DOI:
10.1039/C9QM00441F
(Research Article)
Mater. Chem. Front., 2019,
3, 2702-2706
Ring size affects the kinetic and thermodynamic formation of [2]rotaxanes featuring an unsymmetric bis-crown ether component†
Received
6th July 2019
, Accepted 9th October 2019
First published on 11th October 2019
Abstract
Bridging of a [2]pseudorotaxane formed from an unsymmetric mono-crown ether and an ammonium salt has provided two types of [2]rotaxanes, with the axle component positioned within a large (4L) and small (4S) ring, respectively. 1H NMR spectroscopy has revealed that 4L is the kinetically favored [2]rotaxane, whereas 4S is thermodynamically favored at low temperature.
Introduction
Many rotaxanes have been synthesized for the potential development of their intriguing properties.1 Thermodynamic synthesis is a particularly powerful strategy for the preparation of rotaxanes because of its facile nature and relatively high yields.2 Kinetic studies have enabled investigations into the effectiveness of the reaction systems and the mechanisms of rotaxane formation.3 Selective (pseudo)rotaxane formations under different kinetic and thermodynamic conditions have been observed, with the categorization of both metastable and stable rotaxanes.
A simple example of a thermodynamic system is the synthesis of oligo rotaxanes featuring several macrocyclic components and an axle component bearing several recognition sites; here, the number of macrocyclic components encircling the axle component increased upon extending reaction time.4,5 An example of the kinetic and thermodynamic effects on the orientational isomerism of (pseudo)rotaxanes is that for the threading of unsymmetric macrocycles, cyclodextrins,6 and calix[6]-macrocycles7 with unsymmetric dumbbell-like axle components. An example of kinetic and thermodynamic self-sorting is that of cucurbit[n]urils (CB[n]) and a spermine derivative; here, two types of [4]pseudorotaxanes, CB[6]–CB[7]–CB[6] and CB[6]–CB[6]–CB[6], were organized kinetically and thermodynamically, respectively.8 In the latter two cases, the kinetics of (pseudo)rotaxane formation were affected dramatically by the sizes, shapes, and functionalities of the ring-shaped component and the terminal units of the dumbbell-like axle during the slipping process.9,10 Without the effects of the interactions between macrocycle and terminal unit of an axle, we wondered if it would be possible to differentiate metastable and stable rotaxanes formed under kinetic and thermodynamic conditions, respectively. Although the clipping of macrocycle precursors around dumbbell-like axles might provide an answer, the reactivity of the macrocyclization process can exert a significant influence on the kinetics of rotaxane formation.11
In this paper, we present the kinetics and thermodynamics of formation of a couple of [2]rotaxanes that formed simultaneously from an unsymmetric mono-crown ether upon intramolecular bridging via azine formation.12 To reveal the ring-size effects on the [2]rotaxane formation, we newly developed unsymmetric mono-crown ether 1 which can be easily converted into unsymmetric bis-crown ether to generate two different size rings simultaneously by adding hydrazine (Scheme 1). Insertion of an ammonium-centered axle-like component 3 into the expanded crown ether ring, followed by ring partition, provided two types of rotaxanes under thermodynamic conditions: with the dumbbell-shaped component positioned within either a large (4L) or small (4S) ring. Using this system, we could precisely evaluate the effects of ring size on the kinetics of the rotaxanes formation, without the influence of the terminal units of the dumbbell-like axle during the slipping process or the reactivity of the macrocyclization process.
