Carbon nanomaterials rescue phenanthrene toxicity in zebrafish embryo cultures
Received
27th May 2015
, Accepted 22nd June 2015
First published on 24th June 2015
Abstract
Multi-Walled Carbon Nanotubes (MWCNTs) and Carbon Blacks (CB) are known to carry wide varieties of adsorbed, potentially toxic chemicals resulting from their manufacturing, typically including metals and polyaromatic hydrocarbons (PAHs). Instead of desorption of these adsorbates into aqueous milieu, substantial high-affinity adsorption of the aqueous dispersed PAH, phenanthrene to MWCNTs and CB from aqueous solutions and dispersions is reported. Phenanthrene adsorption to aqueous dispersed carbon nanomaterials phases was measured using isotherms and then exploited to remove toxic levels of phenanthrene from aqueous media incubating zebrafish embryos (ZFEs) as a whole organism toxicity screening system. Remarkable reduction in phenanthrene-induced ZFE toxicity was observed using two experiments: one comparing PAH concentration-dependent rescue of ZFE viability from MWCNTs compared to carbon black (CB), and a second examining kinetics of the ZFE rescue by MWCNTs vs. CB incubations after initial ZFE exposure to phenanthrene for 2 hours. Phenanthrene LD50 concentration in the absence of any carbon-based sorbent increases dramatically to 10 μg ml−1 in the presence of either 81.3 μg ml−1 MWCNTs or 81.5 μg ml−1 CB materials. When CB and MWCNTs were added to ZFEs previously exposed to 10 μg ml−1 phenanthrene for 2 hours, significant rescue of ZFE viability was observed in CB-treated embryos while no ZFE rescue was observed in MWCNT-treated ZFEs. This result is consistent with an expected carbon nanomaterial surface area-dependent ZFE rescue effect: CB exhibits a higher Brunauer–Emmett–Teller (BET) surface area than the MWCNTs used, with higher adsorption capacity likely for phenanthrene, yielding ZFE rescue from toxicity.
Nano impact
Nanotoxicity is an increasing concern for public health given the increasing number of new engineered nanomaterials introduced into consumer products and the environment with potential for wide dispersal. Carbon-based nanomaterials represent a major class of engineered nanomaterials with numerous possible interactions with the environment and living systems. This whole organism toxicity study evaluates the zebrafish embryo toxicity to the common environmental polyaromatic hydrocarbon toxin, phenanthrene, and reports toxicity rescue mediated by multi-walled carbon nanotubes and carbon blacks added to the culture milieu.
|
Introduction
Use of carbon nanotubes (CNTs) in both consumer and medical products has increased dramatically over the past decade and continues to increase.1 Their broadening applications significantly raise the potential for human exposure to CNTs by various uncontrolled, inadvertent (i.e., unintentional environmental and occupational exposure) and controlled, deliberate (i.e., biomedical imaging, drug delivery exposure) routes and dosings. This has implications for human toxicity risks with these carbon-based nanomaterials. Recent findings suggest a variety of material architecture-dependent CNT toxicological effects ranging from asbestosis-like responses to some inhaled single-walled CNTs (SWNTs)2 to increased inflammation when multi-walled carbon nanotubes (MWCNTs) are exposed to the epidermis.3 Beyond intrinsic CNT toxicity mechanisms, another major concern is the potential for CNTs, with their high specific surface areas (200–800 m2 g−1),4 known levels of contamination from their manufacture,5 and lack of adequate surface characterization in many reports6 to carry both adsorbed heavy metal catalysts used in CNT production and adsorbed polyaromatic hydrocarbons (PAHs) as by-products of CNT manufacture7 and other adsorbed ubiquitous environmental contaminants.6,8 These “loadings” of contaminants on CNT surfaces can constitute considerable adsorbate dosing to living systems under reasonable CNT particle exposures.6 However, CNT-PAH adsorption is likely stabilized on CNT surfaces by hydrophobic π-stacking binding affinities comparable in energetics to hydrogen bonds.5,9 Therefore, “delivery” of such adsorbates into biological systems and their resulting dosing and bioavailability from CNT exposure is currently unknown. In many biological milieus, CNT transport into lipid microenvironments (e.g., cell membranes, chylomicrons) and interactions with globular proteins (e.g., albumin, lipoproteins) could facilitate or promote desorption of CNT-associated PAHs into host tissues with possible toxicological implications.
The effects of CNT-PAH exposure in the whole-organism zebrafish embryo (ZFE) toxicity model10 were studied here using phenanthrene (a 3-ring PAH) as a model toxin and adsorbate. Phenanthrene was chosen as a model PAH due to its suspected high adsorptive capacity to MWCNT and finite solubility in 1% DMSO aqueous milieu. The working hypothesis was that ZFE toxicity induced by PAHs in solution would be mitigated by high surface-area carbonaceous nanoparticle adsorbents. MWCNTs were added to ZFEs in 1% DMSO to observe intrinsic MWCNT toxicity and associated toxicity from desorbed species. Previous studies have demonstrated that 1% DMSO exhibits no observable adverse biological effects.11
Carbon black (CB) – well-known as a clinically useful and safe sorbent for various organic compounds12 – was selected for comparison to MWCNTs in this model. Experiments determined phenanthrene toxicities in the ZFE model, then monitored ZFE toxicity under different PAH exposures with carbon nanomaterials in ZFE media to determine LD50 and LD90 values. Phenanthrene concentration in both absence and presence of MWCNTs provided PAH adsorption/desorption isothermal analysis with added MWCNTs. Varying ratios of MWCNTs and CBs in the presence of fixed toxic concentrations (LD90 = 10 μg ml−1) of phenanthrene were assessed after two hours of ZFE pre-exposure to phenanthrene. ZFE viability was then assessed as a function of PAH exposure.
