DOI:
10.1039/C9NA00305C
(Paper)
Nanoscale Adv., 2019,
1, 2748-2760
Enhanced photocatalysis and bacterial inhibition in Nb2O5via versatile doping with metals (Sr, Y, Zr, and Ag): a critical assessment†
Received
16th May 2019
, Accepted 30th May 2019
First published on 30th May 2019
Abstract
Unique optical properties render semiconductor Nb2O5 nanoparticles suitable for light harvesting and photocatalytic applications. This study focuses on determining optical properties such as the band gap, conduction band edge, valence band edge and work function of as-prepared solution combustion synthesized Nb2O5 nanoparticles with the help of UV-vis Diffuse Reflectance spectroscopy (DRS) and ultraviolet photoelectron spectroscopy (UPS) techniques. Phase purity and the oxidation states of the elements present in the material were confirmed from X-ray diffraction (XRD) patterns and X-ray photoelectron spectra (XPS), respectively. Doping semiconductors with different metal ions impacts the activity of the material, and therefore efforts were made to understand the effect on the photocatalytic performance of Nb2O5 due to the incorporation of metal dopants viz. Sr, Y, Zr, and Ag. Lattice parameters were obtained from Rietveld refinement of the XRD patterns. Parameters which are closely related to the photoactivity of the catalysts such as the presence of surface defects, oxygen vacancies, surface area, and charge carrier dynamics were determined from photoluminescence (PL) analysis, Brunauer–Emmett–Teller (BET) surface area measurements and time-resolved fluorescence (TRF) analysis respectively. In addition, the dopant concentrations were optimised for enhanced photocatalytic activity. The doped Nb2O5 nanoparticles showed significant activity towards targeted degradation of organic pollutants like 2-chlorophenol (2-CP) and dye contaminants like methylene blue (MB), orange G (OG) and indigo carmine (IC). This strategy yielded a robust response towards inactivation of E. coli and S. aureus as well. Adsorption and photodegradation of MB followed Lagergren's pseudo 1st order reaction model and the Langmuir Hinshelwood model respectively. Bacterial inactivation and OG, IC and 2-CP photodegradation followed 1st order kinetics. The reusability of the catalyst for 5 cycles was demonstrated. Finally, a plausible mechanism is proposed based on radical trapping experiments and combined analysis of the characterization techniques.
1 Introduction
Water pollution from industrial discharges is a major global concern and tackling such problems requires effective environment-friendly water treatment technology.1 Dyes are a kind of pollutants produced from textile, paint, paper, food, and leather industries. About 280
000 tons of dyes are discharged every year into the aquatic system.2 Chlorophenols representing another class of water pollutants, which are considered toxic to humans by the US EPA, are mainly produced from petroleum and pesticide manufacturing industries.3 Apart from these pollutants, water contaminated from coliform bacteria also poses serious health threats.4,5 To address these issues, conventional water treatment methods such as adsorption,6 coagulation,7 filtration,8 and ion exchange9 are found to be effective but they lead to the generation of secondary pollutants. Traditional disinfection methods such as using UV light are costly10,11 and chlorination generates harmful by-products.12,13 Advanced oxidation processes (AOPs) have been used as an alternative for wastewater treatment since the 1990s. Among AOPs, heterogeneous photocatalysis has shown great potential in disinfecting water and degrading chemical contaminants.14
Photocatalysis involves the usage of semiconductors that function as a catalyst on exposure to irradiation. The photogenerated charge carriers from the semiconductor produce reactive oxygen species to carry out the photodegradation of pollutants.15 TiO2 and ZnO are regarded as efficient photocatalysts for water decontamination16,17 and have a band gap of around 3–3.2 eV.18–20 A similar band gap is observed in Nb2O5 which is an n-type semiconductor.21 It is broadly utilized in the fields of gas sensing,22 glass manufacturing,23 and electrochemical energy storage,24 but limited reports are available on Nb2O5 as a photocatalyst and its related photochemical properties.21,25,26 As Nb2O5 exhibits similar semiconducting properties to those of TiO2, this material has great potential to replace the widely studied titania.
The efficiency of photocatalyzed reactions can reduce due to charge carrier recombination. This can be avoided by doping semiconductors with metal or non-metal impurities.27 Dopants should serve as the trapping sites for electrons or holes to increase the activity of photocatalysts.28 Doping leads to the creation of local energy states in the forbidden gap and could also narrow the band gap.29 Additionally, dopant incorporation could change the particle size, specific surface area, and morphology of nanoparticles which impacts the photoactivity.30 Sood et al. found that Sr2+ doping enhanced the photocatalytic activity of TiO2 towards brilliant green degradation due to an increase in the surface area and delay in the recombination time of excitons.31 Similar changes in the properties of TiO2 due to the incorporation of the Y3+ dopant were observed and the doped material showed excellent activity towards MO photodegradation.32 Silver doping in TiO2 was found to red shift the absorption range and enhance the separation efficiency of electrons and holes, thus showing good photocatalytic activity in the visible region towards Cr(VI) reduction.33 Incorporation of Zr4+ into TiO2 resulted in lattice defects and showed higher photoactivity towards the degradation of rhodamine B.34 Extensive studies were performed on doped TiO2 systems but the literature contains few efforts on the photoelectrochemical properties of doped Nb2O5 systems and their related applications. Nb2O5 doped with Eu3+ exhibited strong emission at 610 nm with long decay times making it suitable for lighting devices.35 Esteves et al. studied the effect of Mo and W doped Nb2O5 for the photooxidation of organic pollutants.36
In view of the captivating features of doped systems, we have initially studied the changes in the physical and chemical properties of solution combustion synthesized Nb2O5 due to incorporation of metal dopants viz. Sr, Y, Zr, and Ag using characterization techniques such as XRD, XPS, SEM, TEM, and BET surface area measurements. We have especially emphasized on analysing the optical properties of pristine and metal-doped Nb2O5 such as narrowing of the band gap, band edge details, the presence of defects and charge carrier dynamics using DRS, UPS, PL, and TRF spectroscopy techniques. From the above analyses, the optimized concentration of these dopants in Nb2O5 was concluded and it was later validated experimentally from MB photodegradation. Next, we have checked the photoactivity of these materials over a wide range of water pollutants. To the best of our knowledge, this is the first time the effect of Sr, Y, Zr, and Ag doped Nb2O5 has been observed for the photocatalytic degradation of various dyes and organics as well as for the inactivation of bacteria. Further, kinetic studies for the degradation reactions and the scavenger test for determining the dominant reactive oxygen species (ROS) were also demonstrated. The reusability test of the catalyst was performed, and structural stability was confirmed from XRD analysis.
2 Experimental
2.1 Materials and methods
The metal oxide was prepared by the solution combustion technique which is considered a quick and efficient technique to yield nanoparticles with high phase purity.37 A detailed procedure of preparing doped and pristine metal oxide by the solution combustion technique can be found elsewhere.29 Ammonium niobate oxalate hydrate (Sigma-Aldrich, USA) was used as the precursor for niobium and urea as the fuel. All other chemicals used were of analytical grade. To prepare Nb2O5, initially, the niobium precursor and urea were dissolved in DI water separately via sonication for 10 min and later mixed together. The mixed clear solution was kept in a preheated muffle furnace at 450 °C for 30 min. Black Nb2O5 was obtained.38 This catalyst was then calcined at 550 °C for 4 h to obtain white powdered pseudo hexagonal Nb2O5.38 Metal nitrates were used as the precursors for dopants Sr, Y, Zr and Ag. The atomic doping percentages for metal doped niobia were varied in the range of 0.05–2.5% and the resulting compounds were termed Nb(2−x) MxO5−δ where x is the dopant% and M is the dopant. For preparing the doped metal oxide the same procedure was used, in addition, the respective dopant's metal nitrate precursor was mixed with the sonicated niobium precursor urea solution.
