DOI:
10.1039/D0SC01848A
(Perspective)
Chem. Sci., 2020,
11, 12386-12400
Electro-organic synthesis – a 21st century technique
Received
31st March 2020
, Accepted 18th May 2020
First published on 20th May 2020
Abstract
The severe limitations of fossil fuels and finite resources influence the scientific community to reconsider chemical synthesis and establish sustainable techniques. Several promising methods have emerged, and electro-organic conversion has attracted particular attention from international academia and industry as an environmentally benign and cost-effective technique. The easy application, precise control, and safe conversion of substrates with intermediates only accessible by this method reveal novel pathways in synthetic organic chemistry. The popularity of electricity as a reagent is accompanied by the feasible conversion of bio-based feedstocks to limit the carbon footprint. Several milestones have been achieved in electro-organic conversion at rapid frequency, which have opened up various perspectives for forthcoming processes.
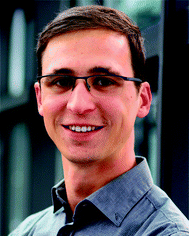 Dennis Pollok | Dennis Pollok received his B.Sc. degree in chemistry from Johannes Gutenberg University Mainz/Max Planck Institute for Polymer Research in Mainz working on polyphosphate-based flame retardants under the supervision of Dr habil. Frederik R. Wurm. During an internship at University of Toronto he worked in the group of Prof. Dr Dwight S. Seferos on conducting polyselenophenes. He received his M.Sc. in 2018 from Johannes Gutenberg University Mainz working under the supervision of Prof. Dr Siegfried R. Waldvogel where he is currently conducting research as a graduate student on electro-organic synthesis. |
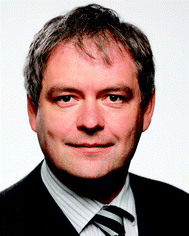 Siegfried R. Waldvogel | Siegfried R. Waldvogel studied chemistry in Konstanz and received his PhD in 1996 from University of Bochum/Max Planck Institute for Coal Research with Prof. Dr M. T. Reetz as supervisor. After postdoctoral research at Scripps Research Institute in La Jolla, CA (Prof. Dr J. Rebek, Jr), he started his own research career in 1998 at University of Münster. After his professorship in 2004 at University of Bonn, he became a full professor for organic chemistry at Johannes Gutenberg University Mainz in 2010. His research interests are novel electro-organic transformations including bio-based feedstocks from electrosynthetic screening to scale-up. In 2018, he co-founded ESy-Labs GmbH, which provides custom electrosynthesis and contract R&D. |
Motivation and background
Current social movement reveals a rising awareness of 21st century challenges. Wide spread news of dramatic natural disasters has led to discussions about climate change and the need for urgent changes. There have already been responses which also reach out to the scientific community to contribute to the restriction of greenhouse gas emissions. The severe limitations of fossil resources intensify the movement towards sustainable synthesis techniques with a strict cutback in the ecological footprint.1 Thus, several techniques have arisen, and amongst them electro-organic synthesis is rising in popularity.2–7 This methodology has re-emerged for synthetic organic chemists after being neglected for several decades, although this approach is common for inorganic transformations including industrial scale basic chemical production, like the chlor-alkali electrolysis and aluminium production by the Hall–Héroult process.8 Since Kolbe’s discoveries using electricity as a reagent for organic transformations over 170 years ago, this technique has not been accepted by the broad organic chemistry community, although less hazardous materials are being used.9 Therefore, several groups have shown interest and explored this subject to provide sufficient knowledge for further research. However, its broad application in organic synthesis is still low, although electro-organic transformations enable access to novel structures due to the unique reactivity and selectivity provided. Technical-scale electro-organic reactions, like the Baizer process carried out by Monsanto, have only rarely been performed since the 1970s.8
The broader use of electro-organic transformations will gain increasing influence due to sustainable transformations when electricity is obtained from renewable resources like wind-, solar-, or hydropower. They could profit from the emerging energy surplus from these sources to serve as a feasible Power-to-X method for storage of abundant electricity in the production of valuable fine chemicals.10,11 Electro-organic synthesis is involved in areas like electrocatalysis, redox-tags, the cation-pool method, bio-electrochemistry, and electro-organic synthesis in continuous flow.3,4,12 These techniques have also gained significant interest from industry and open pathways for various novel developments. This perspective should provide insight into recent electro-organic methods and general trends in this field, and open up prospects for future viewpoints.
Modes of operation
The application of electric current as a reagent in synthetic organic chemistry offers a vast variety of opportunities but many scientists are reluctant due to the essential adaptation of laboratories and ways of thinking with this technique. However, more and more literature is encouraging the use of this method and providing guidelines, showing the simplicity of this technique.13,14 The versatile toolbox of electro-organic synthesis relies on the fundamental principles of redox reactions using electric current as the essential reagent. Oxidations will occur as anodic transformations, whereas reductive processes adding an electron to a substrate in a single electron transfer (SET) take place at the cathode. Thus, the electrodes represent crucial parameters for electro-organic conversions, which take place as heterogenous chemical reactions between a commonly solid electrode and a substrate in a liquid electrolyte system. This electrolyte system consists of a (electro-)chemically inert solvent and supporting electrolytes to facilitate sufficient electrical conductivity. This supporting electrolyte can be a salt, acid, or base and will contribute to the formation of an electrochemical double layer at the electrode surface. This underlines that the electrode and electrolyte system can be considered as a couple that determines the electrochemical features. Inert ions which are highly soluble in organic solvents, like tetraalkylammonium hexafluorophosphate or tetrafluoroborate, have shown versatile applications and excellent performance (Fig. 1). It is worth noting that the use of perchlorates is strongly discouraged since explosive events could occur through the precipitation of organic perchlorates. Further parameters which have a major influence on the electrolysis process are the applied charge which represents the number of equivalents of reagent, the current density which controls the speed of an electrochemical process, and temperature.
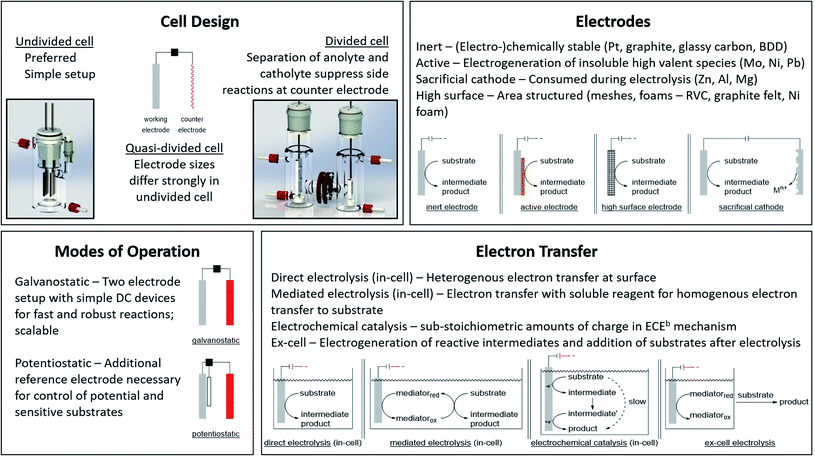 |
| Fig. 1 Modes of operation for electro-organic synthesis and electrochemical facts at a glance. Photography reproduced with permission from ref. 7. Copyright 2018 ACS. | |
Good electrode materials are (electro-)chemically inert, facilitating easy handling, and ecologically and economically beneficial use. The most common inert electrode materials are noble metals like platinum or carbon allotropes such as low-cost graphite, glassy carbon, or the high-performance material boron-doped diamond (BDD). Novel conversions can be facilitated using active electrode materials to generate immobilized high-valent metal species which catalyse the electron transfer and are regenerated by the electric current. The layer acts as a redox filter to improve selectivity, as shown for Ni or Mo in fluorinated alcohols, NiOOH in alkaline media, or PbO2 in acids.15,16
The appropriate design of devices used for electro-organic conversions offers various adjustments. Electrolysis can be performed in conventional laboratory glassware like beakers or round bottom flasks. Besides these self-made setups using standard batteries, some setups are commercially distributed like ElectraSyn and the more advanced screening and flow setups with controllable power sources sold by IKA®.17,18 The application of flow electrolysis is of major importance for scaling up electrolysis for technical application and will be discussed throughout this perspective.19 Suitable undivided cells involve the parallel installation of planar electrodes to ensure a homogeneous electrical field without local potential hot spots leading to uncontrolled side reactions.7 A semipermeable membrane or porous glass frit is used in divided cells to separate the anolyte and catholyte to suppress non-desired diffusion to the counter electrode. This is necessary when the starting material, intermediates, and products are unstable towards the counter electrode. However, a “quick and dirty” but rather practical approach combining these characteristics is a quasi-divided cell. A undivided cell is used with electrodes with electrode surface areas of dramatically different sizes. The decreased surface area of the counter electrode results in an increased current density, thus elevating the electrode potential/driving force. The chemoselectivity of the electron transfer might be reduced, leading to a statistical preference for electrolyte degradation. However, the small electrode surface area of the counter electrode affects only a small quantity of electrolyte – approx. 5% is sacrificed in side reactions which favours recyclability (Fig. 1).20,21
Electrolysis can be performed galvanostatically with constant current using an easy and low-cost setup with equipment available in common hardware stores. This robust process facilitates the possibility of fast reactions and is of high interest for scale-up in organic synthesis or technical application. However, if the substrates are more sophisticated and lack of selectivity occurs, a three-electrode setup with constant potential can be used. This potentiostatic electrolysis offers selectivity towards the desired functionalities by controlling the applied potential but prolongs electrolysis times in an elaborated setup.
A further approach for electro-organic conversion of sensitive substrates carrying labile functionalities deviates from conventional one-pot “in-cell” electrolysis with all chemicals present during electrolysis. The “ex-cell” approach generates reactive intermediates with electricity, and after completion, a substrate is added to react with the reactive species in a conventional manner.