Materials and methods
Chemicals and sample preparation
Phenanthrene (Acros Organics, >97%) and dimethyl sulfoxide (DMSO, HPLC grade, Fisher, USA) were used as supplied. Cleaned multi-walled carbon nanotubes (MWCNT, BuckyUSA) were described as 95% pure by the manufacturer with a diameter of 5–15 nm and a length of 1–10 μm. Mass spectrometric analysis (ICP-MS) was performed on MWCNTs digested in nitric acid, and concentrations of 42 elemental impurities were measured. Nonporous carbon black (CB, Cabot, USA) was classified as a high-purity furnace black with low amounts of total PAHs (<0.5 ppm). Carbon Black was also analyzed by the identical ICP-MS method. Phenanthrene was dissolved in DMSO at a stock concentration of 1 mg ml−1 which was then diluted 1
:
100 in Millipore-polished ASTM grade 1 water to yield a final concentration of 10 μg ml−1 phenanthrene in 1% DMSO aqueous dispersion as a stock solution. Phenanthrene was stored in the dark to avoid photodegradation. Similar separate stock solutions of 2 mg ml−1 CB and MWCNTs in 1% DMSO were also prepared.
Zebrafish embryo (ZFE) exposures
Adult wild-type AB zebrafish (Danio rerio) were bred and maintained using standard procedures.13 All zebrafish experiments were performed in compliance with institutional guidelines and approval from The Institutional Animal Care and Use Committee. Fertilized eggs were collected at 2 hours post fertilization (hpf) and rinsed in a dilute methylene blue solution. Embryos between 3–4 hpf were then added individually to a non-tissue culture 96-well plate (one embryo per well). Each experimental condition included 12 embryos (n = 12) and the concentration-dependent experiment was performed four times (N = 4 independent replicates) (Fig. 3) and the time-dependent experiment (Fig. 4) was performed in triplicate (N = 3 independent replicates). ZFEs were added to wells containing 90 μL of 10 μg ml−1 phenanthrene in 1% DMSO in Millipore ASTM grade 1 water. Carbon nanomaterials (MWCNTs, CB) were then added in 10 μL volumes from stock solutions to wells to reach final concentrations of 200, 100, 50, or 25 μg ml−1 carbon nanomaterials, 10 μg ml−1 phenanthrene, and 1% DMSO in Millipore ASTM grade 1 water. This milieu was always prepared fresh immediately prior to its dosing into ZFE cultures. Embryos were treated for at least 24 hours and visualized on an M80 stereomicroscope (Leica). Positive controls comprised ZFEs in 100 μL of 10 μg ml−1 phenanthrene in 1% DMSO in Millipore ASTM grade 1 water. Negative controls comprised ZFEs in 100 μL of 1% DMSO in Millipore ASTM grade 1 water. ZFE viability was determined by visual assessment of ZFE tissue opacity.14
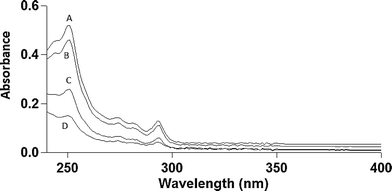 |
| Fig. 1 Ultraviolet optical absorption spectra of phenanthrene after 40 minutes in 1% DMSO aqueous media at room temperature (21 °C): (A) 10 μg ml−1 phenanthrene in 1% DMSO; (B) 10 μg ml−1 phenanthrene in 1% DMSO with 30 μg ml−1 MWCNT (MWCNT separated for UV analysis of supernatant); (C) 10 μg ml−1 phenanthrene with 100 μg ml−1 MWCNT; (D) 10 μg ml−1 phenanthrene with 200 μg ml−1 MWCNT (MWCNT separated for UV analysis of supernatant). | |
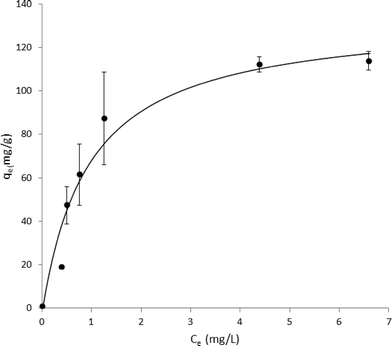 |
| Fig. 2 Adsorption isotherm for phenanthrene from ASTM research-grade aqueous dispersions onto multi-walled carbon nanotubes at 21 °C (qe = adsorbed phenanthrene, Ce = equilibrium concentration). R2 = 0.957 using the Langmuir adsorption model: qe = Q0Ce/(Kd + Ce). A value of Q0 = 137 mg g−1 for the saturation by phenanthrene is derived from the curve fit and coefficient approximation. | |
