DOI:
10.1039/D1RA04283A
(Review Article)
RSC Adv., 2021,
11, 27950-27964
Target identification of anticancer natural products using a chemical proteomics approach
Received
2nd June 2021
, Accepted 26th July 2021
First published on 18th August 2021
Abstract
In recent years, there has been a strong demand worldwide for the identification and development of potential anticancer drugs based on natural products. Natural products have been explored for their diverse biological and therapeutic applications from ancient time. In order to enhance the efficacy and selectivity and to minimize the undesired side effects of anti cancer natural products (ANPs), it is essential to understand their target proteins and their mechanistic pathway. Chemical proteomics is one of the most powerful tools to connect ANP target identification and quantification where labeling and non-labeling based approaches have been used. Herein, we have discussed the various strategies to systemically develop selective ANP based chemical probes to characterise their specific and non-specific target proteins using a chemical proteomic approach in various cancer cell lysates.
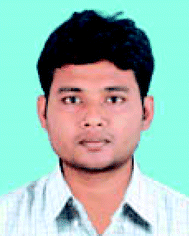 Swadhapriya Bhukta | Swadhapriya Bhukta was born in West Bengal, India, in 1993. He obtained his MSc degree in chemistry from the Indian Institute of Technology, Mandi, Himachal Pradesh. After two years at IIT Mandi he joined TCG Lifescience Ltd (Research Organization) in 2017. He decided to pursue his Doctorate studies in the area of Chemical Biology. Currently, he is a PhD research scholar at the Institute of Chemical Technology Mumbai-IOC Bhubaneswar under the supervision of Dr Rambabu Dandela. His research focuses on the development of new chemical entities to investigate the action of natural products in cancer cells, and he is currently funded by the Institute of Chemical Technology, Mumbai. |
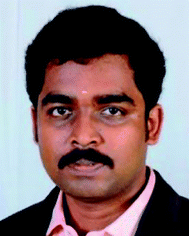 Pushparathinam Gopinath | Dr P. Gopinath received his PhD from the Indian Institute of Technology-Madras under the supervision of Prof. K. M. Muraleedharan in 2013. He accepted his first postdoctoral position in the Herbert Waldmann group (with Dr Kamal Kumar) at the Max-Planck Institute of Molecular Physiology, Germany. After two more postdoctoral positions at the Technion-Israel Institute of technology, Israel (Prof. Ashraf Brik) and Ecole polytechnique federale de Lausanne (EPFL), Switzerland (with Prof. Hilal Lashuel) he accepted an Assistant professor position at SRM Institute of Science and Technology-Chennai in December 2018. His current research interests include synthesis/semisynthesis of natural product analogs and exploring their various biological properties including anticancer activity. |
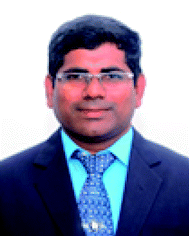 Rambabu Dandela | Dr Rambabu Dandela was born in Telangana, India, in 1981. He obtained his PhD. from Dr Reddy's Institute of Life Sciences, University of Hyderabad campus, in 2013. After postdoctoral studies in Israel with Prof. Michael M. Meijler at Ben-Gurion University of the Negev in Beersheba, Israel, he joined CSIR-National Chemical Laboratory, Pune, India as a Ramanujan Faculty Fellow. In 2018, Dr Dandela became an Assistant Professor of Chemistry at the Institute of Chemical Technology, Indian Oil Odisha Campus Bhubaneswar, India. The research in his group focuses on structure-based drug design, bacterial signalling and polymorphism in pharmaceutical co-crystals. Dr Dandela has authored more than 100 publications, a number of book chapters, has 9 patents issued/pending and has routinely consulted in the area of drug discovery and pharmaceutical process research development. |
Introduction
Cancer has been one of the deadliest diseases from the ancient era to date. Cancer can be defined as uncontrolled cell growth in one or several parts of the body, including lungs, breast, stomach, bones, brain, prostate, and colorectum. As per the WHO report in 2018, over 18.1 million people are affected by cancer, and the death toll is estimated to be 9.6 million that means at least one in 5 men and one in 6 women have been developing cancer in their entire life.1 Furthermore, in 2020, GLOBOCAN published a report stating that 9.9 million people had died due to cancer excluding nonmelenoma skin cancer.2 There are several factors behind cancer prognosis in men and women, such as viral infection (Hepatitis and human papilloma Virus), high tobacco consumption and socioeconomic development.3 Natural products serve as a potential platform for the generation and identification of various potential drug candidates for a variety of therapeutic applications including cancer.4 Several pharmacologically active compounds have been found in various plant extracts (Bark, Roots, Leaves, Seeds, etc.) from different corners of the world. In the years 2006 to 2013 a large number of bioactive components (∼17
000) were found to be in pre-clinical trials and also many traditional medicines have been found to be highly effective against tumour and cancerous cells.5 Development of the novel anticancer agent from the natural product or natural product based derivatives with significant progress have been increased the number of natural product-based derivatives to 131 among 136 was registered during the last 30 years.6 Few of them exhibits promising anticancer activities, such examples are paclitaxel, docetaxel (Breast cancer), vinblastine, vincristine (Bladder & Breast cancer), cabazitaxel and romidepsin (Lung cancer), etc. and are currently available as commercial drugs.7 However, existing drugs are developing resistance in various cancer cells due to several factors (DNA repair, drug metabolism, epigenetic modification, etc.).8 To mitigate this issue, there are constant efforts to bring new anticancer agents with high efficacy and selectivity (Fig. 1). As an example, two important commercially available drugs tamoxifen and ibrutinib have been broadly prescribed during last the five years, but currently, tamoxifen has no significant anticancer activity against human breast cancer probably due to the development of resistance through mutation of ERα (estrogen receptor-α).9 Similarly, ibrutinib has developed resistance against chronic lymphocytic leukemia due to the mutation of BTK (bruton's tyrosine kinase).10 Currently many existing drugs already got resistance, and to circumvent the problem, many pipe-line drug candidates have been discovered using target-based phenotypic and probe based approach.11 Among these, probe based drug design through chemical proteomics approaches helps us to identify target protein and mechanism of action (MOA). Chemical proteomics is a novel and powerful strategy to unravel drug resistance by identifying mutant target site and hypothetical protein network.
