DOI:
10.1039/D1NR06127E
(Paper)
Nanoscale, 2021,
13, 19023-19037
Multimodal fluorescently labeled polymer-coated GdF3 nanoparticles inhibit degranulation in mast cells†
Received
17th September 2021
, Accepted 3rd November 2021
First published on 3rd November 2021
Abstract
Multimodal gadolinium fluoride nanoparticles belong to potential contrast agents useful for bimodal optical fluorescence and magnetic resonance imaging. However, the metallic nature of the nanoparticles, similarly to some paramagnetic iron oxides, might induce allergic and anaphylactic reactions in patients after administration. A reduction of these adverse side effects is a priority for the safe application of the nanoparticles. Herein, we prepared paramagnetic poly(4-styrenesulfonic acid-co-maleic acid) (PSSMA)-stabilized GdF3 nanoparticles with surface modified by Atto 488-labeled poly(styrene-grad-2-dimethylaminoethyl acrylate)-block-poly(2-dimethylaminoethyl acrylate) (PSDA-A488) with reactive amino groups for introduction of an additional imaging (luminescence) modality and possible targeting of anticancer drugs. The saturation magnetization of GdF3@PSSMA particles according to SQUID magnetometry reached 157 Am2 kg−1 at 2 K and magnetic field of 7 T. GdF3@PSSMA-PSDA-A488 nanoparticles were well tolerated by human cervical adenocarcinoma (HeLa), mouse bone marrow-derived mast cells (BMMC), and rat basophilic mast cells (RBL-2H3); the particles also affected cell morphology and protein tyrosine phosphorylation in mast cells. Moreover, the nanoparticles interfered with the activation of mast cells by multivalent antigens and inhibited calcium mobilization and cell degranulation. These findings show that the new multimodal GdF3-based nanoparticles possess properties useful for various imaging methods and might minimize mast cell degranulation incurred after future nanoparticle diagnostic administration.
Introduction
Rare-earth fluoride nanoparticles containing 4f electrons have recently attracted a great deal of attention due to their unique electronic, chemical, and optical characteristics and good chemical stability. In particular, lanthanide fluoride particles are finding interesting applications in X-ray therapy or fluorescence and magnetic resonance imaging (MRI).1–4 However, optical microscopy and MRI suffer from some limitations. While in vivo optical fluorescence imaging provides only limited quantitative analysis, its integration with highly sensitive 3D tomographic MRI in one system represents an interesting approach complementing high resolution and accuracy of fluorescence analysis with a high penetration depth of MRI. This enables fluorescence-guided surgery5 and noninvasive imaging of living subjects with high sensitivity and accuracy and good resolution.6
Recently, gadolinium fluorides with various morphologies (shape, particle size, and dispersity) and specific luminescent properties have been synthesized by changing reaction conditions using numerous methods, including thermal decomposition, two-phase liquid–solid approaches, coprecipitation, hydrothermal methods, reverse micelle techniques, and microwave irradiation.7,8 Typically, the morphology and size of nanoparticles were controlled by using different stabilizing agents.4,9,10 This approach produced highly crystalline particles with desirable purity, narrow size distribution without aggregation, and required surface charge, which has a great impact on protein adsorption and prediction of in vivo particle behavior. The advantage of GdF3 nanoparticles consisted in their biocompatibility, having negligible cytotoxicity, which makes them suitable for biomedical applications.11,12 GdF3 nanoparticles provided promising results also due to their good dispersibility in a range of solvents, high mass relaxivity (i.e., short proton relaxation time in MRI), and easy surface modifications enabling to regulate cellular uptake, trafficking, and export, required by a specific biomedical application.13 Nanoparticles coated with biological ligands triggered specific cell signaling responses and enhanced the ability to crosslink cell surface receptors. As an example, anionic bifunctional Eu3+-doped Gd2O3 nanoparticles modified with citric acid or rhodamine-containing paramagnetic gadolinium particles efficiently labeled tumor cells.14 The particles modified with targeting agents were found useful for in vivo trafficking of the metastatic, stem, and immune cells.15 Gd-based particles also induced maturation of dendritic cells that have a pivotal role in host immune defense, such as elimination of foreign pathogens and inhibition of tumorigenesis.16 However, whether the Gd-based nanoparticles can affect activity of mast cells, which play a pivotal role in allergic reactions, anaphylaxis, as well as in immune tolerance, is unknown.
Mast cells are typically located at body-environment interfaces and may be found in close vicinity of blood vessels, peripheral nerves, mucosal membranes, skin, and subcutaneous tissue. Hence, they are one of the first immune cells likely to encounter and respond to a foreign material exposure initiating the inflammatory response.17 Mast cells express plasma membrane-associated high-affinity IgE receptors (FcεRIs), the aggregation of which by multivalent antigen (Ag)-IgE complex triggers activation pathway leading to tyrosine phosphorylation of the FcεRI β- and γ-subunits. This is followed by enhanced activity of Src and Syk tyrosine kinase families and phosphorylation of transmembrane adaptors that coordinate further signal propagation resulting in Ca2+ influx across the plasma membrane. Activation events culminate in the release of preformed granule mediators and de novo synthesis and secretion of bioactive compounds.18 The action of these mediators is clinically observed as immediate and late-phase allergic reactions. Besides that, mast cell activation by FcεRI aggregation is accompanied by changes in cell morphology and enhanced cell adhesion and migration.19 The interaction of metal-based nanoparticles with mast cells is an emerging new field in the investigation of signaling and cellular responses during activation events and the effect of physicochemical properties of the particles on mast cell responses needs to be investigated. For example, ZnO and TiO2 nanoparticles inhibited or stimulated mast cell degranulation after FcεRI crosslinking, respectively.20 The degranulation was also induced by 5 nm Ag nanoparticles, but not by 100 nm ones,21 while 20 nm Ag nanoparticles increased intracellular Ca2+ level and degranulation in a FcεRI-independent manner.22 Functionalized Au nanoparticles blocked IgE-dependent degranulation.23 It was also interesting to note that ionic Gd3+ chelate contrast agents induced the release of histamine from mast cells.24 When free ionic groups were blocked by methylamide, the Gd3+ toxicity was entirely suppressed. Therefore, it is important to examine the response of the immune system on the GdF3 nanoparticles, which can distinctively affect mast cell viability and degranulation.
In the present work, we focused on the design of GdF3 nanoparticles by a facile coprecipitation of gadolinium chloride and NH4F from ethylene glycol with poly(4-styrenesulfonic acid-co-maleic acid) (PSSMA) stabilizer. Fluorophore-labeled poly(styrene-grad-2-dimethylaminoethyl acrylate)-block-poly(2-dimethylaminoethyl acrylate) copolymer was used as a coating that conferred additional functionalities to the particles, especially, luminescence, biocompatibility, and possible capability to deliver drugs. The new multimodal particles were thoroughly characterized in terms of their physicochemical properties and magnetic measurements, in vitro cytotoxicity on cell lines, and interference with activation of mast cells, playing a pivotal role in allergy, inflammation, and innate immunity.
Experimental
Materials
Gadolinium(III) chloride hexahydrate (99.9%), ammonium fluoride, phosphate-buffered saline (PBS), xylenol orange, Triton X-100, HEPES, dinitrophenyl-albumin (DNP-albumin), 4-nitrophenyl N-acetyl-β-D-glucosaminide (4-NAG), 2-ethoxy-1-ethoxycarbonyl-1,2-dihydroquinoline (EEDQ), 4,4′-azobis(4-cyanovaleric acid) (ACVA), N-hydroxysuccinimide (NHS) ester of Atto 488 (denoted as A488) fluorescent dye, piperidine, and N,N-dimethylacetamide (DMAc) were purchased from Sigma-Aldrich (St. Louis, MO, USA). Sodium salt of poly(4-styrenesulfonic acid-co-maleic anhydride) (PSSMA; Mw = 20
000 g mol−1) was obtained from Scientific Polymer Products (Ontario, NY, USA). N-(9-Fluorenylmethyloxycarbonyl)-ethylenediamine hydrochloride (Fmoc-EDA) was purchased from Iris Biotech (Marktredwitz, Germany). Rhodamine phalloidin stain kit, wheat germ agglutinin (WGA) Alexa Fluor 555 conjugate, Hoechst 33342, and FluoroBrite™ DMEM were from Thermo Fisher Scientific (Waltham, MA, USA). Poly(styrene-grad-2-dimethylaminoethyl acrylate)-block-poly(2-dimethylaminoethyl acrylate) [P(S0.5-grad-DMAEA0.5)70-b-PDMAEA50; PSDA] (Scheme 1) was synthesized by nitroxide-mediated radical polymerization.25 Fura-2-acetoxymethyl ester (Fura-2-AM) was purchased from Invitrogen (Carlsbad, CA, USA). For the degranulation, Tyrode's buffer (112 mM NaCl, 2.7 mM KCl, 0.4 mM NaH2PO4, 1.6 mM CaCl2, 1 mM MgCl2, 5.6 mM glucose, 10 mM HEPES, pH 7.4, 0.1% bovine serum albumin) was used. Ethanol, toluene, ethylene glycol (EG), dimethyl sulfoxide (DMSO), and ammonium hydroxide (25%) were obtained from Lachema (Brno, Czech Republic). All chemicals were used directly without further purification. Ultrapure Q-water ultrafiltered on a Milli-Q Gradient A10 system (Millipore; Molsheim, France) was used in all experiments.
