DOI:
10.1039/C5RA02314A
(Paper)
RSC Adv., 2015,
5, 30197-30205
Curcumin induces structural change and reduces the growth of amyloid-β fibrils: a QCM-D study†
Received
6th February 2015
, Accepted 17th March 2015
First published on 17th March 2015
Abstract
Amyloid-β (Aβ) fibrillation is a crucial factor in the etiology of Alzheimer's disease (AD). Curcumin has been widely studied and considered as a promising drug for AD treatment, but its molecular mechanism in inhibiting Aβ aggregation is still not clearly defined. In the present study, quartz crystal microbalance with dissipation monitoring (QCM-D) was used to analyse the growth behaviors of Aβ fibrils in the presence and absence of curcumin. The viscoelasticity properties that reflect the structural information of the resulting Aβ aggregates were also analysed using a ΔD/ΔF plot. It was found that the presence of curcumin could accelerate the deposition process of Aβ monomers on the initially immobilized fibrils, with the growth rate being 21.1–120.6% higher than that of the control. Viscoelasticity analysis showed that curcumin-induced Aβ aggregates exhibited a much more flexible structure than that of the initial fibrils, and the degree of this kind of structural conversion was related to the concentration of curcumin. Importantly, we found that further deposition of Aβ monomers on these loosely constructed Aβ aggregates was significantly inhibited, with the growth rate being only 34.2% of initial rate. Therefore, the QCM-D study demonstrated that curcumin inhibited the growth of Aβ fibrils through a process that led to the structural conversion of the growing fibrils, and directed these fibrils to an off-pathway aggregation, which may hinder the formation of long Aβ fibrils that could cause neuronal damage.
Introduction
AD is one of the most devastating neurodegenerative diseases, and is histopathologically characterized by the presence of senile plaques and neurofibrillary tangles in the brain.1–3 The major component of amyloid plaques is Aβ, a peptide with 39 to 43 amino acid residues generated from the sequential proteolysis of amyloid precursor protein (APP) by β- and γ-secretases.4,5 The most common isoforms are Aβ40 and Aβ42, which are 40 and 42 residues long, respectively, with Aβ40 being more abundant.6,7 According to the amyloid cascade hypothesis for AD progression, the formation of undesirable Aβ fibrils (containing large quantity of β-sheet) is caused by an imbalance between the production and clearance of Aβ.8–10 The progressive accumulation of various Aβ aggregates in the brain is fundamental to the development of AD, which can then induce a cascade of events such as abnormal hyperphosphorylation and subsequent tangle formation of tau, oxidative damage, inflammation, as well as neuronal loss, which eventually resulting in cognitive impairment.8,11 Despite extensive researches on the etiology of AD, the underlying molecular mechanism of Aβ aggregation and neurotoxicity remains unclear. Aβ aggregation has been causally linked to cellular toxicity. In healthy people, the soluble monomer is nontoxic and can be removed.12 Therefore, preventing Aβ from aggregating and keeping it in the monomer stage would have a positive effect on the individual. For this reason, the majority of studies have concentrated on finding inhibitors that can interfere with the fibrillation process and on understanding the related mechanism of fibrillation.13–20
Curcumin, a natural small molecule compound (Fig. 1A), which is the principal active component of turmeric, has been considered as a promising drug with various pharmacological activities, including anti-cancer, anti-oxidative and anti-inflammatory activities.21–24 Epidemiological studies have shown that regular consumption of turmeric significantly lowers the prevalence of AD, especially among Southeast Asian populations.25,26 Furthermore, many studies have demonstrated the beneficial effects of curcumin for AD using experimental models. These including (i) lowering Aβ deposition in AD transgenic mice (Tg2576);27 (ii) reversing Aβ-induced spatial memory deficits in middle age SD rats;28 (iii) improving the spatial learning and memory of Aβ40-induced AD rats;29 (iv) reducing neurotoxicity in transgenic Drosophila;30 and (v) providing cultured neuronal cells with a protective effect against Aβ-induced cytotoxicity.31 Based on these promising results, curcumin has been considered as a promising drug for AD patients and several clinical trials have been carried out.21 Despite growing evidence that demonstrates the effect of curcumin on Aβ-induced neurotoxicity, the molecular mechanism of neuroprotection provided by curcumin remains unclear.
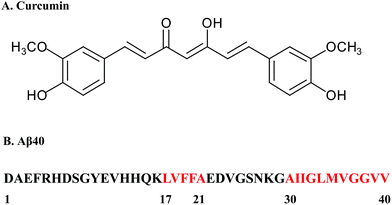 |
| Fig. 1 (A) Structure of curcumin. (B) The sequence of Aβ40 is shown in one-letter amino acids. The amino acids that play a central role in the aggregation are indicated in red. | |
The anti-inflammatory and anti-oxidant properties of curcumin can also have beneficial effect for AD sufferers. It is more important that curcumin can directly modulate Aβ aggregation. Despite the amount of extensive studies being done, the effects of curcumin on the aggregation tendency of Aβ are sometimes contradictory. Curcumin has the ability to bind both Aβ oligomers and fibrils but not the monomer.32,33 Earlier studies have found that curcumin can reduce Aβ plaque size and inhibit the formation of Aβ fibrils as well as their extension.32,34 However, a recent study appears to indicate that curcumin does not decrease the amount of Aβ deposition. Instead, it accelerates Aβ fibril formation through promoting the conversion of prefibrillar aggregates to fibril in Aβ transgenic flies.30 Study that used immunoblot has also demonstrated that curcumin enhances Aβ aggregation.35 Furthermore, emerging evidences have shown that some polyphenolic compounds can promote the generation of nontoxic off-pathway aggregates.36 Through comparative analysis of the differences described above, we speculated that the above inconsistencies might be related to different research methods and Aβ species used in the studies, and different aggregation phases. Therefore, as a promising drug candidate for the treatment of AD, a new method was needed to evaluate the effect of curcumin on the different phases of Aβ aggregation and the related modulating mechanism.
QCM-D is a special type of QCM developed in the past 20 years.37 After specific molecules have been immobilized onto the sensor surface through versatile conjugating methods,38,39 the analyte is allowed to pass into the sample chamber and come into contact with the immobilized molecules. Increase in the mass of the sensor that arises from absorbing analyte to the immobilized molecules would produce a decrease in the frequency, which allows the quantitative investigation of the kinetics of combination. On the other hand, the dissipation change measured simultaneously can characterize the viscoelastic properties so that it provides useful structural information on the adsorbed materials.40,41 By allowing sensitive, label-free, real-time investigation of biomolecule interaction, QCM-D is particularly appropriate for investigating the aggregation of specific “aggregating seed”. Recently, QCM-D has been successfully applied to the studies of amyloid peptides.42–48 Therefore QCM-D is a powerful tool for studying amyloid growth and has potential application in the investigation of small molecules that affect amyloid fibrils growth and their associated mechanisms.
