRef CAS PubMed
.
- J. F. Liu and Y. D. Li, Adv. Mater., 2007, 19, 1118 CrossRef CAS
.
- T. Ming, X. Kou, H. Chen, T. Wang, H. L. Tam, K. W. Cheah, J. Y. Chen and J. Wang, Angew. Chem., Int. Ed., 2008, 120, 9831 CrossRef
.
- A. R. Tao, D. P. Ceperley, P. Sinsermsuksakul, A. R. Neureuther and P. Yang, Nano Lett., 2008, 8, 4033 CrossRef CAS PubMed
.
- J. Huang, F. Kim, A. R. Tao, S. Connor and P. Yang, Nat. Mater., 2005, 4, 896 CrossRef CAS PubMed
.
- Z. Zhu, H. Meng, W. Liu, X. Liu, J. Gong, X. Qiu, L. Jiang, D. Wang and Z. Tang, Angew. Chem., Int. Ed., 2011, 123, 1631 CrossRef
.
- Z. Chen, H. Chen, H. Hu, M. Yu, F. Li, Q. Zhang, Z. Zhou, T. Yi and C. Huang, J. Am. Chem. Soc., 2008, 130, 3023 CrossRef CAS PubMed
.
- G. Wang, Q. Peng and Y. Li, J. Am. Chem. Soc., 2009, 131, 14200 CrossRef CAS PubMed
.
- R. Naccache, F. Vetrone, V. Mahalingam, L. A. Cuccia and J. A. Capobianco, Chem. Mater., 2009, 21, 717 CrossRef CAS
.
- J. Hui, X. Zhang, Z. Zhang, S. Wang, L. Tao, Y. Wei and X. Wang, Nanoscale, 2012, 4, 6967 RSC
.
- X. Zhang, J. Hui, B. Yang, Y. Yang, D. Fan, M. Liu, L. Tao and Y. Wei, Polym. Chem., 2013, 4, 4120 RSC
.
- J. Pan, D. Wan, Y. Bian, H. Sun, C. Zhang, F. Jin, Z. Huang and J. Gong, AIChE J., 2013, 59, 4494 CrossRef CAS
.
|
This journal is © the Partner Organisations 2014 |
Click here to see how this site uses Cookies. View our privacy policy here.