2.2 Photochemical experiments
To check the photocatalytic activity of pristine and doped Nb2O5, MB, OG, IC were taken as model dye pollutants and 2-CP as model organic pollutant were taken as model pollutants. The photoreactor set up consisted of a metal halide lamp with an intensity of 121 W m−2. The actinometry test was performed to check the lamp intensity and the methodology can be found elsewhere.39 To avoid heating and maintain the reaction at 25 °C, the lamp was enclosed in a quartz jacket with inlet and outlet pipes connected to a chiller operating at 10 °C. The photodegradation experiments were performed in a quartz beaker. To attain an adsorption–desorption equilibrium, a 2 h dark reaction was performed before photocatalysis. Samples were collected at regular intervals, centrifuged and filtered for subsequent analysis. The degradation of the dyes was analyzed using UV-visible spectroscopy and the degradation of 2-CP was analyzed using HPLC (Waters Inc., USA). The system was equipped with a C-18 column with a UV detector and the mobile phase consisted of acetonitrile
:
water (20
:
80 v/v) with 0.01 M H3PO4 with a flow rate of 0.8 mL min−1.
2.3 Photocatalytic inactivation of bacteria
E. coli strain K-12 MG1655 and S. aureus strain ATCC 259323 were taken as model organisms to check the antibacterial response of the photocatalysts. Glassware, micro-tips and other plastics used in the experiment were autoclaved at 120 °C for 2 h. The bacterial cells were incubated in Luria broth under constant agitation at 37 °C for 18 h. Later, the centrifuged washed clean bacterial pellet was suspended in 30 mL of sterile water with a final concentration of ∼108 CFU mL−1. The experiments were conducted under dark for 1 h followed by 1 h of light irradiation under a metal halide lamp with an intensity of 15 W m−2. For analysis, 500 μL of the sample was collected at regular intervals of 20 min and the cell counting was done by the plate count method. The samples were serially diluted with sterile water and 50 μL of diluted samples were plated on solid nutrient agar plates. These plates were incubated at 37 °C for 18 h. Subsequently, the number of viable cells was counted.
3 Characterization
XRD patterns were obtained using a Rigaku diffractometer using Cu-Kα as a source of radiation. XPS of the materials were recorded using a Kratos Axis Ultra DLD instrument with monochromatic Al-Kα X-rays as the energy source (1486 eV). UPS data were obtained using the same instrument with a helium-I source. UV-vis DRS data were obtained using a Shimadzu spectrophotometer (UV 2450). PL spectra of the catalysts were recorded using a PerkinElmer spectrophotometer. SEM, Carl Zeiss-Ultra 55 FESEM and TEM, Philips CM 200 were used to understand the surface morphology of the catalyst. BET surface area assessment was performed using a Smart Sorb 92/93 surface area analyzer. TRF data were obtained with a FLS 920 Spectrofluorometer (Edinburgh Instruments) using a laser diode for excitation.
4 Results and discussion
4.1 XRD analysis
To understand the phase purity of the prepared materials, XRD analysis was performed. In Fig. 1, the XRD diffraction patterns of pristine and doped Nb2O5 nanoparticles with 0.25 atom% metal ions (Sr, Y, Zr, and Ag) were compared. All the diffraction patterns correspond to the pure crystalline hexagonal Nb2O5 phase (JCPDS no. 28-0317) and no possible impurity phase was found in the samples. The predominant peak of Nb2O5 at 2θ = 28.52° corresponding to the (1 0 0) plane can be seen to shift to lower diffraction angles 28.27°, 28.35°, 28.43° and 28.34° when doped with Sr2+, Y3+, Zr4+, and Ag+ respectively. This can be attributed to the lattice strain generated due to larger ionic radii of the dopants as compared to Nb5+.40 The ionic radii of Nb5+, Sr2+, Y3+, Zr4+, and Ag+ are 69 pm, 112 pm, 90 pm, 72 pm, and 126 pm respectively.41 The increase in the lattice parameters obtained from Rietveld refinement of the XRD patterns can be found in Table 1. These dopants substitute Nb5+ ions in their lattice sites causing a change in d-spacing and subsequently shifting XRD peaks to lower angles.42
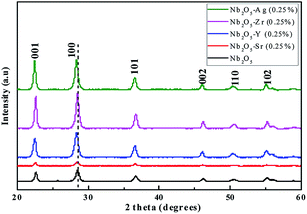 |
| Fig. 1 XRD patterns of Nb2O5 doped with Sr, Y, Zr, and Ag of 0.25 atom%. | |
Table 1 Lattice parameters and particle size for pristine and doped Nb2O5
Catalyst |
a (Å) |
b (Å) |
c (Å) |
Band gap (eV) |
Nb2O5 |
3.6115 |
3.6115 |
3.9269 |
3.00 |
Nb2O5–Sr (0.25%) |
3.6121 |
3.6121 |
3.9282 |
2.95 |
Nb2O5–Y (0.25%) |
3.6132 |
3.6131 |
3.9295 |
2.96 |
Nb2O5–Zr (0.25%) |
3.6216 |
3.6216 |
3.9396 |
2.94 |
Nb2O5–Ag (0.25%) |
3.6133 |
3.6133 |
3.9306 |
2.96 |
4.2 XPS analysis
XPS was performed to obtain the oxidation state of the elements present in the material. In Fig. 2(a) the peaks at binding energies 207.02 eV and 209.8 eV correspond to Nb-3d5/2 and Nb-3d3/2 with a spin–orbit splitting of 2.78 eV.43 This confirms the element to be present in the +5 oxidation state. In Fig. 2(b) the peak at binding energy 529.9 eV can be assigned to lattice oxygen. The O-1s peak at 531.5 eV can be related to surface hydroxyl groups.44 The XPS signals of the dopants were not detected due to their low atomic concentration.
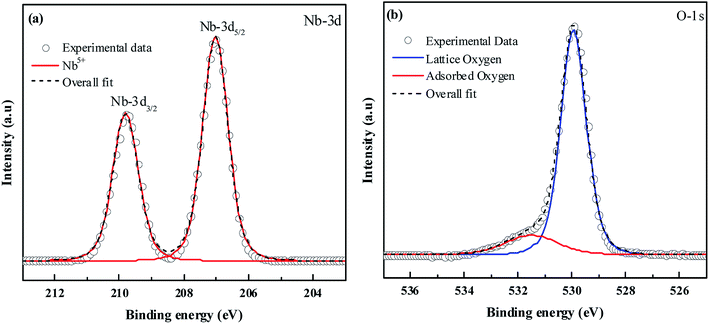 |
| Fig. 2 XPS spectra of (a) Nb-3d and (b) O-1s. | |
4.3 UV-vis DRS
UV-vis DRS analysis can be utilized to compare the shift in the absorption spectra for pristine and doped Nb2O5. Fig. S1(a)† shows the absorption spectra of Sr-doped Nb2O5. It can be seen that with the increase in dopant concentration, the absorption edge of Nb2O5 was slightly red shifted, but as the dopant concentration was increased to 2.5%, the absorption edge tends to shift to a lower wavelength signifying an increase in the band gap. This could be due to the formation of a minute amount of strontium oxide. A similar trend was observed for Y and Zr doped Nb2O5 as shown in Fig. S1(b) and (c).† As known from the literature, strontium oxide, yttrium oxide and zirconium oxide are semiconductors with band gaps of 5.5 eV,45 5.17 eV (ref. 46) and 5 eV (ref. 47) respectively which are relatively higher than that of niobium oxide. Therefore, if the concentration of these dopants is increased beyond a certain limit, a minute formation of these oxides might occur on the surface of Nb2O5 causing a shift in the absorption edge of Nb2O5 to the lower wavelength region. However, silver oxide possesses a band gap of around 2.25 eV which is smaller than that of Nb2O5;48 therefore from Fig. S1(d),† with the increase in Ag+ concentration in Nb2O5, the absorption edge was found to continue to shift to a higher wavelength even at a higher dopant concentration. From this analysis, it can be assumed that 0.25 atom% could be the optimized dopant concentration for Sr2+, Y3+, and Zr4+ in Nb2O5, but for Ag+ the optimized dopant concentration can be concluded from further analyses. However, experimentally from MB degradation reactions in the photocatalysis section, Ag+ at 0.1 atom% in Nb2O5 was found as the optimized dopant concentration.