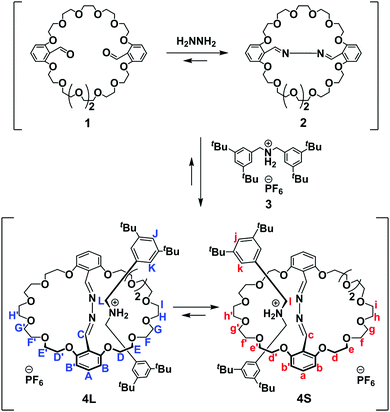 |
| Scheme 1 Formation of the bis-crown ether 2 and the [2]rotaxanes 4L and 4S. | |
Results and discussion
Synthesis and the structures of two [2]rotaxanes 4L and 4S
We prepared the unsymmetric bis-crown ether 2 from the macrocyclic mono-crown ether 1 through bridging of its two formyl moieties by means of reversible azine formation with hydrazine (Scheme 1). Upon mixing 1 (5.0 mM) and hydrazine (1.0 eq.) at 295 K in CDCl3/CD3CN (1
:
1, v/v), 1H NMR spectroscopy revealed that the signal of the formyl proton at 10.5 ppm disappeared immediately and a new signal of the azine proton appeared at 8.9 ppm, indicating that this azine formation proceeded smoothly at ambient temperature (Fig. 1a, b, and Fig. S1, ESI†).
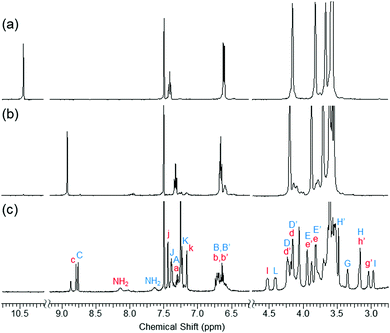 |
| Fig. 1
1H NMR spectra [500 MHz; CDCl3/CD3CN, 1 : 1 (v/v); 295 K] of (a) the mono-crown ether 1 (5.0 mM), (b) a mixture of 1 (5.0 mM) and hydrazine (1.0 eq.), and (c) a mixture of 1 (5.0 mM), hydrazine (1.3 eq.), and the dibenzylammonium salt 3 (1.0 eq.). Annotations: [2]rotaxane 4L, blue lower-case letters; [2]rotaxane 4S, red upper-case letters. | |
Two types of [2]rotaxanes, featuring the dumbbell-shaped component positioned within a large (4L) or small (4S) macrocyclic component, assembled from the bis-crown ether 2 upon adding the dibenzylammonium salt 3 (Scheme 1). 1H NMR spectrum of a mixture of 2 (5.0 mM) and 3 (1.0 eq.) in the presence of an excess of hydrazine (0.3 eq.) featured new signals at 8.7–8.8, 4.4–4.6, and 2.9–3.4 ppm that correspond to the azine, benzylic, and aliphatic protons of these two [2]rotaxanes; such signals are typical of crown ether/dibenzylammonium ion-type rotaxanes (Fig. 1c and Fig. S1, ESI†). We have assigned the set of signals at 8.80 (azine), 4.55 (benzylic), and 3.05 (aliphatic) ppm to 4S and of the set at 8.75, 4.40, and 2.95 ppm to 4L, on the basis of analogy to corresponding [24]crown-8- and [27]crown-9-type dibenzylammonium ion-based rotaxanes, respectively.12,13 The electrospray ionization (ESI) mass spectrum of the mixture featured a signal at m/z 1142.8, corresponding to [4L − PF6]+ and/or [4S − PF6]+ (calcd: m/z 1142.7), supporting the notion of the successful formation of the [2]rotaxanes 4L and 4S (Fig. S7, ESI†).
Kinetic favorability of [2]rotaxane 4L
The [2]rotaxane 4L was formed kinetically upon mixing the bis-crown ether 2 and the dibenzylammonium salt 3. To examine the kinetics of this rotaxane formation, we plotted the relative concentrations of 2 and the [2]rotaxanes 4L and 4S after the addition of the dibenzylammonium salt 3 into a solution of 2, by measuring the intensities of the signals for the respective imino groups in 1H NMR spectra [CD3CN/CDCl3, 1
:
1 (v/v); 5.0 mM of 1, hydrazine, and 3] (Fig. 2 and Fig. S3, S14b, ESI†). The ratios of 2, 4L, and 4S were approximately 93.0, 6.0, and 0.4%, respectively, immediately after the addition of 3 (15 min); the ratio gradually changed to approximately 82, 16 and 2%, respectively after 40 min at 295 K (i.e., a decrease in the relative concentration of 2 and increases in the relative concentrations of both 4L and 4S). We calculated the rates of formation of 4L (vL) and 4S (vS) at 295 K based on the distributions of the ratios of 2, 4L, and 4S during the period of the reaction from 15 to 40 min; the ratio of these rates (vL/vS) was 8.5, indicating that 4L was favored kinetically in this rotaxane-forming process.