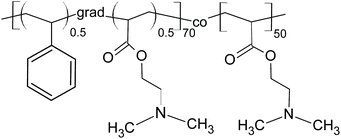 |
| Scheme 1 Poly(styrene-grad-2-dimethylaminoethyl acrylate)-block-poly(2-dimethylaminoethyl acrylate) (PSDA). | |
Labeling of PSDA with A488 fluorescent dye
Synthesis of Atto 488-labeled poly(styrene-grad-2-dimethylaminoethyl acrylate)-block-poly(2-dimethylaminoethyl acrylate) is schematically shown in Scheme 2. Briefly, PSDA (3.78 g; 0.261 mmol) was dissolved in anhydrous toluene (50 ml) at 115 °C with continuous stirring and ACVA (1.46 g; 5.2 mmol) solution in anhydrous DMAc (6 ml) was added in three portions. The reaction mixture was kept at 115 °C for another 3 h, cooled down, and purified by dialysis (MWCO 3500 Da) against water for 72 h. The resulting PSDA-COOH was washed with n-heptane (60 ml) and the aqueous layer was lyophilized. Yield: 2.95 g.
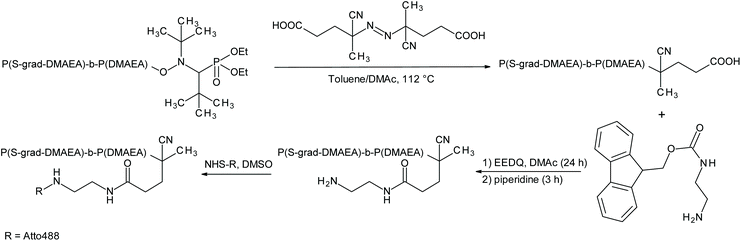 |
| Scheme 2 Synthesis of Atto 488-labeled poly(styrene-grad-2-dimethylaminoethyl acrylate)-block-poly(2-dimethylaminoethyl acrylate). | |
PSDA-COOH (2.83 g) was dissolved in DMAc (20 ml) and the solution of EEDQ (0.48 g; 10 mol excess relative to PSDA-COOH) in DMAc (5 ml) was added at room temperature (RT) with continuous stirring. Separately, Fmoc-EDA (0.18 g; 3.2 mol excess relative to PSDA-COOH) was dissolved in DMAc (5 ml) at 60 °C and added to the PSDA-COOH solution in two portions. The reaction mixture was stirred at RT overnight, heated at 65 °C for 2 h, and resulting PSDA-Fmoc was washed with n-heptane (50 ml), dialyzed against water (pH 3) for 48 h (MWCO 3500 Da), and lyophilized; yield: 2.65 g. PSDA-Fmoc (2.55 g) was dissolved in anhydrous DMAc (20 ml), piperidine (30 ml) was added, and the mixture was stirred at RT for 3 h. The resulting PSDA-NH2 was purified by dialysis (MWCO 3500 Da) against water (pH 4) for 48 h and lyophilized.
Finally, PSDA-NH2 was decorated with A488 fluorescence label. Briefly, PSDA-NH2 (1 g) was dissolved in DMSO (15 ml), NHS ester of A488 (2 mg) was added and the reaction mixture was stirred at RT for 10 h, dialyzed (MWCO 3500 Da) against water for 72 h, and lyophilized. The resulting polymer was denoted as PSDA-A488; yield: 0.59 g. The content of fluorescent label (0.2 wt%) in PSDA-A488 was determined spectrophotometrically at 502 nm.
Synthesis of PSSMA- and EG-stabilized GdF3 nanoparticles
GdF3 nanoparticles were prepared by a coprecipitation method in EG. In a typical procedure, GdCl3·6H2O (5 mmol) and PSSMA (0–15 mg ml−1) were dissolved in EG (20 ml) with magnetic stirring, solution of NH4F (5 mmol) in EG (10 ml) was added dropwise, and the reaction mixture was kept at 75 °C for 3 h with stirring. The solution was cooled to RT, resulting GdF3 precipitate was separated by centrifugation (6000 rpm) for 30 min, washed with water and ethanol several times, and stored in water. Before physicochemical analyses, the particle dispersion was dried at RT for 24 h under vacuum. The PSSMA-stabilized GdF3 nanoparticles and the particles prepared in EG as a solvent and capping agent in the absence of PSSMA were denoted as GdF3@PSSMA and GdF3@EG particles, respectively.
Surface modification of GdF3@PSSMA nanoparticles with PSDA-A488
Aqueous GdF3@PSSMA dispersion (500 μl; 1 mg ml−1) was mixed with aqueous PSDA-A488 solution (1 ml; pH 11) with shaking for 30 min and continuous stirring at RT overnight, which was followed by centrifugation (12
000 rpm) for 10 min. The resulting GdF3@PSSMA-PSDA-A488 particles were washed and resuspended in water to the desired concentration.
Characterization of nanoparticles
Particle morphology was examined by a Philips 200CM transmission electron microscope (TEM). Particle size distribution was obtained by counting several hundreds of particles from the TEM micrographs and by dynamic light scattering (DLS) measurements (Zetasizer NanoZS90; Malvern, UK). While the former method determines the number (Dn)- and weight-average particle diameter (Dw) and dispersity (Đ = Dw/Dn) characterizing the particle size distribution, the latter technique measures the hydrodynamic diameter Dh and polydispersity PD. X-ray diffraction (XRD) patterns of the particles were obtained on a Bruker D8 diffractometer with a scintillation detector and analyzed by the Rietveld method in FullProf program. The mean size of crystallites (DXRD) was evaluated from peak broadening, for which the Thompson–Cox–Hastings pseudo-Voigt profile was applied. The effect of instrumental broadening was determined by measuring a strain-free tungsten powder with the crystallite size of 9.4 μm. Anisotropic size models were based on an expansion of crystallite shape by spherical harmonics consistent with symmetry of respective phases. Fourier-transform infrared (FTIR) spectra in the attenuated total reflection (ATR) mode were obtained with a Thermo Nicolet NEXUS 870 spectrometer (Madison, WI, USA). The magnetic behavior of the particles was investigated in DC field using a Quantum Design MPMS XL superconducting quantum interference device (SQUID; San Diego, CA, USA). Magnetization curves were recorded at 2 and 300 K up to the magnetic field of 7 T. The susceptibility scans were measured with cooling in applied magnetic field B = 0.1 T (H = 79.6 kA m−1). Emission and excitation spectra were recorded on an FS5 Edinburgh Instruments spectrofluorometer (Edinburgh, UK) equipped with both continuous (150 W) and pulsed xenon lamps.
Leaching of Gd3+ ions from GdF3@PSSMA-PSDA-A488 nanoparticles
The leaching of free Gd3+ from the particles was determined by spectrophotometry using xylenol orange as described early.26 Briefly, an aqueous dispersion of GdF3@PSSMA-PSDA-A488 nanoparticles (2 ml; 3.7 mM of Gd3+) was stored at RT for 40 days, the nanoparticles were removed by centrifugation (14
000 rpm) for 30 min, the supernatant (0.15 ml) was mixed with xylenol orange buffer solution (1.5 ml, pH 5.8), and Gd3+ concentration was monitored by a UV-vis Specord 250 Plus UV-Vis spectrophotometer (Analytik Jena; Germany) at 350–650 nm. A calibration curve was obtained using aqueous GdCl3 solutions (0–70 μM Gd3+) and acetate buffer solution (pH 5.8) according to the procedure described earlier.27 The concentration of free Gd3+ was directly proportional to the absorbance ratio at 573 and 433 nm.
Cell cultures and activation
Rat basophilic leukemia cell line RBL-2H3 (cat. no. CRL-2256; RBL), representing a model for mast cell activation,28 and human glioblastoma cell line T98G (cat. no. CRL-1690) were obtained from the American Type Culture Collection (Manassas, VA, USA). Immortalized human retinal pigment epithelial cell line stably expressing telomerase reverse transcriptase hTERT-RPE1 (RPE1) was obtained from Dr. M. Bonhivers (Université Bordeaux; Bordeaux, France). Human cervical adenocarcinoma (HeLa) cells were kindly provided by the Institute of Experimental Medicine, Czech Academy of Sciences, and by Dr. Mělková from the First Faculty of Medicine, Charles University in Prague. A stable cell line of mouse bone marrow-derived mast cells (BMMCs) was donated by Dr. M. Hibbs (Ludwig Institute for Cancer Research, Melbourne, Australia).29 T98G, RPE1, and HeLa cells were maintained in Dulbecco's modified Eagle's medium (DMEM; Lonza; Walkersville, MD, USA) containing 10% fetal bovine serum and antibiotics (100 U ml−1 penicillin and 0.1 mg ml−1 streptomycin). RBL cells were cultivated in 1
:
1 mixture of RPMI 1640 (Sigma-Aldrich) and DMEM media supplemented with serum and antibiotics. BMMCs were cultivated in RPMI 1640 medium supplemented with serum and antibiotics. Cells were grown at 37 °C under 5% CO2 in the air and passaged every 2–3 days. RBL and BMMC cells were specifically activated by antigen (DNP-albumin). Cells were first sensitized with Ab SPE-7 (IgE) to DNP (Sigma-Aldrich, cat. no. D8406; 1 μg ml−1) for 2 h in serum-free DMEM. IgE-sensitized cells were washed and activated with DNP-albumin (30–40 mol DNP per mol albumin; 100 ng ml−1) for 3–60 min in Tyrode's buffer or RPMI 1640 medium without phenol red.