In this study, the effect of curcumin on the growth of Aβ fibril was examined. The structure and morphology of the prepared Aβ fibrils were characterized using ThT assay and TEM, respectively. To confirm that the domains for β-sheet aggregation were not changed during covalent immobilization, anti-Aβ antibodies binding experiment was carried out. The kinetics of Aβ deposition in the absence and presence of curcumin was also being determined using QCM-D and aggregation rates under different conditions were assessed comparatively. Furthermore, the structural information of growing Aβ aggregates in the absence and presence of curcumin was analysed based on ΔD/ΔF plot. The present study provided a detailed description on how curcumin affects Aβ fibril growth kinetics, and demonstrated that QCM-D could be a potent tool to screen for amyloid inhibitors and investigate related modulating mechanism.
Materials and methods
Chemicals
Human Aβ1-40 with a purity higher than 95% was obtained from GL Biochem Ltd (Shanghai, China). Dimethyl sulfoxide (DMSO), 11-mercaptoundecanoic acid (11-MUA), N-hydroxysuccinimide (NHS), N-(2-dimethylaminopropyl)-N′-ethylcarbodiimide (EDC), ethanolamine, curcumin and thioflavin T were purchased from Sigma-Aldrich (MO, USA). 1,1,1,3,3,3-Hexafluoro-2-propanol (HFIP; 99%) and ethanol (99.8%; HPLC/ACS) were purchased from J&K Chemical (Beijing, China). Monoclonal anti-Aβ antibody 4G8 (against Aβ 17–24) was purchased from Signet Laboratories (Dedham, MA, USA). Polyclonal anti-Aβ40 C-terminal antibody (bs-0877M) was purchased from Beijing Biosynthesis Biotechnology Co. LTD (Beijing, China). All other materials and reagents used were of analytical grade. MilliQ water and Phosphate Buffered Saline (PBS) were filtered through a 0.22 μm syringe filter before use.
Preparation of Aβ monomer and fibril
Aβ peptide was dissolved in cold HFIP to a concentration of 1 mM. It was sonicated for 10 min while being kept in an ice water bath. After sonication, it was incubated at room temperature for 1 h. The HFIP was removed by evaporation under a gentle stream of N2 and the resulting peptide film was stored at −20 °C until use. The HFIP-treated Aβ was redissolved in DMSO to a concentration of 5 mM by repeated pipetting, followed by sonication for 1 min, and then diluted in PBS to a series of concentrations. Aβ fibril was prepared by diluting 5 mM Aβ-DMSO stock solution to 100 μM in PBS and agitating it for 48 h at 37 °C. Formation of Aβ fibrils containing orderly stacked β-sheets was confirmed by ThT assay and TEM. Aβ monomer was prepared just prior to QCM-D experiment.
TEM
Aβ fibril (20 μL) was adsorbed onto a carbon-coated copper grid (200 mesh) for 10 min. After washing with 10 μL distilled water for two times, the sample on the grid was negatively stained for 2 min with 2% aqueous phosphotungstic acid. It was then washed again with 10 μL distilled water and dried under air in turn. Finally, the sample was examined under a JEM-2100 transmission electron microscope (JEOL, Japan) at 100 kV.
ThT measurement
ThT can specifically bind to orderly stacked β-sheets structure in Aβ fibril and produce enhanced light emission. The assay has been widely used for evaluating the formation of amyloid fibril. The ThT stock solution was prepared by dissolving ThT in PBS to a final concentration of 10 μM. A 20 μL sample of the Aβ fibril was taken at different agitation times and mixed with 180 μL of 10 μM ThT stock solution in a black 96-well plate. The fluorescence of the sample was measured in triplicate using a Varioskan Flash (Thermo Scientific Co.). Excitation and emission wavelengths were set at 450 nm and 482 nm, respectively.
Immobilization of Aβ fibril onto sensor surface
Gold-coated AT-cut quartz crystals were treated with a piranha solution (70% H2SO4–30% H2O2 = 4
:
1) for 10 min, and then rinsed several times with MilliQ water. The electrode was immersed in 5 mM 11-MUA/ultrapure ethanol solution for 48 h. After washing with ethanol and water, the electrode was activated for 30 min at room temperature using 0.4 M EDC and 0.1 M NHS. Finally, the electrode was immersed in Aβ fibrils for 12 h at room temperature. After washing with PBS and water, the remaining activated sites were blocked by 1 M ethanolamine at pH 8.0 for 1 h to avoid nonspecific binding. The electrode was washed with PBS and water, and then stored at 4 °C.
Quartz crystal microbalance with dissipation monitoring (QCM-D)
A Q-Sense E4 system (Q-Sense, Gothenburg, Sweden) was used to conduct QCM-D measurements. The temperature was maintained at 25 ± 0.05 °C. The resonance frequency was recorded at the third, fifth, seventh, ninth and eleventh overtone corresponding to 15, 25, 35, 45 and 55 MHz, respectively. The frequency and dissipation in this study reflected the third (∼15 MHz) overtone. A flow rate of 50 μL min−1 was maintained for all experiments. PBS buffer was degassed by sonication for 20 min prior to use. For each measurement, PBS was pumped over the sensor crystal surface until a stable baseline was established, at which point the resonant frequencies were found and data collection began. Changes in resonance frequency (ΔF) and dissipation (ΔD) were measured simultaneously. Decrease in ΔF was due to increase in the mass adsorbed onto the crystal. ΔD arises from the quotient of the energy dissipated during the period of oscillation and the energy stored in the oscillating system:49,50 The plot of ΔD/ΔF would reveal information on the change of the viscoelastic properties of the adsorbed materials.
After the immobilization of Aβ fibrils and the blocking steps, the flow was changed to PBS for at least 30 min during which time, the fibril was equilibrated with the buffer. Next, the flow was changed to Aβ monomer in the presence or absence of curcumin for 10 min and then returned to PBS for 10 min (Fig. 2).
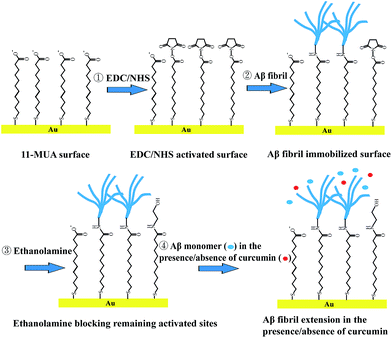 |
| Fig. 2 Schematic diagram of the immobilization and growth of Aβ fibrils in the absence and presence of curcumin. The surface of 11-MUA was activated by EDC/NHS (①). The activated surface was exposed to Aβ fibril to allow the amide bond to be formed between the amino group of the fibril and the carboxylic group on the 11-MUA surface (②). The unreacted sites were blocked by 1 M ethanolamine (③). The Aβ fibril immobilized surface was allowed to make contact with the Aβ monomer in the absence/presence of curcumin and the growth process was studied using QCM-D (④). | |
Statistical analysis
Data were presented as means ± standard errors of the mean (SEM). Linear regression was assessed by the value of the coefficient of determination, r2.