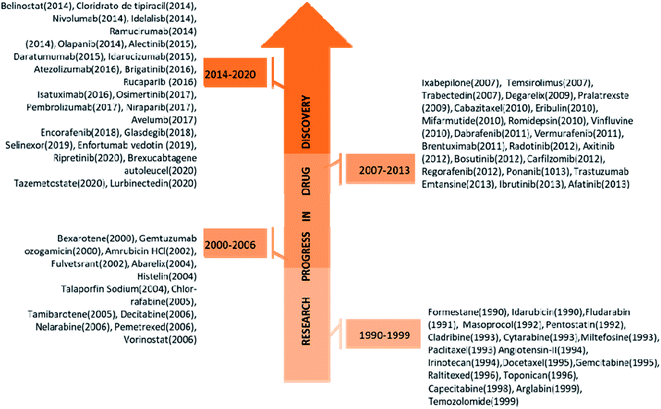 |
| Fig. 1 An overview of nature inspired anticancer drugs since 1990 to 2020. | |
Probe design and validation
Though it is quite challenging to synthesize chemical probes with unaltered parent drug's bioactivity, it helps us to establish the actual probe protein interaction and identification of new proteins involving in MOA of parent drug. In most of the cases, probe has three component-reactive warhead, reporter tag, and linker. Here reactive warhead consisting of several electrophilic moieties such as epoxy-ketone, enone, epoxysuccinates and fluorophosphonate etc. It is incorporated to the linker head to specifically interact with the target protein or any enzyme active site. Reporter tag can be any fluorophore, biotin or an alkyne functionality by unaltered parent drug's bioactivity, linker likely to be used for avoiding non-specific interaction.12,13 Sometimes parent drug molecule has no suitable site for a modification that is a major challenge for target identification and probe design. Subsequently, a non-labelling approach can resolve this issue by adopting new techniques such as – drug affinity responsive target (DARTS), this is most reliable technique where target proteins could be found irrespective of small molecules. It can work by decreasing protease sensitivity of target proteins or resist the target protein proteolysis of small molecule. Since, small molecule modification is not required for DARTS study, it's take a great advantage to identify the target protein of small molecule but the significant effectiveness of DARTS was found only in high concentration limit likely micromolar to milimolar range.14,15 However, other disadvantage using DARTS technique is the complication between target and non-specific target identification for small molecule. Therefore, another technique called SPROX (stability of protein from rate of oxidation) emerged in the proteomics field to identify target proteins more precisely. This straightforward method would have used of small molecule in complex proteome where drugs could form as drugs–protein complex. This protein complex should contain methionine (Met-) residue that could be oxidised by H2O2. According to their probe-protein stability, target proteins have to be identified by LCMS/MS spectrometry. The major drawback of this technique is peptide fragment must be containing Met- residue, simultaneously, assay's shows very high false negative rate.16 However, all these issues are mitigated by demonstrating CETSA (cellular thermal shift assay), using this technique we can study engagement of drugs in cellular environment to monitor target selectivity. Aggregation of target protein is one of the important steps in CETSA based proteomic approach. Despite its heavy uses in drug discovery industry, it has some limitation, take for example, this proteomics approach is not effective in non-homogeneous proteins, means if protein has unfolded drugs binding site may cause to reduce aggregation.17 These non-labeling approach is more admissible towards target proteins identification for small molecule because drugs modification is not required that allowing to keep parent drug information, but the limitation of these approaches are inadequate sensitivity of target and off target as well and other issues have already been discussed. The powerful and nontedious techniques such as chemical proteomics can resolve these challenging tasks by employing measurable modification to the parent drug. Two different approaches are commonly employed to prepare natural products based probes namely “clickable probe design” and “photo-affinity labeling probe”. ANPs based clickable probe would have been synthesized from ANP and alkyne or azide termini, click reaction was performed with ANP and biotin azide/alkyne in presence of a copper catalyst that affords a triazole link in between the reactive group and the affinity probe refer to bio-orthogonal click reaction.18 This strategy becomes renowned and particularly common for target identification of ANPs. This methodology is not useful for those ANPs targeting proteins with secondary non-covalent interactions. In such cases photo-affinity labeling (PAL) technique is highly useful. PAL has a diverse application in the chemical proteomics field due to its photo activatable moiety; even in presence of interfering metal ions such as trivalent Fe ions.19 The leading PAL groups are di-aryl ketone and diazirine have prominent photo-activity due to the formation of stable radical or carbene and other factors is the small size that will minimize the steric hindrance with the proximal vicinity of target protein. Therefore, PAL has to be incorporated into the sensitive part of the core reactive parent ANPs, in addition the evolving of non-interfering inert gas while the sample was treated under UV-irradiation (λ = 380 nm).20 Thereby, probe design is a very crucial part before going to the experiment and insights to the minimalist linker alkyne handle and photo-reactive diazirine motif is required, subsequently, probe was incubated with cell lysate followed by photo-irradiated under 380 nm UV-irradiation.21 A comprehensive study of diazirine synthesis and reactivity is covered by Robertson and co-worker.22 Very less number of review articles have been published to till date with a widespread discussion on chemical proteomics and traditional natural product medicine, highlighting articles are Wang et al. (2016),23 M. H. Wright et al. (2016),24 and Chen et al. (2020),25 and P. Gehrtz et al. (2021).26 A complementary study of quantitative application of chemical proteomics, several strategies to identify target proteins, probe protein interaction and their validation was covered in last ten years, also they disclosed an overview of electrophilic natural products and their target identification. We are fascinated by their work and intend to highlight the scope of ANPs and their chemical probes.