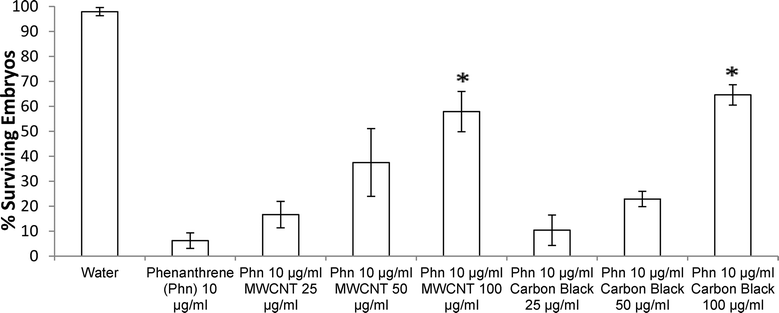 |
| Fig. 3 Phenanthrene toxicity in zebrafish embryos with various concentrations of either carbon black or MWCNTs added to ZFE media. ZFEs were dosed with phenanthrene at 3–4 hpf and visually assessed for mortality at 4 and 20 hpf. Data represent mean ± SE (N = 4) and were analyzed by Dunnett's test (*p < 0.05). *Represents significant difference from phenanthrene only control (10 μg ml−1) (Phn = phenanthrene). | |
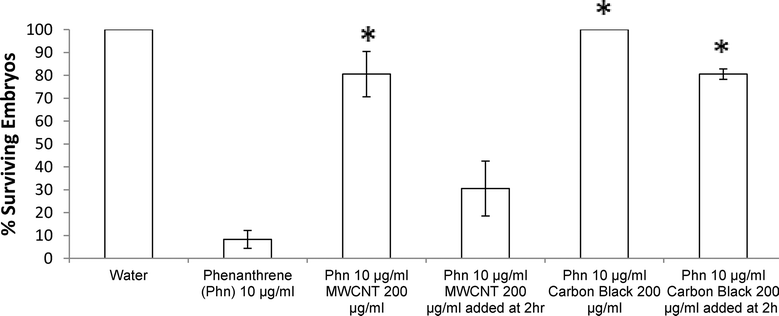 |
| Fig. 4 Phenanthrene toxicity rescue in ZFEs is observed with MWCNTs or CB added 2 hours after phenanthrene dosing (10 μg ml−1). Data represent mean ± SE (N = 3) as analyzed by Dunnett's test (*p < 0.05) relative to phenanthrene-only survival controls shown. *Represents significant difference from phenanthrene only (10 μg ml−1) (Phn = phenanthrene). | |
Materials characterization
Surface areas for MWCNTs and CB were measured by Brunauer–Emmett–Teller (BET) analysis of nitrogen physisorption at 77 K using a Micromeritics ASAP 2000 instrument.15
Measurement of phenanthrene in solution
Phenanthrene solution concentration was determined using UV/Vis spectrophotometry (λmax = 251 nm, Varian Cary 400 Biospectrophotometer 200–900 nm). Supernatant was removed from MWCNT/phenanthrene mixtures to measure extent of PAH adsorption as a function of varying MWCNT concentrations (100 μg ml−1 and 50 μg ml−1). Optical absorbance measurements were performed 20–40 minutes after preparing phenanthrene solutions, time sufficient to allow MWCNTs to settle from solution as evidenced by the disappearance of the MWCNT optical absorbance signature from these solutions. Settling time determined by UV/Vis spectroscopy was reflected by the lack of optical absorbance above 300 nm where MWCNTs exhibit a strong characteristic absorbance, but where phenanthrene does not. Phenanthrene concentration was also measured by HPLC (Thermo Scientific Finnigan Surveyor HPLC with UV/Vis and fluorescence detection, C18 reverse-phase 100 mm × 4.6 cm column) using fluorescence detection at wavelengths of 260/380 nm excitation/emission, respectively.
Monitoring phenanthrene adsorption to MWCNTs
Phenanthrene solutions (10 μg ml−1) in 1% DMSO were prepared by adding a 1
:
100 dilution of 1 mg ml−1 phenanthrene in 100% DMSO to Millipore-polished ASTM grade 1 water. MWCNTs were dispersed in a Branson 5210 bath sonicator at maximum power for 10 minutes and then added to this PAH solution to reach a final concentration of 30, 50, 100, 150, 200, and 500 μg ml−1 from a 2 mg ml−1 stock solution in Millipore-polished ASTM grade 1 purified water. This sonication method is previously reported to effectively disperse MWCNTs.16 The mixture of MWCNTs and phenanthrene was allowed to incubate unstirred at room temperature under ambient air for 90 minutes. MWCNTs were then separated from the solution by standing and the supernatant was measured spectrophotometrically at 251 nm and room temperature. Concentrations of phenanthrene were calculated by comparison to a phenanthrene standard curve generated under these same conditions. Langmuir adsorption isotherms were generated from optical absorbance data for phenanthrene solutions using open-access curve fitting software at http://www.zunzun.com (last accessed June, 2014). Specifically, nonlinear regressions were fit to the Langmuir equation: qe= Q0Ce/(Kd + Ce) where qe (amount of phenanthrene adsorbed per unit MWCNT) and Ce (bulk concentration of phenanthrene) are known, measured quantities. Q0 was derived from the best-fit result.