Results and discussion
Immobilization of Aβ fibrils on the sensor surface
To use QCM-D to study the growth kinetics of Aβ fibrils, a procedure for preparing the Aβ fibrils and stably immobilized them onto the crystal surface was developed. Aβ fibrils were first prepared according to the method described above. ThT assay indicated that orderly stacked β-sheets structures were formed in the Aβ aggregates (Fig. 3A). The ThT fluorescence reached the highest level at 48 h and remained stable thereafter. TEM image also confirmed the fibrillar nature of the prepared Aβ aggregates (Fig. 3B).
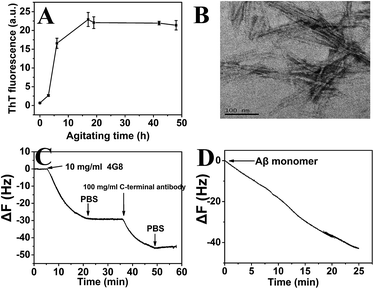 |
| Fig. 3 (A) Preparation of the Aβ fibril. Aliquots were examined in different time point by using ThT assay. Data were expressed as mean fluorescence [in arbitrary units (a.u.)] ± SEM. (B) Transmission electron microscopy image of prepared Aβ fibrils. Image shown is a representative of 20 examined fields. Scale bar represents 100 nm. (C) QCM-D analysis of binding between surface-bound Aβ fibril and two antibodies directed against the middle and C-terminal fragments of Aβ respectively. (D) Example of raw data from the QCM-D measurement Aβ fibril growth kinetics. | |
In order to minimize the effect of immobilization on the growth of the Aβ fibrils, amino groups were used to immobilize the Aβ fibrils. It has been reported that hydrophobic interaction and hydrogen bonding are chiefly responsible for the growth of Aβ fibrils.51,52 The N-terminal residues of Aβ are charged and are therefore expected to be in a random conformation, while the regions corresponding to Leu17–Ala21 (central hydrophobic cluster, CHC) and Ala30–Ala40 are hydrophobic and are thought to play a central role in the aggregation process (Fig. 1B). To confirm that the Aβ fibrils were successfully coupled to the sensor surface and their domains to form β sheets were not changed during coupling, affinity experiments were performed by QCM-D (Fig. 3C). The 4G8 antibody and C-terminal antibody can recognize Aβ (17–24) and C-terminal of Aβ40, respectively. The results showed that sequential injection of the two antibodies across the Aβ fibril-immobilized sensor surface induced a frequency decrease of 28.76 Hz (4G8 antibody) and 15.93 Hz (C-terminal antibody). The results indicated that the Aβ fibrils were firmly attached to the sensor surface and the immobilization process would not affect the aggregating behavior of the Aβ fibrils. Despite the present attachment procedure being effective and simple, recent studies have shown that residues at the N-terminus also confer some structural properties and might be important in the aggregation process, especially in Aβ peptides with familial mutation.53,54 Therefore, versatile immobilization methods need to be developed in future study.
During the injection of Aβ monomers into the flow chamber, both non-specific adsorption of Aβ monomers and growth of Aβ fibrils might occur. Thus, control experiments were conducted, first with the sensor surface being blocked with ethanolamine instead of coupling with Aβ fibrils. The results indicated that the non-specific adsorbed Aβ monomers did not cause any significant changes in the mass (generally <10% of total deposition in positive groups, Fig. S1†). Therefore, the binding responses in the following experiment might be mainly due to the monomers interacting with the Aβ fibrils. Previous study has demonstrated that the use of COOH-terminated self-assembled monolayer (SAM) resulted in the least adsorption of Aβ monomer to the SAM. Aβ peptide was negatively charged at physiological pH (∼7.4) and a negatively-charged COOH group can significantly reduce the adsorption of Aβ by electrostatic repulsion.55
Thus, QCM-D was used to measure the aggregation ability of the immobilized Aβ fibrils. A typical QCM-D experiment for the Aβ fibril growth kinetics is shown in Fig. 3D. After exposing the Aβ fibrils to the monomer solution, the frequency decreased, thereby confirming the increase in mass as a result of the attachment of Aβ monomers to the immobilized fibrils. Furthermore, the continuous growth of immobilized Aβ fibrils could be initiated when the monomers were again exposed to the fibrils (Fig. S2†). This indicated that the aggregation did not reach equilibrium and the fibril surface was not saturated. The result was not surprising since the process of Aβ fibril growth is a polymerization reaction characteristic of infinite aggregation.42,56 Therefore, Aβ could be continuously added to the immobilized fibrils in the presence or absence of curcumin.