Activity and affinity-based chemical probes
Activity-based probes (ABPs) are comprehensively used to identify enzymes by crosslinking with cysteine, lysine or other nucleophilic amino acid residue in the microenvironment of the enzyme, such examples are the identification of protease, hydrolase, glycosidase etc. Design of ABPs are not limited to a certain concept, emerging of substrate mimetic ABPs are being populated in proteomics field, it directly binds to the enzyme active site and impedes their activity that leads to identifying the inhibitory activity of substrate, function of the enzyme in microbial pathogen (serine protease, Kinase/ATPhase, fatty acid synthases, glycoside hydrolase), PTPM (post translational protein modification), etc. application of ABPs was briefly discussed by William P. Heal,27 another research group Natallia C. Sadder et al. give a brief idea in ABPs.28 Along with that, term affinity based probes (AfBP) are often similar to ABPs but strongly differentiated by their mode of binding to their binding partner. Unlike ABPs, AfBP has to be designed by the adjusting of reversible warhead and photo-activable moiety with a compatible linker to optimize the binding affinity (Fig. 2). It is more reliable to use in target identification likely receptors, transferase, metalloprotease etc. where ABPs are failing to subject recognition. However, AfBP are performing to mark out the vulnerable target in two different ways; one is ‘electrophilic fragment profile’29 and other one, ‘tag shift’.30 Other strategies like in situ combinatorial probe synthesis, label based approach also applicable to improve AfBP.31 Sometimes it is spectacular that AfBP enabling drugs susceptible to recognize protein in complex proteome as low as in ppm label.31 However, when the drug is designed as an AfBP, it must be functionalized to immobilize by covalently attached agarose or magnetic bead during the anchoring process, pharmacological activity of drug should not be disturbed. Subsequently, this immobilized probe complex was incubated with extracted proteins (Fig. 3).32 Finally most of the non-specific proteins have been eluted and substantial protein enrichment is identified and quantified by the LCMS/MS-based analysis. In this review, we will cover the target identification of ANPs using chemical proteomics approaches and discussed the probe development and their synthesis. There are also more opportunities to developed new chemical probe of ANPs, such as Collismycin-A, Aaptamine, Erinacine A, Costunolide and Vioprolide-A.
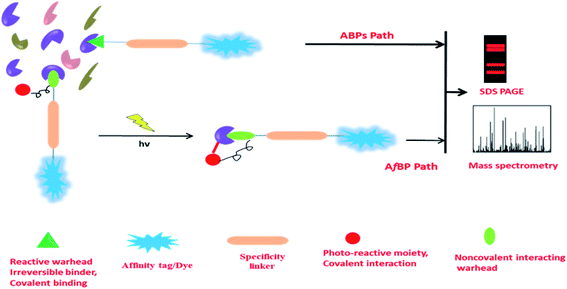 |
| Fig. 2 Schematic diagram of ABPs and AfBP. | |
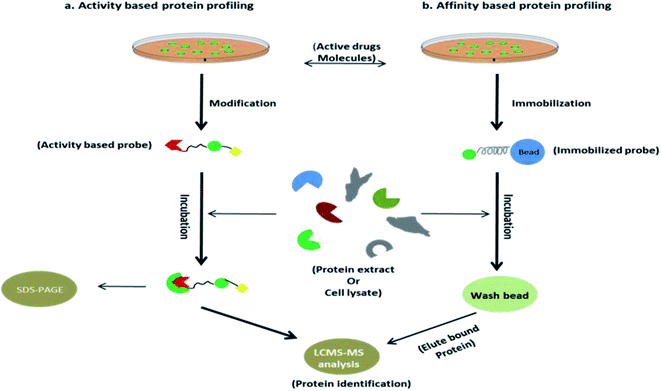 |
| Fig. 3 Schematic workflow of ABP and AfBP chemical probes. | |
Betulinic acid and target screening
Inspired by several plants derived cytotoxic agents, pezzuto and co-workers screened 2500 plant extracts in order to unravel selective cytotoxic agents. In 1995 it was first reported that Betulinic acid (BA) was found to be effective in human melanoma,33 due to the novel property of BA and inexpensive resources.