To compare the band gaps of the different dopants in Nb2O5 with the optimized concentration, their absorbance spectra are shown in Fig. 3. The curve for doped Nb2O5 was red-shifted relative to pristine Nb2O5 from 417 nm to 420 nm, 422 nm, 423 nm and 427 nm due to the incorporation of Ag (0.1%), Y (0.25%), Sr (0.25%) and Zr (0.25%) dopants, respectively. This red shift suggests a slight reduction in the band gap of Nb2O5. The optical band gap was obtained using the Tauc plot49 (F(R) × hν)1/nvs. hν as shown in the inset of Fig. 3. Here hν is the photon energy in eV and F(R) is the Kubelka–Munk function shown in eqn (1) which determines the extent of photon absorption of a semiconductor from its reflectance. From DFT studies, Nb2O5 was found to possess an indirect band gap;50 therefore, n is taken to be 2 for indirect transitions.49 From the Tauc plot, the band gap (Eg) of the combustion synthesized Nb2O5 was found to be 3 eV and for doped Nb2O5, a slight shift in the band gap was observed as shown in Table 1. The reduction in the band gap could be attributed to the insertion of Sr, Y, Zr or Ag cations into the Nb2O5 lattice. Since the conduction band minimum is made up of Nb 4d bands,51 the substitution of Nb5+ with these cations might induce their energy states to extend into the Nb2O5 conduction band, resulting in the narrowing of the band gap. The maximum reduction in the band gap was observed for Zr (0.25%) followed by Sr (0.25%), Y (0.25%) and Ag (0.1%). Decreasing the band gap helps the materials to absorb light in the visible region but this could also lead to faster recombination of charge carriers. Therefore, other parameters which are crucial for the photoactivity of a semiconductor such as BET surface area and lifetime of charge carriers are discussed in a later section.
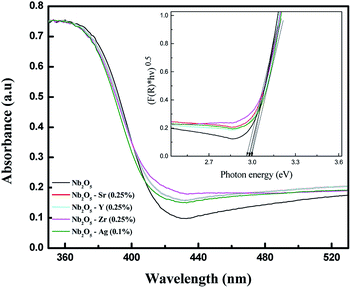 |
| Fig. 3 UV-vis absorbance spectra of pristine and doped Nb2O5 and the inset presents the transformed Kubelka–Munk function vs. photon energy. | |
4.4 UPS analysis
The UPS technique can be employed to understand the valence band properties of a semiconductor. Fig. 4 presents the UPS spectrum of Nb2O5 where the peak at binding energy 14.8 eV was due to hybridization between O-2p and Nb-4d bonds. The peak at 12 eV is formed by the O-2p orbital. The peak at around 8 eV is from the O-2p in the valence band region. These analyses could be found elsewhere which were obtained by comparing the UPS spectrum with DOS results.52 UPS analysis can also be utilized to obtain the exact band edges of a semiconductor.53 The work function (Wf) of a semiconductor surface is given by eqn (2) where hν is the energy of photons from the Helium I source and SECO is the secondary electron cut off energy which is obtained from a linear extrapolation of the higher emission onset edge to zero intensity of higher binding energy.54 Here hν is 21.22 eV and SECO is 18.44 eV, and thus Wf is 2.78 eV. The lower emission onset energy (E2) is obtained by linear extrapolation of the intensity curve tangent to the baseline49 and it is obtained at 4.2 eV. From DRS, the band gap of Nb2O5 is found to be 3 eV, and thus from eqn (3) and (4) the energy level of the valence band maximum (Evb) is obtained at −6.98 V and the conduction band minimum (Ecb) at −3.98 V vs. Absolute vacuum scale (AVS).
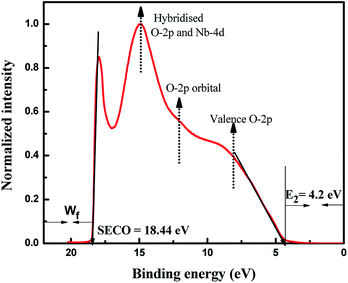 |
| Fig. 4 UPS spectra of Nb2O5. | |
4.5 BET surface area
From BET surface area measurements shown in Table 2, it could be concluded that the surface area of Nb2O5 was enhanced when doped with Sr2+, Y3+, and Zr4+ metal ions. No significant variation in the surface area was observed from the increase in the dopant concentration. However, for Ag+ doped Nb2O5, with the increase in the dopant concentration from 0.1% to 0.25%, the surface area increased by 1.4 times, but then decreased by 1.7 times when the concentration was increased to 2.5%. This could be due to agglomeration resulting in a decrease in the surface area.55 On comparing the surface area of the dopants with the optimised dopant concentration in Nb2O5, the following trend can be concluded Sr (0.25%) > Zr (0.25%) > Y (0.25%) > Ag (0.1%). Along with the increase in the surface area, analysis of the lifetime of charge carriers is important for determining the photoactivity of the material as these parameters need not be directly proportional to each other. To determine the presence of defects and recombination time of excitons, PL and TRF analyses are discussed in later sections.
Table 2 BET surface area and TRF charge carrier dynamics of doped and pristine Nb2O5, and the rate constant of MB photodegradation
Catalyst |
BET surface area (±1 m2 g−1) |
Lifetime of charge carriers τavg (ps) |
MB adsorption rate constant ka (±0.1 h−1) |
MB photodegradation rate constant km (±0.05 h−1) |
Photolysis |
— |
— |
— |
0.01 |
Nb2O5 |
28 |
1088 |
1.6 |
0.19 |
Nb2O5–Sr (0.1%) |
32 |
1223 |
— |
0.42 |
Nb2O5–Sr (0.25%) |
43 |
1290 |
2.3 |
0.60 |
Nb2O5–Sr (2.5%) |
44 |
1212 |
— |
0.41 |
Nb2O5–Y (0.1%) |
38 |
1047 |
— |
0.30 |
Nb2O5–Y (0.25%) |
36 |
1241 |
2.2 |
0.49 |
Nb2O5–Y (2.5%) |
33 |
1127 |
— |
0.46 |
Nb2O5–Zr (0.1%) |
36 |
1143 |
— |
0.43 |
Nb2O5–Zr (0.25%) |
38 |
1162 |
2.4 |
0.54 |
Nb2O5–Zr (2.5%) |
41 |
904 |
— |
0.42 |
Nb2O5–Ag (0.1%) |
32 |
1202 |
2.3 |
0.58 |
Nb2O5–Ag (0.25%) |
45 |
1029 |
— |
0.47 |
Nb2O5–Ag (2.5%) |
26 |
1084 |
— |
0.40 |
4.6 PL analysis
From photoluminescence analysis, information on the photochemical properties of a semiconductor such as oxygen vacancies, defects, and charge carrier transfer can be obtained.56 Nb2O5 with an excitation wavelength of 325 nm was found to exhibit photoluminescence in the range from 350 to 550 nm as shown in Fig. 5. The short wavelength emissions at 409 nm can be attributed to the near band edge emissions where electron transitions take place from the bottom of the conduction band to the top of the valence band.56 The emission spectra at 448, 466, 480, 490 and 560 nm can be related to surface defects and oxygen vacancies56,57 where transitions occur in the energy levels within the band gap.58 No new PL signal was observed from the doped metals but variation in intensity was found. The intensities of Zr (0.25%) and Y (0.25%) doped Nb2O5 were found to be higher than that of Nb2O5. This could be due to the higher content of surface defects and oxygen vacancies that are the source of non-radiative recombination58 and possibly not due to the higher recombination rate as evident from the recombination time of charge carriers from TRF analysis. The intensities of Sr (0.25%) and Ag (0.1%) doped Nb2O5 were found to be lower signifying a lower recombination rate of excitons than that of Nb2O5.