Aβ monomer deposition was promoted in the presence of curcumin
To evaluate the effect of curcumin on the growth of Aβ fibril, QCM-D was chosen because with this technique it was possible to measure the rate of fibril growth based on mass change in real time. When 10, 15 and 20 μM of Aβ monomers were sequentially introduced to the Aβ fibrils for 10 min, both of them caused a linear increase in the mass of the fibrils (Fig. 4A). In order to evaluate the fibril elongation rate under different conditions, the induced frequency shift was fitted to a linear function, and the slope was taken as the growth rate for comparative study.45,57 The growth rate increased linearly with increasing Aβ monomer concentration. Compared to Aβ monomers, 5 μM to 40 μM curcumin alone also increased the mass of the fibrils in a dose-dependent manner, while the increase in mass was very low (Fig. 4B). Moreover, the frequency returned to baseline after the sensor surface was washed with PBS. These results suggested that the adsorption of curcumin onto Aβ fibrils was not strong enough to endure the PBS washing step. However, when 20 μM Aβ monomer were simultaneously injected with curcumin, the rates of mass increase obviously changed (Fig. 4C). In the presence of 5 μM, 10 μM and 20 μM curcumin, the rates of mass increase were respectively, 21.1%, 22.4% and 37.8% higher than that in the absence of curcumin. When the concentration of curcumin was further raised to 40 μM, the deposition rate was significantly increased, being 120.6% higher than that in the absence of curcumin. This indicated that curcumin could promote the deposition of Aβ monomer and the effect was dependent on its concentration (Fig. 4D). Earlier studies have indicated that curcumin can inhibit Aβ fibrils formation as well as their extension.32,34,58 Those results were obtained mainly based on ThT assay. However, a study by Caesar et al. indicates that curcumin does not decrease the amount of Aβ deposition, but instead, it accelerates the conversion of pre-fibrillar to fibril in Aβ transgenic flies.30 Recent in vitro study has also demonstrated that aggregation of Aβ monomer in the presence of curcumin is promoted.35 Therefore, the effect of curcumin on the aggregation tendency of Aβ is still not ascertained. Curcumin has the ability to bind both Aβ oligomers and fibrils,33 and the binding involves the interaction between the aromatic carbons of curcumin and the 12th and 17th to 21st residues of Aβ present in the β-sheet structure.59 According to the results of molecular dynamics simulations, the secondary structure of the β-sheets within the Aβ aggregates is decreased after treatment with curcumin.60 Changes in the structure of Aβ might make the normally accepted methods such as thioflavin T fluorescent (ThT) assay, circular dichroism and several antibodies which measure Aβ aggregation according to content of β-sheet not adaptable for curcumin. For example, ThT assay is widely used for monitoring the kinetics of Aβ fibrillation and evaluating the effect of exogenous compound on Aβ aggregation. Higher ThT values often indicate higher degree of Aβ aggregation. However, ThT assay is not suitable when exogenous compounds are coloured61 or can compete with ThT for binding to the fibril.62 ThT assay is also not suitable for Aβ aggregates which have less orderly stacked β-sheets contents even if the aggregates have β-sheet secondary structure.63 In fact, ThT assay is more appropriate for providing structural information. Moreover, recent studies have shown that curcumin has intrinsic fluorescence and can compete with ThT for binding with amyloid fibrils, thus limiting the application of the assay.62 Previous studies have shown that QCM is a powerful tool for directly monitoring the quantity of monomer incorporated into fibrils based on mass increase.45,64 In addition, it can also be used to probe the effect of small molecules and proteins on the growth of amyloid fibrils.65–67 Our result showed that the deposition of Aβ monomer was promoted in the presence of curcumin, further stressing that the rate of Aβ fibril growth in the presence of an exogenous compound such as curcumin should be assessed using a method other than a dye-based assay. It also demonstrated that QCM-D is a potent complementary method for assessing the fibril growth of Aβ. Since our result showed that deposition of Aβ monomers in the presence of curcumin was promoted, it further supported the findings from recent reports that curcumin promote formation of Aβ aggregates.30,35 We deduced that the incorporation of curcumin into the fibrils changed the structure of the fibrils, making them unsuitable to monitor by ThT assay. To examine this point, structural analysis was carried out and the result is illustrated in the following section.
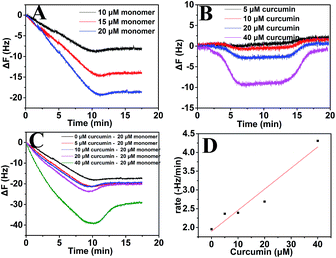 |
| Fig. 4 Monitoring of curcumin affecting surface-bounded Aβ fibrils growth. QCM-D measurements probing the mass increases of the immobilized fibrils in the absence or presence of curcumin to surface-bound Aβ fibrils. ΔF is proportional to the binding mass on the sensor chips. Results are representative of three independent experiments. (A) 10 μM (black line), 15 μM (red line) and 20 μM (blue line) Aβ monomer. (B) 5 (black), 10 (red), 20 (blue), 40 (pink) μM curcumin alone. (C) Aβ monomer in the presence of 0 (black), 5 (red), 10 (blue), 20 (pink) or 40 (green) μM curcumin. (D) The linear regression of aggregation rate during association process and Aβ monomer in the presence of different concentration of curcumin (r2 = 0.93). | |
Aβ monomer deposition in the presence of curcumin caused structural transition of Aβ fibril
Besides monitoring the mass change in real time, the advantage of QCM-D is that it can measure the dissipation change during the adsorption process, so that it can measure the change in viscoelasticity, which is related to the structural change of Aβ fibril tethered to the chip. Therefore, not only could we characterize the effect of curcumin on Aβ fibrils growth, but could also probe the mechanism of interaction. The plot of the energy dissipation versus the change in frequency (ΔD versus ΔF) would make it possible to qualitatively characterize the adsorbing materials. A low ΔD/ΔF value indicates mass addition without significant dissipation increase, which is characteristic of a fairly rigid adsorption. In contrast, a large ΔD/ΔF value signals a soft, dissipative adsorption.44,50 Through this type of analysis, the conformation of the Aβ monomers adsorbed to the Aβ fibril can therefore be deduced. The study of antigen–antibody interaction by QCM-D has shown that specific and non-specific interaction can be distinguished using a ΔD/ΔF plot, in which a higher slope of ΔD/ΔF is indicative of a more disordered antibody film.49 In a surface modification comparison study, the conformation of BSA immobilized on the surface can be deduced by ΔD/ΔF plot, the higher slope value of ΔD/ΔF confirms that the BSA layer is less rigid.68 As shown in Fig. 5A, C and E, ΔD was related to the concentrations of both Aβ monomer and curcumin. In the ΔD/ΔF plot for different concentrations of Aβ monomer, there exists an apparent linearity and all of the data fall on the same line (Fig. 5B). Taken together, the results indicated that the dissipative properties of the growing Aβ fibrils were independent of the monomer concentration and were the same whether the Aβ fibrils were preformed or grown from Aβ monomer addition. This was not surprising since in the study of the kinetics of the growth of polyglutamine peptide fibrils, the values of ΔD/ΔF were identical regardless of the monomer concentration or time of exposure. This further confirmed that the dissipative properties of the growing fibril were constant, consistent with our results.44 However, the slope of ΔD/ΔF started to change when the monomers were in the presence of curcumin. When the concentration of curcumin was increased from 5 μM to 40 μM, the slope gradually increased (Fig. 5D and F). As indicated above, with increasing curcumin concentrations, the viscoelasticity of the growing fibril increased, indicating the structure of the fibril had become softer. Further comparative analysis of endpoint ΔD/ΔF values of the adsorbing process and after rinsing with PBS was also conducted, which could be used to acquire the average structural information of the protein layer in the specific phase.68–72 Commonly, a less compact and softer adsorbed protein layer would lead to a higher endpoint ΔD/ΔF value and vice versa. Our results showed that with increasing curcumin concentrations, both the endpoint ΔD/ΔF values of the adsorbing process and after rinsing with PBS were gradually increased. The rinsing process has less significant effect on the final structure of the Aβ aggregates. Therefore, the final structures of the curcumin–Aβ aggregates were more dissipative and softer than the Aβ fibrils that underwent elongation in the absence of curcumin (Fig. S3†). All these results indicated that more curcumin and Aβ monomer could be introduced to the Aβ fibrils, thus making the structure more flexible. Previous studies have shown that curcumin can bind Aβ fibrils through hydrophobic interaction and hydrogen bond that mainly involve residue 12 and residues 17–21 in the middle region of the β-sheet within Aβ fibril.59 Other studies have also shown that π–π stacking between the aromatic rings of curcumin and side-chains of the residues in the binding hot spots, especially in the central hydrophobic cluster region (17th to 21st residues) of Aβ is important for their interaction.73,74 Therefore, we speculated that when curcumin and Aβ monomers were allowed to associate with the fibrils simultaneously, they could both be introduced to the β-sheet location in the fibrils. The interaction of curcumin with the middle β-sheet of the fibrils would disturb the initial hydrogen bond location for ordered β-sheet growth process. However, with curcumin providing additional hydrogen that can interact with the Aβ monomer, new monomers may deposit onto the fibrils through middle location interacting with curcumin-induced Aβ aggregates and C-terminal location interacting with aggregation sites of initial Aβ fibrils. Therefore new monomers could only be loosely attached to the fibril, and the growing aggregates would extend more from the surface and become softer compared to initial fibrils.