Whenever, BA was treated with human melanoma, ROS (reactive oxygen species) would be generated and that directly involved in programmed cell death, also it was observed that BA could depolarize mitochondria that has keen insight to understand the MOA of BA and validation.34 Inspired by its selective inhibition to human melanoma, several BA analogs were evolved and its SAR studies revealed that modification at C-3, C-28 and C-20 has to be improved to retain its more effectiveness in several bio-activity. For example, compound 8 had shown potent inhibition against HIV-1 mutation.35 Another derivative 9 had a great antitumor activity and 117 times more potent than BA.36 Despite several potential BA analogs were found, MOA and target identification still challenging. In 2017, Haijum et al. first reported the chemical proteomics based proteome profiling of BA in MCF-7 tumor cell, parallel efforts were also put forth to understand its biological targets. Are they similar to known target of BA like NF-κβ, topoisomerase, or it could bind a new target protein. It has been observed that target vary depending on the modification site, in vitro or cell based assay. In order to identify the target unambiguously, two different chemical probes 6 and 7 anchored at two different positions were synthesized (Scheme 1).37 The ideal linker in compound 6 and 7 are the tetrazole and diazirine based linkers which were incorporated in C-3 and C-28 position of BA after the substantial SAR study. The investigation was carried out in presence of only linker tetrazole and diazirine motif as a negative control, subsequently cell-based (assay MCF-7 cell line) and pull down LCMS/MS experiment was performed and the result suggests that the compound 6 is particularly located in the cytosol and lysosome whereas compound 7 preferably located in mitochondria and ER (endoplasmic reticulum), this distinct binding location likely for their photo-crosslinking characteristic.
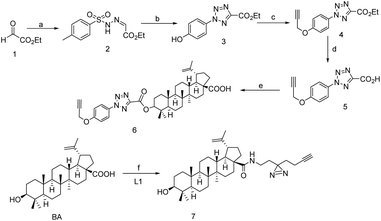 |
| Scheme 1 (a) Compound 1 (14.9 mmol), p-toluensulfonyl hydrazide (11.5 mmol), MeOH, rt, 1 h, (75%); (b) compound 2 (10 mmol), diazonium salt of N-(4-aminophenyl)aceamide (10 mmol), −5 °C to rt, 2 h, (63%); (c) compound 3 (4.3 mmol), K2CO3 (8.6 mmol), propargyl bromide (4.7 mmol), DMF, rt, 8 h (89%); (d) compound 4 (2 mmol), 4 N NaOH (1.5 ml) in 10 ml MeOH, rt, 8 h, (95%); (e) compound 5 (0.08 mmol), DCC (0.08 mmol), DMAP (0.08 mmol), betulinic acid (0.04 mmol), DMF, rt, 6 h, (71%); (f) 2-(3-(But-3-yn-1-yl)-3H-diazirin-3-yl)ethan-1-amine-L1 (0.11 mmol), BA (0.1 mmol), HOBt (0.15 mmol), EDCI (0.15 mmol), TEA (0.15 mmol), DMF (5 ml), rt, 5 h, (73%). | |
Although, it was previously proven that BA follows mitochondria and NF-κβ signalling pathway where AIF (apoptosis inducing factor) was involved in their signalling cascade.38 Chemical proteomics study revealed that 6 could extensively hit two major proteins of their corresponding gene PFKFB1 (6-phosphofructo-2-kinase) and C3 (human complement component C3), simultaneously AIFM1 (apoptosis inducing factor-1), PGK1 (phosphoglycerate kinase-1), APOL2 (apolipoprotein L2), PEX16 (peroxisomal membrane protein) were identified when the probe 7 was applied, and this can also be validated by pull-down WB experiment. However, even after the BA modification that probe 7 can able to identify target protein AIFM1, interestingly, in vitro study of probe 7 and recombinant PGK1 have shown the high affinity among the other identified proteins. Although, the role of these off target is still obscure.37 In this circumstance, all identified BA targeted proteins will help to further study for their anti-tumor activity of BA and BA related derivatives; also it will assist to investigate tumor resistance gene profiling.