Cytotoxicity assay
HeLa, BMMC, and RBL cells were seeded onto 96-well flat bottom plate at a density 10 × 103 cells per well. After 24 h, an aqueous dispersion of GdF3@PSSMA or GdF3@PSSMA-PSDA-A488 particles (10 μl; 0–100 μg ml−1) was added to cells in complete growth medium (100 μl). The incubation with the nanoparticles continued for 24 h and cell viability was measured using a Presto Blue cell viability reagent (Thermo Fisher Scientific) according to the manufacturer's instructions. Briefly, Presto Blue solution (10 μl) was added to cells at 37 °C for 3.5 h in an incubator with 5% CO2 atmosphere and the fluorescence intensity was measured using a Synergy H1 Hybrid Reader (BioTek Instruments; Winooski, VT, USA) with excitation and emission at 550 and 590 nm, respectively. Data were expressed as the percentage of viable cells in the particle-treated group compared to the untreated group (control).
Degranulation assay and determination of intracellular Ca2+ concentration
The degree of degranulation was quantified as the release of β-hexosaminidase from anti-DNP IgE-sensitized RBL-2H3 or BMMC cells activated with antigen for 30 min using 4-NAG as a substrate.30 The total content of enzyme was evaluated in supernatants from cells lysed by 0.1% Triton X-100. The extent of degranulation was calculated as follows: absorbance of culture supernatant × 100/absorbance of total cell lysate and normalized to control cells. Changes in the level of free intracellular Ca2+ were measured using Fura-2-AM as a cell-permeant calcium reporter following the protocol for sample handling.31 The intracellular free Ca2+ was measured in an Infinite M200 microplate reader (Tecan; Männedorf, Switzerland) as Fura emissions at 510 nm after excitation with 340 and 380 nm lasers at the indicated time points. After the measurement of the Ca2+ basic level, activation was triggered by the addition of antigen.
Gel electrophoresis and immunoblotting
Whole-cell lysates for gel electrophoresis (SDS-PAGE) were prepared as described earlier32 and electrophoresis and immunoblotting were performed using standard protocols.33 For immunoblotting, mouse Ab to phosphor-tyrosine (EMD Millipore; La Jolla, CA, USA; cat. no. 05-1050), rabbit Ab to phosphorylated Syk (human Y525/526 equivalent to rat Y519/520; Cell Signaling; Danvers, MA, USA; cat. no. 2710) and rabbit Ab to actin (Sigma-Aldrich; cat. no. A2066) were diluted 1
:
3000, 1
:
2000, and 1
:
50
000, respectively. Secondary anti-mouse (cat. no. W402B) and anti-rabbit (cat. no. W401B) antibodies conjugated with horseradish peroxidase (HRP; Promega Biotec; Madison, WI, USA) were diluted to 1
:
10
000. The HRP signal was detected with SuperSignal West Pico chemiluminescent reagent (Pierce; Rockford, IL, USA) and a LAS 3000 imaging system (Fujifilm; Düsseldorf, Germany).
Fluorescence microscopy and live cell imaging
The cells were fixed in formaldehyde/Triton X-100 as described earlier.34 Non-adherent BMMCs were attached to fibronectin-coated coverslips. Cells on coverslip were overlaid with nanoparticles in cultivation media. Alternatively, RBL and BMMCs on coverslips were placed down on drop of cultivation media with nanoparticles. F-actin (microfilaments) was visualized using rhodamine phalloidin according to the manufacturer's directions. Samples were embedded in poly(vinyl alcohol) (MOWIOL 4-88; Calbiochem; San Diego, CA, USA) and examined with a Delta Vision Core system (Applied Precision; Issaquah, WA, USA) equipped with a 60×/1.42 NA oil objective. For live cell imaging, RBL-2H3 cells were grown on an 8-well glass-bottomed chamber slide (Ibidi; Gräfelfing, Germany). The cells were then incubated with WGA Alexa Fluor 555 conjugate (5 μg ml−1) in DMEM and Hoechst 33342 (5 μg ml−1) to visualize cellular membranes and DNA, respectively. The cells were then washed with PBS, transferred in FluoroBrite™ DMEM, supplemented with 25 mM HEPES, and cultivated with the nanoparticles (2 mg ml−1) for 3, 5, 15, 30, and 60 min. Images were collected in 70 optical slices (0.1 μm steps and 40 μm pinhole size) with an Andor Dragonfly 503 spinning disc confocal system (Oxford Instruments; Abingdon, UK) equipped with a stage top microscopy incubator, solid-state lasers (405 nm 200 mW, 488 nm 150 mW, and 561 nm 100 mW), HC PL APO 63×/1.20 water objective, and Zyla 4.2 PLUS sCMOS 16-bit camera. For each experiment, at least 5 cells were imaged. The images were deconvoluted with Huygens Professional software v. 20.04 (Scientific Volume Imaging; Hilversum, the Netherlands) and processed with Imaris v. 9.6 visualization software (Oxford Instruments). In 3D-reconstruction, the following colors were applied: red, nanoparticles attached/penetrating the plasma membrane; green, nanoparticles localized into the cytoplasm; grey, plasma membrane; blue, nucleus.
Statistical analysis
The cytotoxicity was expressed as the mean ± standard error mean (S.E.M.) of two independent experiments performed in sextuplicate. One-way ANOVA with Dunnett's post hoc test and two-sided unpaired t-test was used for calculation of statistical significance. The degranulation was expressed as mean ± standard deviation (S.D.). The statistical significance was determined by two-tailed unpaired Student's t-test; * p < 0.05, ** p < 0.01, and *** p < 0.001. Statistical analysis was performed using GraphPad Prism 7.01 software.
Results and discussion
Synthesis and physicochemical characterization of GdF3@EG and GdF3@PSSMA nanoparticles
GdF3 nanoparticles were synthesized in a one-pot reaction from GdCl3·6H2O in EG with PSSMA as a stabilizer. If EG alone was used as a solvent and capping agent, GdF3@EG particles were obtained. Morphology, size, and particle size distribution of GdF3@EG and GdF3@PSSMA nanoparticles were analyzed by TEM (see ESI, Fig. S1† and Table 1). GdF3@EG nanoparticles had various shapes, were polydisperse with size in the 5–40 nm range (Dn = 18 nm; Fig. S1a†), and possessed moderately positive ζ-potential (7 mV). To investigate the effect of stabilizer concentration on the size of GdF3 particles, they were prepared with different amounts of PSSMA (1–15 mg ml−1). The GdF3@PSSMA particles were larger (Dn = 4 nm; Fig. S1b†) and with a broader particle size distribution (Đ = 1.8; Table 1) at a low concentration of PSSMA (1 mg ml−1) than those synthesized at 15 mg of PSSMA per ml (Dn = 3 nm; Fig. S1d†), which possessed a narrower particle size distribution (Đ = 1.1; Table 1), because more nuclei, but smaller in size, could be stabilized with a higher concentration of the stabilizer.35 The stabilization of the particles during their nucleation was prompted by hydroxyl groups of EG and/or sulfonate and carboxyl groups of PSSMA, facilitating its adsorption on the particle surface and preventing additional particle growth and agglomeration. The relatively large size of the GdF3@EG particles can be explained by the limited adsorption of EG on the nuclei surface, which agreed with previously reported data on EG-stabilized nanoparticles.36–38 The hydrodynamic diameter of the GdF3@PSSMA (Dh = 69–110 nm) and GdF3@EG nanoparticles (Dh = 120 nm) indicated a small tendency to aggregation (Table 1). Changes in ζ-potential (from −28 to −45 mV) pointed to the presence of increasing amounts of PSSMA on the particle surface at a higher concentration of the stabilizer in the reaction mixture. The addition of increasing amounts of PSSMA in the reaction system effectively inhibited the growth of GdF3 nanocrystals, which in turn resulted in a smaller size and a narrower particle size distribution. PSSMA was bound to the nanoparticle surface via a strong chelating interaction between carboxyl groups of the polymer and Gd3+ ions. PSSMA polyelectrolyte thus played two crucial roles; it not only provided functionalized nanoparticles with enhanced colloidal stability but also controlled the surface charge density and size of the particles.