When specific reactivity is required which is difficult to address in sophisticated substrates, mediators can be used. Mediators are electrochemically generated reagents which promote reactions, shifting the heterogenous substrate redox reactions at the electrodes towards homogeneous electron transfers from the mediator to the substrate in solution. The redox potential of the substrate is thus decoupled from the electrode, revealing novel reaction pathways of the reagent or catalyst via outer and inner sphere mechanisms.22
Despite the broad application of electrosynthesis in redox reactions, the possibility of using electric current in small amounts for redox-neutral transformations is an underexplored area. This electrochemical catalysis can enhance the performance of thermodynamically favoured but kinetically hindered transformations via an electron transfer with subsequent chemical reaction and final backward electron transfer, where the latter can either occur at the electrode surface or intermolecularly (Fig. 1).23
Current state of the art
The revival of electro-organic conversions has led to an immense variety of processes successfully replacing harmful terminal oxidizers and reducing agents, thus making chemistry “greener”.24 A good survey of these developments was provided by Baran et al., revealing the broad variety of approaches.6
Carbon–carbon bond formation has been a crucial tool in synthetic organic chemistry over years of research and is an integral part of organic synthesis. Several approaches use organo-catalysis or transition metal-based catalysts to selectively form these bonds. Little et al. investigated electro-organic approaches such as reductive carbon–carbon couplings with olefin and carbonyl compounds focusing on mechanistic investigations to provide deeper insight into electro-organic reaction mechanisms.25 Schäfer et al. used Kolbe electrolysis for cascade reactions, forming novel carbon–carbon bonds in complex architectures (Scheme 1).26
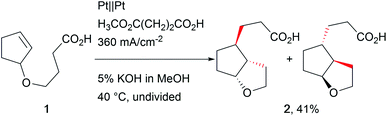 |
| Scheme 1 Kolbe cascade reaction by Schäfer et al. | |
One of the major challenges in organic chemistry is direct, selective C–H activation due to the high oxidation potentials. In this regard, Moeller et al. have established anodic olefin coupling reactions to access cyclic substrates which include sophisticated functionalities using a conventional 6 V battery in an undivided beaker cell (Scheme 2).11,27,28
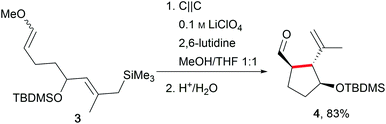 |
| Scheme 2 Anodic olefin coupling reactions by Moeller et al. | |
Major contributions to direct electrochemical C–H activation have been made by Yoshida et al. with the development of the “cation-pool” method, which traps electro-chemically generated highly reactive intermediates at low temperature, and the addition of a nucleophile enables the formation of new bonds including valuable carbon–carbon bonds (Scheme 3).5,29
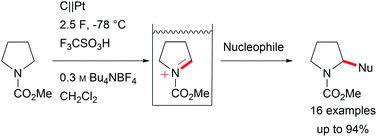 |
| Scheme 3 Versatile cation-pool method by Yoshida et al. | |
Focusing on biaryls which are highly important for materials science and active pharmaceutical ingredients (APIs), and occur in natural products, electro-organic transformations enable a broad variety of possibilities.30 Conventional reductive cross-couplings require pre-functionalization of the substrates, and oxidative reagent-mediated reactions lack selectivity.31 Selective electro-catalysis employing halide functionalized substrates for challenging transformations enables less waste generation but still requires pre-functionalization for arenes with high oxidation potentials.7 However, these approaches are hindered by waste production or reagent necessity. Thus, important developments in direct oxidative couplings have been made by Waldvogel et al. who gained access to several symmetric and non-symmetric biphenols as well as phenol–(hetero)arene cross-coupled products with reagent- and metal-free electro-organic protocols (Scheme 4). The application of boron-doped diamond (BDD) electrodes in combination with 1,1,1,3,3,3-hexafluoropropan-2-ol (HFIP) as solvent exhibited high performance and robustness.32 It is worth noting that the selectivity for cross-coupling is determined by a solvent effect.33
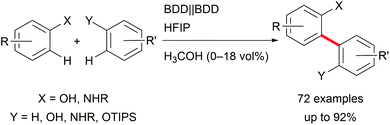 |
| Scheme 4 Phenol/aniline–arene cross-coupling by Waldvogel et al. | |
Despite the necessity for additional reagents and increasing distance from the principles of green and sustainable chemistry, electro-catalytic transformations are gaining increasing attention for promoting novel reactions with metal complexes, organo-catalysts, or bio-electro catalysts. In comparison to photo-redox catalysis, electro-catalysis can not only use a small part of the solar spectrum but take advantage of the whole energy range without loss.34 Ackermann et al. are among the leading researchers in this field, achieving a broad variety of different selective C–H activations towards valuable structural motifs using earth-abundant 3d metals. They found outstanding properties for cobalt electro-catalysts in oxidations involving alcohols, alkenes, alkynes, amines, allenes, carbon monoxide, carboxylic acids, and isocyanides (Scheme 5).34,35 Mei et al. adopted these transformations for unsaturated and saturated aliphatic substrates using palladium, copper, and iridium in electro-catalysis.36
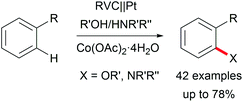 |
| Scheme 5 Electro-catalytic C–H activation by Ackermann et al. | |
The di-functionalization of unsaturated aliphatic carbon–carbon bonds was intensively studied by Lin et al. The application of transition metal electro-catalysts promoted the shuttle of electrons and atoms for a broad substrate scope with installed azide, chloride, bromide, trifluoromethyl, phosphonate, and nitrile moieties including tandem reactions for intramolecular ring closure (Scheme 6).37 Nitrogen moieties are crucial in natural products and APIs and thus among the hot topics in contemporary research.38 Thus, the importance of azide functionalities for subsequent reduction to primary amines is highly acknowledged.39
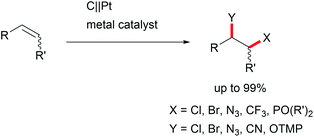 |
| Scheme 6 Electro-catalysis for alkene di-functionalization by Lin et al. | |
Various valuable structures carrying primary aromatic amine functionalities are found in APIs, natural products, and advanced materials.40,41 However, electrochemical access to anilines faces the issue of low oxidation potentials of arylamines and overoxidation of the products. A game-changing breakthrough was achieved by Yoshida et al. by the C–H amination of arenes through trapping radical cations with pyridinium intermediates and subsequent aminolysis using piperidine (Scheme 7).41,42
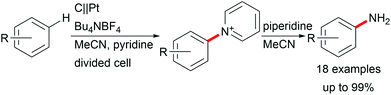 |
| Scheme 7 Arene C–H amination by Yoshida et al. | |
The positively charged intermediate Zincke salts prevent overoxidation.43 The high tolerance of labile functional groups was extended by Waldvogel et al. using BDD anodes to overcome the limitations of electron-rich substrates.40 Primary amines are of high importance, whereas secondary amines can only be electro-generated by using heterocyclic compounds with further ring opening or subsequent ring closure.42,44
Baran et al. demonstrated the electro-catalytic amination of arenes with different amino moieties using nickel compounds to form secondary and tertiary amino moieties.45 Xu et al. developed a variety of protocols for generating radicals, leading to a broad variety of heterocyclic motifs. Using the properties of (2,2,6,6-tetramethylpiperidin-1-yl)oxyl (TEMPO) or ferrocene as efficient redox mediators, the researchers accessed valuable nitrogen-containing heterocycles. They achieved these transformations by the generation of N-centred radicals like N-amidyl radicals or iminoxyl radicals.46 A further approach for utilization of N-centred radicals was reported by Waldvogel et al. for the facilitation of intramolecular N–N bond formation.47
The biological activity of modern APIs is highly enhanced by installation of fluoro moieties which increases cell penetration and leads to effective drugs.48 Thus, replacing hazardous conventional fluorination agents like Selectfluor with electrochemically generated fluoride is of major importance. Waldvogel et al. have reported several transformations towards heterocyclic compounds using less harmful reagents like triethylamine–hydrogen fluoride to anodically generate hypervalent difluoroiodoarenes to serve as mediators.49 Fuchigami et al. developed various techniques for selective electro-organic fluorinations (Scheme 8).50 Anhydrous reaction conditions are key for these transformations due to the decreased nucleophilicity of fluoride in the presence of water, and ionic liquids are viable media (Scheme 8).51 Alkali-metal fluorides and poly(ethylene glycol) provide appropriate fluoride nucleophilicity.52
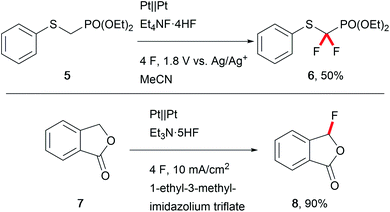 |
| Scheme 8 Electro-organic fluorination reactions by Fuchigami et al. | |
These seminal developments in the successful replacement of hazardous oxidizers and reducing agents and the unique reactivity of electro-organic conversions promote novel reaction pathways with highly tuneable selectivity. The opportunity to generate intermediates which are only accessible in this way promotes the application of electro-organic conversions in future organic chemical synthesis and enables transformations which are difficult to perform with conventional techniques. Therefore, industrial processes are even performed on the several ton scale, for example the production of precursors for nylon by the Baizer process, the production of fragrances, or the fluorination of hydrocarbons in the Simons process. Besides these, various other electro-organic transformations are carried out on a smaller scale in industry for the production of agrochemicals, anti-inflammatories, or antibiotics (Fig. 2).8,16,20,53,54
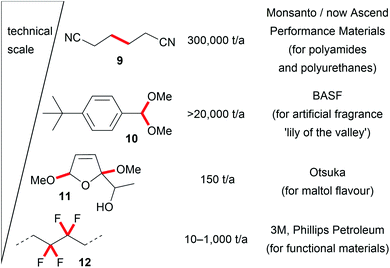 |
| Fig. 2 Some electro-organic processes on the technical scale. | |
As stated above, electro-organic transformations offer a significant advantage for organic laboratories in cases where (I) appropriate reagents are not easily accessible, (II) the reactivity has to be precisely controlled, (III) acceleration of complex catalytic cycles using electricity is possible, (IV) redox reactions with disadvantageous kinetics can be performed easily, and (V) no runaway reactions are possible. These aspects show clearly that electro-organic synthesis is a technique with future prospects.