Statistical analysis
Statistical analysis was performed by two-tailed Dunnett's test using ANOVA provided by the XLSTAT add-in module in Microsoft Excel v.2010. Significance was defined as p < 0.05. Pairwise differences were compared between untreated 10 μg ml−1 phenanthrene-treated ZFEs and 10 μg ml−1 phenanthrene-treated ZFEs in the presence of either MWCNTs or CB.
Results
MWCNTs were found to be 98.6% pure by ICP-MS analysis, with the largest impurities being nickel (0.86%) and iron (0.2%). No other non-carbon elements were present above 0.2%. Carbon Black was found to be 99.8% pure with the largest impurity being potassium (0.14%). BET analysis performed to compare the surface areas of MWCNTs and CB samples determined 138.9 m2 g−1 for MWCNTs and 232.9 m2 g−1 for CB. These results agree well with previous surface area data for analogous carbon nanomaterials.17–20
Phenanthrene concentration was measured by HPLC to characterize the physical state of phenanthrene in solution. Two distinct fluorescence peaks were observed in HPLC traces (data not shown): one early-eluting sharp peak (ex 260/em 380 nm) and one later-eluting broad peak (ex 260/em 380 nm). The relative area under this broad peak decreased over a period of hours and at one week was undetectable (attributed to aqueous phase separation of suspended phenanthrene). In order to determine the adsorption capacity of phenanthrene on MWCNTs, phenanthrene presence in solution was analyzed by optical spectroscopy to generate adsorption isotherms for phenanthrene on MWCNTs. Absorption spectra are shown in Fig. 1 with phenanthrene concentrations calculated from optical absorption peaks at 251 nm. Analogous adsorption isotherm construction for CB was hindered by the greater CB aqueous dispersive properties (i.e., no sedimentation), increasing solution opacity and preventing precise optical density measurements for phenanthrene. Adsorption data were fit to a Langmuir adsorption isotherm (R2 = 0.957, Fig. 2).
Both exposure of ZFEs to 1% DMSO in aqueous media, or to MWCNTs or CBs at variable solid dispersed dosings in 1% DMSO aqueous dispersions (from 25 to 10
000 μg ml−1) produced no visible ZFE toxicity or mortality. Exposure of ZFEs to aqueous phenanthrene alone (10 μg ml−1) resulted in significant toxicity and reliable mortality in 90% of embryos (n = 96, LD90 = 10 μg ml−1). Importantly, incubation of CB or MWCNTs to phenanthrene solutions of 10 μg ml−1 ZFEs significantly reduces ZFE phenanthrene-induced toxicity (Fig. 3). This is observed to be carbon nanomaterial dose-dependent, an effect akin to ZFE “rescue” from PAH-induced toxicity. ZFE rescue from phenanthrene toxicity was most prominently observed when CB or MWCNTs were present at concentrations of 200 μg ml−1 or greater (20× mass ratio excess with respect to phenanthrene). At this MWCNT loading in ZFE media, only infrequent, minor changes in ZFE morphological phenotypes due to phenanthrene exposure were noted (data not shown, ~5% of surviving embryos exhibited tail malformations representing a score of 1 on a published 4-point malformation scale21 – a incidence we deemed as insignificant to the study's conclusions), with no significant differences in embryo survival rates relative to untreated controls (see Fig. 3). However, at reduced carbon nanomaterial loadings of 50 μg ml−1 or 25 μg ml−1 in cultures, minor ZFE phenotypic abnormalities were common with significant decreases in ZFE survival (Fig. 3). These abnormalities included enlarged pericardial sac, malformed yolk sac, and shortened tail, but these defects were associated with nearly complete embryo mortality.22 Occasional ZFE survival was noted in 10 μg ml−1 phenanthrene at a threshold dose of 25 μg ml−1 of CB and MWCNTs (Fig. 3). Further, 50% ZFE rescue from phenanthrene (10 μg ml−1) toxicity corresponds to respective additions of 81.3 μg ml−1 MWCNTs or 81.5 μg ml−1 CB. Correlation of these results with the Fig. 2 adsorption isotherm facilitated determination of the phenanthrene LD50 for ZFEs. Given that 50% of the ZFEs survived in 10 μg ml−1 phenanthrene with a MWCNT concentration of 81.3 μg ml−1, the LD50 of phenanthrene should correlate with the unadsorbed concentration of phenanthrene at this same MWCNT concentration of 81.3 μg ml−1. Based on this assessment, the phenanthrene LD50 for 3–4 hpf ZFEs is determined to be 2.42 μg ml−1.
No significant differences were noted between MWCNTs and CB ZFE rescue efficacies (i.e., same nanomaterials dosing for each rescued LD50). However, the duration of ZFE exposure to phenanthrene prior to exposure to carbon nanomaterial addition to cultures discriminates the two carbon nanomaterial treatment conditions. Adding CB (200 μg ml−1) two hours post-phenanthrene exposure to ZFEs provides significant rescue effects (80% ZFE survival) while an equal concentration of MWCNTs added after the same PAH exposure time yields only 30% ZFE survival (see Fig. 4).