Table 1 TEM, DLS and XRD analysis of GdF3-based nanoparticles
Particles |
PSSMA (mg ml−1) |
D
n (nm) |
Đ
|
D
h (nm) |
ζ-Potential (mV) |
D
XRD (nm) |
Crystal structure |
PSSMA – poly(4-styrenesulfonic acid-co-maleic acid); PSDA-A488 – poly(styrene-grad-2-dimethylaminoethyl acrylate)-block-poly(2-dimethylaminoethyl acrylate)-Atto 488; Dn – number-average particle diameter (TEM); Đ – dispersity (TEM); Dh – hydrodynamic diameter (DLS); DXRD – mean particle diameter from X-ray diffraction. Aggregates of tiny particles. |
GdF3@EG |
— |
18 |
1.4 |
120 |
7 |
9 |
Hexagonal |
GdF3@PSSMA |
1 |
4 |
1.8 |
96 |
−28 |
— |
— |
5 |
3 |
2.4 |
110 |
−41 |
— |
— |
15 |
3 |
1.1 |
69 |
−45 |
6 |
Orthorhombic |
GdF3@PSSMA-PSDA-A488 |
15 |
50a |
— |
98 |
−60 |
— |
— |
The crystal structure of EG- and PSSMA-stabilized GdF3 nanoparticles was examined by a powder X-ray diffraction (XRD) analysis (Fig. 1). GdF3@EG particles crystallized in the hexagonal phase described by the P63/mcm space group.39 In contrast, GdF3@PSSMA particles crystallized in the orthorhombic phase and the refined lattice parameters were slightly smaller than those in GdF3@EG nanoparticles. The mean sizes of crystallites calculated from the peak width of primary GdF3@EG and GdF3@PSSMA nanoparticles reached 9 and 6 nm, respectively, confirming a good control of the particle size by using a PSSMA stabilizer. The differences between the sizes of particles according to TEM and those calculated from XRD indicated that EG- and PSSMA-stabilized GdF3 nanoparticles can be combined in doublets or triplets during drying. Magnetic measurements of EG- and PSSMA-stabilized GdF3 nanoparticles showed that they were paramagnetic at RT and no transition to a magnetically ordered state was observed with cooling down to 2 K (Fig. 2a). In the literature, the transition of GdF3 to magnetically ordered state occurred at temperatures as low as 1.25 K,40 although the onset of ferromagnetic interactions between Gd3+ ions generally takes place at <50 K.41 Isothermal magnetization curves of GdF3@EG and GdF3@PSSMA nanoparticles at 2 and 300 K in magnetic fields ≤7 T showed that the particles reached saturation magnetization at <3.5 T (Fig. 2b). The minor variation of the specific magnetization reflected rather the changing content of the fluoride phase and diamagnetic coating; the saturation magnetization of GdF3@EG and GdF3@PSSMA particles at 2 K and magnetic field 7 T reached 168 and 157 Am2 kg−1, respectively. The results indicated that the magnetic behavior did not depend on the distance between the particles and the magnetic interparticle interactions were absent. The data were also comparable to those reported previously for GdF3 nanoparticles,42 which potentially qualifies them for bioimaging and bioseparation.
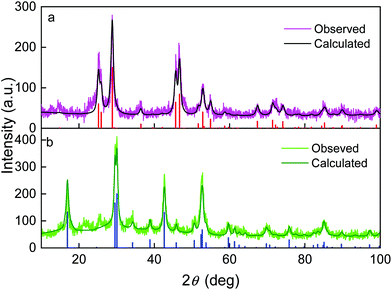 |
| Fig. 1 XRD diffractograms of (a) GdF3@EG and (b) GdF3@PSSMA nanoparticles and comparison with calculated patterns. The vertical lines indicate diffractions of the hexagonal (red) and orthorhombic LnF3 phases (blue). The original data retrieved from Inorganic Crystal Structure Database were based on the P63/mcm of CeF3 no. 42470 and Pnma of EuF3 no. 95244 reflected by calculated patterns no. 01-074-3116 and 01-089-1933 in PDF, respectively. | |
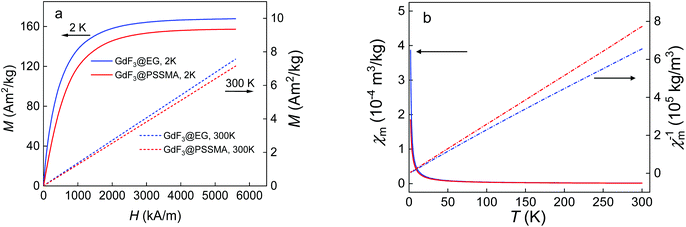 |
| Fig. 2 (a) Magnetization curves of GdF3@EG (blue) and GdF3@PSSMA nanoparticles (red) at 2 (solid line) and 300 K (dot line) and (b) temperature dependence of mass magnetic susceptibility (χm; solid line) and inverse mass magnetic susceptibility of particles (χm−1; dot line) measured at cooling in the applied magnetic field of 0.1 T. | |
The presence of functional groups on the GdF3 nanoparticle surface was determined by ATR-FTIR spectroscopy (Fig. 3). The absorption bands of Gd–F were situated at 160–400 cm−1, while EG and PSSMA generated the C–H stretching (2800–3000 cm−1) and O–H stretching and bending vibrations (∼3600–3000 and 1640–1636 cm−1, respectively). In the fingerprint region, νC–O asymmetric stretching vibrations or bending vibrations of the C–H groups of EG were observed at 1126 or ∼1456 and 725 cm−1, respectively.43,44 In the spectrum of GdF3@PSSMA nanoparticles, the characteristic PSSMA peaks included the C–H bending (1410 cm−1) and C
O stretching vibrations of maleic acid (1720 cm−1), aromatic C
C vibrations of styrene (1636 cm−1), S–O stretching in SO3H (1178 cm−1), antisymmetric and symmetric stretching vibrations in SO3− (1038 and 1010 cm−1, respectively), and C–S stretching in 4-styrenesulfonic acid (638 cm−1).45 The relative absorption intensities from the sulfonic acid almost did not change, which suggests that the majority of sulfonate groups of PSSMA were still present under basic conditions. New peaks at ∼752 and 498 cm−1 were ascribed to the out-of-plane bending vibrations of C–H groups in the monosubstituted aromatic rings46 and Gd–O vibrations.47 In the FTIR spectrum of GdF3@PSSMA nanoparticles, band at 1578 cm−1 corresponding to COOH groups of PSSMA disappeared (Fig. 3b), indicating complete deprotonation of the carboxyl groups and their interaction with the nanoparticle surface. Moreover, intensities of the absorption peaks at ∼1114 and 624 cm−1 ascribed to C–O and Gd–O vibrations,48 respectively, were much higher compared to those of GdF3@EG and PSSMA, confirming the interaction of the carboxyl groups with Gd3+ ions. The characteristic absorption bands in the FTIR spectra of GdF3@EG and GdF3@PSSMA particles thus demonstrated the presence of EG and/or PSSMA coating.
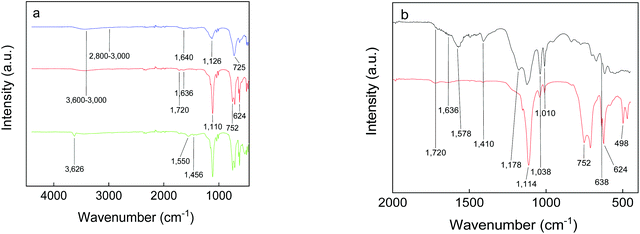 |
| Fig. 3 FTIR spectra of PSSMA (black), GdF3@EG (blue), GdF3@PSSMA (red), and GdF3@PSSMA-PSDA-A488 nanoparticles (green). | |
Functionalization of GdF3@PSSMA nanoparticles with PSDA-A488
To render PSSMA-stabilized GdF3 nanoparticles prepared with 15 mg of PSSMA per ml luminescent, they were modified with fluorescent PSDA-A488 polymer (Mn = 14.5 kDa; polydispersity 1.2). PSDA is a novel pH- and thermo-responsive ion-sensitive amphiphilic diblock copolymer, built of hydrophilic poly(2-dimethylaminoethyl acrylate) (PDMAEA) and amphiphilic poly(styrene-grad-2-dimethylaminoethyl acrylate) [P(S-grad-DMAEA)] blocks (Scheme 1). PDMAEA was widely studied as a drug or gene delivery carrier, because of its low toxicity and high transfection efficiency.49 TEM micrographs of the GdF3@PSSMA-PSDA-A488 nanoparticles showed that they tended to aggregate during the surface modification and a moderately thick (∼9 nm) and uniform PSDA-A488 coating layer was formed around small aggregates (∼50 nm size; Fig. S1e†). Susceptibility of GdF3@PSSMA-PSDA-A488 nanoparticles to aggregation was caused by electrostatic interactions between the positively charged amino groups of PSDA and the negatively charged sulfonate groups of PSSMA. In agreement with TEM data, DLS confirmed the increased hydrodynamic size of the GdF3@PSSMA-PSDA-A488 nanoparticles (98 nm) in water compared to the Dh of GdF3@PSSMA (69 nm) due to the presence of an additional PSDA layer. Functionalization of the particles with PSDA-A488 was also confirmed by their highly negative surface charge (ζ-potential = −60 mV; Table 1) originating from sulfonate groups of A488 dye. The hydrodynamic diameter of GdF3@PSSMA-PSDA-A488 particles was determined also in the cell cultivation media with and without serum and in Tyrode's buffer reaching 100, 283, and 390 nm, respectively; such values are still in the nanoscale range.
Comparison between FTIR spectra of the nanoparticles before and after functionalization with PSDA-A488 demonstrated the successful modification due to the appearance of a peak at 1456 cm−1 ascribed to the N(CH3)2 deformational stretching in the spectrum of the GdF3@PSSMA-PSDA-A488 nanoparticles (Fig. 3a). Additional bands at 3626 and 1550 cm−1 were attributed to N–H stretching and bending vibrations, respectively.
Modification of the GdF3@PSSMA nanoparticles with PSDA-A488 was further corroborated by photoluminescence spectra of GdF3@PSSMA-PSDA-A488 particles and PSDA-A488 (Fig. 4). After the excitation at 507 nm, the intense emission at 522 nm was assigned to A488. A shift of GdF3@PSSMA-PSDA-A488 peak (by 3 nm) from that of free PSDA-A488 was ascribed to the conjugation of PSDA-A488 to the nanoparticles.
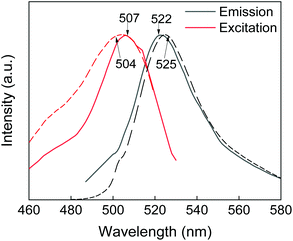 |
| Fig. 4 Photoluminescence spectra of PSDA-A488 (dotted line) and GdF3@PSSMA-PSDA-A488 nanoparticles (solid line). | |
Aqueous GdF3@PSSMA-PSDA-A488 nanoparticle dispersion (3.7 mM of Gd3+) stored at RT for 40 days was also examined for the release of toxic Gd3+ from the particles. A very low concentration of free Gd3+ ions (5.5 μM) was found in the supernatant (Fig. S2†), indicating negligible leaching from the particles and thus non-toxicity of the particles.