New frontiers in electro-organic conversions
As increasing numbers of organic chemists stumble upon the benefits of electro-organic conversions, the field offers a broad perspective for developments in the near future. However, several emerging branches have to consider holding to the principles of “green chemistry” which is not always considered now.55,56
Reproducibility as a key parameter in process development
For successful establishment of electro-organic synthesis in laboratories, crucial aspects have to be considered to facilitate its application. A major criterion is the parameter of reproducibility of experiments which is accompanied by a variety of different parameters influencing the reaction (Fig. 3).
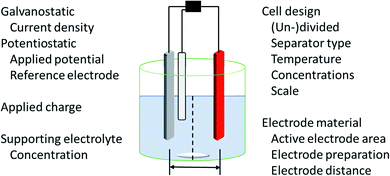 |
| Fig. 3 Crucial parameters for reproducibility in electro-organic synthesis (batch-type). | |
Electro-organic chemistry is in an early stage of development and faces the challenge that entire data sets need to be given. Newcomers in this interdisciplinary field are often unaware of important electrolysis parameters. This hinders reproducibility and thus further distribution of the technique. Thus, standardization of the data sets given has to be established along with an inexpensive and easily applicable setup for beginners and advanced researchers, where beaker cells are the desired design due to their availability in every laboratory. The first developments have been made by Baran et al. with ElectraSyn and Waldvogel et al. with a screening system for up to eight simultaneous reactions in undivided or divided cells as well as a package for electro-organic synthesis in flow-reactors.17,18 These systems should be chosen in preference to amateurish home-made flasks with non-defined parameters which were used in the past, for example using simple batteries as electricity sources does not allow sufficient control over the reaction and hinders reproducibility, although it demonstrates the easy application of electro-organic setups.57 Parameters such as the electrode distance are often not given but are crucial for electrochemical success.58 It is important for the literature to distinguish between simple demonstrations of possible electrolysis and their application in a useful laboratory synthetic protocol. These challenges can be overcome through the widespread establishment of electro-organic synthesis in education.
Novel electrode materials beyond limits
The development of novel electrode materials enhances the performance of electro-organic conversions. Less electrode corrosion with metal-free, carbon-based materials such as boron-doped diamond (BDD) is preferred.59 Electrode materials like BDD offer unique selectivity, and are (electro-)chemically robust, resistant to fouling processes, and self-cleaning, enabling easy, maintenance-free application in electro-organic chemistry in a wide potential window.60 Thus, outstanding performances in the synthesis of complex molecular structures with good yields are feasible for the synthesis of drugs like licarin A (14, Scheme 9).61
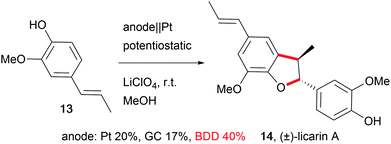 |
| Scheme 9 Performance of boron-doped diamond anodes in electro-organic synthesis. | |
In particular, carbon allotrope materials will gain significant influence in society due to the sustainable character of the materials and their short- to long-term economic benefits in comparison to the scarcity of transition metals. Still, metal electrodes offer potential for development in the application of alloys, for example using leaded bronze instead of lead as the cathode preserves the properties of lead but suppresses cathodic corrosion. This enables easier handling due to the lowered toxicity and enhanced mechanical properties of this material.12,62 The efficiency of electro-organic transformations can be improved by the design of three-dimensional structured electrodes like foams or meshes which increases the active electrode surface area which results in shorter reaction times. A well-known, inert, and sustainable electrode material in this category used by many groups is reticulated vitreous carbon (RVC).27,41,44,63 Alternatives with successful performance are graphite felt or foam materials like nickel.64 Moreover, several electro-organic processes generate gaseous products in side reactions, which makes safe handling challenging, and the adsorption of gases at the electrode lowers the active electrode surface area. Novel designs can include gas-diffusion electrodes which are already employed as oxygen-depolarized cathodes in the chlor-alkali process to enable the addition of gaseous reagents to suppress cathodic hydrogen evolution.65 The design of novel electrodes has to focus on performance, sustainability, and economical aspects. For cathodic processes, the counter anode requires cost-intensive maintenance which is a barrier for technical application.
Robust processes for broad applications
A major drawback of electro-organic transformations is the long reaction time due to the sensitivity of substrates towards higher current densities, which hampers broader acceptance of the technique in organic chemical laboratories. A broad current density range is beneficial for easy application and makes the system robust towards fluctuations in electricity consumption and reaction parameters.66 Still, only a few electro-organic processes are carried out at high current densities, for example the Kolbe electrolysis and the Baizer process.54 Exemplary developments have been reported by Waldvogel et al. for the anodic cross-coupling of phenols with arenes, yielding the product 17 with excellent selectivity and good yields of up to 70% at a high current density of 100 mA cm−2 which shortens the electrolysis time drastically (Scheme 10).67 Mass transport is enhanced by the low viscosity and microdomains in the HFIP–methanol mixture to facilitate robustness over a current density range of two orders of magnitude.
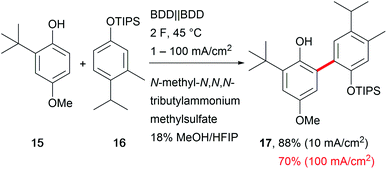 |
| Scheme 10 Robust anodic carbon–carbon bond formation at different current densities; selectivity for the cross-coupling reaction >100 : 1. | |
Galvanostatic electrolyses are robust processes which yield the desired product and are unaffected by potential fluctuations, leading to selective reactions with limited waste stream. The reactions are unsusceptible to over-conversion of substrates which would lead to a vast range of undesired by-products and therefore a demanding workup procedure. For the limited examples of robust electro-organic processes, research should focus on considering variations in the parameters which do not lead to inferior results or failure. This is important for researchers who wish to start using electro-organic conversions, as it will facilitate a trouble-free start.
Paired electrolysis
The development of novel processes is driven by the issues of sustainability and cost-efficiency. However, awareness of the whole electrolysis system is often neglected and could reveal powerful processes if both electrode reactions generate value-added products.3,4 Decomposition of the solvent resulting in hydrogen evolution is not considered as such. This approach is known as a paired electrochemical process and can facilitate highly sustainable synthesis due to the efficient use of electricity and minimization of energy and chemical requirements as well as waste generation. Industrial scale inorganic electrolysis is already taking advantage of this concept in the refining of metals as well as the generation of chlorine and sodium hydroxide in the chlor-alkali process. Paired electrolysis can be performed using different approaches including undivided setups (Scheme 11).68
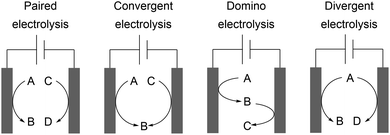 |
| Scheme 11 Different types of paired electrolysis. | |
An example of paired electrolysis on a multi-ton scale is performed by BASF SE to obtain p-tert-butylbenzaldehyde dimethyl acetal (10) and phthalide (7) in a single paired process with 100% atom efficiency and 180% current efficiency (Scheme 12).69 This process is only feasible if the chemicals involved can be easily separated and purified by distillation, crystallization, and filtration, which is applicable for this reaction.
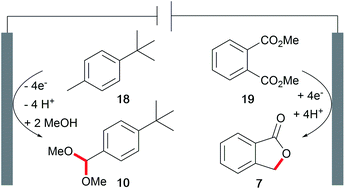 |
| Scheme 12 Paired electrolysis in the synthesis of phthalide and p-tert-butylbenzaldehyde dimethyl acetal performed by BASF SE. | |
Convergent electrolysis generates one product from two substrates and depends on accurate matching of the oxidation potentials, for example the synthesis of glyoxylic acid from glyoxal and oxalic acid.70 Divergent electrolysis transforms one substrate into two different products, for example the conversion of glucose into gluconic acid and sorbitol.71 An elaborate technique using mediated electrolysis generates the desired product at both electrodes from a single substrate. A substrate is converted into a product whereby the counter electrode generates a powerful redox reagent which facilitates substrate conversion to the desired product. Sodium gluconate can be converted into D-arabinose in a linear paired electrolysis with a Fe2+/Fe3+ mediator system with current efficiencies of up to 127%. This counterintuitive process is enabled by the direct anodic transformation and indirect cathodic oxidation by electrogenerated peroxide species as powerful oxidizers produced by reduction of oxygen in aqueous medium.72 Successful linear paired electrolysis depends on the cooperation of several kinetic processes and detailed reaction optimization. A further possibility is domino synthesis where a substrate is firstly converted at the working electrode and then after diffusion is converted at the counter electrode in an undivided cell. An example was reported by Waldvogel et al. for converting aldoximes into the corresponding nitriles via nitrile oxide intermediates.73
Scale-up – electro-organic synthesis in flow cells
Despite the successful development of electro-organic processes, the challenging task of scale-up for industrial application has to be faced. The barrier for technical application is mainly centred in the limitations of less robust electro-organic transformations.8,20,74 This is accompanied by one of the most significant disadvantages of electrochemical processes, the frequently used potentiostatic approach. This requires an elaborate three-electrode arrangement and long reaction times due to the decreasing amount of substrate undergoing electrolysis which lowers the current in order to maintain the fixed potential. Similar to conventional transformations, several parameters have to be considered for scale-up. The volume-to-surface area ratio shrinks in larger batch reactors which can be encountered in the application of bipolar stacked electrode setups.7 Besides this, mass transport occurs from an electrode into the bulk solution for post-electrolysis processes and requires efficient mixing. Moreover, electrolysis generates huge amounts of heat, which mostly occurs at the electrodes and therefore, a larger surface area faces challenges in efficient cooling. These issues have led to the development of flow-electrolysis cells which can operate in galvanostatic electrolysis and do not require a sophisticated and cost-intensive electronic setup to convert large amounts of substrates with high efficiency.18 Flow-electrolysis enables the economically beneficial continuous generation of value-added products. Several flow-electrolysis cells in a row, each applying a certain part of the necessary charge, can be used to tackle the mentioned challenges (vide supra).75 The conversion of sensitive substrates can be enabled by continuous removal of products, decreasing potential overoxidation (Table 1).