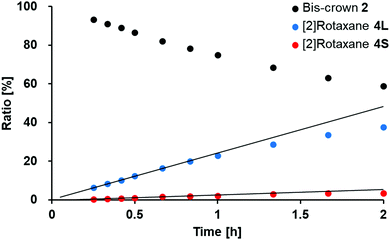 |
| Fig. 2 Distributions of the bis-crown ether 2 (black dots) and the [2]rotaxanes 4L (blue dots) and 4S (red dots) after mixing of the bis-crown ether 2 (5.0 mM, prepared in situ from the mono-crown ether 1 and an equimolar amount of hydrazine) and the dibenzylammonium salt 3 (1.0 eq.), as determined using 1H NMR spectroscopy [600 MHz; CDCl3/CD3CN, 1 : 1 (v/v); 295 K]. | |
Thermodynamic favorability of [2]rotaxane 4S
The [2]rotaxane 4S was thermodynamically favored at low (ambient) temperature when mixing the bis-crown ether 2 and the dibenzylammonium salt 3. A mixture of 2 and 3 in the presence of excess hydrazine (0.2 eq.) reached its equilibrium state after 4 h at 295 K;14 here, the relative concentrations of 2 and the [2]rotaxanes 4L and 4S were 25, 37, and 38%, respectively—again calculated from the intensities of the signals for their respective imino groups in 1H NMR spectra [CD3CN/CDCl3, 1
:
1 (v/v); 5.0 mM of 1 and 3] (Fig. 3 and Fig. S4, ESI†). The association constants of 4L (KL) and 4S (KS) at equilibrium at 295 K were 1180 ± 40 M−1 and 1240 ± 40 M−1, respectively, indicating that 4S was slightly favored thermodynamically at ambient temperature. The thermodynamic favorability of 4S at low temperature was demonstrated using variable-temperature 1H NMR spectroscopy (277–323 K). Upon decreasing the temperature, the relative concentration of 2 decreased, whereas those of 4L and 4S increased, after reaching the new equilibrium (Fig. S5, ESI†). Indeed, the ratio of 4S to 4L ([4S]/[4L]) increased remarkably upon decreasing the temperature, from 0.92 at 323 K to 1.35 at 277 K. From van’t Hoff plots recorded using the association constants of 4L (KL) and 4S (KS) at equilibrium at 277–323 K, we calculated the reaction enthalpies (ΔH) and entropies (ΔS) for the formation of the two [2]rotaxanes: for 4L, they were (ΔHL) −36.2 ± 1 kJ mol−1 and (ΔSL) −63.8 ± 2 J mol−1 K−1, and for 4S, they were (ΔHS) −42.8 ± 1 kJ mol−1 and (ΔSS) −85.6 ± 4 J mol−1 K−1 (Fig. S6, ESI†). The absolute value of ΔHS is larger than that of ΔHL, consistent with the thermodynamic favorability of 4S in this reaction at low temperature. The negative values of ΔSL and ΔSS reveal that this reaction provides conformationally restricted structures after the interlocking of the axle-like and macrocyclic components. The larger absolute value of ΔSS, relative to that of ΔSL, suggests that 4S possesses a more constrained structure, consistent with its smaller encircling crown ether component.