Binding site identification of staurosporine-A
Staurosporine (STS) belongs to the alkaloid class of natural products with bis-indole structural characteristics. It was discovered in 1977 from the bacterial species Streptomyces staurosporine.39 However, in 1987 Weinreb synthesized it using [4 + 2] Diels–Alder reaction from sulfinylcarbamate and aromatic lactone.40
R. Bertrand et al. observed that staurosporine covered a wide range of programmed cell death in different cell lines (MOLT-4, HL-60 cell line).41 Moreover, STS has dynamic inhibitory activity including protease and phosphatase, but the programmed cell death is not well understood. Many research groups have speculated to unravel the inhibitory activity of STS, meanwhile, C. Meyer et al. observed that STS selectively inhibit cAMP dependent PKA (protein kinase A).42 Overall, deregulation of protein kinase-A increase abnormal cellular activities to initiate cancer progress, therefore emerging of PKA inhibitor is mandate to regulate the cellular abnormality. Interestingly, those small molecules that have high affinity towards PKA are not vulnerable in complex proteome, therefore, improvement of selectivity is highly desirable and this challenge was accepted by Jencks and Ricouart, to improve the PKA inhibitor selectivity by introducing bisubstrate analogy.43,44 However off target identification of STS probe has occurred prior to give more clarity in bisubstrate inhibitor. So far solid support affinity based profiling technique is useful while small molecule–protein interaction would be investigated for the study of STS and protein kinase.45 J. J. Fishar et al. strategically developed a water soluble probe 10, which include three major components; active part, linker and biotin to construct a AfBP. Finally, 100 kinases were identified in HepG2 cell lysate by capture compound mass spectrometry (CCMS) technique.46 Traditionally STS is known to be a competitive protein kinase (PK) inhibitor into the ATP-binding site and analysis suggests that it has multiple kinase binding sites. Haibin Shi et al. developed STS based clickable probe 11 and used it to investigate off target identification by in vitro and in vivo analysis. The IC50 analysis, cellular image and dose dependent experiment shows probe 11 exhibit a similar activity profile with STS and thus probe 11 enabled to identify 43 kinase, among these, 35 and 25 kinase are identified by in vitro and in situ respectively from liver cell HepG2.47 However, to reduce the non-specific proteins, Xiamin et al. evaluated STS by introducing chloro-acetamide functionality to STS such that it was transformed to an irreversible covalent probe and this small change is highly tolerable to retain its bioactivity and specifically selective to cysteine residue near to ATP-binding site of protein kinase. Therefore, desired probe, 17 was developed (Scheme 2) and applied for in vitro analysis. Data strongly supports that the probe 17 is selectively inhibits c-Src kinase probably for due to the high reactive proximal residue Cys-277, where Cys-277 having in proximal distance of ATP-binding pocket in c-Src kinase. This clue motivates them to further investigate in precise STS-binding partner. Thereby, an in situ experiment was performed and the presence of c-Src kinase in HepG2 liver cancer cell has been confirmed, which indicates that the tyrosine kinase c-Src was selectively targeted by probe 17. Not only kinase c-Src, 11 kinases were also identified but their score is moderate to low. However, probe 17 is much useful to identify unidentified proteins in kinase cysteinome.48
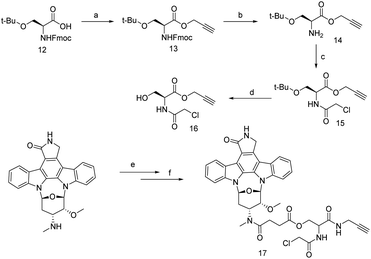 |
| Scheme 2 (a) Compound 12 (0.7 mmol), 10% Na2CO3 (5.2 ml) in dioxan (3.9 ml), Fmoc-Cl (1 mmol), rt, 20 h, (85%); (b) compound 13 (0.5 mmol), propargylamine (49 μL), HOBt (0.78 mmol), HBTU (0.78 mmol), DIEA (1.56 mmol), DMF, rt, 12 h, (93%); compound 2 (4.8 mmol), piperidine/DCM (1 : 4, 50 ml), rt, 0.5 h, (c) compound 14 (5 mmol), DIEA (15 mmol), 2-chloro-acetyl (10 mmol), dry-DCM, 0 °C to rt, 0.5–2 h, (72%); (d) compound 15 (2.9 mmol), TFA (15 ml), rt, 0.9 h, (39%); (e) compound STS (0.01 mmol), succinic anhydride (0.015 mmol), DMAP (0.02 mmol), TFA in water (0.1%), DMSO, rt, 30 h, (92%); (f) compound 16 (17.8 pmol), STS-acid (8.8 pmol), EDC (80 pmol), DMAP (20 pmol), DMSO, rt, 12 h, (7.4%). | |
Zerumbone and target identification
Zerumbone, sesquiterpene found in Zingiber zerumbet smith plant is eleven member cyclic compound (Fig. 4) with C2, C6, C10 alkene functionalities.49,50 Zerumbone have been used in a broad range of therapeutic applications, such as antibacterial, antipyretic, anti-hypersensitive, anti-inflammatory and several immunomodulatory activities,51,52 This broad spectrum medicinal properties of zerumbone attracted to investigate the antiproliferative activity of zerumbone. Finally, it was found that zerumbone is potential for cancer treatment. Recently, T. Sithara et al. and S. Girisha et al. gave a brief review of zerumbone to the several types of cancer treatment.53,54 Unfortunately, this discussion is limited to various pathway by which cancer growth might be suppressed, but our provocation is more insightful to realize the target proteins of zerumbone where it follows in several pathways to suppress cancer growth. Many studies found that α,β-unsaturation in zerumbone acts as Michel acceptor and some of pro-inflammatory gene as such COX-2, iNOS and their expression could be suppressed.55 Therefore, to identify the cellular target of zerumbone, probe 20 (Schemes 2 and 3) was envisaged and by incorporating alkyne handle for click chemistry. Being a cell permeable probe, by incubating with HeLa cells, followed by click reaction with azido-TAMRA-biotin (AzTB) the potential targets were identified. Subsequently, gel based proteome analysis was performed including fluorescence scanning image analysis. These data encouraged them to investigate the ‘spike-in’ SILAC52 based global or large scale analysis in protein profiling of probe 20, ‘spike-in’ SILAC protein quantification strongly differentiate 151 kinase among all 600 proteins. Though, only 20 proteins are sensitive to zerumbone from low to high concentration and many of these 20 proteins are directly involved in apoptosis, DNA repair, DNA damage, electron transport, host–virus interaction, cell survival etc. Bioinformatics analysis of target identification of zerumbone was consent to the SILAC experiments and 20 proteins are abundantly identified that comes into the upper right quadrant in scattered plot and the majority of these 20 proteins mostly belong to cytoplasm, mitochondria, and nucleus.56 We have noticed all 20 important proteins are associated in many disease including cancer and one most important things should be noted so far no inhibitors are readily available for these identified proteins. This competitive study and interactome network will help to understand the function of these identified proteins in biological pathways and discovery of selective and potential inhibitor to suppress cancer growth (Scheme 3).