Table 1 Comparison of batch and flow electrolysis
Parameter |
Batch 
|
Flow 
|
Continuous |
No |
Yes |
Sensitive substrates |
Potentiostatic |
Galvanostatic |
Temperature control |
Less efficient |
Efficient |
Volume-to-surface area ratio |
Low |
High |
Several systems have been developed for flow-electrolysis, in which micro-flow setups suffer from a small electrode surface area and inefficient economic productivity. Long-channel flow cells are only applicable for processes which are robust towards a wide range of current densities and lack possibilities for gas liberation (single pass conversions).76 Thus, scale-up is usually achieved by increasing the number of flow cells and switching between flow cells to optimize the electrolysis conditions and increase the overall capacity. Despite this, research has revealed the benefits of flow cells including high surface area-to-volume ratio, narrow electrode gaps for low electrical resistance, and efficient temperature control.19,77 Narrow gap electrolysis cells need no supporting electrolyte when residual conductivity is present, e.g. due to traces of water.75
Electrochemistry in the synthesis of natural products and APIs
The improvement of several syntheses which use electricity has initiated the application of these transformations in complex total synthesis protocols, as reviewed by Kärkäs et al.27,57,78 The major advantage of electro-organic synthesis in comparison to conventional transformation is the absence of metal contamination in the products if carbon allotropes are used as electrodes, which is highly favoured in the synthesis of active pharmaceutical ingredients (APIs).79 Electro-organic synthesis possesses several relevant features for these syntheses like mild reaction conditions, shortened pathways, atom- and cost-efficiency, and avoidance of (over-)stoichiometric hazardous reagents. However, electro-organic conversions of complex molecules are still rare because most electro-organic protocols use ordinary substrates containing a single redox-active functionality.80 This hampers their application in total syntheses due to selectivity challenges as well as difficulties in scalability. Some electro-organic syntheses of complex molecular architectures are only possible using electricity and/or are not accessible with conventional methods, for example the synthesis of dixiamycin B (20). This natural product was synthesized using a direct intermolecular anodic N–N coupling reaction of two xiamycin B molecules (Fig. 4).81
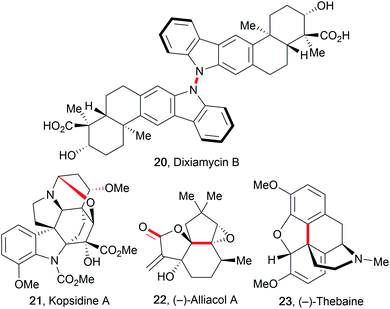 |
| Fig. 4 Natural products and APIs with electro-organic key transformations. | |
An early and prominent example of late stage functionalization in electro-organic synthesis is the cyclization technique used by Moeller et al. to form alliacol A (22). A carbon–carbon bond was formed intramolecularly between a protected enol and a furan moiety.27,57 Alkaloids are a major class of naturally occurring compounds used in medicine. Thus, shortened synthesis protocols for kopsidine A (21), (−)-thebaine (23), and (−)-oxycodone are of high importance.82 The synthesis of kopsidine A involved an oxidation at the bridging nitrogen which resulted in the formation of an intramolecular C–O bond for ring closure after addition of methanol. (−)-Thebaine and (−)-oxycodone were formed from a trioxygenated laudanosine derivative by a regio- and diastereoselective anodic C–C bond formation.
Recently, a patent by Bayer reported the concise synthesis of finerenone (25), an API for heart disease treatment, through racemisation of the wrong enantiomer via an anodic and cathodic sequence for substrate recovery (Scheme 13).83
 |
| Scheme 13 Electro-organic racemisation in the synthesis of an API by Bayer. | |
Stereoselective electro-organic conversions
The synthesis of natural products requires the installation of chiral information. In comparison to conventional asymmetric catalysis, only a few electro-organic conversions facilitate asymmetric reactions, commonly with unsatisfactory enantiomeric excess (ee).84–86 The early days of asymmetric electrolysis focused on chiral supporting electrolytes, solvents and asymmetric functionalization of electrodes, whereas modern techniques rely on chiral mediators. Electro-catalytic asymmetric synthesis takes advantage of precisely investigated asymmetric organic synthesis with electrons as sustainable reagents. The use of chiral auxiliaries enables pre-functionalization with the desired stereo information using recyclable electro-auxiliaries (Scheme 14).84,85
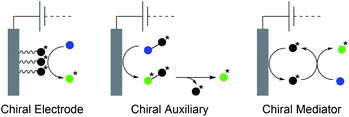 |
| Scheme 14 Asymmetric electrolysis modes with significant enantiomeric excess. | |
For the modification of electrode surfaces with chiral molecules, carbon electrodes with polymers, organometallic complexes, or biomolecules are frequently used as chiral frameworks which are recyclable with high turnover numbers (TON).85 The first successful approach was reported in 1975 by Miller et al. for coating graphite with (S)-(−)-phenylalanine methyl ester to reduce 4-acetylpyridine asymmetrically.87 Modern approaches use poly-L-valine or spiroxyl motifs to obtain higher coating efficiencies and higher enantiomeric excesses (Scheme 15).88
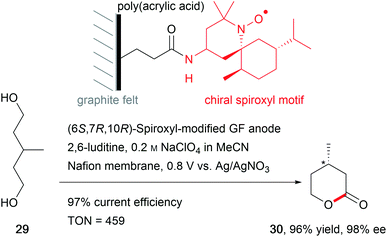 |
| Scheme 15 Spiroxyl-modified graphite felt (GF) anode for asymmetric electrolysis. | |
Novel approaches to chiral electrode surfaces use inexpensive, readily available materials for chiral encoding of the surface to obtain enantiomeric excesses of up to 80% in pulsed electro-organic synthesis. This imprinting is performed by metal (e.g. Pt, Ni) deposition on a liquid crystal with adsorbed asymmetric molecules as a template for the later product formation during electrolysis. After removal of the template, a mesoporous metal remains which is decorated with stable chiral cavities. During electrolysis, these cavities hinder formation of the non-desired enantiomer by trapping the substrate in the cavity and blocking one side of the molecule to attack by the co-reagent. In contrast, conventional metal electrodes give racemates due to there being no preferred orientation of the substrate at the electrode surface (Fig. 5).89
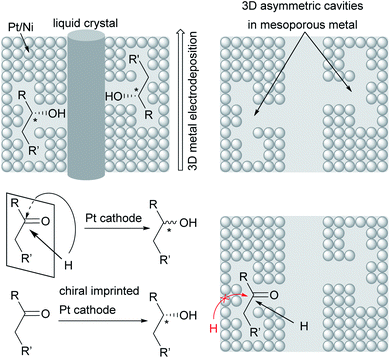 |
| Fig. 5 Schematic illustration of the manufacture of a chiral imprinted Pt or Ni electrode by 3D metal deposition on a liquid crystal with adsorbed chiral molecules, including a demonstration of enantiomer discrimination at the imprinted electrode surface during electrolysis. | |
The rising interest in the application of chiral mediators, especially for organic compounds, correlates with the formation of the desired stereoisomer in higher optical yields. N-oxyl motifs90 and iodoarenes91 are frequently used compounds. In several reports, the application of a chiral amine promoted asymmetric electrolysis (Scheme 16).92
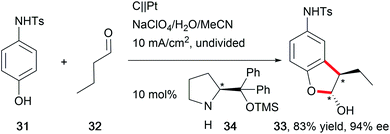 |
| Scheme 16 Enantiomerically pure amine as electro-organocatalyst. | |
Transition metal complexes benefit from fine tuning of ligands and thus are tailored catalysts. However, this leads to undesired metal contamination in the products.93 A topic of current interest is biocatalysis which can be combined with electro-catalysis to perform stereoselective epoxidation.94 The combination of enzyme-based reduction with electro-catalysis enabled access to asymmetric amino acids in optical yields up to almost 100%.95 Besides these approaches which use external stereo information, the use of chiral auxiliaries is a further approach.96 Several promising results have been reported in this field, with the drawbacks of the application of metals and low enantiomeric excess encouraging the scientific community.
Electro-valorisation of renewable bio-based feedstocks
In the past, a huge number of resources for chemical synthesis were of fossil origin. The limitations of fossil resources caused research to focus on the sustainable conversion of chemicals and generation of value-added products derived from biomass like lignin, carbohydrates, proteins, terpenes, and fats as promising feedstocks.16,55,97 Electrochemistry has exhibited powerful capabilities and can be combined with renewable feedstocks for the generation of fuels and chemicals.