Discussion
This study assessed the toxicity of the model PAH, phenanthrene, on the ZFE whole organism culture model, and ZFE toxicity rescue resulting from additions of carbon nanomaterials, CB and MWCNT, as known sorbent phases shown to remove the model PAH, phenanthrene, from ZFE solution. Phenanthrene is a known toxin with reported toxicities in Danio rerio reporting an LD50 of 0.293 μg ml−1 when exposed to adult fish for 14 days.23 Despite limited aqueous solubility (1.28 μg ml−1),23 phenanthrene as a model PAH exhibits profound ZFE toxicities in their aqueous culture model at 10 μg ml−1. However, its aqueous toxicity to ZFEs as a model whole organism can be modulated by adding carbon nanomaterials to ZFE cultures. This modulation is correlated with nanomaterial adsorption of PAHs, removing PAHs from solution to mitigate intrinsic ZFE toxicity. MWCNT or CB material addition alone to ZFE cultures did not produce observed toxicity at levels up to 10
000 μg ml−1. A clear trend relates ZFE survival to CB or MWCNT dosing in the presence of phenanthrene, regardless of carbon nanomaterial physical structure. Higher concentrations of CB or MWCNTs promote ZFE survival in the presence of phenanthrene, demonstrating the interaction of carbon nanomaterials with phenanthrene in ZFE media to remove PAH toxicity. Important to the study design, aqueous 1% DMSO media was employed versus ZFE E3 embryo medium to minimize carbon nanomaterial aggregation: media ionic strength can prompt increased nanomaterial aggregation. No significant toxicity differences were observed for ZFEs in 1% DMSO/water over that in E3 ZFE medium.
Phenanthrene has four distinct characteristic optical absorption peaks between 250 and 291 nm,24 allowing spectrophotometric detection of phenanthrene in 1% DMSO solution. Optical absorbance measurements using the 251 nm primary absorption peak characteristic of the phenanthrene/MWCNT solution were converted to phenanthrene concentration using an extinction coefficient of 6.31 × 104,24 In the absence of MWCNTs, the baseline optical absorbance for 10 μg ml−1 phenanthrene was elevated for all wavelengths above its characteristic absorbance bands due to light scattering of colloidal phenanthrene aggregates (λ > 300 nm, Fig. 4). This suggests that the 10 μg ml−1 phenanthrene aqueous preparation is likely a biphasic system comprising both dissolved phenanthrene and suspended insoluble phenanthrene aggregate particles.25 This is supported qualitatively by an observed visual increase in solution opacity when phenanthrene in DMSO is added to water. Additionally, the solubility of phenanthrene in water has been reported to be 1.28 μg ml−1,23 suggesting that a majority of phenanthrene in the 10 μg ml−1 solutions is suspended as a dispersion. Reductions in solution fluorescence over time noted in HPLC experimental data also support this conclusion. This system could have been simplified to homogeneous solutions by reducing phenanthrene concentrations; however, phenanthrene is not reliably toxic to ZFEs at these lower concentrations where it is readily water-soluble. Additionally, measuring lower concentrations of MWCNTs can be problematic due to their tendency to aggregate in solution, leading to imprecise addition of MWCNT masses as volumetric suspensions to the samples (i.e., for any two 25 μl MWCNT aqueous dispersed samples, one may have a different MWCNT mass from the other due to aggregation). The consequence of both of these issues is that studying the full range of soluble phenanthrene concentrations causing ZFE toxicity across the entire range of carbon nanophase surface areas is neither readily nor reliably achieved.
Phenanthrene adsorption to MWCNTs is rapid: UV measurements taken within 30 minutes of addition of MWCNTs to phenanthrene solutions demonstrated a MWCNT concentration-dependent depletion in both baseline and characteristic phenanthrene optical absorbance (Fig. 1). As seen in Fig. 2, the good adsorption isotherm fit suggests that a concentration of 50 μg ml−1 MWCNTs adsorbs 56.1% of available phenanthrene at 10 μg ml−1 (Table 1). When MWCNTs in 1% DMSO were dosed to ZFEs, no intrinsic MWCNT toxic effects were observed. This is attributed both to the tightly MWCNT-adsorbed species (and their low aqueous solubility prompting this partitioning to MWCNT surfaces) and to poor organismal access due to MWCNTs and their aggregates in ZFE media.
