DOI:
10.1039/C6LC00895J
(Communication)
Lab Chip, 2016,
16, 3689-3694
Measurement of the hematocrit using paper-based microfluidic devices†
Received
14th July 2016
, Accepted 30th August 2016
First published on 30th August 2016
Abstract
The quantification of blood cells provides critical information about a patient's health status. Sophisticated analytical equipment, such as hematology analyzers, have been developed to perform these measurements, but limited-resource settings often lack the infrastructure required to purchase, operate, and maintain instrumentation. To address these practical challenges, paper-based microfluidic devices have emerged as a platform to develop diagnostic assays specifically for use at the point-of-care. To date, paper-based microfluidic devices have been used broadly in diagnostic assays that apply immunoassay, clinical chemistry, and electrochemistry techniques. The analysis of cells, however, has been largely overlooked. In this communication, we demonstrate a paper-based microfluidic device that enables the controlled transport of red blood cells (RBCs) and the measurement of the hematocrit—the ratio of RBC packed cell volume to total volume of whole blood. The properties of paper, device treatment, and device geometry affect the overall extent and reproducibility of transport of RBCs. Ultimately, we developed an inexpensive (US$0.03 per device) thermometer-styled device where the distance traveled by RBCs is proportional to the hematocrit. These results provide a foundation for the design of paper-based microfluidic devices that enable the separation and detection of cells in limited-resource settings.
Introduction
A critical hematological index—the hematocrit—is the ratio of the packed red blood cell (RBC) volume to the total volume of blood. In a healthy adult, the hematocrit can range from 35–47% for women and 41–54% for men.1 Deviations from these ranges can be used to aid diagnosis of certain conditions related to health status, such as dehydration, which is indicated at higher percentages,2 or anemia, which is indicated at lower percentages in patients.3 The hematocrit may be determined using techniques that range from simple (e.g., centrifugation)4 to sophisticated (e.g., automated analyzers).5 Upon application of a centrifugal field, cells suspended within blood plasma will sediment and stratify based on their densities. This process results in a pellet of RBCs packed densely at the bottom of a sample container. When using a cylindrical microcapillary tube, the hematocrit is determined as the ratio of the length of the microcapillary occupied by the packed cell volume (PCV) of RBCs compared to the total length occupied by the sample.6 In contrast, hematology analyzers use a combination of impedance measurements, differential lysis, and light scattering techniques to measure hematological indices.7 The hematocrit is calculated from two measured parameters related to RBCs: total count and mean corpuscular volume.6
Centrifuges and hematology analyzers are not readily available in limited-resource settings due to the high costs associated with their purchase, maintenance, and routine use. A number of alternative technologies have been developed to address some of the restrictions presented by limited-resource settings for the separation of blood components. For example, centrifuges that rely on simple mechanical power have been used to determine the hematocrit8 or separate plasma for analysis of solutes by clinical chemistry,9 while centrifuges powered by rechargeable batteries have enabled the diagnosis of sickle cell disease at the point-of-care.10 However, these approaches rely on prolonged manual labor or access to a power source, and, while less expensive than traditional laboratory equipment, they still require the purchase of modified instrumentation in addition to any consumables needed to perform a separation or analysis.