The conversion of the greenhouse gas CO2 for application as a C1-building block in synthetic chemistry is a major challenge. Electrochemistry offers possibilities for this conversion due to its tunability in a wide potential window to overcome the high redox potential of CO2. Progress has been made in transformations into valuable building blocks like CO for conversion into syngas, formic acid, or ethylene depending on the cathode material.98 Copper cathodes yield mainly methane and ethylene, whereas lead and mercury mostly give formic acid, and gold, silver, and zinc generate CO. These developments are covered by several reviews.99
The cross-linked polyphenol lignin is the second most abundant biopolymer and is accumulated in the paper industry as a by-product. As well as its common use in heat and electricity generation, lignin can be used as an inexpensive feedstock for aromatic fine chemicals like the common aroma chemical vanillin (35).100–103 The conversion of lignin is an immensely growing area of chemical research.104–107 Kraft lignin is formed in the pulping process and is challenging to decompose using conventional chemical methods due to the high robustness of the material. Therefore, it is necessary to use hazardous reagents and methods.107,108 The superior performance of electro-organic processes in this field can be shown by several processes established to generate value-added products like vanillin on Au, Cu, Ni, PbO2, Pt, or dimensionally stable anodes.106,109 Moreover, approaches using Ni/P-foam and Ni/NiOOH electrodes, and a photo-electrochemical method using TiO2 nanotubes and Ta2O5–IrO2 films have been reported (Scheme 17).100–102,110
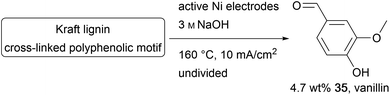 |
| Scheme 17 Electro-organic valorization of lignin as a renewable feedstock. | |
However, many approaches facilitate electrochemical Kraft lignin degradation but generate mixtures of several products which are difficult to separate, and only a few methods enable highly selective transformation.100 Stahl et al. used an aminoxyl-mediated anodic process to convert the primary alcohol moieties in lignin into carboxylic acids which can be used as polyelectrolytes.105 A paired approach for the conversion of less robust organosolv lignin was reported by Zhu et al., with direct anodic oxidation at a RuO2/Ti-mesh electrode and generation of H2O2 at the graphite felt cathode, which initiated further degradation of the lignin.111 Stephenson et al. obtained a broad variety of value-added compounds from native lignin with photo- and electro-redox catalysis under ambient conditions.112 Moeller et al. electrochemically accessed valuable aromatic chemical building blocks from sawdust as an ordinary raw material.113
The abundance of saccharides in nature offers opportunities for renewable biofuel or chemical production. Thus, several electro-organic conversions have been established to selectively synthesise the corresponding moieties either by direct anodic oxidation or by using a TEMPO mediator. Glucose was used as a bio-feedstock for sorbitol synthesis at Raney®-Ni cathodes, for use in cosmetics and medical applications, or as a sweetener in the food industry.114 The electro-conversion of saccharides provides further access to valuable fine chemicals like the pyridoxine vitamin B6 (37, Scheme 18).115 The value-added products furfural and 5-hydroxymethylfurfural, obtained from saccharides using conventional chemical transformations, show promising structures for further modification into potential biofuels and chemicals for biopolymer synthesis.116
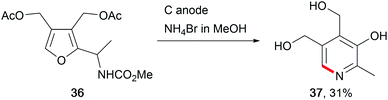 |
| Scheme 18 Electro-conversion of naturally derived furans into pyridoxines, like vitamin B6. | |
Versatile functionalization is possible in fatty acids as natural energy stores to obtain value-added products. Electro-organic conversion was successfully performed to obtain diesel-like olefin–ether mixtures as potential biofuels.117 Moreover, the common Kolbe electrolysis is suitable for di-decarboxylation in a cross-coupling reaction to form muscone precursor 40 (Scheme 19).118 Recent developments include the generation by Kolbe electrolysis of bio-based epoxy resins from viscous tall oil which is a by-product in the pulping industry.119
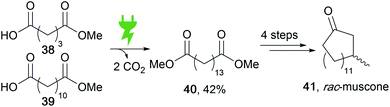 |
| Scheme 19 Mixed Kolbe electrolysis of fatty acids in the synthesis of rac-muscone. | |
The abundance of hydrocarbon-based bio-feedstocks is supplemented by amino acids as versatile renewable nitrogen sources to replace the synthesis from petrochemicals with hazardous external nitrogen sources. The profitable one-pot electro-organic conversion of glutamic acid into adiponitrile (9), which is a building block for polyamides and polyurethanes, is an alternative approach in contrast to the petro-based industrially applied Baizer process (Scheme 20).120 Moreover, the cathode dependent conversion of lysine to the corresponding amine, amide, or nitrile was established and adopted for several substrates.121
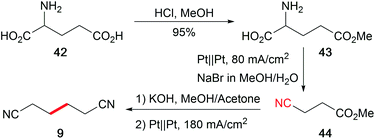 |
| Scheme 20 Electro-conversion of amino acids in industrial processes to form nylon precursors. | |
Anodic processes in water splitting for value-added chemicals
There is no doubt that a future society will rely on hydrogen or methanol as chemical fuel equivalents for mobility applications. Electro-chemical water splitting is a current topic of research for producing high quality hydrogen and oxygen. However, the over-potential for oxygen evolution within water splitting is still a major issue.122 Instead of producing molecular oxygen, the generation of high-performance oxidizers in an ex-cell process can overcome this challenge. These high-performance oxidizers could be persulfate or periodate and can catalyse further processes.123 Here, integrated solutions for the recovery and recycling of the oxidizers are required (Fig. 6).
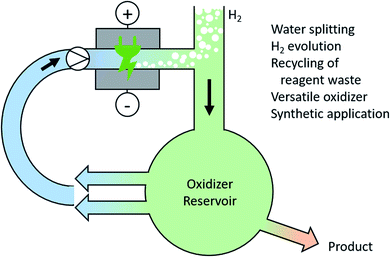 |
| Fig. 6 Anodic generation of high-performance oxidizers during water splitting and their versatile use for synthetic applications. | |
Mechanistic investigations as the key to novel pathways
Electro-organic conversions have emerged at a rapid speed, providing numerous techniques. Reports usually provide a mechanistic rationale for the electro-organic transformation observed. However, in most cases the postulated mechanism is supported by cyclic voltammetry (CV) only.13 Cyclic voltammetry provides access to the redox potentials of the substrates and investigation of setup limitations with respect to electrolyte and electrodes. The analysis of solvent potential windows and calculation of the electrochemical potentials of several molecular motifs has enhanced our understanding of the processes that occur during and after electrolysis.20,124 However, cyclic voltammetry only traces the heterogeneous electron transfer at the solid–liquid interface. Thus, often it does not provide access to the following chemical processes which shows a lack of structural sensitivity. This blindness leads to a recommendation to use different techniques and to combine CV measurements with appropriate spectroscopy and suitable control experiments.125 Understanding these micro processes will enable the optimization of processes on a macromolecular scale and the development of even more elaborate processes.
Conclusions
The consideration of different aspects in electro-organic synthesis indicates that it is a promising technique with several discoveries but plenty of hidden treasures which lead to increasing developments. In particular, the use of fluctuating and abundant electricity to make value-added chemicals will be a game-changer in future chemical operations. The use of already established electro-organic methods to replace difficult conventional transformations and the successful conversion of renewable bio-based feedstocks are the first evidence of its potential in research. Mastering these challenges will lead organic chemical synthesis into a new era of sustainable chemistry.
Conflicts of interest
The authors declare no conflict of interest.
Acknowledgements
Financial support from the Verband der Chemischen Industrie (Kekulé Fellowship to D. Pollok) and the Deutsche Forschungsgemeinschaft in the frame of UNODE (Wa1276/24-1) is gratefully acknowledged.
Notes and references
- R. Cernansky, Nature, 2015, 519, 379 CrossRef PubMed.
-
(a) E. J. Horn, B. R. Rosen and P. S. Baran, ACS Cent. Sci., 2016, 2, 302 CrossRef CAS PubMed;
(b) M. D. Kärkäs, Chem. Soc. Rev., 2018, 47, 5786 RSC.
- S. R. Waldvogel and B. Janza, Angew. Chem., Int. Ed., 2014, 53, 7122 CrossRef CAS PubMed.
- A. Wiebe, T. Gieshoff, S. Möhle, E. Rodrigo, M. Zirbes and S. R. Waldvogel, Angew. Chem., Int. Ed., 2018, 57, 5594 CrossRef CAS PubMed.
- J.-i. Yoshida, K. Kataoka, R. Horcajada and A. Nagaki, Chem. Rev., 2008, 108, 2265 CrossRef CAS PubMed.
- M. Yan, Y. Kawamata and P. S. Baran, Chem. Rev., 2017, 117, 13230 CrossRef CAS PubMed.
- S. R. Waldvogel, S. Lips, M. Selt, B. Riehl and C. J. Kampf, Chem. Rev., 2018, 118, 6706 CrossRef CAS PubMed.
-
D. Pletcher and F. Walsh, Industrial Electrochemistry, Blackie Academic & Professional, London, New York, 2nd edn, 1993 Search PubMed.
- H. Kolbe, J. Prakt. Chem., 1847, 41, 137 CrossRef.
- A. Sternberg and A. Bardow, Energy Environ. Sci., 2015, 8, 389 RSC.
- B. H. Nguyen, A. Redden and K. D. Moeller, Green Chem., 2014, 16, 69 RSC.
-
F. Marken and M. Atobe, Modern Electrosynthetic Methods in Organic Chemistry, CRC Press, Taylor & Francis Group, Boca Raton, 2018 Search PubMed.
- N. Elgrishi, K. J. Rountree, B. D. McCarthy, E. S. Rountree, T. T. Eisenhart and J. L. Dempsey, J. Chem. Educ., 2018, 95, 197 CrossRef CAS.
- C. Kingston, M. D. Palkowitz, Y. Takahira, J. C. Vantourout, B. K. Peters, Y. Kawamata and P. S. Baran, Acc. Chem. Res., 2020, 53, 72 CrossRef CAS PubMed.
- S. B. Beil, T. Müller, S. B. Sillart, P. Franzmann, A. Bomm, M. Holtkamp, U. Karst, W. Schade and S. R. Waldvogel, Angew. Chem., Int. Ed., 2018, 57, 2450 CrossRef CAS PubMed.
- S. Möhle, M. Zirbes, E. Rodrigo, T. Gieshoff, A. Wiebe and S. R. Waldvogel, Angew. Chem., Int. Ed., 2018, 57, 6018 CrossRef.
- C. Gütz, B. Klöckner and S. R. Waldvogel, Org. Process Res. Dev., 2016, 20, 26 CrossRef.
- C. Gütz, A. Stenglein and S. R. Waldvogel, Org. Process Res. Dev., 2017, 21, 771 CrossRef.
- B. Gleede, M. Selt, C. Gütz, A. Stenglein and S. R. Waldvogel, Org. Process Res. Dev., 2020 DOI:10.1021/acs.oprd.9b00451.
-
T. Fuchigami, S. Inagi and M. Atobe, Fundamentals and Applications of Organic Electrochemistry, John Wiley & Sons, Hoboken, 1st edn, 2014 Search PubMed.
-
O. Hammerich and B. Speiser, Organic Electrochemistry, CRC Press, Taylor & Francis Group, Boca Raton, 2016 Search PubMed.
- R. Francke and R. D. Little, Chem. Soc. Rev., 2014, 43, 2492 RSC.
- R. Francke and R. D. Little, ChemElectroChem, 2019, 6, 4373 CrossRef CAS.
- T. H. Meyer, L. H. Finger, P. Gandeepan and L. Ackermann, Trends Chem., 2019, 1, 63 CrossRef.
-
(a)
R. D. Little and M. K. Schwaebe, in Electrochemistry VI Electroorganic Synthesis: Bond Formation at Anode and Cathode, ed. A. Meijere, K. N. Houk, J.-M. Lehn, S. V. Ley, J. Thiem, B. M. Trost, F. Vögtle, H. Yamamoto and E. Steckhan, Springer Berlin Heidelberg, Berlin, Heidelberg, 1997, vol. 185, pp. 1–48 Search PubMed;
(b) A. J. Fry, R. D. Little and J. Leonetti, J. Org. Chem., 1994, 59, 5017 CrossRef CAS.