Cytotoxicity of GdF3@PSSMA-PSDA-A488 nanoparticles
Effect of modification of GdF3@PSSMA with PSDA-A488 on cell viability of adenocarcinoma cervical HeLa, BMMCs, and RBL mast cells was assessed with resazurin cell viability assay (Fig. 5). In the case of HeLa cells, their viability did not decrease after 24 h of incubation with both types of nanoparticles used in the range of concentrations 0–100 μg ml−1 (Fig. 5a). As a result, both GdF3@PSSMA and GdF3@PSSMA-PSDA-A488 particles can be considered non-toxic for these cells. However, in the case of RBL rat mast cells, GdF3@PSSMA nanoparticles in the highest concentration (100 μg ml−1) were weakly toxic according to international standards50 with the cell viability within 80–60% (p < 0.001; Fig. 5c). In contrast, modification of the GdF3@PSSMA nanoparticles with PSDA-A488 favorably reduced the cytotoxicity of particles and the RBL cell survival was >80%. Such viability is recognized by the International Organization for Standardization as non-toxic.50 Non-cytotoxic behavior of GdF3@PSSMA-PSDA-A488 towards mast cells were also confirmed on another mast cell line, BMMCs (Fig. 5b). GdF3@PSSMA-PSDA-A488 decreased cell viability of BMMCs only by ∼7.5% compared to the control. Moreover, the PSDA-A488 modification, similarly as in RBL cells, slightly reduced the cytotoxicity of GdF3@PSSMA nanoparticles; the viability of cells incubated with GdF3@PSSMA and GdF3@PSSMA-PSDA-A488 increased from 90 to 92.5%. The results thus corroborate findings that the toxicity of free Gd3+ blocked by methylamide or hyaluronic acid is entirely suppressed.24,51
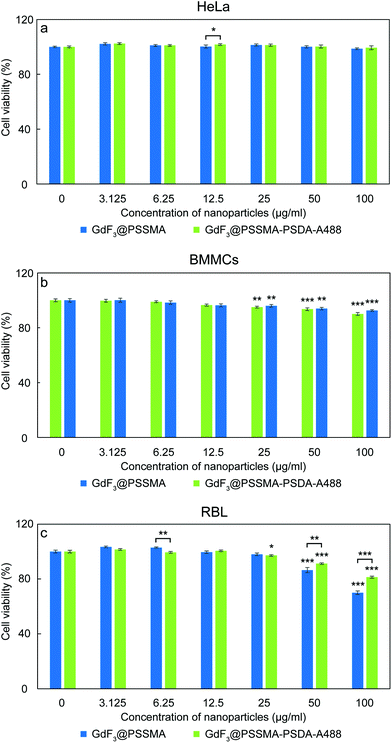 |
| Fig. 5 Viability of (a) cervical adenocarcinoma HeLa, (b) bone marrow-derived mast cells BMMC, and (c) RBL mast cells after 24 h of incubation with GdF3@PSSMA and GdF3@PSSMA-PSDA-A488 nanoparticles. The values represent the mean ± standard error mean (S.E.M.) of two independent experiments performed in sextuplicate; * < 0.05, ** < 0.01, *** < 0.001 indicate statistical significance compared to control (one-way ANOVA with Dunnett's post hoc test) and significant difference in cell viability after exposure to the same concentration of GdF3@PSSMA and GdF3@PSSMA-PSDA-A488 particles (Student t-test). | |
Interference of GdF3@PSSMA-PSDA-A488 nanoparticles with activation of mast cells and their degranulation
Specific activation of mast cells after the crosslinking of FcεRI receptor-bound IgE by multivalent allergens results in morphological changes and the release of mediators of the allergic response. We investigated the effect of GdF3@PSSMA-PSDA-A488 nanoparticles on the morphology of cells of different tissue origin including RBL cells and BMMCs by fluorescence microscopy. Increased cell spreading, delineated by phalloidin staining of F-actin, was detectable in RBL cells incubated with the particles. Morphological changes were detected in BMMCs as well. On the other hand, changes in cell morphology were not found in retinal pigment epithelial cells RPE1 and glioblastoma cells T98G (Fig. 6a). Morphological changes in RBL and BMMCs were observed after overlying cells with medium containing nanoparticles as well as after placing down cells, attached on a coverslip, on drop of medium with nanoparticles (not shown). This ruled out the possibility that changes were due to sinking of particles on cell surface. Prominent remodeling of microfilaments in RBL cells could reflect their activation with a typical temporal increase of overall protein tyrosine phosphorylation (P-Tyr) and activation of Syk kinase.31 It was confirmed by examination of whole-cell lysates from RBL cells by immunoblotting that revealed the increase of P-Tyr and Syk activation, marked by phosphorylation of Syk (P-Syk[Y525/526]) not only in the course of specific cell activation by antigen (see +Ag in Fig. 6a) but also during incubation with the nanoparticles (see +NPs in Fig. 6a). On the other hand, particles did not affect P-Tyr in RPE1 and T98G cells (Fig. 6b). Changes in P-Tyr indicated that the nanoparticles alone could interfere with signaling pathways characteristic for activated RBL cells.
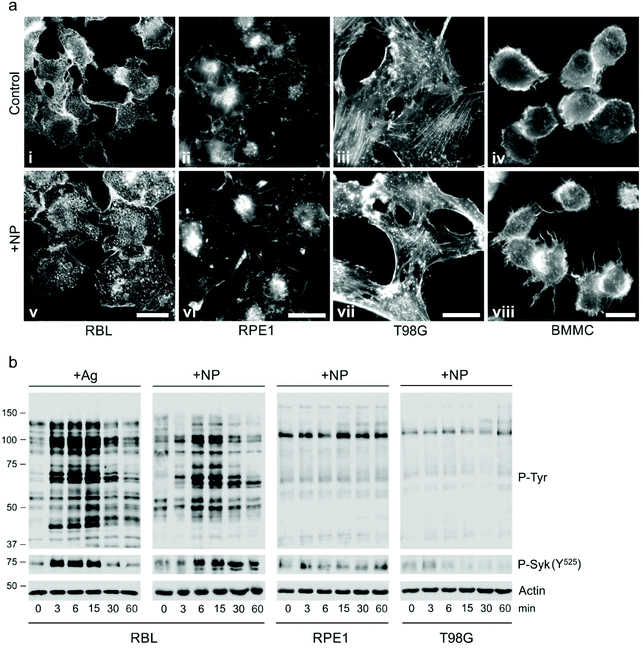 |
| Fig. 6 Effect of GdF3@PSSMA-PSDA-A488 nanoparticles (NPs) on cell morphology and protein tyrosine phosphorylation (P-Tyr) level in various cell lines. (a) Fluorescence micrographs of microfilaments in rat mast cell line RBL, human retinal pigment epithelial cell line RPE1, human glioblastoma cell line T98G, and mouse bone marrow-derived mast cell line BMMC in the absence (control) or presence of nanoparticles. RBL, RPE1, and T98G cells were incubated with the particles (2 mg ml−1) for 15 min, fixed, and stained with rhodamine phalloidin. BMMCs were incubated with nanoparticles (1 mg ml−1) for 5 min before fixation. The images (i and v), (ii and vi), (iii and vii), and (iv and viii) were collected and processed in the same manner. Scale bar (v–vii) 20 and (viii) 10 μm. (b) The immunoblot comparison of the P-Tyr and phosphorylation of Syk kinase (P-Syk[Y525/526]) in whole-cell lysates from RBL, RPE1, and T98G cells incubated with the particles (2 mg ml−1) for 0–60 min. Specific activation of IgE-sensitized (1 μg ml−1) RBL cells with antigen (100 ng ml−1; +Ag) represents the positive control (left). Actin served as the loading control. Representative images from three repetitions. | |
To test whether the elevation of P-Tyr level in RBL cells by GdF3@PSSMA-PSDA-A488 nanoparticles alone can lead to sustained Ca2+ influx across the plasma membrane and to degranulation, the level of intracellular Ca2+ and β-hexosaminidase release was measured in the presence of different concentrations of particles. IgE-sensitized RBL cells activated by multivalent antigen served as a positive control. The Ca2+ influx was recorded only at the highest particle concentration. The Ca2+ level, however, reached only ∼50% of that in control cells and was rapidly attenuated (Fig. 7a). The small increase in Ca2+ was not sufficient to initiate cell degranulation, measured by β-hexosaminidase release (Fig. 7b). These data demonstrated that the GdF3@PSSMA-PSDA-A488 nanoparticles alone were not capable, even at the highest concentration (2 mg ml−1), to induce sustained Ca2+ influx leading to degranulation in RBL cells. Similarly, the nanoparticles alone did not induce degranulation in BMMCs. However, the mechanism for effects of GdF3@PSSMA-PSDA-A488 nanoparticles on mast cells is still unknown and warrants further investigation. On the other hand, concentration-dependent inhibition of Ca2+ mobilization (Fig. 7c) and degranulation (Fig. 7d) were observed in the presence of particles during activation of IgE-sensitized cells by antigen in adherent RBL cells. Similar interfering effect of particles on mast cell activation by FcεRI aggregation was also observed in non-adherent BMMCs that more closely resemble mast cells in situ. This was documented by Ca2+ mobilization (Fig. 8a) and degranulation (Fig. 8b). As the hydrodynamic diameter of particles increased in standard Tyrode's buffer used for the assay, the degranulation was also done in RPMI 1630 medium, where the nanoparticles have hydrodynamic diameter 100 nm. Under these conditions, the nanoparticles also showed inhibitory effects (Fig. S3†). These data suggested that the nanoparticles interfered with signaling cascades in the course of specific mast cell activation. Moreover, the overall P-Tyr level in antigen-activated cells was suppressed in the presence of nanoparticles (data not shown).