Since the definition of the hematocrit is a ratio, an alternative interpretation of this measurement considers the total volume of plasma in blood rather than the PCV. Thus, a simple method to quantify the volume of the liquid component of blood would greatly facilitate the determination of the hematocrit at the point-of-care. Paper-based microfluidic devices can offer such an opportunity to meter liquids. Fluids wick through paper due to capillary action. Patterning paper either with hydrophobic barriers or by cutting can control wicking by defining hydrophilic channels within planar (i.e., two-dimensional)11 or multilayer (i.e., three-dimensional)12,13 devices. In addition to their ability to control the flow of fluids, paper-based microfluidic devices offer a number of additional advantages as a platform for the development of point-of-care diagnostic assays: (i) paper is inexpensive and widely available;14 (ii) reagents can be stored within paper-based devices;15–17 (iii) paper-based microfluidic devices can be prototyped and manufactured rapidly (ca. minutes)18 using relatively simple techniques (e.g., wax printing);19 (iv) three-dimensional devices can support a wide range of sophisticated microfluidic functions.20–22
To date, applications of paper-based microfluidic devices have focused on the detection of soluble analytes (e.g., small molecules or proteins).23–27 For those assays using blood as the biological sample, cells must first be removed prior to the analysis of the plasma in order to ensure that wicking is reproducible.28,29 Blood cells have diameters on the order of 6–14 μm and vary in stiffness and deformability.30–32 If the grade of paper used to fabricate paper-based microfluidic devices has a small average pore size, then, by blocking pores, cells may affect the wicking of plasma or impede it entirely. It has also been shown that the type of fiber (e.g., from hard or soft woods) influences the interaction between paper and cells.33 Irreproducible flow can result in assays with diminished or unreliable analytical performance. To address this problem, plasma separation membranes are often integrated into paper-based microfluidic devices in order to purify plasma prior to analysis.23,29 Moreover, by excluding cells from the device, plasma separation membranes remove non-specific contributions to an observed signal. For example, the presence of RBCs within a paper-based microfluidic device can interfere with the interpretation of colorimetric assays because of the intrinsic red color of hemoglobin.28 The color of hemoglobin, however, can enable the use of paper-based microfluidic devices in agglutination assays (e.g., to determine blood type34,35 or plasma separation28) or to determine the solubility of hemoglobin (e.g., to diagnose sickle cell disease).36 These approaches all rely on impeding, rather than enabling, the migration of RBCs. To date, no method has been developed for the controlled transport of blood cells in paper-based microfluidic devices.
In this communication, we describe a paper-based microfluidic device that permits the transport of RBCs with the wicking front of plasma. We demonstrate how the properties of the device—pore size of paper, treatment with reagents, and channel geometry—affect the extent and reproducibility of transport of RBCs in whole blood. We exploited the relationship between the properties of paper and cells to develop a paper-based microfluidic device where the distance traveled by RBCs in a thermometer-styled channel can be used to determine the hematocrit of whole blood.
Results and discussion
Design of the paper-based device
Distance-based measurements have been used previously to enable the quantitative detection of solutes using microfluidic devices composed of single layers of paper.37–39 We developed a three-dimensional paper-based device that combines vertical and lateral channels to control and direct the flow of cells in a sample of applied whole blood (Fig. 1). This device is composed of two layers of chromatography paper. The top layer serves as a sample addition layer, which is used to prohibit white blood cells (WBCs) from entering the channel in the bottom layer and interfering with the operation of the device (i.e., impeding flow by blocking pores). The bottom layer is designed with a thermometer-styled readout to facilitate the determination of RBC transport distances. The two layers of chromatography paper are affixed to each other using double-sided permanent adhesive that is patterned using a robotic cutting plotter. Once assembled, each face of the device is sealed with an adhesive sheet of laminate. The laminate protects the user from contamination, reduces the evaporation of the sample during the assay, and allows a clear field of view for the user to observe the readout. The color of RBCs provides a simple means to measure transport distances within the thermometer channel.
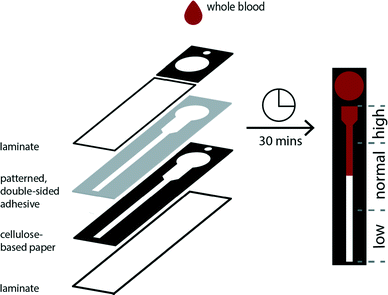 |
| Fig. 1 Schematic of a paper-based microfluidic device used to measure the hematocrit of whole blood. The black regions represent the hydrophobic zones patterned by wax printing and the white regions are the hydrophilic zones of the paper. The gray layer denotes the patterned, double-sided adhesive used to affix the two layers of chromatography paper. The guides indicate distances traveled by red blood cells in the device that correlate to high, normal, and low hematocrits. After a 50 μL sample of whole blood is applied, an incubation period of thirty minutes is required before a measurement is obtained. | |
We evaluated several thermometer designs to determine the device geometry that provided the most resolved and reproducible transport distances for RBCs (Fig. S1†). The final design uses segmented elements instead of a single straight channel to permit the wicking of whole blood over a large range of hematocrits (ESI†). We designed the thermometer with an initial wide, short segment that permitted the loading and visualization of higher hematocrits—characterized by a high concentration of cells and low volume of plasma—in the channel by minimizing the aggregation of cells. Conversely, lower hematocrits, which have a lower concentration of cells and larger volumes of plasma, are determined within a thinner, longer channel whose dimensions allow for improved resolution of transport distances of the sample (Fig. S2†).