- M. Huhtasaari, H. J. Schäfer and L. Becking, Angew. Chem., Int. Ed. Engl., 1984, 23, 980 CrossRef.
- J. Mihelcic and K. D. Moeller, J. Am. Chem. Soc., 2004, 126, 9106 CrossRef CAS PubMed.
-
(a) R. Feng, J. A. Smith and K. D. Moeller, Acc. Chem. Res., 2017, 50, 2346 CrossRef CAS PubMed;
(b) D. A. Frey, N. Wu and K. D. Moeller, Tetrahedron Lett., 1996, 37, 8317 CrossRef CAS;
(c) D. A. Frey, J. A. Marx and K. D. Moeller, Electrochim. Acta, 1997, 42, 1967 CrossRef CAS.
-
(a) J.-i. Yoshida, S. Suga, S. Suzuki, N. Kinomura, A. Yamamoto and K. Fujiwara, J. Am. Chem. Soc., 1999, 121, 9546 CrossRef CAS;
(b) J.-i. Yoshida, A. Shimizu and R. Hayashi, Chem. Rev., 2018, 118, 4702 CrossRef CAS PubMed;
(c) J.-i. Yoshida and S. Suga, Chem.–Eur. J., 2002, 8, 2650 CrossRef CAS.
-
(a) R. Franke, D. Selent and A. Börner, Chem. Rev., 2012, 112, 5675 CrossRef CAS PubMed;
(b) F. von Nussbaum, M. Brands, B. Hinzen, S. Weigand and D. Häbich, Angew. Chem., Int. Ed., 2006, 45, 5072 CrossRef CAS PubMed;
(c) K. Okamoto, J. Zhang, J. B. Housekeeper, S. R. Marder and C. K. Luscombe, Macromolecules, 2013, 46, 8059 CrossRef CAS.
-
(a) N. Miyaura, K. Yamada and A. Suzuki, Tetrahedron Lett., 1979, 20, 3437 CrossRef;
(b) M. Grzybowski, K. Skonieczny, H. Butenschön and D. T. Gryko, Angew. Chem., Int. Ed., 2013, 52, 9900 CrossRef CAS PubMed.
-
(a) J. L. Röckl, D. Pollok, R. Franke and S. R. Waldvogel, Acc. Chem. Res., 2020, 53, 45 CrossRef PubMed;
(b) A. Kirste, G. Schnakenburg, F. Stecker, A. Fischer and S. R. Waldvogel, Angew. Chem., Int. Ed., 2010, 49, 971 CrossRef CAS PubMed;
(c) A. Kirste, B. Elsler, G. Schnakenburg and S. R. Waldvogel, J. Am. Chem. Soc., 2012, 134, 3571 CrossRef CAS PubMed.
-
(a) L. Schulz and S. Waldvogel, Synlett, 2019, 30, 275 CrossRef CAS;
(b) B. Elsler, A. Wiebe, D. Schollmeyer, K. M. Dyballa, R. Franke and S. R. Waldvogel, Chem.–Eur. J., 2015, 21, 12321 CrossRef CAS PubMed.
- L. Ackermann, Acc. Chem. Res., 2020, 53, 84 CrossRef CAS PubMed.
-
(a) N. Sauermann, T. H. Meyer, C. Tian and L. Ackermann, J. Am. Chem. Soc., 2017, 139, 18452 CrossRef CAS PubMed;
(b) W.-J. Kong, L. H. Finger, A. M. Messinis, R. Kuniyil, J. C. A. Oliveira and L. Ackermann, J. Am. Chem. Soc., 2019, 141, 17198 CrossRef CAS PubMed;
(c) N. Sauermann, T. H. Meyer, Y. Qiu and L. Ackermann, ACS Catal., 2018, 8, 7086 CrossRef CAS.
- K.-J. Jiao, Y.-K. Xing, Q.-L. Yang, H. Qiu and T.-S. Mei, Acc. Chem. Res., 2020, 53, 300 CrossRef CAS PubMed.
- J. C. Siu, N. Fu and S. Lin, Acc. Chem. Res., 2020, 547 CrossRef CAS PubMed.
- E. Vitaku, D. T. Smith and J. T. Njardarson, J. Med. Chem., 2014, 57, 10257 CrossRef CAS PubMed.
- N. Fu, G. S. Sauer, A. Saha, A. Loo and S. Lin, Science, 2017, 357, 575 CrossRef CAS PubMed.
- S. Herold, S. Möhle, M. Zirbes, F. Richter, H. Nefzger and S. R. Waldvogel, Eur. J. Org. Chem., 2016, 1274 CrossRef CAS.
- T. Morofuji, A. Shimizu and J.-i. Yoshida, J. Am. Chem. Soc., 2013, 135, 5000 CrossRef CAS.
- L. J. Wesenberg, S. Herold, A. Shimizu, J.-i. Yoshida and S. R. Waldvogel, Chem.–Eur. J., 2017, 23, 12096 CrossRef CAS PubMed.
- S. R. Waldvogel and S. Möhle, Angew. Chem., Int. Ed., 2015, 54, 6398 CrossRef CAS PubMed.
- T. Morofuji, A. Shimizu and J.-i. Yoshida, J. Am. Chem. Soc., 2015, 137, 9816 CrossRef CAS PubMed.
- Y. Kawamata, J. C. Vantourout, D. P. Hickey, P. Bai, L. Chen, Q. Hou, W. Qiao, K. Barman, M. A. Edwards, A. F. Garrido-Castro, J. N. deGruyter, H. Nakamura, K. Knouse, C. Qin, K. J. Clay, D. Bao, C. Li, J. T. Starr, C. Garcia-Irizarry, N. Sach, H. S. White, M. Neurock, S. D. Minteer and P. S. Baran, J. Am. Chem. Soc., 2019, 141, 6392 CrossRef CAS PubMed.
- P. Xiong and H.-C. Xu, Acc. Chem. Res., 2019, 52, 3339 CrossRef CAS PubMed.
-
(a) T. Gieshoff, A. Kehl, D. Schollmeyer, K. D. Moeller and S. R. Waldvogel, J. Am. Chem. Soc., 2017, 139, 12317 CrossRef CAS PubMed;
(b) T. Gieshoff, D. Schollmeyer and S. R. Waldvogel, Angew. Chem., Int. Ed., 2016, 55, 9437 CrossRef CAS PubMed;
(c) T. Gieshoff, A. Kehl, D. Schollmeyer, K. D. Moeller and S. R. Waldvogel, Chem. Commun., 2017, 53, 2974 RSC;
(d) A. Kehl, T. Gieshoff, D. Schollmeyer and S. R. Waldvogel, Chem.–Eur. J., 2018, 24, 590 CrossRef CAS PubMed.
- S. Purser, P. R. Moore, S. Swallow and V. Gouverneur, Chem. Soc. Rev., 2008, 37, 320 RSC.
-
(a) J. D. Haupt, M. Berger and S. R. Waldvogel, Org. Lett., 2019, 21, 242 CrossRef CAS PubMed;
(b) J. D. Herszman, M. Berger and S. R. Waldvogel, Org. Lett., 2019, 21, 7893 CrossRef CAS PubMed.
-
(a) T. Fuchigami and S. Inagi, Acc. Chem. Res., 2020, 53, 322 CrossRef CAS PubMed;
(b) A. Konno and T. Fuchigami, J. Org. Chem., 1997, 62, 8579 CrossRef CAS PubMed.
- T. Fuchigami and S. Inagi, Chem. Commun., 2011, 47, 10211 RSC.
- T. Sawamura, K. Takahashi, S. Inagi and T. Fuchigami, Angew. Chem., Int. Ed., 2012, 51, 4413 CrossRef CAS.
-
(a) G. G. Botte, Electrochem. Soc. Interface, 2014, 23, 49 CrossRef;
(b) J. H. Simons, J. Electrochem. Soc., 1949, 95, 47 CrossRef CAS;
(c)
A. J. Fry, in Kirk-Othmer Encyclopedia of Chemical Technology, John Wiley & Sons, Inc, Hoboken, NJ, USA, 2000, vol. 170, p. 114 Search PubMed;
(d) C. A. C. Sequeira and D. M. F. Santos, J. Braz. Chem. Soc., 2009, 20, 387 CrossRef CAS.
-
O. Hammerich and H. Lund, Organic Electrochemistry, M. Dekker, New York, 4th edn, 2001 Search PubMed.
- H. C. Erythropel, J. B. Zimmerman, T. M. de Winter, L. Petitjean, F. Melnikov, C. H. Lam, A. W. Lounsbury, K. E. Mellor, N. Z. Janković, Q. Tu, L. N. Pincus, M. M. Falinski, W. Shi, P. Coish, D. L. Plata and P. T. Anastas, Green Chem., 2018, 20, 1929 RSC.
-
(a) P. Anastas and N. Eghbali, Chem. Soc. Rev., 2010, 39, 301 RSC;
(b) Y. Yuan and A. Lei, Nat. Commun., 2020, 11, 802 CrossRef CAS PubMed.
- K. J. Frankowski, R. Liu, G. L. Milligan, K. D. Moeller and J. Aubé, Angew. Chem., Int. Ed., 2015, 54, 10555 CrossRef CAS PubMed.
- T. Gieshoff, V. Trieu, J. Heijl and S. R. Waldvogel, Beilstein J. Org. Chem., 2018, 14, 1578 CrossRef CAS PubMed.
-
(a) S. Lips and S. R. Waldvogel, ChemElectroChem, 2019, 6, 1649 CrossRef CAS;
(b) S. R. Waldvogel, S. Mentizi and A. Kirste, Top. Curr. Chem., 2012, 320, 1 CAS.
-
(a) J. Xu, Y. Yokota, R. A. Wong, Y. Kim and Y. Einaga, J. Am. Chem. Soc., 2020, 142, 2310 CrossRef CAS PubMed;
(b)
C. A. Martínez-Huitle and S. R. Waldvogel, in Novel Aspects of Diamond, ed. N. Yang, Springer International Publishing, Cham, 2019, vol. 121, pp. 173–197 Search PubMed.
- T. Sumi, T. Saitoh, K. Natsui, T. Yamamoto, M. Atobe, Y. Einaga and S. Nishiyama, Angew. Chem., Int. Ed., 2012, 51, 5443 CrossRef CAS PubMed.