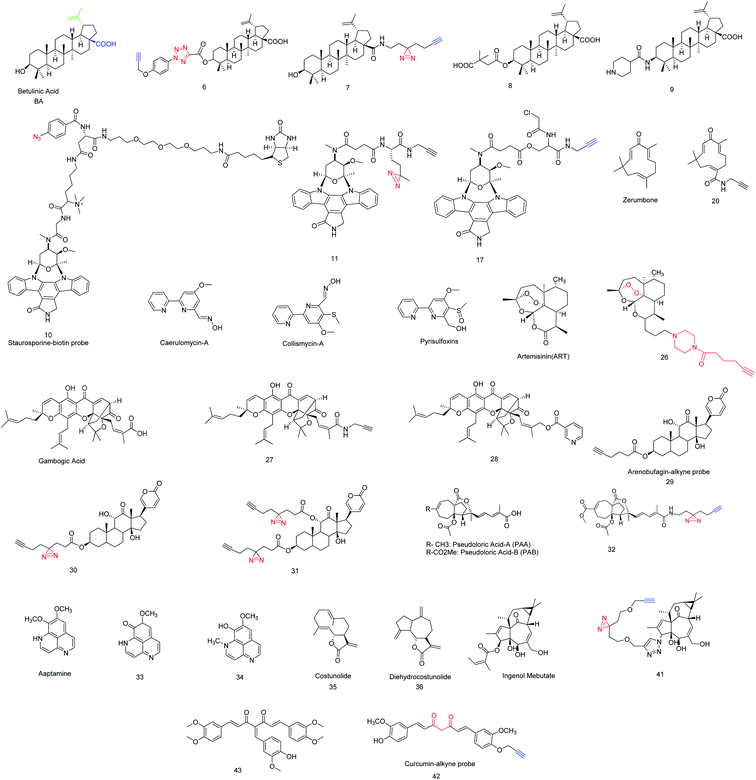 |
| Fig. 4 Chemical proteomics probes by modifying the listed ANPs, these are Betulinic Acid, zerumbone, collismycin-A, artemisinin, gambogic acid, arenobufagin, pseudoloric acid, costunolide and curcumin. | |
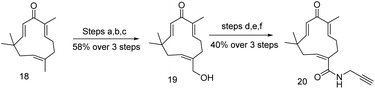 |
| Scheme 3 (a) NBS, acetonitrile/water (1 : 1), rt, 1 min.; (b) sodium acetate, DMF, rt, 16 h.; (c) NaOH, H2O, rt, 6 h.; (d) TEMPO/BAIB, CH3CN/H2O (1 : 1), rt, 16 h; (e) NaClO2, NaH2PO4, t-BuOH, H2O, 2-methyl-2-butene, rt, 6 h; (f) propargyl amine, HATU, DIPEA, DMF, rt, 1 h. | |
Collismycin-A and iron chelator
Collimycin-A (CMA) was found in Streptomyces sp. micro-organism known to be an anti-microbial as well as anti proliferative agent in cancer cells having a bipyridyl system similar to pyrisulfoxins and caerulomycins (Fig. 4).57 Epigenetic regulation, hypoxia, and many cellular processes exist in iron-requiring protein such as Fe–S cluster protein.58 The interchanging of the iron oxidation state will produce a free electron that participates in several biological processes such as generating reactive oxygen species (ROS) that could damage lipid, DNA, and several proteins.59 Since, iron is the dietary element in cancer cell, dysregulation of iron metabolism can enhance cancer risk. Moreover, iron dependent channel disruption and the mechanistic role of iron in cancer cell is still obscured. Makoto's group observed the CMA has particularly chelated with cellular iron in cancer cells and chemo-proteomics of CMA in HeLa cell lysate described the proteomics changes of glycolysis and phosphorylated related proteins and their off targets. 2D-gel electrophoresis and overlaid image processing explored 296 differential spots. Proteomic changes, those are up-regulated came from gene expression instead of protein modification and for further validation, more research should be necessary to give a better clarity in their metabolic dysregulation. Glycolysis related proteins such as ALDOA (fructose–biphosphate aldolase), PGAMI (phosphoglycerate mutase 1), PGK1 (phosphoglycerate kinase 1), TPI1 (triosephospahate isomerase) found in up-regulated spot, also proteomics data revealed that especially the glycolytic pathway was disrupted by the layout of HIF-1α (hypoxia inducible factor 1-alpha) expression.60
Artemisinin and profiling of multiple targets
Artemisinin (ART) a sesquiterpene lactone, also with unusual peroxides is extracted from Artemisia annua known as ‘sweet wormwood’,61 native in Asia.