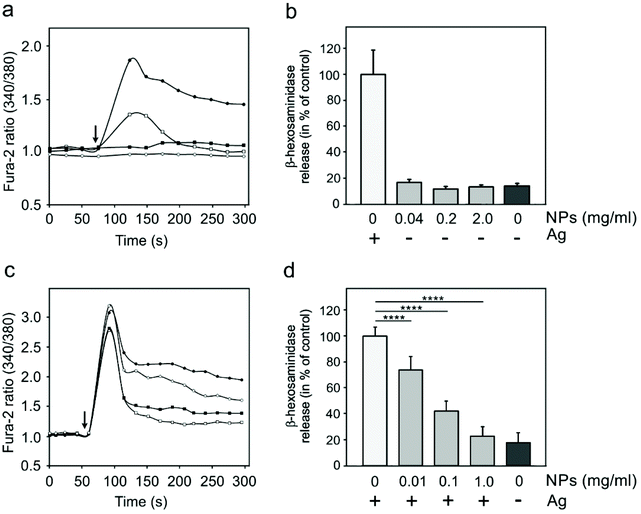 |
| Fig. 7 Effect of GdF3@PSSMA-PSDA-A488 nanoparticles (NPs) on calcium mobilization and degranulation in RBL cells. Calcium mobilization in (a) resting cells with added particles or (c) IgE-sensitized cells with added particles and antigen (Ag). Arrows in (a and c) indicate the time when antigen, nanoparticles alone, or particles with antigen were added. Fura-2-AM served as a cell-permeant calcium reporter. The typical experiments, out of three repetitions, are shown. (●) Positive control, (○) 0.01, (■) 0.1, and (□) 1 mg of particles per ml. Degranulation in (b) resting or (d) IgE-sensitized cells incubated with nanoparticles alone or particles with antigen for 30 min, respectively. Degranulation was measured by β-hexosaminidase release. The data represent the mean ± S.D. (n = 3) from the independent experiments performed in triplicates. Two-tailed unpaired Student's t test was performed to determine statistical significance. Activation of IgE-sensitized (1 μg ml−1) cells by Ag (100 ng ml−1) served in (a–d) as the positive control. | |
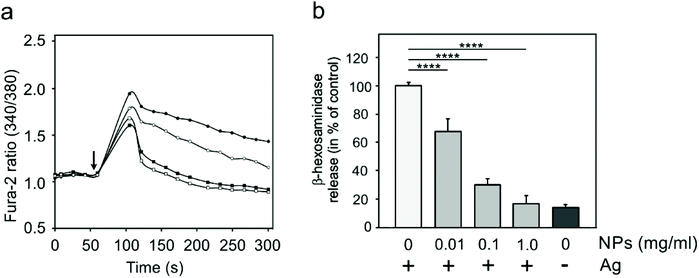 |
| Fig. 8 Effect of GdF3@PSSMA-PSDA-A488 nanoparticles (NPs) on calcium mobilization and degranulation in BMMCs. (a) Calcium mobilization in IgE-sensitized cells with added nanoparticles and antigen (Ag). Arrow indicates the time when antigen or particles with antigen were added. Fura-2-AM served as a cell-permeant calcium reporter. The typical experiment, out of three repetitions, is shown. (●) Positive control, (○) 0.01, (■) 0.1, and (□) 1 mg of particles per ml. (b) Degranulation in IgE-sensitized cells incubated with particles and antigen for 30 min measured by β-hexosaminidase release. The data represent the mean ± S.D. (n = 4) from the independent experiments performed in triplicates. Two-tailed unpaired Student's t test was performed to determine statistical significance. Activation of IgE-sensitized (1 μg ml−1) cells by Ag (100 ng ml−1) served in (a and b) as the positive control. | |
To visualize green fluorescence of nanoparticles by fluorescence microscopy on RBL cells or BMMCs, the cells were overlaid with cultivation media containing nanoparticles at concentration 0.1–2.0 mg ml−1 and incubated for 15 min. After fixation, the preparations were stained for F-actin. While the morphological changes between control and nanoparticle-treated cells were clearly seen in RBL cells (Fig. 9a and b; rhodamine phalloidin staining), only very faint diffuse green fluorescence was detectable (Fig. 9c; fluorescence of nanoparticles). The similar results were obtained for BMMCs. On the other hand, when the cells were incubated with clustered nanoparticles (after more than 40 days of storage at concentration 20 mg ml−1), bright green fluorescence was clearly detectable as shown by live cell imaging (Fig. 9d and e). Particles after 15 min of incubation were associated mainly with the plasma membrane, marked by WGA conjugate with Alexa Fluor 555 (Fig. 9d). After 60 min of incubation, the clustered particles were relocated intracellularly, as demonstrated in 3D cell reconstruction (Fig. 9e), not entering the nuclei. These results were in contrast to the literature, where uptake of 20 nm silver nanoparticles by mast cells was minimal.23 As the GdF3@PSSMA-PSDA-A488 nanoparticles attenuated P-Tyr (data not shown) and Ca2+ concentration 6 min after cell activation by antigen, when the degranulation was detectable, it is supposed that the particle internalization did not contribute to inhibition of degranulation.
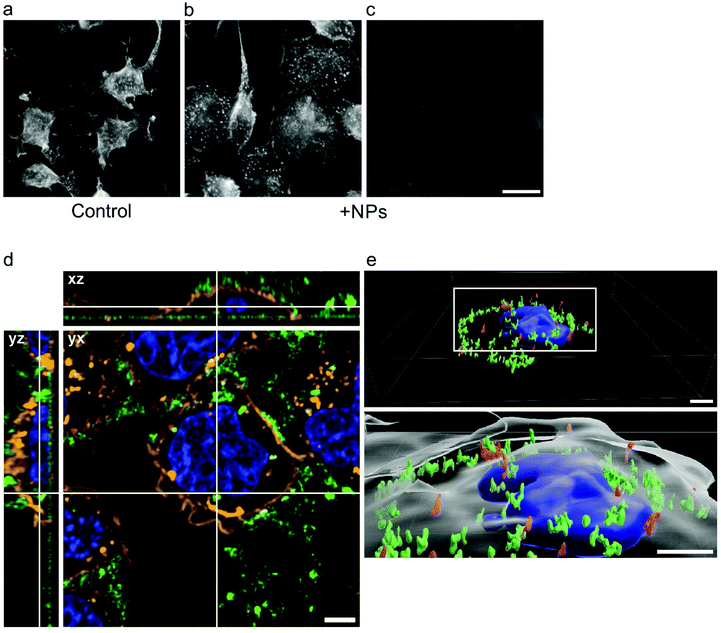 |
| Fig. 9 Distribution of GdF3@PSSMA-PSDA-A488 nanoparticles (NPs) in RBL cells. (a–c) Fluorescence micrographs of (a and b) microfilaments and (c) nanoparticles in the absence (a; control) and presence of nanoparticles (b and c). Cells were incubated with nanoparticles (2 mg ml−1) for 15 min and after the fixation they were stained with rhodamine phalloidin; (b and c) represent the same cells. Scale bar 20 μm. (d) Still image and (e) 3D-reconstruction from live-cell imaging of RBL cells incubated with clustered nanoparticles (2 mg ml−1) for (d) 15 min and (e) 60 min. (d) Nanoparticles (green), Alexa Fluor 555 conjugate of wheat germ agglutinin to mark cell membranes (orange), and Hoechst 33342 to label DNA in nuclei (blue). Top view (yx, single plane) and side views (xz and yz) from a z-stack. (e) 3D-reconstruction in the upper panel shows the nanoparticles attached/penetrating the plasma membrane (red) and localized into the cytoplasm (green) but not in the nucleus (blue). The boxed area is enlarged in the lower panel, where the plasma membrane is also depicted (grey). Scale bars 5 μm. | |
The obtained data suggest that the GdF3@PSSMA-PSDA-A488 nanoparticles bind to the RBL cell surface, interfere with signaling pathways initiated by crosslinking of FcεRIs with multivalent antigen, and suppress sustained Ca2+ mobilization. This results in the inhibition of mast cell degranulation, which usually initiates allergic and anaphylactic reactions reported in the case of carbon nanotubes, dendrimers, positively charged silica, and quantum dots.52–54 The allergic reactions associated with exposure to contrast agent are known to be also induced in some patients, e.g., by superparamagnetic iron oxide nanoparticles, which were approved and later declined by FDA and EMA for application as an in vivo contrast agent.55,56 In this respect, GdF3@PSSMA-PSDA-A488 nanoparticles might minimize mast cell degranulation and allergic responses. Inherent properties of the paramagnetic and luminescent GdF3@PSSMA-PSDA-A488 nanoparticles, especially their ability to suppress mast cell degranulation, indicate that they may have therapeutic potential for the treatment of allergies and multimodal imaging.