- C. Gütz, M. Selt, M. Bänziger, C. Bucher, C. Römelt, N. Hecken, F. Gallou, T. R. Galvão and S. R. Waldvogel, Chem.–Eur. J., 2015, 21, 13878 CrossRef PubMed.
-
(a) E. J. Horn, B. R. Rosen, Y. Chen, J. Tang, K. Chen, M. D. Eastgate and P. S. Baran, Nature, 2016, 533, 77 CrossRef CAS PubMed;
(b) T. Morofuji, A. Shimizu and J.-i. Yoshida, J. Am. Chem. Soc., 2014, 136, 4496 CrossRef CAS PubMed.
- D. Schmitt, C. Regenbrecht, M. Schubert, D. Schollmeyer and S. R. Waldvogel, Holzforschung, 2017, 71, 35 CAS.
-
(a)
A. Bulan, J. Kintrup and R. Weber, EU Pat., 2398101 A1, 2011;
(b) J. Jörissen, T. Turek and R. Weber, Chem. Unserer Zeit, 2011, 45, 172 CrossRef;
(c) I. Moussallem, J. Jörissen, U. Kunz, S. Pinnow and T. Turek, J. Appl. Electrochem., 2008, 38, 1177 CrossRef CAS.
- H. Salehzadeh, D. Nematollahi and H. Hesari, Green Chem., 2013, 15, 2441 RSC.
- A. Wiebe, B. Riehl, S. Lips, R. Franke and S. R. Waldvogel, Sci. Adv., 2017, 3, eaao3920 CrossRef PubMed.
-
(a) J. G. Ibanez, B. A. Frontana-Uribe and R. Vasquez-Medrano, J. Mex. Chem. Soc., 2016, 60, 247 CAS;
(b) C. A. Paddon, M. Atobe, T. Fuchigami, P. He, P. Watts, S. J. Haswell, G. J. Pritchard, S. D. Bull and F. Marken, J. Appl. Electrochem., 2006, 36, 617 CrossRef CAS;
(c) E. Steckhan, T. Arns, W. R. Heineman, G. Hilt, D. Hoormann, J. Jörissen, L. Kröner, B. Lewall and H. Pütter, Chemosphere, 2001, 43, 63 CrossRef CAS PubMed.
-
(a)
H. Pütter and H. Hannebaum, US Pat., 6063256, 2000;
(b)
G. Kreysa, K.-i. Ota and R. F. Savinell, Encyclopedia of Applied Electrochemistry, Springer, New York, NY, 2014 CrossRef;
(c) R. Matthessen, J. Fransaer, K. Binnemans and D. E. de Vos, Beilstein J. Org. Chem., 2014, 10, 2484 CrossRef PubMed.
- A. F. Jalbout and S. Zhang, Acta Chim. Slov., 2002, 49, 917 CAS.
- K. Park, P. N. Pintauro, M. M. Baizer and K. Nobe, J. Electrochem. Soc., 1985, 132, 1850 CrossRef CAS.
- C.-F. Chou and T.-C. Chou, J. Appl. Electrochem., 2003, 33, 741 CrossRef CAS.
- M. F. Hartmer and S. R. Waldvogel, Chem. Commun., 2015, 51, 16346 RSC.
- M. J. Orella, Y. Román-Leshkov and F. R. Brushett, Curr. Opin. Chem. Eng., 2018, 20, 159 CrossRef.
- A. A. Folgueiras-Amador and T. Wirth, J. Flow Chem., 2017, 7, 94 CrossRef CAS.
-
(a) D. Pletcher, R. A. Green and R. C. D. Brown, Chem. Rev., 2018, 118, 4573 CrossRef CAS PubMed;
(b) M. Elsherbini and T. Wirth, Acc. Chem. Res., 2019, 52, 3287 CrossRef CAS PubMed;
(c) G. Laudadio, W. de Smet, L. Struik, Y. Cao and T. Noël, J. Flow Chem., 2018, 8, 157 CrossRef PubMed.
- T. Noël, Y. Cao and G. Laudadio, Acc. Chem. Res., 2019, 52, 2858 CrossRef PubMed.
-
(a) B. K. Peters, K. X. Rodriguez, S. H. Reisberg, S. B. Beil, D. P. Hickey, Y. Kawamata, M. Collins, J. Starr, L. Chen, S. Udyavara, K. Klunder, T. J. Gorey, S. L. Anderson, M. Neurock, S. D. Minteer and P. S. Baran, Science, 2019, 363, 838 CrossRef CAS PubMed;
(b) A. Shatskiy, H. Lundberg and M. D. Kärkäs, ChemElectroChem, 2019, 6, 4067 CrossRef CAS.
- D. R. Abernethy, A. J. Destefano, T. L. Cecil, K. Zaidi and R. L. Williams, Pharm. Res., 2010, 27, 750 CrossRef CAS PubMed.
- D. Pletcher, Electrochem. Commun., 2018, 88, 1 CrossRef CAS.
-
(a) B. R. Rosen, E. W. Werner, A. G. O’Brien and P. S. Baran, J. Am. Chem. Soc., 2014, 136, 5571 CrossRef CAS PubMed;
(b) J. Barjau, G. Schnakenburg and S. R. Waldvogel, Angew. Chem., Int. Ed., 2011, 50, 1415 CrossRef CAS PubMed.
-
(a) T.-S. Kam, T.-M. Lim and G.-H. Tan, J. Chem. Soc., Perkin Trans. 1, 2001, 1594 RSC;
(b) A. Lipp, D. Ferenc, C. Gütz, M. Geffe, N. Vierengel, D. Schollmeyer, H. J. Schäfer, S. R. Waldvogel and T. Opatz, Angew. Chem., Int. Ed., 2018, 57, 11055 CrossRef CAS PubMed;
(c) A. Lipp, M. Selt, D. Ferenc, D. Schollmeyer, S. R. Waldvogel and T. Opatz, Org. Lett., 2019, 21, 1828 CrossRef CAS PubMed.
-
J. Platzek, K. Gottfried, J. Assmann and G. Lolli, WO Pat., 2017032678 A1, 2017.
- Q. Lin, L. Li and S. Luo, Chem.–Eur. J., 2019, 25, 10033 CrossRef CAS PubMed.
- M. Ghosh, V. S. Shinde and M. Rueping, Beilstein J. Org. Chem., 2019, 15, 2710 CrossRef CAS PubMed.
- X. Chang, Q. Zhang and C. Guo, Angew. Chem., Int. Ed., 2020 DOI:10.1002/anie.202000016.
- B. F. Watkins, J. R. Behling, E. Kariv and L. L. Miller, J. Am. Chem. Soc., 1975, 97, 3549 CrossRef CAS.
-
(a) S. Abe, T. Nonaka and T. Fuchigami, J. Am. Chem. Soc., 1983, 105, 3630 CrossRef CAS;
(b) T. Osa, Y. Kashiwagi, T. Ono, F. Kurashima and U. AKIBA, Int. J. Soc. Mater. Eng. Resour., 2014, 20, 49 CrossRef CAS.
-
(a) S. Assavapanumat, M. Ketkaew, A. Kuhn and C. Wattanakit, J. Am. Chem. Soc., 2019, 141, 18870 CrossRef CAS PubMed;
(b) C. Wattanakit and A. Kuhn, Chem.–Eur. J., 2020, 26, 2993 CrossRef CAS PubMed;
(c) C. Wattanakit, T. Yutthalekha, S. Asssavapanumat, V. Lapeyre and A. Kuhn, Nat. Commun., 2017, 8, 2087 CrossRef PubMed;
(d) T. Yutthalekha, C. Wattanakit, V. Lapeyre, S. Nokbin, C. Warakulwit, J. Limtrakul and A. Kuhn, Nat. Commun., 2016, 7, 12678 CrossRef CAS.
-
(a) Y. Kashiwagi, F. Kurashima, C. Kikuchi, J.-i. Anzai, T. Osa and J. M. Bobbitt, Chem. Commun., 1999, 1983 RSC;
(b) Y. Kashiwagi, F. Kurashima, C. Kikuchi, J.-i. Anzai, T. Osa and J. M. Bobbitt, Tetrahedron Lett., 1999, 40, 6469 CrossRef CAS;
(c) M. Kuroboshi, H. Yoshihisa, M. N. Cortona, Y. Kawakami, Z. Gao and H. Tanaka, Tetrahedron Lett., 2000, 41, 8131 CrossRef CAS.
- W.-C. Gao, Z.-Y. Xiong, S. Pirhaghani and T. Wirth, Synthesis, 2019, 51, 276 CrossRef CAS.
-
(a) N. Fu, L. Li, Q. Yang and S. Luo, Org. Lett., 2017, 19, 2122 CrossRef CAS PubMed;
(b) K. L. Jensen, P. T. Franke, L. T. Nielsen, K. Daasbjerg and K. A. Jørgensen, Angew. Chem., Int. Ed., 2010, 49, 129 CrossRef CAS PubMed.
-
(a) X. Huang, Q. Zhang, J. Lin, K. Harms and E. Meggers, Nat. Catal., 2019, 2, 34 CrossRef CAS;
(b) Q. Zhang, X. Chang, L. Peng and C. Guo, Angew. Chem., Int. Ed., 2019, 58, 6999 CrossRef CAS PubMed.
- F. Hollmann, K. Hofstetter, T. Habicher, B. Hauer and A. Schmid, J. Am. Chem. Soc., 2005, 127, 6540 CrossRef CAS PubMed.
-
(a) S. Kawabata, N. Iwata and H. Yoneyama, Chem. Lett., 2000, 29, 110 CrossRef;
(b) L. Wan, R. S. Heath, B. Siritanaratkul, C. F. Megarity, A. J. Sills, M. P. Thompson, N. J. Turner and F. A. Armstrong, Green Chem., 2019, 21, 4958 RSC.
-
(a) M. Feroci, A. Inesi, M. Orsini and L. Palombi, Org. Lett., 2002, 4, 2617 CrossRef CAS PubMed;
(b) C. Zielinski and H. J. Schäfer, Tetrahedron Lett., 1994, 35, 5621 CrossRef CAS.