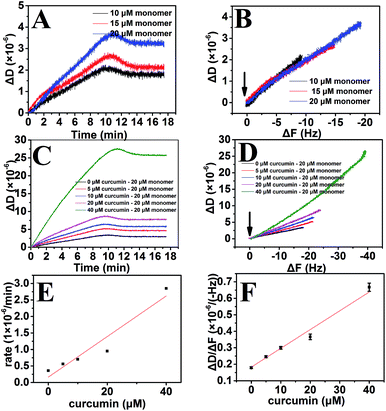 |
| Fig. 5 QCM-D measurements probing the dissipation (ΔD) of the fibril growing process in the absence or presence of curcumin and related ΔD/ΔF plot. Results are representative of three independent experiments. Dissipation measurement: (A) 10 (black line), 15 (red line) and 20 μM (blue line) Aβ monomer. (C) Aβ monomer in the presence of 0 (black), 5 (red), 10 (blue), 20 (pink) or 40 (green) μM curcumin. ΔD/ΔF plot of: 10 (black line), 15 (red line) and 20 μM (blue line) Aβ monomer (B) or Aβ monomer in the presence of 0 (black), 5 (red), 10 (blue), 20 (pink) or 40 (green) μM curcumin (D) to surface-bound Aβ fibrils. Arrow in the graph indicated the time at which the fibril began to contact with monomer in the absence or presence of curcumin. The linear regression of (E) dissipation change rate during association process and Aβ monomer in the presence of different concentration of curcumin (r2 = 0.90), (F) slope of ΔD/ΔF during association process and Aβ monomer in the presence of different concentration of curcumin (r2 = 0.97). | |
The promotion of Aβ monomer deposition by curcumin may have important influence in vivo. One study has shown that feeding transgenic flies with curcumin increases the deposition of Aβ which can be stained with a luminescent conjugated oligothiophene (LCO), p-FTAA.30 However, p-FTAA can interact with non-thioflavinophilic Aβ aggregates.75 Therefore, the deposition of Aβ stained by p-FTAA may include Aβ aggregates that do not have orderly stacked β-sheets structure. Our study further suggested that curcumin treatment may promote the formation of Aβ aggregates with structural characteristics that differed from orderly stacked fibrils. Therefore, the inhibitory effect of curcumin on Aβ aggregation in ThT assay could be ascribed to the interference of curcumin by the ThT assay, and curcumin-induced Aβ aggregates might not be examined in the ThT assay. In fact, the existence of curcumin could increase the quantity of curcumin-induced Aβ aggregates. Therefore, the results of our QCM-D experiments may provide a possible explanation for the discrepancy discussed above.
Curcumin-induced Aβ aggregates inhibit growth process
As described above, curcumin-induced aggregates have more flexible structure that differed from the orderly stacked β-sheet structure of the fibrils. Deposition of Aβ monomers on the curcumin-induced Aβ aggregates has not been reported. To better elucidate the effect of curcumin-induced aggregates on Aβ aggregation, surface-bound Aβ fibrils were exposed to Aβ monomer alone, Aβ monomer in the presence of curcumin followed by Aβ monomer alone. The results showed that the growth rates of Aβ monomer on curcumin-induced aggregates were significantly reduced (Fig. 6A and B). It was only 34.2% and 15.5% of those observed for the initial fibrils and 40 μM curcumin-induced aggregates, respectively. This suggested that growth of the curcumin-induced aggregates was distinguished from that of the fibrils with orderly stacked β-sheet structure. Our finding proved that curcumin-dependent Aβ deposition was responsible for the overall fibril structure being more flexible and able to inhibit further growth of the process. Previous studies by surface plasmon resonance (SPR) have also shown that reduced ordered β-sheet structure of aggregated Aβ caused by epigallocatechin gallate or metals can lead to a reduction of the elongation rate.76 Therefore, we speculated that the highly flexible structure of Aβ aggregates, regardless of how they came to be, could inhibit Aβ aggregation.
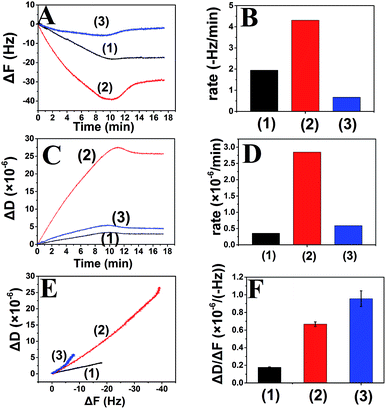 |
| Fig. 6 QCM-D measurements probing the frequency (ΔF) (A and B), dissipation (ΔD) (C and D) and ΔD/ΔF (E and F) of Aβ monomer upon its addition to curcumin-induced Aβ aggregates. Data are representatives of three independent experiments. Surface-bound Aβ fibrils were exposed to 20 μM Aβ monomer (1, black line), 20 μM monomer in the presence of 40 μM curcumin (2, red line) and 20 μM Aβ monomer alone again (3, blue line) in turn. Arrow in the (E) indicated the time at which the fibril began to contact with monomer in the absence or presence of curcumin. | |
When curcumin was depleted, the change in ΔD showed similar trend to that of ΔF (Fig. 6C and D). It was more important that the viscoelasticity of Aβ deposited on curcumin-induced aggregates be significantly increased compared to that of the initial Aβ fibrils (Fig. 6E and F). The results suggested that three different aggregation steps could give rise to the differences in the characteristics of the growing aggregates (Fig. 7). In the first step, the β-sheet-rich fibril structure remained unchanged as it grew. The β-sheet fibril structure was related to the low slope in the ΔD/ΔF plot. In the second step, introduction of the new hydrogen bond provided by curcumin promoted the deposition of Aβ monomers onto the fibrils, and deposition in the presence of curcumin caused the structure of the growing section to become more flexible, and this was reflected in a significant higher slope in the ΔD/ΔF plot. In the third step, because the structure of curcumin induced-aggregates differs from the initial fibril, the nature of the new binding sites was not appropriate for Aβ monomer extension to form β-sheet structure. Therefore, with the depletion of curcumin under the experimental condition, the aggregation process would be finally inhibited. In brief, the observation demonstrated that curcumin inhibited the growth of Aβ fibrils mainly through deactivating the growth sites by binding with these sites and with the monomer. This changed the structure of the fibrils and thus reduced their ability to grow.