Preparation of samples of plasma and whole blood
We acquired blood from a local supplier (Research Blood Components, Brighton, MA, USA), which allowed experiments to be performed the same day blood was drawn from donors. We determined the hematocrit of each donor using centrifugation to measure the PCV. We prepared a desired range of hematocrits by either removing or adding plasma. All plasma was derived from the original donor sample.
Relationship between transport distance and grade of paper
We evaluated the performance of paper-based microfluidic devices fabricated from commercially available chromatography papers that varied in reported average particle retention size: 6 μm, 10 μm, 15 μm, and 25 μm (ESI†). For our application, we desired a paper that enabled reproducible transport of RBCs and could effectively remove WBCs through filtration. Since WBCs have a larger diameter and are less deformable than RBCs,32 their presence within the lateral channel could interfere with transport of RBCs and the performance of the assay. We compared these four types of paper using: (i) volumes of plasma that correspond to the desired range of hematocrits and (ii) samples of whole blood (Fig. S3 and S4†).
In assays performed using samples of plasma, we expected to observe a linear relationship between the volume added and the measured wicking distance. Different grades of paper, however, could produce unique slopes (i.e., transport resolution) based on differences in their material characteristics (e.g., thickness and porosity). We observed a poor linear response with devices fabricated from 6 μm paper. Other grades of paper offered improved performance; the 15 μm paper achieved the best linear response and resolution over the range of plasma volumes (Fig. S3†). When evaluating samples of whole blood, the 15 μm paper promoted the transport of RBCs with the highest resolution and linearity. The 6 μm paper—whose average particle retention size is similar to the diameter of a red blood cell—hindered the transport of RBCs in this range of hematocrits. While it was characterized by the largest particle retention size of the papers evaluated, devices fabricated from 25 μm paper did not offer an improvement in transport of RBCs over those prepared from 15 μm paper (Fig. 2). We attribute this performance, in part, to the relatively large thickness of this grade of paper. In comparison to thinner papers, a sample must fill a greater pore volume per area before it can wick laterally, which results in lower resolution. We observed this trend in experiments using both plasma and whole blood.
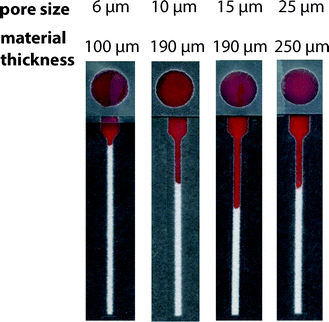 |
| Fig. 2 Transport of whole blood on devices fabricated using different grades of papers. Images demonstrate how the properties of the paper, which differed in reported particle retention size (6–25 μm) and in thickness (100–250 μm), used to manufacture paper-based microfluidic devices affected the transport of red blood cells. The sample of whole blood applied to these devices had a hematocrit of 45%. | |
We evaluated the ability of WBCs to travel through these papers using cultured T cells (D1.1 Jurkat) as a representative cell type for the WBC population (ESI†). We labeled T cells with DiIC18(3), a fluorescent general membrane stain, which allowed the use of fluorescence microscopy to determine their transport distances within the hematocrit device (Fig. S5†). We observed that WBCs remained primarily on the sample layer for all four types of paper. Ultimately, we chose the 15 μm paper as the material for our device because it permitted the reproducible transport of only RBCs in samples of whole blood.