Table 1 Fractional MWCNT uptake of phenanthrene from a 10 μg ml−1 phenanthrene aqueous solution
Concentration MWCNTs (μg ml−1) |
% phenanthrene adsorbed |
Standard deviation |
50 |
56.2 |
3.1 |
100 |
87.5 |
24.4 |
150 |
92.5 |
22.8 |
200 |
95.0 |
18.17 |
This supports the hypothesis underlying this research that MWCNT-mediated PAH adsorption for both the suspended and dissolved phenanthrene phases is responsible for the observed ZFE viability rescue effects in ZFE cultures. It is important to note that in an environmental scenario, many endogenous aqueous compounds can compete for available surface adsorption sites on carbon nanomaterials. Surface-active molecules such as humic acids and other biosurfactants and polyaromatic species will compete with PAHs for adsorption sites on MWCNTs and CB, reducing MWCNT-based organism rescue abilities against water-based toxins.26
Together, these data suggest that irreversible ZFE toxicity occurs following just over 2 hours of phenanthrene exposure starting at 3–4 hpf ZFEs. Moreover, these data also suggest that CB is able to deplete phenanthrene from the ZFE media more quickly and effectively than MWCNTs for the observed ZFE protective effect. This difference is attributed to direct carbon nanomaterial-phenanthrene adsorption differences in media that make this PAH unavailable to the ZFE cultures – the mechanism proposed for the observed ZFE toxicity rescue. Differences in phenanthrene adsorption amounts and kinetics by these carbon nanomaterials may arise from the higher CB surface area as well as different carbon surface structures and their respective intrinsic sorbate thermodynamics for adsorbing phenanthrene. Alternatively, differences may be due to different carbon nanoparticle dispersion physical states in the aqueous media exposed to phenanthrene. CB was observed to disperse more homogeneously in 1% DMSO solutions than MWCNTs at all solid loadings used, suggesting that this physical aqueous dispersion is an additional cause for the observed significant differences in ZFE survival rates by providing different phenanthrene access to each carbon nanomaterial surface in partitioning and adsorption from the media.27,28
These data also support tight binding of phenanthrene to both CB and MWCNT nanomaterials in aqueous dispersions, not easily desorbed into solution. Previous data concluded that adsorption hysteresis was not observed for phenanthrene and MWCNTs in water.7 Conversely, the ZFE rescue effect observed here, and associated decrease in visible phenanthrene concentration measured by UV/Vis (Fig. 1) suggest that irreversible hysteresis may occur in this model system. It is also possible that hysteresis does occur in this case, but at the time of phenanthrene desorption, ZFEs may have reached a developmental maturity more resistant to its toxicity. The increased rescue effect observed for CB dosed at 2 hours post-phenanthrene exposure is consistent with the increased BET surface area of CB relative to MWCNTs. Additionally, another recent report also confirmed that activated carbon exhibited higher PAH adsorption in aqueous media than MWCNTs.5
According to Table 1 and Fig. 3, fractional adsorption of phenanthrene to MWCNT correlates well with in vivo data for ZFE survival, reflecting the amounts of phenanthrene not available to ZFEs in the presence of MWCNTs. The high standard deviations observed for 100, 150, and 200 μg ml−1 MWCNT concentrations is likely due to MWCNT aggregation, blocking potential adsorption sites and possibly resulting in non-uniform mass/volume additions of MWCNTs from the 2 mg ml−1 MWCNT stock solution to create each isotherm. For comparison, theoretical total surface area of dissolved phenanthrene at a concentration of 10 μg ml−1 was estimated using the assumptions that each molecule has a molecular diameter of 0.79 nm29 and a maximum close-packed fraction of surface area occupied by spherical molecules of 0.74. Given these parameters, complete adsorption of 10 μg ml−1 of phenanthrene would be expected to require a total MWCNT adsorbed surface area of 0.0166 m2 ml−1 from this solution. For comparison, respective total surface areas for CB and MWCNTs at concentrations of 100 μg ml−1 were calculated to be 0.0172 m2 ml−1 and 0.0103 m2 ml−1, respectively. For CB, this concentration and adsorption capacity would theoretically accommodate adsorption of ~104% of all phenanthrene molecules in the 10 μg ml−1 solution. The observed 70% ZFE survival rate with CB rescue is therefore less than that predicted by this 100% non-bioavailable theoretical adsorption value. However, multiple factors influence nanomaterial adsorption capacity in actual ZFE settings, including nanomaterials aggregation, PAH adsorption/desorption deviation from Langmuir isotherm models, including reported PAH multilayers30 and desorption hysteresis.7 The collective BET-determined surface area from MWCNT addition is theoretically sufficient to bind 62% of the phenanthrene in this system, correlating well with the observed ZFE rescue of 60% at this concentration. Actual phenanthrene adsorption from 1% DMSO Millipore water aqueous solutions, however, shows higher phenanthrene adsorption (87.5%, Table 1, Fig. 2) than these theoretically predicted MWCNT values.
Adsorption of various organic compounds to MWCNTs, including phenanthrene, has also been previously described by Langmuir models.31,32 Phenanthrene adsorption to MWCNTs here fits moderately well to a Langmuir equation consistent with its fit to a previous Langmuir adsorption isotherm.33 The R2 value correlating these data with the Langmuir model (0.957) is likely reduced by MWCNT aggregation in solution. Aggregation at higher MWCNT concentrations in water will reduce their PAH adsorption capacity, deviating from model Langmuir adsorption. Despite this, the theoretical phenanthrene adsorption capacity of 137 mg g−1 shown here is higher than that reported for previous MWCNT data.33 Previous adsorption capacities of MWCNTs and phenanthrene reported a Q0 value of 41.7 mg g−1.33,34 However, the surface area of the MWCNTs were reported to be lower and phenanthrene was added in methanol rather than DMSO in these studies, which could account for the discrepancy.
Activated charcoal has been used historically to remove toxins by solution adsorption35,36 and has shown efficacy even added 1.5 hours after exposure to known toxins.37 With the 3–4 hpf ZFEs used in this experiment, ZFE death typically occurred within 6 hours of phenanthrene introduction in control studies. Thus, carbon nanomaterials were needed to provide ZFE rescue within a few hours of PAH exposure: 2 hours was the latest point producing any significant ZFE rescue effect. Significant differences in this ZFE rescue effect from phenanthrene toxicity were observed with CB additions, but not with MWCNT additions (both relative to phenanthrene-only control). This result correlates well with the higher CB surface area for phenanthrene and its increasing adsorption capacity for phenanthrene. Importantly, overall results shown are consistent with previous reports of simple PAH adsorption to MWCNTs in aqueous solutions,27,33 supporting unique, new assertions here that 1) MWCNTs do not cause significant toxicity in ZFEs, and 2) that MWCNTs have a high adsorptive capacity for PAHs. This is the first report to correlate this phenanthrene (or any PAH) adsorption with viability influences on living whole organism cultures such as ZFEs.