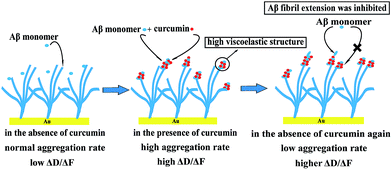 |
| Fig. 7 Three different aggregation steps of the growing Aβ aggregates. | |
The present study demonstrated that curcumin induced critical alteration to the Aβ fibril structure by directly influencing the deposition of monomers, which might be related to the therapeutic function of curcumin. Previous study has shown that curcumin can induce the formation of quasi-fibrillar77 and amorphous Aβ aggregates, which are different from ordered fibrils32 and some polyphenolic compounds can also promote formation of nontoxic off-pathway aggregates.36 Amorphous aggregates could be transferred to the proteasomal machinery for more readily degradation, and it has been considered as less toxic Aβ species.78,79 However, a recent study has reported that amorphous aggregates are more neurotoxic than ordered Aβ fibrils80 and the viscoelastic nature of Aβ species might be an important common property of aggregates related to their neurotoxicity.50 Moreover, it is assumed that higher toxic oligomers may increase as a result of inhibition of fibril formation. All of these have shown that more in depth research is needed and application of curcumin may need to proceed with caution, especially for patients who have developed abundant Aβ fibrils in the brain. Further investigation is needed to explore the physiological function of different structures of Aβ aggregates and to develop novel curcumin derivatives and conjugates to improve its therapeutic efficacy in the light of its limited solubility and stability in physiological condition.81,82
Conclusion
In this study, QCM-D was used to investigate the effect of curcumin on Aβ fibrillogenesis. The results demonstrated that, in the initial stage, curcumin promoted the deposition of Aβ monomer on the immobilized Aβ fibrils, but curcumin-induced deposition made the structure of the aggregates become more flexible compared to initial fibrils. Finally, this structural transition of the Aβ fibril further inhibited the growth process when curcumin was depleted. This study promoted the understanding of the mechanism by which curcumin may inhibit Aβ aggregation. Furthermore, it also showed that it is feasible to use QCM-D to provide a detailed study of the mechanism by which small molecules modulate amyloid aggregation. Such study may represent an important step toward better understanding of the drug that influences the aggregation of Aβ and one that may assist in the design of inhibitors and development of therapeutic strategies against AD and other neurodegenerative diseases.
Acknowledgements
We thank Dr Alan K. Chang for useful discussion. This work was supported by National Natural Science Foundation of China (no. 31100612 and 21104008) and the Fundamental Research Funds for the Central Universities (DUT10LK32 and DUT13LAB07).
Notes and references
- C. Ballard, S. Gauthier, A. Corbett, C. Brayne, D. Aarsland and E. Jones, Lancet, 2011, 377, 1019–1031 CrossRef.
- D. J. Selkoe, Science, 2012, 337, 1488–1492 CrossRef CAS PubMed.
- D. J. Selkoe, Physiol. Rev., 2001, 81, 741–766 CAS.
- M. Goedert and M. G. Spillantini, Science, 2006, 314, 777–781 CrossRef CAS PubMed.
- W. J. Strittmatter, Science, 2012, 335, 1447–1448 CrossRef CAS PubMed.
- R. Roychaudhuri, M. Yang, M. M. Hoshi and D. B. Teplow, J. Biol. Chem., 2009, 284, 4749–4753 CrossRef CAS PubMed.
- A. Thapa, B. C. Vernon, K. De la Pena, G. Soliz, H. A. Moreno, G. P. Lopez and E. Y. Chi, Langmuir, 2013, 29, 11713–11723 CrossRef CAS PubMed.
- K. G. Mawuenyega, W. Sigurdson, V. Ovod, L. Munsell, T. Kasten, J. C. Morris, K. E. Yarasheski and R. J. Bateman, Science, 2010, 330, 1774 CrossRef CAS PubMed.
- A. Morimoto, K. Irie, K. Murakami, Y. Masuda, H. Ohigashi, M. Nagao, H. Fukuda, T. Shimizu and T. Shirasawa, J. Biol. Chem., 2004, 279, 52781–52788 CrossRef CAS PubMed.
- A. S. Lakdawala, D. M. Morgan, D. C. Liotta, D. G. Lynn and J. P. Snyder, J. Am. Chem. Soc., 2002, 124, 15150–15151 CrossRef CAS PubMed.
- J. Hardy and D. J. Selkoe, Science, 2002, 297, 353–356 CrossRef CAS PubMed.
- S. Nag, B. Sarkar, A. Bandyopadhyay, B. Sahoo, V. K. Sreenivasan, M. Kombrabail, C. Muralidharan and S. Maiti, J. Biol. Chem., 2011, 286, 13827–13833 CrossRef CAS PubMed.
- A. J. Doig and P. Derreumaux, Curr. Opin. Struct. Biol., 2015, 30C, 50–56 CrossRef PubMed.
- B. Tarus, P. H. Nguyen, O. Berthoumieu, P. Faller, A. J. Doig and P. Derreumaux, Eur. J. Med. Chem., 2015, 91, 43–50 CrossRef CAS PubMed.
- M. Necula, R. Kayed, S. Milton and C. G. Glabe, J. Biol. Chem., 2007, 282, 10311–10324 CrossRef CAS PubMed.
- K. A. Dasilva, J. E. Shaw and J. McLaurin, Exp. Neurol., 2010, 223, 311–321 CrossRef CAS PubMed.
- Q. M. Wang, X. Yu, K. Patal, R. D. Hu, S. Chuang, G. Zhang and J. Zheng, ACS Chem. Neurosci., 2013, 4, 1004–1015 CrossRef CAS PubMed.
- T. Hard and C. Lendel, J. Mol. Biol., 2012, 421, 441–465 CrossRef PubMed.
- J. A. Lemkul and D. R. Bevan, ACS Chem. Neurosci., 2012, 3, 845–856 CrossRef CAS PubMed.