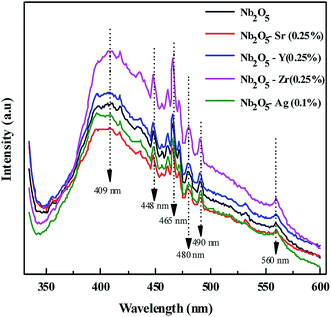 |
| Fig. 5 PL spectra of the doped and pristine Nb2O5. | |
4.7 Charge carrier dynamics by TRF analysis
The lifetime of charge carriers can be obtained from TRF analysis. The lifetime emission decays of pristine and metal-doped Nb2O5 spectra were measured at a maximum emission wavelength of 415 nm (as obtained from PL analysis) using a 305 nm laser diode. Fig. 6 shows the TRF decay curve for Nb2O5. The decay curve was fitted using a two parameter biexponential decay model (eqn (5)) with R2 higher than 0.97. Here A1 and A2 are the decay constants; the shorter lifetime τ1 corresponds to the non-radiative process which is due to heat losses and the longer lifetime τ2 corresponds to the radiative process which provides information related to the electron–hole recombination.59 The average lifetime was calculated using eqn (6).60 The lifetime of charge carriers (τavg) is shown in Table 2. For Nb2O5, τavg was found to be 1088 picoseconds (ps) and with the incorporation of dopants into the host Nb2O5, the lifetime of charge carriers was found to have increased. Indeed Sr (0.25%), Y (0.25%), Zr (0.25%) and Ag (0.1%) were found to have a longer lifetime compared to 0.1% and 2.5% dopant concentration. With the excess dopant concentration of 2.5%, τavg decreased as the increased dopant concentration must have acted as the recombination centers.61 On comparing the lifetimes of optimised dopants the following trend can be observed Sr (0.25%) > Y (0.25%) > Zr (0.25%) > Ag (0.1%). The significance of the longer lifetime of charge carriers vs. the photoactivity of the catalysts towards different pollutants is discussed in the photocatalysis section. | y = A1 exp(−t/τ1) + A2 exp(−t/τ2) | (5) |
| τavg = (A1 × τ12 + A2 × τ22) / (A1 × τ1+ A2 × τ2) | (6) |
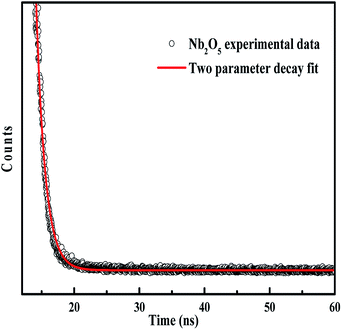 |
| Fig. 6 TRF decay curve of Nb2O5. | |
4.8 SEM and TEM analysis
Fig. 7(a) and (b) show the SEM images of Nb2O5 and Sr (0.25%) doped Nb2O5 nanoparticles which are found to have an earth rock like structure. No specific morphology change was observed due to the incorporation of dopants. The SEM images for the other doped materials are shown in Fig. S2(a)–(c).† The d spacings of 3.0 Å and 3.9 Å obtained from the HR TEM images in Fig. 7(c) and (d) correspond to planes (1 0 0) and (0 0 1) respectively, and the inset shows the FFT images. These planes with the respective d spacing can be validated from the XRD patterns.
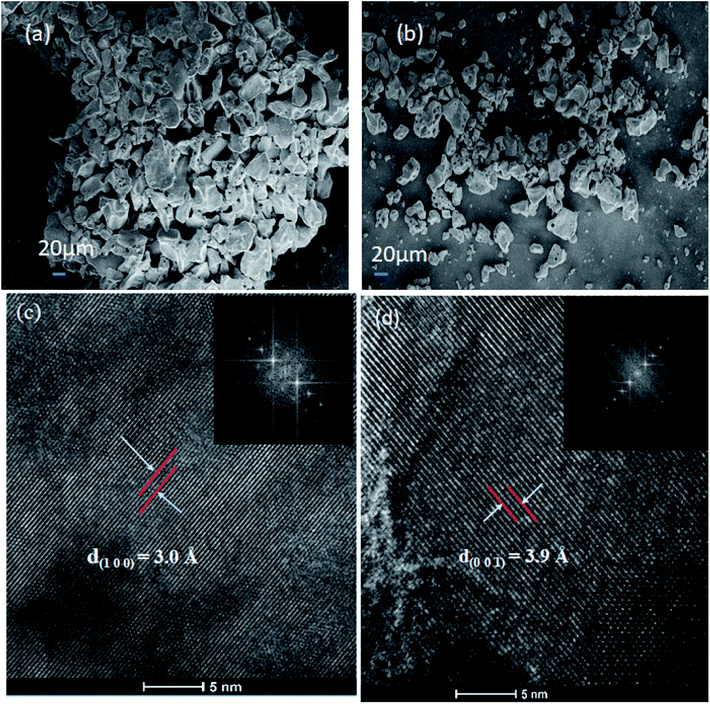 |
| Fig. 7 (a) SEM image of Nb2O5, (b) SEM image of Sr (0.25%)–Nb2O5, (c) HRTEM image of Nb2O5 showing the d spacing of the (1 0 0) plane and the inset shows the FFT image, and (d) HRTEM image of Nb2O5 showing the d spacing of the (0 0 1) plane and the inset presents the FFT image. | |
4.9 Photocatalysis
4.9.1 MB photo degradation.
4.9.1.1 Effect of catalyst loading.
The degradation of MB was evaluated by monitoring the decrease in the absorbance peak at 665 nm using UV-visible spectroscopy. Fig. S3 in the ESI† presents the effect of catalyst loading for the degradation of MB with an initial concentration of 15 ppm. The experiment was carried out at a neutral pH of 7. The zero-point charge (pHzpc) of Nb2O5 (as obtained from the pH drift method62) is 4, as shown in Fig. S4.† The surface of Nb2O5 is positively charged at pH below pHzpc and negatively charged at pH above pHzpc.53 Therefore, MB which is a cationic dye gets adsorbed readily on the surface of Nb2O5 at pH 7. For control, photolysis was performed which showed no degradation of the dye. The adsorption–desorption equilibrium for the catalyst was achieved in 2 h of reaction under dark conditions. A catalyst loading of 0.2 g L−1 degraded the dye by 20% at the end of 2 h. This lower catalyst loading was not sufficient to generate enough ROS to degrade the dye. A loading of 0.5 g L−1 degraded the dye by 46%. On further increasing the catalyst loading to 1 g L−1, the solution became turbid. This hindered the penetration of light which resulted in a decrease in activity, thereby degrading the dye by 44% at the end of 2 h.63 Therefore, 0.5 g L−1 was taken as the optimum catalyst loading to perform further experiments.