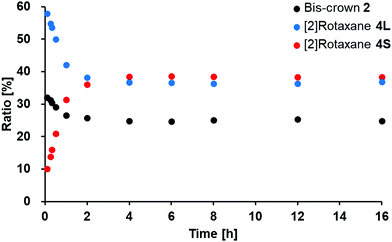 |
| Fig. 3 Distributions of the bis-crown ether 2 (black dots) and the [2]rotaxanes 4L (blue dots) and 4S (red dots) after mixing 2 (5.0 mM, prepared in situ from the mono-crown ether 1 and 1.2 eq. of hydrazine) and the dibenzylammonium salt 3 (1.0 eq.), as determined using 1H NMR spectroscopy [600 MHz; CDCl3/CD3CN, 1 : 1 (v/v); 295 K]. | |
Mechanistic rationale for the differences of kinetic and thermodynamic favorabilities of [2]rotaxanes 4L and 4S
The kinetic favorability of the [2]rotaxane 4L can be rationalized by considering the energy barriers for the ring-partition process (Fig. 4). Because of the bulkiness of the terminal 3,5-di-tert-butylphenyl units of the axle-shaped unit, axle-like component 3 can be inserted into the only expanded crown ether ring. Therefore the formation of the [2]rotaxanes presumably proceeds through three steps: (i) opening of the bis-crown ether ring, (ii) inclusion of the axle-shaped unit within the expanded macrocycle, and (iii) closing of the crown ether ring. It is likely that the ring-opening and -closing processes occur through inter- and intramolecular aminolysis by hydrazine and hydrazone, because the reaction rates of [2]rotaxane formation were significantly affected by the concentration of the excess hydrazine (Fig. 2, 3 and Fig. S3, S4, S14, ESI†).15 When the axle-like unit was positioned within the larger of the two macrocycles, the steric hindrance between the ammonium center and macrocyclic component and/or conformational restriction experienced during the ring-closing process were lower than those during the closing of the small ring, resulting in the kinetic favorability of the formation of 4L.
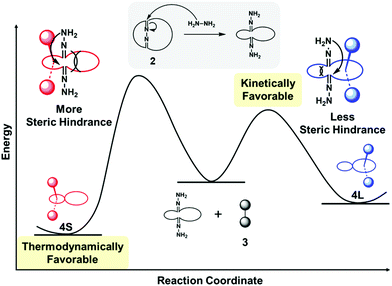 |
| Fig. 4 Schematic representation of the kinetic and thermodynamic processes leading to the [2]rotaxanes 4L and 4S. | |
The enthalpic favorability of the [2]rotaxane 4S can be rationalized by considering the greater suitability of the cavity size of its macrocyclic component (Fig. 4). When an ammonium center is encapsulated within these crown ether components, the small and large rings can be regarded as a pseudo[24]crown-8 and a pseudo[27]crown-9, respectively. The association constants of [24]crown-8/dibenzylammonium ion-type rotaxanes are generally larger than those of their [27]crown-9 counterparts because of the better fit of the cavity of the macrocyclic component around the dumbbell-shaped component;16 as a result, 4S was favored thermodynamically at low temperature.
Conclusions
In conclusion, we have examined the effects of ring size on the kinetics and thermodynamics of formation of the two [2]rotaxanes 4L and 4S. These two rotaxanes were prepared simultaneously from the unsymmetric mono-crown ether 1 and the dibenzylammonium salt 3 through bridging of the crown ring using azine formation. The kinetic favorability of 4L was revealed using time-dependent 1H NMR spectral analysis during the initial stages of the rotaxane formation process; the thermodynamic favorability of 4S at relatively low temperatures was demonstrated using variable-temperature 1H NMR spectroscopy of the systems in their equilibrium states. The flipping of the kinetically and thermodynamically favored [2]rotaxanes can be rationalized by considering the balances of two factors: the energy barriers during the ring-closing process and the fits of the sizes of the macrocyclic ring components of these [2]rotaxanes.