- Y. Chebaro and P. Derreumaux, Proteins, 2009, 75, 442–452 CrossRef CAS PubMed.
- T. Hamaguchi, K. Ono and M. Yamada, CNS Neurosci. Ther., 2010, 16, 285–297 CrossRef CAS PubMed.
- Y. Shen and L. C. Yu, Neurochem. Res., 2008, 33, 2112–2117 CrossRef CAS PubMed.
- M. S. Niu, S. J. Wu, L. Mao and Y. L. Yang, Traffic, 2013, 14, 1042–1052 CrossRef CAS PubMed.
- M. Salem, S. Rohani and E. R. Gillies, RSC Adv., 2014, 4, 10815–10829 RSC.
- C. Ramassamy, Eur. J. Pharmacol., 2006, 545, 51–64 CrossRef CAS PubMed.
- H. Hatcher, R. Planalp, J. Cho, F. M. Tortia and S. V. Torti, Cell. Mol. Life Sci., 2008, 65, 1631–1652 CrossRef CAS PubMed.
- G. P. Lim, T. Chu, F. S. Yang, W. Beech, S. A. Frautschy and G. M. Cole, J. Neurosci., 2001, 21, 8370–8377 CAS.
- S. A. Frautschy, W. Hu, P. Kim, S. A. Miller, T. Chu, M. E. Harris-White and G. M. Cole, Neurobiol. Aging, 2001, 22, 993–1005 CrossRef CAS.
- H. L. Yin, Y. L. Wang, J. F. Li, B. Han, X. X. Zhang, Y. T. Wang and S. Geng, GMR, Genet. Mol. Res., 2014, 13, 2039–2047 CrossRef CAS PubMed.
- I. Caesar, M. Jonson, K. P. R. Nilsson, S. Thor and P. Hammarstrom, PLoS One, 2012, 7, e31424 CAS.
- X. Y. Qin, Y. Cheng and L. C. Yu, Neurosci. Lett., 2010, 480, 21–24 CrossRef CAS PubMed.
- F. S. Yang, G. P. Lim, A. N. Begum, O. J. Ubeda, M. R. Simmons, S. S. Ambegaokar, P. P. Chen, R. Kayed, C. G. Glabe, S. A. Frautschy and G. M. Cole, J. Biol. Chem., 2005, 280, 5892–5901 CrossRef CAS PubMed.
- D. Yanagisawa, H. Taguchi, A. Yamamoto, N. Shirai, K. Hirao and I. Tooyama, J. Alzheimer's Dis., 2011, 24, 33–42 CAS.
- K. Ono, K. Hasegawa, H. Naiki and M. Yamada, J. Neurosci. Res., 2004, 75, 742–750 CrossRef CAS PubMed.
- J. N. Fawver, K. T. Duong, O. Wise-Scira, R. P. Chapa, H. E. Schall, O. Coskuner, X. W. Zhu, L. V. Colom and I. V. J. Murray, J. Alzheimer's Dis., 2012, 32, 197–215 CAS.
- A. Thapa, E. R. Woo, E. Y. Chi, M. G. Sharoar, H. G. Jin, S. Y. Shin and I. S. Park, Biochemistry, 2011, 50, 2445–2455 CrossRef CAS PubMed.
- M. Rodahl and B. Kasemo, Rev. Sci. Instrum., 1996, 67, 3238–3241 CrossRef CAS PubMed.
- A. K. Buell, D. A. White, C. Meier, M. E. Welland, T. P. Knowles and C. M. Dobson, J. Phys. Chem. B, 2010, 114, 10925–10938 CrossRef CAS PubMed.
- F. Cheng, J. Shang and D. M. Ratner, Bioconjugate Chem., 2011, 22, 50–57 CrossRef CAS PubMed.
- H. Ogi, Proc. Jpn. Acad., Ser. B, 2013, 89, 401–417 CrossRef CAS.
- B. Becker and M. A. Cooper, J. Mol. Recognit., 2011, 24, 754–787 CrossRef CAS PubMed.
- J. A. Kotarek, K. C. Johnson and M. A. Moss, Anal. Biochem., 2008, 378, 15–24 CrossRef CAS PubMed.
- H. Ogi, Y. Fukunishi, T. Yanagida, H. Yagi, Y. Goto, M. Fukushima, K. Uesugi and M. Hirao, Anal. Chem., 2011, 83, 4982–4988 CrossRef CAS PubMed.
- R. H. Walters, K. H. Jacobson, J. A. Pedersen and R. M. Murphy, J. Mol. Biol., 2012, 421, 329–347 CrossRef CAS PubMed.
- T. P. Knowles, W. Shu, G. L. Devlin, S. Meehan, S. Auer, C. M. Dobson and M. E. Welland, Proc. Natl. Acad. Sci. U. S. A., 2007, 104, 10016–10021 CrossRef CAS PubMed.
- H. Okuno, K. Mori, T. Okada, Y. Yokoyama and H. Suzuki, Chem. Biol. Drug Des., 2007, 69, 356–361 CAS.
- M. B. Hovgaard, M. D. Dong, D. E. Otzen and F. Besenbacher, Biophys. J., 2007, 93, 2162–2169 CrossRef CAS PubMed.
- M. K. Mustafa, A. Nabok, D. Parkinson, I. E. Tothill, F. Salam and A. Tsargorodskaya, Biosens. Bioelectron., 2010, 26, 1332–1336 CrossRef CAS PubMed.
- M. Bianco, A. Aloisi, V. Arima, M. Capello, S. Ferri-Borgogno, F. Novelli, S. Leporatti and R. Rinaldi, Biosens. Bioelectron., 2013, 42, 646–652 CrossRef CAS PubMed.
- A. M. Saraiva, M. C. Pereira and G. Brezesinski, Langmuir, 2010, 26, 12060–12067 CrossRef CAS PubMed.
- A. T. Petkova, Y. Ishii, J. J. Balbach, O. N. Antzutkin, R. D. Leapman, F. Delaglio and R. Tycko, Proc. Natl. Acad. Sci. U. S. A., 2002, 99, 16742–16747 CrossRef CAS PubMed.
- J. D. Schmit, K. Ghosh and K. Dill, Biophys. J., 2011, 100, 450–458 CrossRef CAS PubMed.
- P. H. Nguyen, B. Tarus and P. Derreumaux, J. Phys. Chem. B, 2014, 118, 501–510 CrossRef CAS PubMed.
- M. H. Viet, P. H. Nguyen, P. Derreumaux and M. S. Li, ACS Chem. Neurosci., 2014, 5, 646–657 CrossRef CAS PubMed.
- Q. M. Wang, N. Shah, J. Zhao, C. S. Wang, C. Zhao, L. Y. Liu, L. Y. Li, F. M. Zhou and J. Zheng, Phys. Chem. Chem. Phys., 2011, 13, 15200–15210 RSC.