Conclusions
GdF3@PSSMA-PSDA-A488 nanoparticles represent a new platform for the construction of contrast agents with multiple imaging modalities and/or targeting capabilities. The morphology of particles (in particular, size) and crystallinity can be controlled by the reaction conditions involving steric stabilization with EG and/or PSSMA at different concentrations. The GdF3-based core is paramagnetic with high longitudinal relaxivity in MRI, while PSSMA-PSDA-A488 shell confers luminescent characteristics on the nanoparticles, making them useful for optical fluorescence imaging. The combination of magnetic and fluorescent properties of GdF3@PSSMA-PSDA-A488 nanoparticles can thus allow their applications in dual-mode imaging. The presence of amino groups of PSDA-A488 on the particle surface will make them accessible for additional conjugation with anticancer drugs, monoclonal antibodies, and/or photosensitizers that are important for the potential development of specific theranostic agents for multimodal bioimaging and drug delivery. GdF3@PSSMA-PSDA-A488 nanoparticles are biocompatible and non-toxic towards adenocarcinoma HeLa, BMMCs, and RBL mast cells. Besides, the nanoparticles inhibit degranulation of RBL mast cells, probably due to the prevention of Ca2+ mobilization, which might favorably suppress allergic reactions after prospective in vivo administration improving immune tolerance of the nanoparticles. All these properties make multimodal GdF3@PSSMA-PSDA-A488 nanoparticles safer and promising diagnostic tools useful for various applications, e.g., monitoring of the biodistribution, blood circulation, and accumulation of drugs in the treatment of various severe disorders. As the GdF3@PSSMA-PSDA-A488 nanoparticles suppress mast cell degranulation, they might minimize potential allergic reactions. They could be also suitable for investigations of signaling pathways during mast cell activation and the development of new strategies for the treatment of allergic diseases.
Conflicts of interest
There are no conflicts to declare.
Acknowledgements
Support of the Czech Science Foundation (no. 19-00676S and 18-27197S), Light Microscopy Service Laboratory (LM2018129 from MEYS), and Institutional Research Support (RVO 68378050) are acknowledged. We thank Dr. M. Hibbs (Ludwig Institute for Cancer Research, Melbourne, Australia) for the BMMC cell line and Dr. M. Bonhivers (Université Bordeaux, Bordeaux, France) for RPE1 cells.
Notes and references
- D. Bekah, D. Cooper, K. Kudinov, C. Hillc, J. Seuntjens, S. Bradforth and J. Nadeau, Synthesis and characterization of biologically stable, doped LaF3 nanoparticles co-conjugated to PEG and photosensitizers, J. Photochem. Photobiol. A, 2016, 329, 26–34 CrossRef CAS
.
- X. Zhu, J. Zhang, J. Liu and Y. Zhang, Recent progress of rare-earth doped upconversion nanoparticles: Synthesis, optimization and applications, Adv. Sci., 2019, 6, 1901358 CrossRef CAS PubMed
.
- D. M. Samhadaneh, G. A. Mandl, Z. Han, M. Mahjoob, S. C. Weber, M. Tuznik, D. A. Rudko, J. A. Capobianco and U. Stochaj, Evaluation of lanthanide-doped upconverting nanoparticles for in vitro and in vivo applications, ACS Appl. Bio Mater., 2020, 3, 4358–4369 CrossRef CAS
.
- E. N. M. Cheung, R. D. A. Alvares, W. Oakden, R. Chaudhary, M. L. Hill, J. Pichaandi, G. C. H. Mo, C. Yip, P. M. Macdonald, G. J. Stanisz, F. C. J. M. van Veggel and R. S. Prosser, Polymer-stabilized lanthanide fluoride nanoparticle aggregates as contrast agents for magnetic resonance imaging and computed tomography, Chem. Mater., 2010, 22, 4728–4739 CrossRef CAS
.
- Y. Wang, R. Song, K. Guo, Q. Meng, R. Zhang, X. Kong and Z. Zhang, A gadolinium(III) complex based dual-modal probe for MRI and fluorescence sensing of fluoride ions in aqueous medium and in vivo, Dalton Trans., 2016, 45, 17616–17623 RSC
.
- W. Mnasri, M. Parvizian and S. Ammar-Merah, Design and synthesis of luminescent lanthanide-based bimodal nanoprobes for dual magnetic resonance (MR) and optical imaging, Nanomaterials, 2021, 11, 354 CrossRef CAS PubMed
.
- R. K. Sharma, A. V. Mudring and P. Ghosh, Recent trends in binary and ternary rare-earth fluoride nanophosphors: How structural and physical properties influence optical behavior, J. Lumin., 2017, 189, 44–63 CrossRef CAS
.
- N. Halttunen, F. Lerouge, F. Chaput, M. Vandamme, S. Karpati, S. Si-Mohamed, M. Sigovan, L. Boussel, E. Chereul, P. Douek and S. Parola, Hybrid Nano-GdF3 contrast media allows pre-clinical in vivo element-specific K-edge imaging and quantification, Sci. Rep., 2019, 9, 12090 CrossRef PubMed
.
- T. Passuello, M. Pedroni, F. Piccinelli, S. Polizzi, P. Marzola, S. Tambalo, G. Conti, D. Benati, F. Vetrone, M. Bettinelli and A. Speghini, PEG-capped, lanthanide doped GdF3 nanoparticles: Luminescent and T2 contrast agents for optical and MRI multimodal imaging, Nanoscale, 2012, 4, 7682–7689 RSC
.
- S. Morsy, Role of surfactants in nanotechnology and their applications, Int. J. Curr. Microbiol. Appl. Sci., 2014, 3, 237–260 Search PubMed
.
- S. Rodriguez-Liviano, N. O. Nunez, S. Rivera-Fernandez, J. M. de la Fuente and M. Ocana, Ionic liquid mediated synthesis and surface modification of multifunctional mesoporous Eu:GdF3 nanoparticles for biomedical applications, Langmuir, 2013, 29, 3411–3418 CrossRef CAS PubMed
.
- M. Runowski and S. Lis, Nanocrystalline rare earth fluorides doped with Pr3+ ions, J. Rare Earths, 2016, 34, 802–807 CrossRef CAS
.
- F. Evanics, P. R. Diamente, F. C. J. M. van Veggel, G. J. Stanisz and R. S. Prosser, Water-soluble GdF3 and GdF3/LaF3 nanoparticles - physical characterization and NMR relaxation properties, Chem. Mater., 2006, 18, 2499–2505 CrossRef CAS
.
- Z. Shi, K. G. Neoh, E. T. Kang, B. Shuter and S.-C. Wang, Bifunctional Eu3+-doped Gd2O3 nanoparticles as a luminescent and T1 contrast agent for stem cell labeling, Contrast Media Mol. Imaging, 2010, 5, 105–111 CAS
.
- K. Vuu, J. Xie, M. A. McDonald, M. Bernardo, F. Hunter, Y. Zhang, K. Li, M. Bednarski and S. Guccione, Gadolinium-rhodamine nanoparticles for cell labeling and tracking via magnetic resonance and optical imaging, Bioconjugate Chem., 2005, 16, 995–999 CrossRef CAS PubMed
.
- D. Yang, Y. Zhao, H. Guo, Y. Li, P. Tewary, G. Xing, W. Hou, J. J. Oppenheim and N. Zhang, [Gd@C(82)(OH)(22)](n) nanoparticles induce dendritic cell maturation and activate Th1 immune responses, ACS Nano, 2010, 4, 1178–1186 CrossRef CAS PubMed
.
- E. Passante, C. Ehrhardt, H. Sheridan and N. Frankish, RBL-2H3 cells are an imprecise model for mast cell mediator release, Inflammation Res., 2009, 58, 611–618 CrossRef CAS PubMed
.
- J. Kalesnikoff and S. J. Galli, New developments in mast cell biology, Nat. Immunol., 2008, 9, 1215–1223 CrossRef CAS PubMed
.
- P. Dráber, V. Sulimenko and E. Dráberová, Cytoskeleton in mast cell signaling, Front. Immunol., 2012, 3, e130 Search PubMed
.
- B. N. Feltis, A. Elbaz, P. F. Wright, G. A. Mackay, T. W. Turney and A. L. Lopata, Characterizing the inhibitory action of zinc oxide nanoparticles on allergic-type mast cell activation, Mol. Immunol., 2015, 66, 139–146 CrossRef CAS PubMed
.
- H. Kang, S. Kim, K. H. Lee, S. Jin, S. H. Kim, K. Lee, H. Jeon, Y. G. Song, S. W. Lee, J. Seo, S. Park and I. H. Choi, 5 nm silver nanoparticles amplify clinical features of atopic dermatitis in mice by activating mast cells, Small, 2017, 13, 1602363 CrossRef PubMed
.
- N. B. Alsaleh, I. Persaud and J. M. Brown, Silver nanoparticle-directed mast cell degranulation is mediated through calcium and PI3K signaling independent of the high affinity IgE preceptor, PLoS One, 2016, 11, e0167366 CrossRef PubMed
.
- I. M. Yasinska, L. Calzolai, U. Raap, R. Hussain, G. Siligardi, V. V. Sumbayev and B. F. Gibbs, Targeting of basophil and mast cell pro-allergic reactivity using functionalised gold nanoparticles, Front. Pharmacol., 2019, 10, e333 CrossRef PubMed
.
- T. Kun and L. Jakubowski, Influence of MRI contrast media on histamine release from mast cells, Pol. J. Radiol., 2012, 77, 19–24 CrossRef PubMed
.
- M. Rabyk, A. Destephen, A. Lapp, S. King, L. Noirez, L. Billon, M. Hruby, O. Borisov, P. Stepanek and E. Deniau, Interplay of thermosensitivity and pH sensitivity of amphiphilic block-gradient copolymers of dimethylaminoethyl acrylate and styrene, Macromolecules, 2018, 51, 5219–5233 CrossRef CAS
.