ART is widely used as antimalarial drug for the last few decades, during this period many ART related derivatives were evaluated and their MOA against malaria was well documented by Jing Yang.62 Y. Tu et al. proposed that the dihydro form of ART is ten times more effective than normal ART in consent of both anti-malarial and drug stability.63 Since the dihydro form has greater anti-malarial activity than ART and this phenomenon attracted many people to investigate the biological activity of dihydro ART derivatives. Interestingly, few of them have found to potentiate in tumor suppression and reduce several types of cancer risk and this was comprehensively reviewed by A. K. Das et al.64 Despite artemisinin being an effective anticancer therapeutic agent, but there has much controversy in fundamental mechanism and it has been considered that the dihydro-form of ART perturbed ferroptosis in cancer cell by increasing GPX4 (glutathione peroxidise 4) enzyme.65 The earlier experiment had shown that without modification of ART targeted proteins are non-covalent and reversibly interact with ART and mostly follows iron uptake from cancer cell. Since membrane glutathione S-transferase (GST) in malaria parasite is one of the ART targeted protein, thereby investigation of ART as anti-cancer agent in human have chosen. GST is a key protein and its depletion was observed in cancer cell. Therefore, insight of Yiqing et al. described precisely the action of ART on GSTs in the cancer cell to ensuring the significant of endoperoxide-ART in cancer cell. Clickable probe 26 was synthesizing by multistep reaction as shown in Scheme 4. Proteomic analysis of 26 in cancer cells in presence of ART and subsequent chemo-proteomics workflow would suggest that 26 is hemin dependent and it was alkylating to the target protein by one-electron reduction of hemin to heme. Ultimately four GSTs members of proteins GSTP1 (glutathione S-transferase P), GSTK1 (glutathione S-transferase κ-1), GSTO1 (glutathione S-transferase omega-1), GST3 have been found in HeLa cell membrane, but the study of human recombinant GSTs member proteins with 26 shows that artemisinin is feeble towards GSTO1, which means that GSTO1 is non-specific binder. Also it was being notified that 26 does not penetrate cells and hence, further investigation required to develop ART permeable probes for more precise cellular target identification.66,67
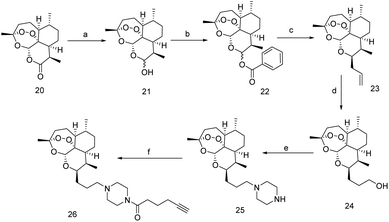 |
| Scheme 4 (a) NaBH4, MeOH, 0 °C; (b) benzoyl chloride, pyridine, DMAP, CH2Cl2, rt; (c) AllylTMS, ZnCl2, 0 °C.; (d) (1) BH3·SMe2, Et2O, −20 °C.; (2) H2O2, Na2CO3, H2O, rt; (e) (1) MsCl, Et3N; (2) piperizine, THF, reflux; (f) 5-hexanoic acid, EDCI, HOBt, dry DCM, rt (97%). | |
Gambogic acid and target profiling
Gambogic acid (GA) is a xanthonoid based NP discovered from Garcinia hanburyi. GA could also be used as folk medicine in Southeast Asia and exhibits anti-proliferation in tumors and various cancer cells.68,69
Many of the cancer suppression have known, among this, heat-shock protein inhibition is widely accepted mechanism of action. Therefore, several drugs are clinical as well as preclinical approved to inhibit heat-shock protein 90 (Hsp90) but none of the drugs exhibit selectivity towards a specific heat-shock protein. Biotinylated gambogic acid enables probe 27 selective towards heat-shock protein 90-β (Hsp90β) instead of their isoform and it is key protein for the survival of cancer cell. Many Hsp90 inhibitors are available that could specifically bind to nucleotide pocket in N-terminal domain but their selectivity in a particular protein in Hsp90 family was very less.70,71 Instead of this, GA can exclusively inhibit nuclear ex protein-1 (CRM1) binding in two nucleophilic site cys942 and cys939 leading to successively target identification of GA.72 Therefore, searching the tangible binding protein of GA, clickable ABP probe 27 was developed by acid-amine coupling reaction with GA and propargyl amine (Scheme 5) and subsequently, TMT (tandem mass tagging) based quantitative analysis was revealed several binding targets of GA in the native cellular environment.73
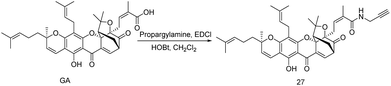 |
| Scheme 5 (a) GA (0.012 mmol), EDCI (0.02 mmol), HOBt (0.02 mmol), DIPEA (0.05 mmol), propargyl amine (0.02 mmol), rt, 12 h, (50%). | |
Pull-down assay were successively performed and subsequent data suggested the high enrichment of RPS27A (40S ribosomal protein) which was previously found in many cancer cell line where proliferation in normal cell cycle was blocked by this ribosomal protein.74 This investigation elucidates that ability of probe 27 to identify RPS27A as protein target. Another essential component S1P (sphingosine-1-phosphate) is directly involved in the precancerous cellular process, mainly it could reduce the normal cellular apoptosis, so inhibition of S1P biosynthesis should be required and this de novo synthesis was catalyzed by SPT (serin palmitoyltransferase).75 Human SPT is stimulated by especially two activating subunits SPTSSA (serine palmitoyltransferase complex-A) and SPTSSB (serine palmitoyltransferase complex-B).76 After the substantial chemo-proteomics workflow, it was confirmed that GA could covalently binds to SPTSSB and it was fascinating to investigate the activity in similar kind of derivative of GA. Surprisingly, probe 28 (Fig. 4) had shown prominent affinity in SPTSSB over the GA and also reduces spingolipid level including S1P and phosphorylation of oncogenic kinase AKT.77
Arenobufagin and covalent binding partner
Arenobufagin is a traditional anticancer drug in china, mostly treated for liver cancer over the last few decades. It is a highly toxic steroid usually found in the venom of Bufo gargarizans.