Treatment of paper-based microfluidic devices
Although devices prepared from 15 μm paper provided a linear response over the desired range of hematocrits, the results using whole blood did not match those from plasma alone (Fig. S6†). Notably, samples of whole blood at high hematocrits (>45%) did not enter the lateral channel of the device as expected. While whole blood is more complex than plasma, these results suggested that the volume of liquid in the sample was not the only parameter that controlled the performance of the assay. Since the paper in these devices was untreated, one source of these variations could be non-specific interactions between the paper and blood cells. We pre-treated the lateral channel with blocking agents (e.g., casein or bovine serum albumin) traditionally used to minimize non-specific adsorption of proteins in immunoassays.40 However, we observed that blocking adversely affected the performance of the hematocrit assay by impeding transport of RBCs and reducing the reproducibility of filling the device (data not shown). We next treated the paper with additives: ethylenediaminetetraacetic acid (EDTA) and NaCl (see ESI† for experimental details). EDTA is already present in our samples of whole blood as the anti-coagulant. If EDTA adsorbs to paper during the assay, the concentration of EDTA in plasma could decrease and lead to the agglutination of RBCs and the subsequent blocking of pores within the paper-based microfluidic device. This effect would be most prominent at high hematocrits. Additional EDTA could stabilize the sample of blood during the assay. Adding NaCl to the device increases the osmolality of blood plasma and creates a hypertonic environment. To reestablish equilibrium, RBCs release water and reduce in size, both of which could improve transport of RBCs in paper-based microfluidic devices. If NaCl is added in excess, however, RBCs could become crenated and stiff, which could affect their transport.41 We determined that treating devices with 4.5 mM EDTA and 50 mM NaCl provided a superior linear response over the full range of clinically-relevant hematocrits. Importantly, using these conditions, transport distances for plasma and whole blood were now in excellent agreement (Fig. 3). Assays are completed in approximately 30 minutes. This duration reflects the time required for low hematocrits to be transported the full length of the device (ca. cm). Images of completed assays using fully treated devices demonstrate how transport distances vary for different hematocrits (Fig. 4) and how visual inspection might be used to determine if the hematocrit falls outside of a normal range.
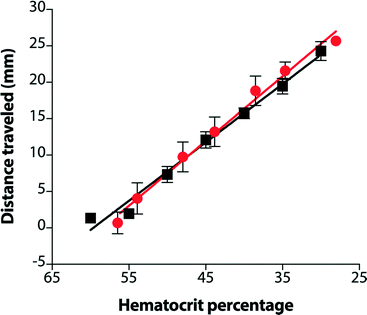 |
| Fig. 3 Comparison of the distances traveled by plasma (black) and whole blood (red) in a paper-based microfluidic device over a range of prepared hematocrits. Samples of whole blood were treated with EDTA as anti-coagulant. Paper-based microfluidic devices were treated with NaCl and EDTA to improve the transport of red blood cells in the device. The solid lines are the linear fit of the data (whole blood: R2 = 0.991; plasma only: R2 = 0.986). Each data point is the average of five technical replicates and the error bars are standard error of the mean. | |
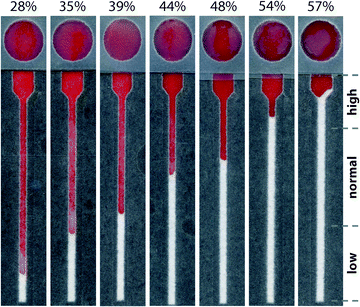 |
| Fig. 4 Representative images of paper-based microfluidic devices used to determine the hematocrits of whole blood. Images correspond to hematocrits that span a range from 28–57%. At a high hematocrit (ca. 57%), a sample of whole blood cannot enter the narrow channel because there is an insufficient volume of plasma to fill this zone of the device. For all other samples, red blood cells are transported a distance that is proportional to their hematocrit. | |
Reproducibility of hematocrit assay
We investigated the reproducibility of the hematocrit assay using: (i) multiple replicates of whole blood applied directly from the vacutainer (i.e., no sample preparation prior to analysis), (ii) multiple donors, and (iii) multiple anti-coagulants. We added a sample of EDTA-treated whole blood with a hematocrit of 42% to twenty devices. The average RBC transport distance was 20.1 ± 3.2 mm (Fig. 5). These devices had a coefficient of variation (CV) of 16% when the results of two outliers were excluded from analysis. We next examined the performance of the hematocrit assay using samples of whole blood treated with either EDTA or sodium heparin (NaHep) as the anti-coagulant (N = 3 donors each). We observed large variations between donors: for blood treated with EDTA, while the relationship between the hematocrit and the measured transport distance remained linear, the responses were dissimilar (Fig. S9†). In samples of blood treated with NaHep, however, RBC transport distances were irreproducible and did not maintain the expected linear relationship (Fig. S10†).