- H. Hifumi, S. Yamaoka, A. Tanimoto, T. Akatsu, Y. Shindo, A. Honda, D. Citterio, K. Oka, S. Kuribayashi and K. Suzuki, Dextran coated gadolinium phosphate nanoparticles for magnetic resonance tumor imaging, J. Mater. Chem., 2009, 19, 6393–6399 RSC
.
- A. Barge, G. Cravotto, E. Gianolio and F. Fedeli, How to determine free Gd and free ligand in solution of Gd chelates. A technical note, Contrast Media Mol. Imaging, 2006, 1, 184–188 CrossRef PubMed
.
- W. Alelwani, R. A. Alharbi, D. Wan, D. Vllasaliu, F. H. Falcone and S. Stolnik, Use of engineered nanoparticles (ENPs) for the study of high-affinity IgE FcεRI receptor engagement and rat basophilic leukemia (RBL) cell degranulation, Basophils and Mast Cells, Methods in Molecular Biology, 2020, 2163 CAS
.
- M. L. Hibbs, D. M. Tarlinton, J. Armes, D. Grail, G. Hodgson, R. Maglitto, S. A. Stacker and A. R. R. Dunn, Multiple defects in the immune-system of Lyn-deficient mice, culminating in autoimmune-disease, Cell, 1995, 83, 301–311 CrossRef CAS PubMed
.
- K. Nishida, S. Yamasaki, Y. Ito, K. Kabu, K. Hattori, T. Tezuka, H. Nishizumi, D. Kitamura, R. Goitsuka, R. S. Geha, T. Yamamoto, T. Yagi and T. Hirano, FcεRI-mediated mast cell degranulation requires calcium-independent microtubule-dependent translocation of granules to the plasma membrane, J. Cell Biol., 2005, 170, 115–126 CrossRef CAS PubMed
.
- Z. Hájková, V. Bugajev, E. Dráberová, S. Vinopal, L. Dráberová, J. Janáček, P. Dráber and P. Dráber, STIM1-directed reorganization of microtubules in activated cells, J. Immunol., 2011, 186, 913–923 CrossRef PubMed
.
- A. Klebanovych, V. Sládková, T. Sulimenko, V. Vosecká, Z. Rubíková, M. Čapek, E. Dráberová, P. Dráber and V. Sulimenko, Regulation of microtubule nucleation in mouse bone marrow-derived mast cells by protein tyrosine kinase SHP-1, Cells, 2019, 8, e345 CrossRef PubMed
.
- V. Caracciolo, L. D'Agostino, E. Dráberová, V. Sládková, C. Crozier-Fitzgerald, D. P. Agamanolis, J. P. de Chadarevian, A. Legido, A. Giordano, P. Dráber and C. D. Katsetos, Differential expression and cellular distribution of ν-tubulin and βIII-tubulin in medulloblastomas and human medulloblastoma cell lines, J. Cell Physiol., 2010, 223, 519–529 CAS
.
- E. Dráberová and P. Dráber, A microtubule-interacting protein involved in coalignment of vimentin intermediate filaments with microtubules, J. Cell Sci., 1993, 106, 1263–1273 CrossRef
.
- S. Shen, E. D. Sudol and M. S. El-Aasser, Dispersion polymerization of methyl methacrylate: Mechanism of particle formation, J. Polym. Sci., Part A: Polym. Chem., 1994, 32, 1087–1100 CrossRef CAS
.
- Q. Zhao, B. Shao, W. Lü, Y. Jia, W. Lv, M. Jiao and H. You, Ba2GdF7 nanocrystals: Solution-based synthesis, growth mechanism, and luminescence properties, Cryst. Growth Des., 2014, 14, 1819–1826 CrossRef CAS
.
- J. Xu, S. Gai, P. Ma, Y. Dai, G. Yang, F. He and P. Yang, Gadolinium fluoride mesoporous microspheres: Controllable synthesis, materials and biological properties, J. Mater. Chem. B, 2014, 2, 1791–1801 RSC
.
- F. N. Sayed, V. Grover, V. Sudarsan, B. N. Pandey, A. Asthana, R. K. Vatsa and A. K. Tyagi, Multicolored and white-light phosphors based on doped GdF3 nanoparticles and their potential bio-applications, J. Colloid Interface Sci., 2012, 367, 161–170 CrossRef CAS PubMed
.
- M. L. Afanasiev, S. P. Habuda and A. G. Lundin, The symmetry and basic structures of LaF3, CeF3, PrF3 and NdF3, Acta Crystallogr., Sect. B: Struct. Crystallogr. Cryst. Chem., 1972, 28, 2903–2905 CrossRef
.
- T. E. Katila, V. K. Typpi, G. K. Shenoy and L. Niinisto, Mössbauer studies of Gd3+ compounds at very low temperatures, Solid State Commun., 1972, 11, 1147–1150 CrossRef CAS
.
- Y. C. Chen, J. Prokleska, W. J. Xu, J. L. Liu, J. Liu, W. X. Zhang, J. H. Jia, V. Sechovsky and M. L. Tong, A brilliant cryogenic magnetic coolant: Magnetic and magnetocaloric study of ferromagnetically coupled GdF3, J. Mater. Chem. C, 2015, 3, 12206–12211 RSC
.
- T. Grzyb, L. Mrówczyńska, A. Szczeszak, Z. Śniadecki, M. Runowski, B. Idzikowski and S. Lis, Synthesis, characterization, and cytotoxicity in human erythrocytes of multifunctional, magnetic, and luminescent nanocrystalline rare earth fluorides, J. Nanopart. Res., 2015, 17, 399 CrossRef PubMed
.
-
L. G. Wade Jr., Organic Chemistry, Prentice Hall, New York, 4th edn, 1999, pp. 1208–1213 Search PubMed
.
- R. S. Oliveira, B. S. Brito, J. Kulesza, S. Alves Jr. and B. S. Barros, Tunable photoluminescence of nanostructured LaPO4:Eu3+/Tb3+ synthesized via a microwave-assisted ethylene glycol route, Ceram. Int., 2017, 43, 8276–8283 CrossRef
.
- D. S. Kim, M. D. Guiver and S. Y. Nam, Preparation of ion exchange membranes for fuel cell based on crosslinked poly(vinyl alcohol) with poly(styrene sulfonic acid-co-maleic acid), J. Membr. Sci., 2006, 281, 156–162 CrossRef CAS
.
- J. C. Yang, M. J. Jablonsky and J. W. Mays, NMR and FTIR studies of sulfonated styrene-based homopolymers and copolymers, Polymer, 2002, 43, 5125–5132 CrossRef CAS
.
- D. Stefanakis and D. F. Ghanotakis, Synthesis and characterization of gadolinium nanostructured materials with potential applications in magnetic resonance imaging, neutron-capture therapy and targeted drug delivery, J. Nanopart. Res., 2010, 12, 1285–1297 CrossRef CAS
.
-
V. V. Korolev, T. N. Lomova, D. V. Korolev, A. G. Ramazanova, E. G. Mozhzhukhina and E. N. Ovchenkova, New nanoscaled paramagnetic complexes, in Advanced Environmental Analysis: Application of Nanomaterials, ed. C. M. Hussain and B. Kharisov, Royal Society of Chemistry, Cambridge, 2016, vol. 2, pp. 14–47 Search PubMed
.
- Y. Yang, F. Mo, Y. Chen, Y. Liu, S. Chen and J. Zuo, Preparation of 2-(dimethylamino)ethyl methacrylate copolymer micelles for shape memory materials, J. Appl. Polym. Sci., 2015, 132, 42312–42319 Search PubMed
.
- ISO 10993-5:2009 Biological evaluation of medical devices. Part 5: Tests for in vitro cytotoxicity, International organization for standardization; Geneva, Switzerland, 2009.
- M. Russo, P. Bevilacqua, P. A. Netti and E. Torino, A microfluidic platform to design crosslinked hyaluronic acid nanoparticles (cHANPs) for enhanced MRI, Sci. Rep., 2016, 6, 1–10 CrossRef PubMed
.
- U. C. Nygaard, J. S. Hansen, M. Samuelsen, T. Alberg, C. D. Marioara and M. Lovik, Single-walled and multi-walled carbon nanotubes promote allergic immune responses in mice, Toxicol. Sci., 2009, 109, 113–123 CrossRef CAS PubMed
.
- T. Toyama, H. Matsuda, I. Ishida, M. Tani, S. Kitaba, S. Sano and I. Katayama, A case of toxic epidermal necrolysis-like dermatitis evolving from contact dermatitis of the hands associated with exposure to dendrimers, Contact Dermatitis, 2008, 59, 122–123 CrossRef CAS PubMed
.
- S. Jatana, B. C. Palmer, S. J. Phelan and L. A. DeLouise, Immunomodulatory effects of nanoparticles on skin allergy, Sci. Rep., 2017, 7, 3979 CrossRef PubMed
.
- V. M. Runge, Safety of approved MR contrast media for intravenous injection, J. Magn. Reson. Imaging, 2000, 12, 205–213 CrossRef CAS
.
- D. J. Korchinski, M. Taha, R. Yang, N. Nathoo and J. F. Dunn, Iron oxide as an MRI contrast agent for cell tracking, Magn. Reson. Insights, 2015, 8, 15–29 Search PubMed
.
Footnote |
† Electronic supplementary information (ESI) available. See DOI: 10.1039/d1nr06127e |
|
This journal is © The Royal Society of Chemistry 2021 |
Click here to see how this site uses Cookies. View our privacy policy here.