Experimental section
Materials and general methods
1,17-Bis(2-formyl-3-hydroxyphenoxy)-3,6,9,12,15-pentaoxaheptadecane and 1,14-bis(tosyloxy)-3,6,9,12-tetraoxatetradecane were prepared according to procedures reported previously.12 All solvents and chemicals were obtained from commercial sources and used without further purification, except for DMF, which was dried over 4 Å molecular sieves. 1H and 13C NMR spectra were recorded using JEOL ECX-500 and ECA-600 spectrometers with TMS as the internal standard. Mass spectra were recorded using JEOL JMS-700T (FAB) and Bruker Daltonics micrOTOF II (ESI) spectrometers. Infrared (IR) spectra were recorded using a JASCO FT/IR-4100 spectrometer. Silica gel column chromatography was performed using Kanto Chemical silica gel 60N. Thin-layer chromatography was performed using Merck Kieselgel 60 F254. Gel permeation chromatography (GPC) was performed using a Japan Analytical Industry LC-9201 apparatus (columns: JAIGEL-2H and JAIGEL-2.5H).
Preparation of the mono-crown ether 1
A suspension of 1,17-bis(2-formyl-3-hydroxyphenoxy)-3,6,9,12,15-pentaoxaheptadecane (0.333 g, 0.637 mmol), 1,14-bis(tosyloxy)-3,6,9,12-tetraoxatetradecane (0.348 g, 0.637 mmol), and Cs2CO3 (0.831 g, 2.55 mmol) in dry DMF (80 mL) was stirred at 100 °C for 22 h. After evaporation of the solvent, the residue was treated with water (80 mL) and extracted with CH2Cl2 (3 × 80 mL). The combined extracts were dried (Na2SO4) and concentrated under reduced pressure. The residue was chromatographed (SiO2; CH2Cl2/CH3OH, 4
:
1), with subsequent GPC purification giving 1 (0.193 g, 42%) as a colorless oil. IR (NaCl, νmax): 2933, 2871, 1685, 1595, 1473, 1418, 1351, 1302, 1255, 1180, 1109, 945, 858, 781, 720, 669 cm−1. 1H NMR (500 MHz, CDCl3): δ 3.62–3.67 (m, 20H), 3.72–3.76 (m, 8H), 3.86–3.90 (m, 8H), 4.16–4.20 (m, 8H), 6.56 (d, J = 8.6 Hz, 4H), 7.37 (t, J = 8.6 Hz, 2H), 10.50 (s, 2H) ppm. 13C NMR (125 MHz, CDCl3): δ 68.9, 69.4, 70.6, 70.69, 70.71, 71.1, 105.40, 105.43, 115.2, 135.5, 161.2, 161.3, 189.1 ppm. HRMS (FAB) m/z calcd for C36H53O15+ [M + H]+ 725.3385, found 725.3378.
Kinetic studies of the rotaxane formation
A solution of the mono-crown ether 1 in CD3CN (1.0 × 10−2 M, 3.0 × 10−1 mL, 3.0 × 10−3 mmol) and hydrazine monohydrate in CD3CN (3.0 × 10−1 M, 1.0 × 10−2 mL, 3.0 × 10−3 mmol) were mixed in a 5 mm φ NMR tube at 295 K. The mixture was added a solution of the dibenzylammonium salt 3 in CDCl3 (1.0 × 10−2 M, 3.0 × 10−1 mL, 3.0 × 10−3 mmol) in the tube at 295 K. After mixing the solution, the kinetics was traced by NMR spectroscopy. The relative concentrations of 2, 4L and 4S were determined by measuring the intensities of the signals for the respective imino groups in 1H NMR spectra (Fig. 2 and Fig. S3, ESI†).
Thermodynamic studies of the rotaxane formation
A solution of the mono-crown ether 1 in CD3CN (1.0 × 10−2 M, 3.0 × 10−1 mL, 3.0 × 10−3 mmol) and hydrazine monohydrate in CD3CN (3.0 × 10−1 M, 1.2 × 10−2 mL, 3.6 × 10−3 mmol) were mixed in a 5 mm φ NMR tube at 295 K. The mixture was added a solution of the dibenzylammonium salt 3 in CDCl3 (1.0 × 10−2 M, 3.0 × 10−1 mL, 3.0 × 10−3 mmol) in the tube at 295 K. After mixing the solution, the relative concentrations of 2, 4L and 4S were traced by the same method described in the kinetic studies (Fig. 3 and Fig. S4, ESI†).