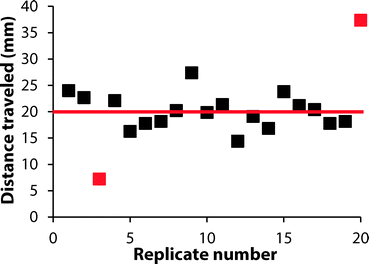 |
| Fig. 5 Demonstration of the reproducibility of the hematocrit assay using the paper-based microfluidic device. These assays were performed on paper-based microfluidic devices treated with EDTA and NaCl. A sample of whole blood, whose hematocrit was determined to be 42%, was applied to twenty devices. The average distance the sample traveled was 20.1 ± 3.2 mm (red line) and the coefficient of variation (CV) was 16%. The red squares mark the outliers of the data set, which were determined using the Grubb's test (α = 0.05). | |
Overall, these results suggest that there are limitations to this current iteration of the paper-based hematocrit assay: (i) RBC transport distances are not quantitative and they cannot be used to calculate a hematocrit directly from a measured distance. However, since the hematocrit is interpreted clinically in high, normal, and low ranges, we expect that a semi-quantitative assay using “bins” of transport distances would be valuable at a point-of-care setting. (ii) The type of anti-coagulant influences the transport of RBCs in paper-based devices. We optimized devices for use with EDTA-treated whole blood; alternative anti-coagulants may require unique device pre-treatments.
Conclusion
We have introduced a new class of point-of-care diagnostic assays by developing a paper-based microfluidic device for the measurement of the hematocrit of whole blood. While we prepared ranges of hematocrits using a small pool of healthy donors, future applications of these devices will include their use to evaluate clinical samples for dehydration (i.e., a high hematocrit) and anemia (i.e., a low hematocrit). Hematocrit devices are composed of paper and tape, and the only reagents required to perform the assay are small amounts of EDTA and NaCl. As a result, these devices can be disposed of easily and the cost to manufacture them at a laboratory scale is exceptionally low—approximately US$0.03 per device (Table S1†). The hematocrit assay operates by metering the liquid volume (i.e., plasma) of an applied sample of blood, but relies on the unimpeded transport of red blood cells through paper. It is also, therefore, critical to apply a controlled volume of blood to the device. We currently use a micropipette to dispense blood, but alternative methods (e.g., by filling a microcapillary tube) may be needed for the use of this device at the point-of-care. We demonstrate that the properties of paper (e.g., pore size) play a critical role in controlling how cells interact with the device. The careful selection of materials can, for example, selectively filter white blood cells while still permitting red blood cells to be transported by the wicking plasma. Although we focus only on the transport of red blood cells in this manuscript, we expect it will be possible to apply these design rules to develop a broad class of inexpensive and disposable paper-based cytometers.
Acknowledgements
This work was supported by Tufts University and by a generous gift from Dr. James Kanagy. This material is based upon work supported by the National Science Foundation Graduate Research Fellowship Program under Grant No. (DGE-1325256) that was awarded to S. C. F.
References
- R. Giorno, J. H. Clifford, S. Beverly and R. G. Rossing, Am. J. Clin. Pathol., 1980, 74, 765–770 CrossRef CAS PubMed.