4.9.1.2 Optimization of Sr, Y, Zr and Ag concentration doped in Nb2O5 towards MB degradation.
To inhibit charge carrier recombination, optimization of dopant concentration is necessary for achieving higher photocatalytic activity.30 Fig. S5(a)† shows the effect of Sr doping concentration in Nb2O5 for MB photodegradation. Sr content at 0.25% was found to be the optimized concentration and the same was observed for Y and Zr doped Nb2O5 from Fig. S5(b) and (c).† This could be attributed to the greater number of active sites at this dopant concentration as evident from 2 h of dark adsorption. As the dopant concentration was increased to 2.5%, the photocatalytic activity for all the above dopants decreased which is also complemented by TRF analysis. The reduction in the distance between the trap sites resulted in faster electron–hole recombination as the excess dopant concentration acted as the recombination centers.61 For Ag-doped Nb2O5 the optimized dopant loading was 0.1%, as shown in Fig. S5(d).† When the loading increased beyond the optimal level, reduction in the activity could be due to faster recombination. Therefore, when Ag loading is increased, clusters of Ag species form overlapping agglomerates which shadow the photocatalytic activity due to surface plasmon absorption.64
4.9.1.3 Rate kinetics for MB adsorption and photodegradation using optimized dopant concentration.
The adsorption of MB on metal doped Nb2O5 shown in Fig. S6† was found to follow Lagergren's pseudo-first-order reaction model (eqn (7)), where ka is the adsorption rate constant (h−1) and qe and qt are the amounts of adsorbed dye (mg g−1) at equilibrium and at time t.65 Following adsorption, the photodegradation of MB shown in Fig. 8 followed the Langmuir–Hinshelwood model (eqn (8)).66 Here km is the photodegradation rate constant and | ln(qe − qt) = ln(qe) − kat | (7) |
| ln(C0/C) = kmt + ln(C0/C1) | (8) |
C0 is the initial dye concentration. C1 and C are the concentrations at reaction time zero and at time t. The rate constants for MB adsorption and photodegradation kinetics are shown in Table 2. The rate constants for photodegradation of the dye were found to follow the order Sr > Ag > Zr > Y. However, from TRF analysis, the lifetime of the charge carriers followed the order Sr > Y > Ag > Zr. The non-complementary relation between the photoactivity and the lifetime of charge carriers could be the presence of deep trap states in the catalyst. The electrons trapped in these states show a longer lifetime but are unable to reduce electron acceptors, thus lowering the efficiency of photocatalytic reactions.67 The number of active sites for adsorption, increase in surface area and the lifetime of charge carriers all play roles in degrading the pollutant. It would be difficult to identify the dominant property of the material for the activity towards pollutant degradation.
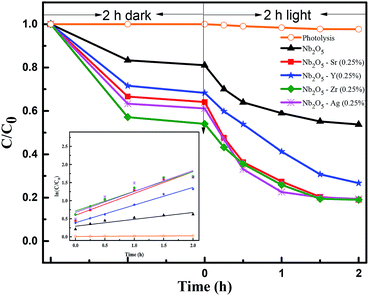 |
| Fig. 8 Effect of dopants in Nb2O5 for MB degradation and the inset represents rate kinetics. | |
4.9.2 OG photodegradation: effect of pH and dopants.
The initial concentration of OG was taken as 20 ppm. The photodegradation of the dye was monitored by checking the absorbance at 480 nm using UV-vis spectroscopy. OG is an anionic dye and at a neutral pH of 7.6, with an optimized catalyst loading of 1.2 g L−1 (Fig S7†), the dye was degraded only by 2% as seen from Fig. S8.† With the decrease in pH, the degradation increased. A maximum degradation of 30% was observed at pH 3.25. However, at a very low pH of 2.5, the catalyst surface was masked by a greater number of H+ adsorbing on it, preventing the photoexcitation of Nb2O5 and leading to a decrease in the photocatalytic activity. Similar phenomena were observed in some other studies.68
Fig. 9 presents the photodegradation profile of OG with Nb2O5 doped with Sr, Y, Zr, and Ag with the optimized concentration of the dopants at pH 3.25 and a loading of 1.21 g L−1. No significant discoloration of the dye was observed in 2 h of dark reaction. For control, photolysis was performed which showed no degradation of the dye. The doped material was found to perform better than pristine Nb2O5 following the order Sr > Zr ∼ Y > Ag > pristine Nb2O5. This order could be attributed to the same increasing trend in the surface area of the doped catalyst. The photodegradation of OG was found to follow 1st order kinetics (eqn (9)), where ko is the rate constant, Co is the initial concentration and C is the concentration at time t. The rate constants are shown in Table 3.
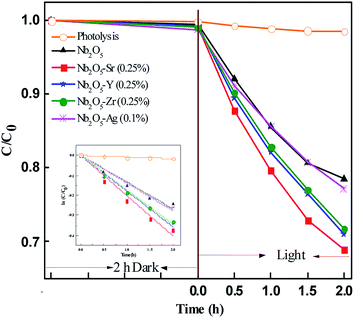 |
| Fig. 9 Effect of dopants in Nb2O5 for OG degradation and the inset presents rate kinetics. | |
Table 3 Rate constants for OG, IC, 2-CP, E. coli and S. aureus inactivation
Catalyst |
OG degradation rate constant ko (±0.01 h−1) |
IC degradation rate constant ki (±0.01 h−1) |
2-CP degradation rate constant kc (±0.01 h−1) |
E. coli inactivation rate constant ke (±0.01 min−1) |
S. aureus inactivation rate constant ks (±0.005 min−1) |
Photolysis |
0.008 |
0.14 |
0.04 |
0.027 |
0.008 |
Nb2O5 |
0.134 |
0.29 |
0.13 |
0.076 |
0.019 |
Nb2O5–Sr (0.25%) |
0.201 |
1.11 |
0.58 |
0.120 |
0.069 |
Nb2O5–Y (0.25%) |
0.178 |
0.37 |
0.49 |
0.098 |
0.028 |
Nb2O5–Zr (0.25%) |
0.172 |
0.52 |
0.50 |
0.115 |
0.053 |
Nb2O5–Ag (0.1%) |
0.138 |
0.35 |
0.44 |
0.118 |
0.051 |
4.9.3 IC photodegradation.
The initial concentration of IC was taken as 40 ppm. The photodegradation of the dye was monitored by checking the absorbance at 610 nm using UV-vis spectroscopy. Since IC is also an anionic dye, no degradation of the dye was observed at a neutral pH of 7.5. From Fig. S9,† around maximum degradation of the dye was observed at pH 3 with a catalyst loading of 0.5 g L−1. The doped materials were found to perform better than pristine Nb2O5, Fig. 10 following the order Sr > Zr > Y > Ag > pristine Nb2O5 following 1st order kinetics (eqn (10)) with a maximum degradation of 95% from Sr (0.25%) doped Nb2O5 where ki is the rate constant for IC photodegradation. The rate constants are shown in Table 3.
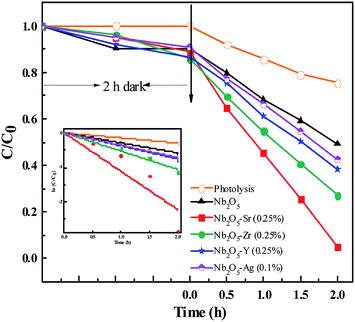 |
| Fig. 10 Effect of dopants in Nb2O5 for IC degradation and the inset presents rate kinetics. | |
4.9.4 2-CP photodegradation.