Conclusions
The collective data presented herein demonstrate whole organism viability rescue from introduced phenanthrene toxicity in ZFE cultures by addition of both CB and MWCNT dispersions to ZFE aqueous milieu. These carbonaceous nanomaterials dispersions act as sorptive substrates for rapid phenanthrene removal from ZFE media. The comparatively larger BET surface area for CB yields greater PAH adsorption capacity for phenanthrene in time-dependent carbon nanomaterials rescue assays, but does not significantly increase ZFE viability in concentration-dependent rescue experiments. These results establish that carbon nanomaterial phases: 1) act as significant sorbent phases for adsorption of sparingly soluble or hydrophobic compounds from aqueous media, 2) rescue ZFEs from aqueous toxicity from added known toxins and 3) do not readily release adsorbed adventitious PAH contaminants known to reside on MWCNTs and CBs from their commercial production into these solutions. Hence, possible toxicities due to desorption of adventitious adsorbed PAH compounds might not necessarily occur from carbon nanomaterials in certain aqueous conditions. Also, in the manufacturing of MWCNTs, it may not be always necessary to completely remove certain tightly adsorbed PAHs present after synthesis as these compounds may be unlikely to leach or cause significant toxicity in such assays. Future studies should determine if MWCNT-resident PAHs are more likely to desorb in more hydrophobic environments in physiological systems, such as partitioning into adipose tissues, fatty tissues (e.g., brain), cell membranes, hydrophobic pockets of abundant globular plasma proteins like albumin, or lipid aggregates like lipoproteins or chylomicrons.
Acknowledgements
The authors acknowledge support from a University of Utah SEED grant (DWG) and University UROP support (to SL).
References
- E. G. Rakov, Carbon nanotubes in new materials, Russ. Chem. Rev., 2013, 82, 27 CrossRef PubMed
.
- C. A. Poland,
et al., Carbon nanotubes introduced into the abdominal cavity of mice show asbestos-like pathogenicity in a pilot study, Nat. Nanotechnol., 2008, 3, 423–428 CrossRef CAS PubMed
.
- N. A. Monteiro-Riviere, R. J. Nemanich, A. O. Inman, Y. Y. Wang and J. E. Riviere, Multi-walled carbon nanotube interactions with human epidermal keratinocytes, Toxicol. Lett., 2005, 155, 377–384 CrossRef CAS PubMed
.
- R. Bacsa,
et al., High specific surface area carbon nanotubes from catalytic chemical vapor deposition process, Chem. Phys. Lett., 2000, 323, 566–571 CrossRef CAS
.
- A. Brooks, H. Lim and J. E. Kilduff, Adsorption uptake of synthetic organic chemicals by carbon nanotubes and activated carbons, Nanotechnology, 2012, 23, 294008 CrossRef CAS PubMed
.
- D. W. Grainger and D. G. Castner, Nanobiomaterials and nanoanalysis: opportunities for improving the science to benefit biomedical technologies, Adv. Mater., 2008, 20, 867–877 CrossRef CAS PubMed
.
- K. Yang and B. Xing, Desorption of polycyclic aromatic hydrocarbons from carbon nanomaterials in water, Environ. Pollut., 2007, 145, 529–537 CrossRef CAS PubMed
.
- C. F. Jones and D. W. Grainger,
In vitro assessments of nanomaterial toxicity, Adv. Drug Delivery Rev., 2009, 61, 438–456 CrossRef CAS PubMed
.
- M. Keiluweit and M. Kleber, Molecular-level interactions in soils and sediments: the role of aromatic π-systems, Environ. Sci. Technol., 2009, 43, 3421–3429 CrossRef CAS
.
- A. J. Hill, H. Teraoka, W. Heideman and R. E. Peterson, Zebrafish as a model vertebrate for investigating chemical toxicity, Toxicol. Sci., 2005, 86, 6–19 CrossRef CAS PubMed
.
- C. Y. Usenko, S. L. Harper and R. L. Tanguay,
In vivo evaluation of carbon fullerene toxicity using embryonic zebrafish, Carbon, 2007, 45, 1891–1898 CrossRef CAS PubMed
.
- Z. Király,
et al., Selective sorption of phenol and related compounds from aqueous solutions onto graphitized carbon black, Adsorption and flow microcalorimetric studies, Langmuir, 1996, 12, 423–430 CrossRef
.
-
M. Westerfield, The zebrafish book, Univ. of Oregon Pr., 2000 Search PubMed
.
- S. Ali, H. G. J. van Mil and M. K. Richardson, Large-scale assessment of the zebrafish embryo as a possible predictive model in toxicity testing, PLoS One, 2011, 6, e21076 CAS
.