- D. B. Dill and D. L. Costill, J. Appl. Physiol., 1974, 37, 247–248 CAS.
- M. Kosiborod, G. L. Smith, M. J. Radford, J. M. Foody and H. M. Krumholz, Am. J. Med., 2003, 114, 112–119 CrossRef PubMed.
- P. B. Goldenfarb, F. P. Bowyer, E. Hall and E. Brosious, Am. J. Clin. Pathol., 1971, 56, 35–39 CrossRef CAS PubMed.
- M. Fleisher, M. Gladstone, D. Crystal and M. K. Schwartz, Clin. Chem., 1989, 35, 1532–1535 CAS.
-
H. H. Billet, in Clinical Methods: The History, Physical, and Laboratory Examinations, ed. H. K. Walter, W. D. Hall and J. W. Hurst, Butterworths, Boston, 3rd edn, 1990, ch. 151, pp. 718–719 Search PubMed.
-
J. C. Aster, in Pathophysiology of Blood Disorders, ed. H. Bunn and J. C. Aster, McGraw-Hill, New York, 1st edn, 2011, ch. 1, pp. 1–13 Search PubMed.
- J. Brown, L. Theis, L. Kerr, N. Zakhidova, K. O'Connor, M. Uthman, M. Oden and R. Richards-Kortum, Am. J. Trop. Med. Hyg., 2011, 85, 327–332 CrossRef PubMed.
- A. P. Wong, M. Gupta, S. S. Shevkoplyas and G. M. Whitesides, Lab Chip, 2008, 8, 2032–2037 RSC.
- A. A. Kumar, M. R. Patton, J. W. Hennek, S. Y. R. Lee, G. D'Alesio-Spina, X. X. Yang, J. Kanter, S. S. Shevkopylas, C. Brugnara and G. M. Whitesides, Proc. Natl. Acad. Sci. U. S. A., 2014, 111, 14864–14869 CrossRef CAS PubMed.
- E. Fu, T. Liang, P. Spicar-Mihalic, J. Houghtaling, S. Ramachandran and P. Yager, Anal. Chem., 2012, 84, 4574–4579 CrossRef CAS PubMed.
- A. W. Martinez, S. T. Phillips and G. M. Whitesides, Proc. Natl. Acad. Sci. U. S. A., 2008, 105, 19606–19611 CrossRef CAS PubMed.
- H. Liu and R. M. Crooks, J. Am. Chem. Soc., 2011, 133, 17564–17566 CrossRef CAS PubMed.
- A. W. Martinez, S. T. Phillips, G. M. Whitesides and E. Carrilho, Anal. Chem., 2010, 82, 3–10 CrossRef CAS PubMed.
- A. W. Martinez, S. T. Phillips, M. J. Butte and G. M. Whitesides, Angew. Chem., Int. Ed., 2007, 46, 1318–1320 CrossRef CAS PubMed.
- G. E. Fridley, H. Q. Le, E. Fu and P. Yager, Lab Chip, 2012, 12, 4321–4327 RSC.
- J. E. Schonhorn, S. C. Fernandes, A. Rajaratnam, R. N. Deraney, J. P. Rolland and C. R. Mace, Lab Chip, 2014, 14, 4653–4658 RSC.
- C. R. Mace and R. N. Deraney, Microfluid. Nanofluid., 2014, 16, 801–809 CrossRef CAS.
- E. Carrilho, A. W. Martinez and G. M. Whitesides, Anal. Chem., 2009, 81, 7091–7095 CrossRef CAS PubMed.
- H. Noh and S. T. Phillips, Anal. Chem., 2010, 82, 8071–8078 CrossRef CAS PubMed.
- A. K. Yetisen, M. S. Akram and C. Lowe, Lab Chip, 2013, 13, 2210–2251 RSC.
- J. T. Connelly, J. P. Rolland and G. M. Whitesides, Anal. Chem., 2015, 87, 7595–7601 CrossRef CAS PubMed.