Conflicts of interest
There are no conflicts to declare.
Acknowledgements
This study was supported by JSPS KAKENHI (JP grant number JP16K05691).
Notes and references
- Selected reviews on the applications of rotaxanes:
(a) S. J. Loeb, Chem. Soc. Rev., 2007, 36, 226–235 RSC
;
(b) B. Champin, P. Mobian and J.-P. Sauvage, Chem. Soc. Rev., 2007, 36, 358–366 RSC
;
(c) M. J. Langton and P. D. Beer, Acc. Chem. Res., 2014, 47, 1935–1949 CrossRef CAS PubMed
;
(d) A. Harada, Y. Takashima and M. Nakahata, Acc. Chem. Res., 2014, 47, 2128–2140 CrossRef CAS PubMed
;
(e) C. J. Bruns and J. F. Stoddart, Acc. Chem. Res., 2014, 47, 2186–2199 CrossRef CAS PubMed
;
(f) M. Xue, Y. Yang, X. Chi, X. Yan and F. Huang, Chem. Rev., 2015, 115, 7398–7501 CrossRef CAS PubMed
;
(g) S. Erbas-Cakmak, D. A. Leigh, C. T. McTernan and A. L. Nussbaumer, Chem. Rev., 2015, 115, 10081–10206 CrossRef CAS PubMed
;
(h) J. E. M. Lewis, M. Galli and S. M. Goldup, Chem. Commun., 2017, 53, 298–312 RSC
;
(i) T. Takata, Bull. Chem. Soc. Jpn., 2019, 92, 409–426 CrossRef CAS
.
- Selected reviews on the thermodynamic synthesis of rotaxanes:
(a) S. J. Rowan, S. J. Cantrill, G. R. L. Cousins, J. K. M. Sanders and J. F. Stoddart, Angew. Chem., Int. Ed., 2002, 41, 899–952 CrossRef CAS
;
(b) R. L. E. Furlan, S. Otto and J. K. M. Sanders, Proc. Natl. Acad. Sci. U. S. A., 2002, 99, 4801–4804 CrossRef CAS PubMed
;
(c) T. Takata, Polym. J., 2006, 38, 1–20 CrossRef CAS
;
(d) C. D. Meyer, C. S. Joiner and J. F. Stoddart, Chem. Soc. Rev., 2007, 36, 1705–1723 RSC
;
(e) M. E. Belowicha and J. F. Stoddart, Chem. Soc. Rev., 2012, 41, 2003–2024 RSC
;
(f) X. Han, G. Liu, S. H. Liu and J. Yin, Org. Biomol. Chem., 2016, 14, 10331–10351 RSC
;
(g) D. Quaglio, G. Zappia, E. De Paolis, S. Balducci, B. Botta and F. Ghirga, Org. Chem. Front., 2018, 5, 3022–3055 RSC
.
- Selected reviews on the kinetic and thermodynamic synthesis of rotaxanes:
(a) W. R. Dichtel, O. S. Miljanić, W. Zhang, J. M. Spruell, K. Patel, I. Aprahamian, J. R. Heath and J. F. Stoddart, Acc. Chem. Res., 2008, 41, 1750–1761 CrossRef CAS PubMed
;
(b) A. E. Kaifer, Acc. Chem. Res., 2014, 47, 2160–2167 CrossRef CAS PubMed
.
-
(a) W. Herrmann, B. Keller and G. Wenz, Macromolecules, 1997, 30, 4966–4972 CrossRef CAS
;
(b) C. A. Smith and D. H. Macartney, J. Org. Chem., 1998, 63, 9243–9251 CrossRef
;
(c) Y. Furusho, T. Hasegawa, A. Tsuboi, N. Kihara and T. Takata, Chem. Lett., 2000, 18–19 CrossRef CAS
.
- The equilibrium system of a kinetically favored [3]rotaxane and a thermodynamically favored [2]rotaxane has been reported: M. K. Sinha, O. Reany, M. Yefet, M. Botoshansky and E. Keinan, Chem. – Eur. J., 2012, 18, 5589–5605 CrossRef CAS PubMed
.