- M. J. Cannon, A. D. Williams, R. Wetzel and D. G. Myszka, Anal. Biochem., 2004, 328, 67–75 CrossRef CAS PubMed.
- L. Q. Xu, S. Wu, A. K. Buell, S. I. A. Cohen, L. J. Chen, W. H. Hu, S. A. Cusack, L. S. Itzhaki, H. Zhang, T. P. J. Knowles, C. M. Dobson, M. E. Welland, G. W. Jones and S. Perrett, Philos. Trans. R. Soc., B, 2013, 368, 20110410 CrossRef PubMed.
- H. Kim, B. S. Park, K. G. Lee, C. Y. Choi, S. S. Jang, Y. H. Kim and S. E. Lee, J. Agric. Food Chem., 2005, 53, 8537–8541 CrossRef CAS PubMed.
- Y. Masuda, M. Fukuchi, T. Yatagawa, M. Tada, K. Takeda, K. Irie, K. Akagi, Y. Monobe, T. Imazawa and K. Takegoshi, Bioorg. Med. Chem., 2011, 19, 5967–5974 CrossRef CAS PubMed.
- L. N. Zhao, S. W. Chiu, J. Benoit, L. Y. Chew and Y. Mu, J. Phys. Chem. B, 2012, 116, 7428–7435 CrossRef CAS PubMed.
- H. LeVine 3rd, Methods Enzymol., 1999, 309, 274–284 CAS.
- S. A. Hudson, H. Ecroyd, T. W. Kee and J. A. Carver, FEBS J., 2009, 276, 5960–5972 CrossRef CAS PubMed.
- D. Jiang, I. Rauda, S. Han, S. Chen and F. Zhou, Langmuir, 2012, 28, 12711–12721 CrossRef CAS PubMed.
- A. K. Buell, C. M. Dobson, T. P. J. Knowles and M. E. Welland, Biophys. J., 2010, 99, 3492–3497 CrossRef CAS PubMed.
- M. Suenaga, H. Takahashi, H. Imagawa, M. Wagatsuma, S. Ouma, Y. Tsuboi, A. Furuta and Y. Matsunaga, Curr. Alzheimer Res., 2014, 11, 745–754 CrossRef CAS.
- S. L. Shammas, C. A. Waudby, S. Y. Wang, A. K. Buell, T. P. J. Knowles, H. Ecroyd, M. E. Welland, J. A. Carver, C. M. Dobson and S. Meehan, Biophys. J., 2011, 101, 1681–1689 CrossRef CAS PubMed.
- C. A. Waudby, T. P. J. Knowles, G. L. Devlin, J. N. Skepper, H. Ecroyd, J. A. Carver, M. E. Welland, J. Christodoulou, C. M. Dobson and S. Meehan, Biophys. J., 2010, 98, 843–851 CrossRef CAS PubMed.
- A. Cifuentes and S. Borros, Langmuir, 2013, 29, 6645–6651 CrossRef CAS PubMed.
- M. Rodahl, F. Hook, C. Fredriksson, C. A. Keller, A. Krozer, P. Brzezinski, M. Voinova and B. Kasemo, Faraday Discuss., 1997, 107, 229–246 RSC.
- A. Dolatshahi-Pirouz, K. Rechendorff, M. B. Hovgaard, M. Foss, J. Chevallier and F. Besenbacher, Colloids Surf., B, 2008, 66, 53–59 CrossRef CAS PubMed.
- F. Hook, M. Rodahl, P. Brzezinski and B. Kasemo, J. Colloid Interface Sci., 1998, 208, 63–67 CrossRef CAS PubMed.
- F. Hook, J. Voros, M. Rodahl, R. Kurrat, P. Boni, J. J. Ramsden, M. Textor, N. D. Spencer, P. Tengvall, J. Gold and B. Kasemo, Colloids Surf., B, 2002, 24, 155–170 CrossRef CAS.
- M. Zhu, A. De Simone, D. Schenk, G. Toth, C. M. Dobson and M. Vendruscolo, J. Chem. Phys., 2013, 139, 035101 CrossRef PubMed.
- Y. Chebaro, P. Jiang, T. Zang, Y. G. Mu, P. H. Nguyen, N. Mousseau and P. Derreumaux, J. Phys. Chem. B, 2012, 116, 8412–8422 CrossRef CAS PubMed.
- A. Aslund, C. J. Sigurdson, T. Klingstedt, S. Grathwohl, T. Bolmont, D. L. Dickstein, E. Glimsdal, S. Prokop, M. Lindgren, P. Konradsson, D. M. Holtzman, P. R. Hof, F. L. Heppner, S. Gandy, M. Jucker, A. Aguzzi, P. Hammarstrom and K. P. Nilsson, ACS Chem. Biol., 2009, 4, 673–684 CrossRef PubMed.
- X. R. Cheng, B. Y. Hau, A. J. Veloso, S. Martic, H. B. Kraatz and K. Kerman, Anal. Chem., 2013, 85, 2049–2055 CrossRef CAS PubMed.
- V. S. Mithu, B. Sarkar, D. Bhowmik, A. K. Das, M. Chandrakesan, S. Maiti and P. K. Madhu, J. Biol. Chem., 2014, 289, 11122–11131 CrossRef CAS PubMed.
- C. J. Sarell, S. R. Wilkinson and J. H. Viles, J. Biol. Chem., 2010, 285, 41533–41540 CrossRef CAS PubMed.
- N. Rezaei-Ghaleh, K. Giller, S. Becker and M. Zweckstetter, Biophys. J., 2011, 101, 1202–1211 CrossRef CAS PubMed.
- X. Dai, Y. Sun, Z. Gao and Z. Jiang, J. Mol. Neurosci., 2010, 41, 66–73 CrossRef CAS PubMed.
- D. Yanagisawa, N. F. Ibrahim, H. Taguchi, S. Morikawa, K. Hirao, N. Shirai, T. Sogabe and I. Tooyama, Neurobiol. Aging, 2015, 36, 201–210 CrossRef CAS PubMed.
- S. Palmal, A. R. Maity, B. K. Singh, S. Basu, N. R. Jana and N. R. Jana, Chem.–Eur. J., 2014, 20, 6184–6191 CrossRef CAS PubMed.
Footnote |
† Electronic supplementary information (ESI) available: Nonspecific adsorption on Aβ monomer on ethanolamine blocked surface, continuous growth of immobilized Aβ fibril by monomer addition, and endpoint ΔD/ΔF values of adsorbing process and rinsing with PBS. See DOI: 10.1039/c5ra02314a |
|
This journal is © The Royal Society of Chemistry 2015 |
Click here to see how this site uses Cookies. View our privacy policy here.