- N. R. Pollock, J. P. Rolland, S. Kumar, P. D. Beattie, S. Jain, F. Noubary, V. L. Wong, R. A. Pohlmann, U. S. Ryan and G. M. Whitesides, Sci. Transl. Med., 2012, 4, 152ra129 Search PubMed.
- S. Y. Wong, M. Cabodi, J. Rolland and C. M. Klapperich, Anal. Chem., 2014, 86, 11981–11985 CrossRef CAS PubMed.
- J. Noiphung, T. Songjaroen, W. Dungchai, C. S. Henry, O. Chailapakul and W. Laiwattanapaisal, Anal. Chim. Acta, 2013, 788, 39–45 CrossRef CAS PubMed.
- P. R. DeGregory, Y.-J. Tsai, K. Scida, I. Richards and R. M. Crooks, Analyst, 2016, 141, 1734–1744 RSC.
- R. N. Deraney, C. R. Mace, J. P. Rolland and J. E. Schonhorn, Anal. Chem., 2016, 88, 6161–6165 CrossRef CAS PubMed.
- X. Yang, O. Forouzan, T. P. Brown and S. S. Shevkoplyas, Lab Chip, 2012, 12, 274–280 RSC.
- T. Songjaroen, W. Dungchai, O. Chailapakul, C. S. Henry and W. Laiwattanapaisal, Lab Chip, 2012, 12, 3392–3398 RSC.
- G. W. Schmid-Schönbein, Y. Y. Shih and S. Chien, Blood, 1980, 56, 866–875 Search PubMed.
- J. Yang, Y. Huang, X. Wang, X. B. Wang, F. F. Becker and P. R. Gascoyne, Biophys. J., 1999, 76, 3307–3314 CrossRef CAS PubMed.
- M. E. Fay, D. R. Myers, A. Kumar, C. T. Turbyfield, R. Byler, K. Crawford, R. G. Mannino, A. Laohpant, E. A. Tyburski, Y. Sakurai, M. J. Rosenbluth, N. A. Switz, T. A. Sulchek, M. D. Graham and W. A. Lam, Proc. Natl. Acad. Sci. U. S. A., 2016, 113, 1987–1992 CrossRef CAS PubMed.
- L. Li, X. Huang, W. Liu and W. Shen, ACS Appl. Mater. Interfaces, 2014, 6, 21624–21631 CAS.
- M. S. Khan, G. Thouas, W. Shen, G. Whyte and G. Garnier, Anal. Chem., 2010, 82, 4158–4164 CrossRef CAS PubMed.
- M. Li, J. Tian, M. Al-Tamimi and W. Shen, Angew. Chem., Int. Ed., 2012, 51, 5497–5501 CrossRef CAS PubMed.
- X. Yang, J. Kanter, N. Z. Piety, M. S. Benton, S. M. Vignes and S. S. Shevkoplyas, Lab Chip, 2013, 13, 1464–1467 RSC.
- D. M. Cate, W. Dungchai, J. C. Cunningham, J. Volckens and C. S. Henry, Lab Chip, 2013, 13, 2397–2404 RSC.
- X. Yang, N. Z. Piety, S. M. Vignes, M. S. Benton, J. Kanter and S. S. Shevkoplyas, Clin. Chem., 2013, 59, 1506–1513 CAS.
- E. Fu, Analyst, 2014, 139, 4750–4757 RSC.
-
B. O'Farrell, in Lateral Flow Immunoassays, ed. R. C. Wong and H. Y. Tse, Humana Press, New York, 1st edn, 2009, ch. 1, pp. 1–34 Search PubMed.
- A. Nilghaz and W. Shen, RSC Adv., 2015, 5, 53172–53179 RSC.
Footnotes |
† Electronic supplementary information (ESI) available: Detailed materials and methods. Schematics of device architectures. Results of preliminary experiments. See DOI: 10.1039/c6lc00895j |
‡ These authors contributed to this manuscript equally. |
|
This journal is © The Royal Society of Chemistry 2016 |
Click here to see how this site uses Cookies. View our privacy policy here.