-
(a) B. E. Dale, J. Chem. Technol. Biotechnol., 2003, 78, 1093 CrossRef CAS;
(b) N. Chu, Q. Liang, Y. Jiang and R. J. Zeng, Biosens. Bioelectron., 2019, 111922 Search PubMed;
(c) F. Harnisch and U. Schröder, ChemElectroChem, 2019, 6, 4126 CrossRef CAS;
(d) F. Harnisch and C. Urban, Angew. Chem., Int. Ed., 2018, 57, 10016 CrossRef CAS PubMed;
(e) H. Li, H. Guo, Z. Fang, T. M. Aida and R. L. Smith, Green Chem., 2020, 47, 852 Search PubMed.
- G. A. Olah, G. K. S. Prakash and A. Goeppert, J. Am. Chem. Soc., 2011, 133, 12881 CrossRef CAS PubMed.
-
(a) S. Nitopi, E. Bertheussen, S. B. Scott, X. Liu, A. K. Engstfeld, S. Horch, B. Seger, I. E. L. Stephens, K. Chan, C. Hahn, J. K. Nørskov, T. F. Jaramillo and I. Chorkendorff, Chem. Rev., 2019, 119, 7610 CrossRef CAS PubMed;
(b) R. Francke, B. Schille and M. Roemelt, Chem. Rev., 2018, 118, 4631 CrossRef CAS PubMed;
(c) F. P. García de Arquer, C.-T. Dinh, A. Ozden, J. Wicks, C. McCallum, A. R. Kirmani, D.-H. Nam, C. Gabardo, A. Seifitokaldani, X. Wang, Y. C. Li, F. Li, J. Edwards, L. J. Richter, S. J. Thorpe, D. Sinton and E. H. Sargent, Science, 2020, 367, 661 CrossRef PubMed;
(d) M. Wang, K. Torbensen, D. Salvatore, S. Ren, D. Joulié, F. Dumoulin, D. Mendoza, B. Lassalle-Kaiser, U. Işci, C. P. Berlinguette and M. Robert, Nat. Commun., 2019, 10, 3602 CrossRef PubMed;
(e) S. Zhang, Q. Fan, R. Xia and T. J. Meyer, Acc. Chem. Res., 2020, 53, 255 CrossRef CAS PubMed;
(f) A. Tortajada, F. Juliá-Hernández, M. Börjesson, T. Moragas and R. Martin, Angew. Chem., Int. Ed., 2018, 57, 15948 CrossRef CAS PubMed.
- M. Zirbes, D. Schmitt, N. Beiser, D. Pitton, T. Hoffmann and S. R. Waldvogel, ChemElectroChem, 2019, 6, 155 CrossRef CAS.
- M. Zirbes and S. R. Waldvogel, Curr. Opin. Green Sustain. Chem., 2018, 14, 19 CrossRef.
- D. Schmitt, C. Regenbrecht, M. Hartmer, F. Stecker and S. R. Waldvogel, Beilstein J. Org. Chem., 2015, 11, 473 CrossRef CAS PubMed.
- R. Rinaldi, R. Jastrzebski, M. T. Clough, J. Ralph, M. Kennema, P. C. A. Bruijnincx and B. M. Weckhuysen, Angew. Chem., Int. Ed., 2016, 55, 8164 CrossRef CAS PubMed.
-
(a) A. Rahimi, A. Ulbrich, J. J. Coon and S. S. Stahl, Nature, 2014, 515, 249 CrossRef CAS PubMed;
(b) M. Davaritouchaee, W. C. Hiscox, E. Terrell, R. J. Mancini and S. Chen, Green Chem., 2020, 60, 4923 Search PubMed;
(c) J. S. Luterbacher, D. Martin Alonso and J. A. Dumesic, Green Chem., 2014, 16, 4816 RSC.
- M. Rafiee, M. Alherech, S. D. Karlen and S. S. Stahl, J. Am. Chem. Soc., 2019, 141, 15266 CrossRef CAS PubMed.
- C. Zhang and F. Wang, Acc. Chem. Res., 2020, 53, 470 CrossRef CAS PubMed.
- J. Zakzeski, P. C. A. Bruijnincx, A. L. Jongerius and B. M. Weckhuysen, Chem. Rev., 2010, 110, 3552 CrossRef CAS PubMed.
- K. Freudenberg, W. Lautsch and K. Engler, Ber. Dtsch. Chem. Ges. A/B, 1940, 73, 167 CrossRef.
-
(a) P. Parpot, A. P. Bettencourt, A. M. Carvalho and E. M. Belgsir, J. Appl. Electrochem., 2000, 30, 727 CrossRef CAS;
(b) A. Caravaca, W. E. Garcia-Lorefice, S. Gil, A. de Lucas-Consuegra and P. Vernoux, Electrochem. Commun., 2019, 100, 43 CrossRef CAS.
-
(a) M. Tian, J. Wen, D. MacDonald, R. M. Asmussen and A. Chen, Electrochem. Commun., 2010, 12, 527 CrossRef CAS;
(b) M. Zirbes, L. L. Quadri, M. Breiner, A. Stenglein, A. Bomm, W. Schade and S. R. Waldvogel, ACS Sustainable Chem. Eng., 2020, 8, 7300 CrossRef CAS.
- H. Zhu, Y. Chen, T. Qin, L. Wang, Y. Tang, Y. Sun and P. Wan, RSC Adv., 2014, 4, 6232 RSC.
- I. Bosque, G. Magallanes, M. Rigoulet, M. D. Kärkäs and C. R. J. Stephenson, ACS Cent. Sci., 2017, 3, 621 CrossRef CAS PubMed.
- B. H. Nguyen, R. J. Perkins, J. A. Smith and K. D. Moeller, J. Org. Chem., 2015, 80, 11953 CrossRef CAS PubMed.
- A. Bin Kassim, C. L. Rice and A. T. Kuhn, J. Appl. Electrochem., 1981, 11, 261 CrossRef.
- T. Shono, Y. Matsumura, K. Tsubata and J. Takata, Chem. Lett., 1981, 10, 1121 CrossRef.
-
(a) Y. Cao and T. Noël, Org. Process Res. Dev., 2019, 23, 403 CrossRef CAS PubMed;
(b) J. Carneiro and E. Nikolla, Annu. Rev. Chem. Biomol. Eng., 2019, 10, 85 CrossRef CAS PubMed;
(c) Y. Kwon, K. J. P. Schouten, J. C. van der Waal, E. de Jong and M. T. M. Koper, ACS Catal., 2016, 6, 6704 CrossRef CAS;
(d) A. M. Román, J. C. Hasse, J. W. Medlin and A. Holewinski, ACS Catal., 2019, 9, 10305 CrossRef;
(e) Y.-R. Zhang, B.-X. Wang, L. Qin, Q. Li and Y.-M. Fan, Green Chem., 2019, 21, 1108 RSC.
-
(a) S. Lestari, P. Mäki-Arvela, J. Beltramini, G. Q. M. Lu and D. Y. Murzin, ChemSusChem, 2009, 2, 1109 CrossRef CAS PubMed;
(b) T. R. dos Santos, P. Nilges, W. Sauter, F. Harnisch and U. Schröder, RSC Adv., 2015, 5, 26634 RSC;
(c) F. J. Holzhäuser, G. Creusen, G. Moos, M. Dahmen, A. König, J. Artz, S. Palkovits and R. Palkovits, Green Chem., 2019, 21, 2334 RSC;
(d) F. J. Holzhäuser, J. B. Mensah and R. Palkovits, Green Chem., 2020, 22, 286 RSC.
-
(a) H. J. Schäfer, Eur. J. Lipid Sci. Technol., 2012, 114, 2 CrossRef;
(b) G. Creusen, F. J. Holzhäuser, J. Artz, S. Palkovits and R. Palkovits, ACS Sustainable Chem. Eng., 2018, 6, 17108 CrossRef CAS;
(c) H. J. Schäfer, M. Harenbrock, E. Klocke, M. Plate and A. Weiper-Idelmann, Pure Appl. Chem., 2007, 79, 2047 Search PubMed.
- G. H. M. de Kruijff, T. Goschler, L. Derwich, N. Beiser, O. M. Türk and S. R. Waldvogel, ACS Sustainable Chem. Eng., 2019, 7, 10855 CrossRef CAS.
- J.-J. Dai, Y.-B. Huang, C. Fang, Q.-X. Guo and Y. Fu, ChemSusChem, 2012, 5, 617 CrossRef CAS PubMed.
- R. Matthessen, L. Claes, J. Fransaer, K. Binnemans and D. E. de Vos, Eur. J. Org. Chem., 2014, 6649 CrossRef CAS.
-
(a) B. H. R. Suryanto, Y. Wang, R. K. Hocking, W. Adamson and C. Zhao, Nat. Commun., 2019, 10, 5599 CrossRef CAS PubMed;
(b) I. Roger, M. A. Shipman and M. D. Symes, Nat. Rev. Chem., 2017, 1, 928 Search PubMed;
(c) W. Xu, Z. Lu, X. Sun, L. Jiang and X. Duan, Acc. Chem. Res., 2018, 51, 1590 CrossRef CAS PubMed;
(d) B. You and Y. Sun, Acc. Chem. Res., 2018, 51, 1571 CrossRef CAS PubMed.
- S. R. Waldvogel, S. Arndt, D. Weis and K. Donsbach, Angew. Chem., Int. Ed., 2020, 59, 8036 CrossRef PubMed.
- H. Roth, N. Romero and D. Nicewicz, Synlett, 2016, 27, 714 CAS.
-
(a) C. Sandford, M. A. Edwards, K. J. Klunder, D. P. Hickey, M. Li, K. Barman, M. S. Sigman, H. S. White and S. D. Minteer, Chem. Sci., 2019, 10, 6404 RSC;
(b)
F. Scholz, Electroanalytical Methods, Springer Berlin Heidelberg, Berlin, Heidelberg, 2005 CrossRef;
(c)
B. Speiser, in Encyclopedia of Electrochemistry, ed. A. J. Bard, Wiley-VCH Verlag GmbH & Co. KGaA, Weinheim, Germany, 2007, vol. 69, p. 269 Search PubMed;
(d)
W. Vielstich and C. H. Hamann, Elektrochemie, Wiley-VCH, Weinheim, 4th edn, 2005 Search PubMed.
|
This journal is © The Royal Society of Chemistry 2020 |
Click here to see how this site uses Cookies. View our privacy policy here.