The photodegradation of 2-CP was analyzed using HPLC with an initial concentration of 25 ppm. The adsorption–desorption equilibrium was observed in 2 h of dark reaction. Fig. 11 presents the effect of pristine and doped Nb2O5 for 2-CP photodegradation and the inset presents the rate kinetics. Around 90% degradation of the pollutant was observed from the doped catalysts as compared to 15% degradation from photolysis and around 50% from pristine Nb2O5. The photodegradation followed 1st order kinetics (eqn (11)), where kp is the rate constant at a neutral pH of 7.3 and a catalyst loading of 0.5 g L−1. The rate constants are shown in Table 3 following the order Sr > Zr > Y > Ag > Nb2O5
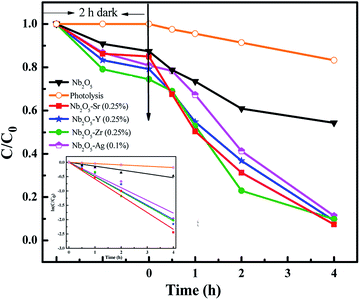 |
| Fig. 11 Effect of dopants in Nb2O5 for 2-CP degradation and the inset presents rate kinetics. | |
4.9.5 Antibacterial experiments.
To assess the antibacterial response of the catalysts, E. coli was taken as a model Gram-negative bacterium. Fig. S10† presents the effect of catalyst loading using Sr (0.25%) doped Nb2O5 as a catalyst for the photoinactivation of E. coli. The initial bacterial concentration was taken as 2 × 108 CFU mL−1. It was observed that a loading of 0.05 g L−1 was too low to inactivate bacteria and 0.25 g L−1 caused turbidity causing a decrease in the rate of inactivation. The optimized loading was found to be 0.1 g L−1 resulting in a 3.5 log reduction of E. coli cells. Fig. 12 shows the effect of different dopants in Nb2O5 for E. coli photoinactivation and the inset presents the rate kinetics. For control, photolysis was performed and less than 1-log reduction was observed. A 1.5-log reduction in bacterial cells was observed with Nb2O5. The doped material was found to perform better than Nb2O5 with rate constants following the order Sr ∼ Zr ∼ Ag > Y > Nb2O5. Around 3.5 log reduction was observed from Ag, Zr and Sr-doped Nb2O5 and a 3-log reduction was found from Y doped Nb2O5. However, when a model Gram-positive strain S. aureus was taken with an initial count of 108 CFU mL−1, the bactericidal efficacy was maximum of ∼2 log fold from Sr doped Nb2O5 as shown in Fig. 13. From photolysis, insignificant reduction in S. aureus cells was observed and around 0.5 log reduction from pristine Nb2O5. Since the major bacterial contamination pertaining in the domestic water system is by fecal coliforms and not predominantly due to Gram-positive species,4,69 our catalyst can remediate water effectively. The photoinactivation of E. coli and S. aureus was found to follow 1st order kinetics fitting the data using eqn (12) and (13) where ke and ks are the rate constants for E. coli and S. aureus, respectively, Co is the initial concentration and C is the concentration at time t. The rate constants are shown in Table 3.
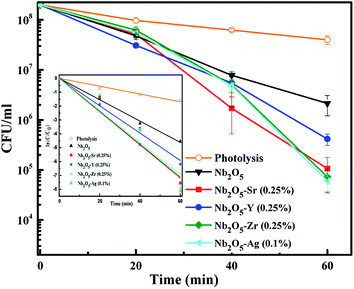 |
| Fig. 12 Effect of dopants in Nb2O5 for E. coli inactivation and the inset presents rate kinetics. | |
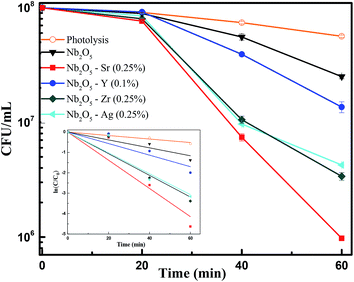 |
| Fig. 13 Effect of dopants in Nb2O5 for S. aureus inactivation and the inset represents rate kinetics. | |
4.10 Scavenger test
To identify the active species taking part in the photodegradation reactions, trapping experiments were carried out. MB and E. coli were taken as the test pollutants and Sr (0.25%) doped Nb2O5 as the catalyst. 1 mM t-butanol,70 1 mM EDTA-Na70 and 2 mM TEMPO71 were used as scavengers for OH, h+ and O2˙− radicals respectively in the photoreaction containing the dye/E. coli and the catalyst in the presence of light. Control experiments included reaction in the presence of scavengers, dye/E. coli and light without the catalyst and it was observed that there was insignificant dye degradation or bacterial inactivation from Fig. 14 and S11.† The main active species identified were superoxide radicals suppressing the degradation rate of the dye by ∼60%. Generation of superoxide radicals at −4.17 V vs. the AVS scale was evident from UPS analysis. The holes were also capable of directly oxidizing the pollutant as the scavenger EDTA could prevent the dye degradation by 40%. In the case of antibacterial experiments as well, superoxide radicals and holes were found to be the main active species. Scavengers TEMPO and EDTA were found to suppress the inactivation by almost 90%.
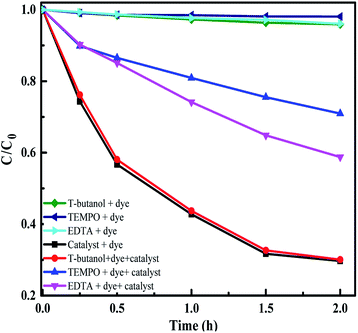 |
| Fig. 14 Radical trapping experiment with scavengers and MB as the pollutant. | |
4.11 Proposed mechanism
A possible mechanism is proposed using the combined analysis from PL, DRS, UPS, TRF and scavenger tests. A schematic of such a mechanism with energy levels of Nb2O5 is shown in Fig. 15. The as-prepared Nb2O5 nanoparticles were found to absorb in the near visible region with a band gap Eg of 3 eV and the energy level of the conduction band minimum Ecb at −3.98 V and that of the valence band maximum Evb at −6.98 V vs. AVS. The conduction band of Nb2O5 is made up of Nb-4d orbitals and the valence band consists of O-2p orbitals.51 When photons (hν) with energy equal to or greater than 3 eV are incident on Nb2O5, electronic transitions can take place from Evb to Ecb of the semiconductor or to the defect states. The presence of defects in the Nb2O5 semiconductor was detected from PL analysis, generated due to the presence of vacancies, impurities or interstitial atoms.72 These defects lying near Ecb or Evb can capture the electrons or the holes evading charge recombination. Also, incorporation of dopants induces their own energy states in the semiconductor which captures the photoinduced electrons. This enhances charge separation resulting in prolonged recombination time as validated from TRF analysis. The orbitals of the dopants Sr, Y, Zr, and Ag could be lying around or merging with the Nb-4d orbitals of Nb2O5 as a slight reduction in the band gap of Nb2O5 was observed on doping. These trapped electrons or the electrons in Ecb can react with oxygen to produce O2˙− radicals which are the main active species responsible for organic pollutant photodegradation as well as for bacterial inactivation. The other active species as evident from scavenger experiments are the positive holes which are created in the Evb or the trapped holes that could directly attack the pollutants.73
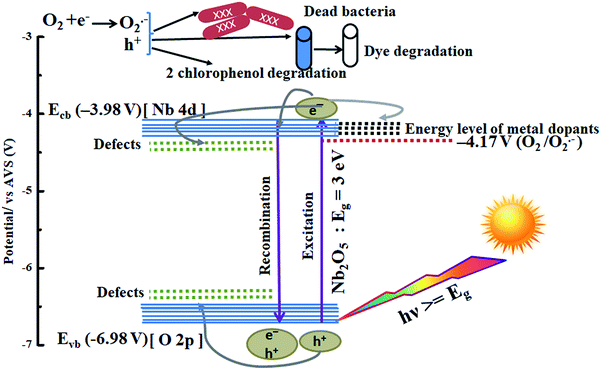 |
| Fig. 15 Photocatalysis mechanism in metal-doped Nb2O5. | |
4.12 Reusability and structural stability
Fig. S12† shows the reusability of the Sr 0.25% doped Nb2O5 catalyst for the degradation of MB. After five cycles the catalyst could degrade the dye by 70%, and the activity was found to decrease only by 12.5% compared to the fresh catalyst. Fig. S13† shows the XRD pattern of the catalyst before and after use for 5 cycles. No change in the pattern was observed confirming its stability.