-
(a) R. Isnin and A. E. Kaifer, J. Am. Chem. Soc., 1991, 113, 8188–8190 CrossRef CAS
;
(b) T. Oshikiri, Y. Takashima, H. Yamaguchi and A. Harada, J. Am. Chem. Soc., 2005, 127, 12186–12187 CrossRef CAS PubMed
;
(c) A. J. Baer and D. H. Macartney, Org. Biomol. Chem., 2005, 3, 1448–1452 RSC
;
(d) T. Oshikiri, Y. Takashima, H. Yamaguchi and A. Harada, Chem. – Eur. J., 2007, 13, 7091–7098 CrossRef CAS PubMed
;
(e) J. W. Park, H. J. Song, Y. J. Cho and K. K. Park, J. Phys. Chem. C, 2007, 111, 18605–18614 CrossRef CAS
;
(f) M. Quiroga, M. Parajo, P. Rodríguez-Dafonte and L. García-Río, Langmuir, 2016, 32, 6367–6375 CrossRef CAS PubMed
.
- P. L. Manna, C. Talotta, C. Gaeta, A. Soriente, M. D. Rosa and P. Neri, J. Org. Chem., 2017, 82, 8973–8983 CrossRef PubMed
.
- E. Masson, X. Lu, X. Ling and D. L. Patchell, Org. Lett., 2009, 11, 3798–3801 CrossRef CAS PubMed
.
- Chelate cooperativity and spacer length affect the thermodynamic and kinetic assembly of divalent pseudorotaxanes; see: W. Jiang, K. Nowosinski, N. L. Löw, E. V. Dzyuba, F. Klautzsch, A. Schäfer, J. Huuskonen, K. Rissanen and C. A. Schalley, J. Am. Chem. Soc., 2012, 134, 1860–1868 CrossRef CAS PubMed
.
- For a recent example of the effects of terminal functionality on the thermodynamics and kinetics of pseudorotaxane formation, see: I. Neira, M. D. García, C. Peinador and A. E. Kaifer, Org. Chem., 2019, 84, 2325–2329 CrossRef CAS PubMed
.
- M. Horn, J. Ihringer, P. T. Glink and J. F. Stoddart, Chem. – Eur. J., 2003, 9, 4046–4054 CrossRef CAS PubMed
.
- Previously, we have constructed [2]- and [3]rotaxanes through bridging using a symmetric crown ether; see: T. Fujino, H. Naitoh, S. Miyagawa, M. Kimura, T. Kawasaki, K. Yoshida, H. Inoue, H. Takagawa and Y. Tokunaga, Org. Lett., 2018, 20, 369–372 CrossRef CAS PubMed
.
- The 1H NMR spectroscopic signals of 4L and 4S were assigned using 2D NMR spectroscopy (Fig. S10–S13, ESI†).
- Using an excess of hydrazine promoted the formation of the [2]rotaxanes. Ring-opening aminolysis of the bis-crown ether, mediated by hydrazine, is presumably the major process leading to the formation of 4S. Similar thermodynamic syntheses of rotaxanes have been reported by the Stoddart group; for example, see: S. J. Rowan and J. F. Stoddart, Org. Lett., 1999, 1, 1913–1916 CrossRef CAS
.
- Although catalytic amount of adventitious hydrazine could promote the formation of 4L and 4S in the experiments of Fig. 2 and Fig. S3, S14a, b, ESI,† the kinetic favorability of the formation of [2]rotaxane 4L was reproducible under these conditions.
- Y. Tokunaga, M. Yoshioka, T. Nakamura, T. Goda, R. Nakata, S. Kakuchi and Y. Shimomura, Bull. Chem. Soc. Jpn., 2007, 80, 1377–1382 CrossRef CAS
.
Footnote |
† Electronic supplementary information (ESI) available. See DOI: 10.1039/c9qm00441f |
|
This journal is © the Partner Organisations 2019 |
Click here to see how this site uses Cookies. View our privacy policy here.