- S. Li, Y. Wu and K. J. Whitty, Ash deposition behavior during char− slag transition under simulated gasification conditions, Energy Fuels, 2010, 24, 1868–1876 CrossRef CAS
.
- Y. Liu,
et al., Debundling of single-walled carbon nanotubes by using natural polyelectrolytes, Nanotechnology, 2007, 18, 365702 CrossRef
.
- A. Peigney, C. Laurent, E. Flahaut, R. Bacsa and A. Rousset, Specific surface area of carbon nanotubes and bundles of carbon nanotubes, Carbon, 2001, 39, 507–514 CrossRef CAS
.
- Q. Richard and R. T. Yang, Carbon nanotubes as superior sorbent for dioxin removal, J. Am. Chem. Soc., 2001, 123, 2058–2059 CrossRef
.
- F. Rodriguez-Reinoso, J. M. Martin-Martinez, C. Prado-Burguete and B. McEnaney, A standard adsorption isotherm for the characterization of activated carbons, J. Phys. Chem., 1987, 91, 515–516 CrossRef CAS
.
- T. M. Sager and V. Castranova, Surface area of particle administered versus mass in determining the pulmonary toxicity of ultrafine and fine carbon black: comparison to ultrafine titanium dioxide, Part. Fibre Toxicol., 2009, 6, 1–11 CrossRef PubMed
.
- V. E. Fako and D. Y. Furgeson, Zebrafish as a correlative and predictive model for assessing biomaterial nanotoxicity, Adv. Drug Delivery Rev., 2009, 61, 478–486 CrossRef CAS PubMed
.
- T. C. King-Heiden,
et al., Quantum dot nanotoxicity assessment using the zebrafish embryo, Environ. Sci. Technol., 2009, 43, 1605–1611 CrossRef CAS
.
- C. M. Prosser, M. A. Unger and W. K. Vogelbein, Multistressor interactions in the zebrafish (Danio rerio): Concurrent phenanthrene exposure and Mycobacterium marinum infection, Aquat. Toxicol., 2011, 102, 177–185 CrossRef CAS PubMed
.
-
H. H. Jaffé and M. Orchin, Theory and applications of ultraviolet spectroscopy, 1962 Search PubMed
.
- G. Langergraber, N. Fleischmann and F. Hofstaedter, A multivariate calibration procedure for UV/VIS spectrometric quantification of organic matter and nitrate in wastewater, Water Sci. Technol., 2003, 47, 63–71 CAS
.
- X. Wang, S. Tao and B. Xing, Sorption and competition of aromatic compounds and humic acid on multiwalled carbon nanotubes, Environ. Sci. Technol., 2009, 43, 6214–6219 CrossRef CAS
.
- J. Chen, W. Chen and D. Zhu, Adsorption of nonionic aromatic compounds to single-walled carbon nanotubes: Effects of aqueous solution chemistry, Environ. Sci. Technol., 2008, 42, 7225–7230 CrossRef CAS
.
- J. Cheng, E. Flahaut and S. H. Cheng, Effect of carbon nanotubes on developing zebrafish (Danio rerio) embryos, Environ. Toxicol. Chem., 2009, 26, 708–716 CrossRef PubMed
.
- S. Sawamura, Pressure Dependence of the Solubilities of Anthracene and Phenanthrene in Water at 25° C, J. Solution Chem., 2000, 29, 369–375 CrossRef CAS
.
- B. Pan and B. Xing, Adsorption mechanisms of organic chemicals on carbon nanotubes, Environ. Sci. Technol., 2008, 42, 9005–9013 CrossRef CAS
.
- S. G. Wang,
et al., Adsorption of fulvic acids from aqueous solutions by carbon nanotubes, J. Chem. Technol. Biotechnol., 2007, 82, 698–704 CrossRef CAS PubMed
.
- C. Lu, Y.-L. Chung and K.-F. Chang, Adsorption thermodynamic and kinetic studies of trihalomethanes on multiwalled carbon nanotubes, J. Hazard. Mater., 2006, 138, 304–310 CrossRef CAS PubMed
.
- K. Yang, L. Zhu and B. Xing, Adsorption of polycyclic aromatic hydrocarbons by carbon nanomaterials, Environ. Sci. Technol., 2006, 40, 1855–1861 CrossRef CAS
.
- K. Yang, X. Wang, L. Zhu and B. Xing, Competitive sorption of pyrene, phenanthrene, and naphthalene on multiwalled carbon nanotubes, Environ. Sci. Technol., 2006, 40, 5804–5810 CrossRef CAS
.
- A. Dąbrowski, P. Podkościelny, Z. Hubicki and M. Barczak, Adsorption of phenolic compounds by activated carbon—a critical review, Chemosphere, 2005, 58, 1049–1070 CrossRef PubMed
.
- K. R. Olson, Activated charcoal for acute poisoning: one toxicologist's journey, J. Med. Toxicol., 2010, 6, 190–198 CrossRef PubMed
.
- R. A. Curtis, J. Barone and N. Giacona, Efficacy of ipecac and activated charcoal/cathartic: prevention of salicylate absorption in a simulated overdose, Arch. Intern. Med., 1984, 144, 48 CrossRef CAS PubMed
.
|
This journal is © The Royal Society of Chemistry 2015 |
Click here to see how this site uses Cookies. View our privacy policy here.