5 Conclusions
This study presents the optical and photocatalytic properties of solution combustion synthesized Nb2O5 nanoparticles. The derived photocatalyst exhibited a band gap of 3 eV with a valence band edge at −6.98 V and conduction band edge at −3.98 V vs. AVS signifying the energy level to be sufficient enough to generate superoxide radicals at −0.417 V. The work function of the material was found to be 2.78 eV. Several properties of this host material were influenced on doping with metals (Sr, Y, Zr, and Ag) such as increment in the lattice parameters as analyzed from Rietveld refinement of the XRD patterns of pristine and doped Nb2O5, and enhancement in the surface area from BET analysis. A red shift in the absorption edge of Nb2O5 on doping was found from DRS analysis. The as-prepared niobium oxide was found to have surface defects and oxygen vacancies from PL analysis. Doping Nb2O5 with metals facilitated better charge separation and the optimized concentrations of dopants Sr2+, Y3+ and Zr4+ were found to be 0.25% and 0.1% for the Ag+ dopant from the TRF technique. The optimization of this dopant concentration was also validated experimentally by analyzing MB photodegradation. The doped materials showed excellent photoactivity towards dye and chlorophenol degradation as well as bacterial inactivation. The affinity of these materials towards the degradation of each pollutant was different, but Sr (0.25%) doped Nb2O5 was found to be the best amongst all the other catalysts. From the scavenger test, superoxide radicals and holes were found to be the dominant reactive oxygen species in the photodegradation reactions. The catalyst was found to be stable after 5 cycles and the structural stability was confirmed from XRD analysis. This study suggests that combustion synthesized Sr (0.25%) doped Nb2O5 is chemically stable and could be used as a potential photocatalyst for antimicrobial action and degradation of recalcitrant organic contaminants.
Conflicts of interest
There are no conflicts to declare.
Acknowledgements
The authors would like to thank the Department of Science and Technology (DST), India for financial support. The authors thank the IPC and CeNSE for characterization facilities, the Centre for Earth Science, IISc for ICP-MS analysis and the SSCU for DRS and PL facility. The authors are grateful to Prof. Anshu Pandey for TRF facility and Mr Paresh Samantaray for SEM imaging.
References
- P. K. Samantaray, S. Baloda, G. Madras and S. Bose, Adv. Sustainable Syst., 2019, 1800153 CrossRef
.
- C. A. Martínez-Huitle and E. Brillas, Appl. Catal., B, 2009, 87, 105–145 CrossRef
.
- N. Rao, A. Dubey, S. Mohanty, P. Khare, R. Jain and S. Kaul, J. Hazard. Mater., 2003, 101, 301–314 CrossRef CAS PubMed
.
- P. K. Samantaray, G. Madras and S. Bose, ChemistrySelect, 2017, 2, 7965–7974 CrossRef CAS
.
- P. K. Samantaray, G. Madras and S. Bose, ACS Sustainable Chem. Eng., 2018, 7, 1580–1590 CrossRef
.
- V. Gupta, J. Environ. Manage., 2009, 90, 2313–2342 CrossRef CAS PubMed
.
- M. R. Gadekar and M. M. Ahammed, Desalin. Water Treat., 2016, 57, 26392–26400 CrossRef CAS
.
- P. K. Samantaray, G. Madras and S. Bose, J. Membr. Sci., 2018, 548, 203–214 CrossRef CAS
.
- S. Raghu and C. A. Basha, J. Hazard. Mater., 2007, 149, 324–330 CrossRef CAS PubMed
.
- V. Lazarova, P. Savoye, M. Janex, E. Blatchley Iii and M. Pommepuy, Water Sci. Technol., 1999, 40, 203–213 CrossRef CAS
.
- S. Bose and P. Samantaray, Proc. Indian Natl. Sci. Acad., 2018, 84, 669–679 Search PubMed
.
-
J. Chapman, F. Regan and T. Sullivan, Nanoparticles in Anti-microbial Materials: Use and Characterisation, Royal Society of Chemistry, 2012 Search PubMed
.
- N. Padmavathy, P. K. Samantaray, L. D. Ghosh, G. Madras and S. Bose, Nanoscale, 2017, 9, 12664–12676 RSC
.
- J.-M. Herrmann, C. Guillard and P. Pichat, Catal. Today, 1993, 17, 7–20 CrossRef CAS
.
- A. Fujishima, X. Zhang and D. A. Tryk, Surf. Sci. Rep., 2008, 63, 515–582 CrossRef CAS
.
- P. A. Pekakis, N. P. Xekoukoulotakis and D. Mantzavinos, Water Res., 2006, 40, 1276–1286 CrossRef CAS PubMed
.
- K. M. Lee, C. W. Lai, K. S. Ngai and J. C. Juan, Water Res., 2016, 88, 428–448 CrossRef CAS PubMed
.
- A. Fujishima and X. Zhang, C. R. Chim., 2006, 9, 750–760 CrossRef CAS
.
- O. Ola and M. M. Maroto-Valer, J. Photochem. Photobiol., C, 2015, 24, 16–42 CrossRef CAS
.
- C. Gadiyar, B. Boruah, C. Mascarenhas and V. Shetty, Int. J. Res. Eng. Technol., 2013, 2277–4106 Search PubMed
.
- A. G. Prado, L. B. Bolzon, C. P. Pedroso, A. O. Moura and L. L. Costa, Appl. Catal., B, 2008, 82, 219–224 CrossRef CAS
.
- A. Mozalev, M. Bendova, R. Vazquez, Z. Pytlicek, E. Llobet and J. Hubalek, Sens. Actuators, B, 2016, 229, 587–598 CrossRef CAS
.
- M. Zhang, H. Wen, J. Yu, F. Ai, H. Yu, X. Pan, H. Shao, M. Tang and L. Gai, Opt. Mater. Express, 2017, 7, 3222–3230 CrossRef CAS
.
- Y. Zhao, C. Ding, Y. Hao, X. Zhai, C. Wang, Y. Li, J. Li and H. Jin, ACS Appl. Mater. Interfaces, 2018, 10, 27106–27115 CrossRef CAS PubMed
.
- S. Furukawa, T. Shishido, K. Teramura and T. Tanaka, ChemPhysChem, 2014, 15, 2665–2667 CrossRef CAS PubMed
.
- X. Chen, T. Yu, X. Fan, H. Zhang, Z. Li, J. Ye and Z. Zou, Appl. Surf. Sci., 2007, 253, 8500–8506 CrossRef CAS
.
- R. Asahi, T. Morikawa, T. Ohwaki, K. Aoki and Y. Taga, Science, 2001, 293, 269–271 CrossRef CAS PubMed
.
- K. Wilke and H. Breuer, J. Photochem. Photobiol., A, 1999, 121, 49–53 CrossRef CAS
.
- R. Gupta, N. K. Eswar, J. M. Modak and G. Madras, RSC Adv., 2016, 6, 85675–85687 RSC
.
- Y. Ma, J. Zhang, B. Tian, F. Chen and L. Wang, J. Hazard. Mater., 2010, 182, 386–393 CrossRef CAS PubMed
.
- S. Sood, A. Umar, S. K. Mehta, A. Sinha and S. K. Kansal, Ceram. Int., 2015, 41, 3533–3540 CrossRef CAS
.
- N. Xinshu, L. Sujuan, C. Huihui and Z. Jianguo, J. Rare Earths, 2011, 29, 225–229 CrossRef
.
- X. Lei, X. Xue and H. Yang, Appl. Surf. Sci., 2014, 321, 396–403 CrossRef CAS
.
- S.-m. Chang and R.-a. Doong, J. Phys. Chem. B, 2006, 110, 20808–20814 CrossRef CAS PubMed
.
- D. Falcomer, A. Speghini, G. Ibba, S. Enzo, C. Cannas, A. Musinu and M. Bettinelli, J. Nanomater., 2007, 2007, 94975 Search PubMed
.
- A. Esteves, L. C. Oliveira, T. C. Ramalho, M. Goncalves, A. S. Anastacio and H. W. Carvalho, Catal. Commun., 2008, 10, 330–332 CrossRef CAS
.
- T. Mimani and K. Patil, Mater. Phys. Mech., 2001, 4, 134–137 CAS
.
- S. Li, Q. Xu, E. Uchaker, X. Cao and G. Cao, CrystEngComm, 2016, 18, 2532–2540 RSC
.
- R. Gupta, B. Boruah, J. M. Modak and G. Madras, J. Photochem. Photobiol., A, 2019, 372, 108–121 CrossRef CAS
.
-
V. Morris, R. Farrell, A. Sexton and M. Morris, 2006.
-
S. Ruben, Handbook of the Elements, Open Court, 1985 Search PubMed
.
- D. P. Dutta, M. Ramakrishnan, M. Roy and A. Kumar, J. Photochem. Photobiol., A, 2017, 335, 102–111 CrossRef CAS
.
- J.-H. Jang, T.-Y. Kim, N.-J. Kim, C.-H. Lee, E.-M. Park, C. Park and S.-J. Suh, Mater. Sci. Eng., B, 2011, 176, 1505–1508 CrossRef CAS
.
- A. Naldoni, M. Allieta, S. Santangelo, M. Marelli, F. Fabbri, S. Cappelli, C. L. Bianchi, R. Psaro and V. Dal Santo, J. Am. Chem. Soc., 2012, 134, 7600–7603 CrossRef CAS PubMed
.
- S. Stankic, J. Bernardi, O. Diwald and E. Knözinger, J. Phys. Chem. C, 2007, 111, 8069–8074 CrossRef CAS
.
- G. Bhavani, S. Ganesan, S. Selvasekarapandian, S. Monisha and M. Premalatha, Ionics, 2016, 22, 581–592 CrossRef CAS
.
- C. Gionco, M. C. Paganini, E. Giamello, O. Sacco, V. Vaiano and D. Sannino, J. Energy Chem., 2017, 26, 270–276 CrossRef
.
- A. Varkey and A. Fort, Sol. Energy Mater. Sol. Cells, 1993, 29, 253–259 CrossRef CAS
.
- B. Boruah, R. Gupta, J. M. Modak and G. Madras, J. Photochem. Photobiol., A, 2019, 373, 105–115 CrossRef CAS
.
- S. Sathasivam, B. A. Williamson, S. A. Althabaiti, A. Y. Obaid, S. N. Basahel, M. Mokhtar, D. O. Scanlon, C. J. Carmalt and I. P. Parkin, ACS Appl. Mater. Interfaces, 2017, 9, 18031–18038 CrossRef CAS PubMed
.
- T. El-Shazly, W. M. Hassan, S. T. A. Rahim and N. K. Allam, Int. J. Hydrogen Energy, 2015, 40, 13867–13875 CrossRef CAS
.
- Z. Weibin, W. Weidong, W. Xueming, C. Xinlu, Y. Dawei, S. Changle, P. Liping, W. Yuying and B. Li, Surf. Interface Anal., 2013, 45, 1206–1210 CrossRef
.
- B. Boruah, R. Gupta, J. M. Modak and G. Madras, J. Photochem. Photobiol., A, 2019, 373, 105–115 CrossRef CAS
.
- S. Farsinezhad, H. Sharma and K. Shankar, Phys. Chem. Chem. Phys., 2015, 17, 29723–29733 RSC
.
- Y. Abdollahi, A. Abdullah, Z. Zainal and N. Yusof, Int. J. Basic Appl. Sci., 2011, 11, 62–69 Search PubMed
.
- N. Usha, R. Sivakumar, C. Sanjeeviraja and M. Arivanandhan, Optik, 2015, 126, 1945–1950 CrossRef CAS
.
- V. Agrahari, M. C. Mathpal, M. Kumar and A. Agarwal, J. Alloys Compd., 2015, 622, 48–53 CrossRef CAS
.
- J. Liqiang, Q. Yichun, W. Baiqi, L. Shudan, J. Baojiang, Y. Libin, F. Wei, F. Honggang and S. Jiazhong, Sol. Energy Mater. Sol. Cells, 2006, 90, 1773–1787 CrossRef
.
- S. P. Phivilay, A. A. Puretzky, K. Domen and I. E. Wachs, ACS Catal., 2013, 3, 2920–2929 CrossRef CAS
.
- A. K. Simlandy, B. Bhattacharyya, A. Pandey and S. Mukherjee, ACS Catal., 2018, 8, 5206–5211 CrossRef CAS
.
- N. Sobana, K. Selvam and M. Swaminathan, Sep. Purif. Technol., 2008, 62, 648–653 CrossRef CAS
.
- S. Nethaji, A. Sivasamy, G. Thennarasu and S. Saravanan, J. Hazard. Mater., 2010, 181, 271–280 CrossRef CAS PubMed
.
- R. J. Tayade, T. S. Natarajan and H. C. Bajaj, Ind. Eng. Chem. Res., 2009, 48, 10262–10267 CrossRef CAS
.
- Y. Liu, Y. Ohko, R. Zhang, Y. Yang and Z. Zhang, J. Hazard. Mater., 2010, 184, 386–391 CrossRef CAS PubMed
.
- A. Gürses, Ç. Doğar, M. Yalçın, M. Açıkyıldız, R. Bayrak and S. Karaca, J. Hazard. Mater., 2006, 131, 217–228 CrossRef PubMed
.
- L. Zhang, H. Li, Y. Liu, Z. Tian, B. Yang, Z. Sun and S. Yan, RSC Adv., 2014, 4, 48703–48711 RSC
.
- R. Godin, Y. Wang, M. A. Zwijnenburg, J. Tang and J. R. Durrant, J. Am. Chem. Soc., 2017, 139, 5216–5224 CrossRef CAS PubMed
.
- M. Meetani, M. Rauf, S. Hisaindee, A. Khaleel, A. AlZamly and A. Ahmad, RSC Adv., 2011, 1, 490–497 RSC
.
- P. K. Samantaray, S. Baloda, G. Madras and S. Bose, J. Mater. Chem. A, 2018, 6, 16664–16679 RSC
.
- F. Zhou and Y. Zhu, J. Adv. Ceram., 2012, 1, 72–78 CrossRef CAS
.
- R. Gupta, N. K. Eswar, J. M. Modak and G. Madras, Catal. Today, 2018, 300, 71–80 CrossRef CAS
.
- R. Georgiev, B. Georgieva, M. Vasileva, P. Ivanov and T. Babeva, Adv. Condens. Matter Phys., 2015, 2015, 403196 Search PubMed
.
- C.-H. Wu and J.-M. Chern, Ind. Eng. Chem. Res., 2006, 45, 6450–6457 CrossRef CAS
.
Footnote |
† Electronic supplementary information (ESI) available. See DOI: 10.1039/c9na00305c |
|
This journal is © The Royal Society of Chemistry 2019 |
Click here to see how this site uses Cookies. View our privacy policy here.