In 2013, it was observed that arenobufagin can potentially suppress several tumor growths and follows an important pathway by blocking Na/K ATPase α-subunit cavity to inhibit the imports of ions in human carcinoma HeLa cell. It was also found that arenobufagin inhibit G2/M cycle in cancer cell.78 Beside this, arenobufagin has also showed multiple effects in the cancer cells. In order to identify arenobufagin target, three active model compounds 29, 30, 31 (Fig. 4) were ingeniously executed for in vitro/vivo analysis 29 and 30 both these clickable probes were incubated in HePG2 cancer cells. Subsequently the chemo-proteomics workflow was performed by following biotin azide/TAMRA-N3, standard click chemistry condition,79 and SDS-PAGE. When the experiment was completed in presence of excess arenobufagin, some spot in gel-fluorescence disappeared while 29 was subjected for the corresponding probe-target but the binding propensity of 30 was relatively high due to covalently reversible binding to the target in the native cellular environment that is supported by live-cell imaging.81 This live-cell imaging would suggest that 30 is more selective and proficient because of photo-affinity labeling minimalist linker. Substantial chemo-proteomics workflow and large scale pull-down/WB experiments would reveal the true target for arenobufagin. Universally known target ATP1A1 (sodium/potassium-transporting ATPase alpha-1) and one unknown target PARP1 [poly(ADP-ribose) polymerase 1] were identified exclusively in MDA-MD 436 breast cancer cells.80,81 Therefore to understand the arenobufagin mechanistic pathway, siRNA transfection was imposed leading to knockdown of PARP1. Again the study of recombinant PARP1 and arenobufagin shows a high affinity of arenobufagin towards PARP1. However, it was found that arenobufagin has merely anticancer activity against MCF-7 cell line.81 Thereby we can come to conclusion that arenobufagin is a very specific anticancer agent in the sake of PARP1 sensitive cancer cells (Scheme 6).
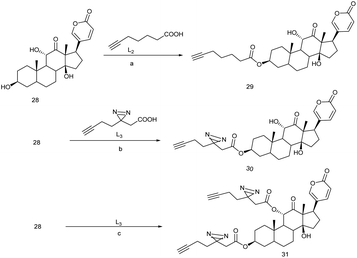 |
| Scheme 6 (a) L2 (0.13 mmol), DCC (0.16 mmol), DMAP (0.066 mmol), compound 28 (0.13 mmol), dry DCM, rt, 5 h (50%); (b) L3 (0.066 mmol), DCC (0.079 mmol), DMAP (0.033 mmol), compound 28 (0.066 mmol), dry DCM, rt, (43%); (c) L3 (0.13 mmol), DCC (0.079 mmol), DMAP (0.033 mmol), compound 28 (0.066 mmol), dry DCM, rt, 24 h, (60%). | |
Pseudoloric acid
Pseudoloric acid is a class of terpenoid usually found in the root and bark of Pseudolarix Kaempferi Gordon (Pinaceae). Pseudoloric acid A (PAA) and pseudoloric acid B (PAB) both of these traditional medicines are widely used in china.82
However, the medicinal activity of PAB is much better than PAB while they were subjected to a range of disorders or infections such as antifertility, cytotoxic, and fungal infection.83 It exhibits cytotoxicity against multidrug-resistant human liver cancer cell (SMMG-7721). This inspired to further explore its anticancer activities against HSO578T breast cancer cell, lungs cancer cell and P-388 lymphocytic leukemia and observed ED50 of 0.26, 0.60, 0.57 and 0.08 μg mL−1.83 It was found that PAB was deleteriously interfering in (p53, ERK/MAPK, NF-κB) signaling process.84,85 However,a recent study shows PAB is a potential inhibitor of microtubule polymerization within IC50 of 1.1 μM, and loosely bound to the colchicin binding site, half life of tublin-PAB complex lesser a second.86 To elucidate the actual binding protein of PAB, a photo-affinity labeling with alkyne handle (Fig. 4) was introduced in the optimized location of PAB by keeping all drug-able information of parent PAB. Afterwards, chemo-proteomics workflow leads to the performance of probe 32, (Scheme 7) were probe hits to the four important proteins; VDAC1 (voltage dependent anion selective channel 1), VDAC2 (voltage dependent anion channel 2), CD147 (cluster of differentiation 147), and SLC3A2 (heteromeric amino acid transporter), among these CD147 and SLC3A2 have chosen because these are highly expressed type-1 glycosylated transmembrane protein in the cancer cell. Resulting data suggest that 32 would be selectively targeted CD147 rather SLC3A2.87 Multiple experiments would reveal that 32 could perturb CD147 oligomerization and simultaneously downregulates MMP-7, MMP-2, MMP-9 which are considered to be essential proteins of tumor progression.87 We are anticipating more pseudoloric acid analogues that could exert their anticancer activities via selective target.
 |
| Scheme 7 (a) Pseudoloric acid-B (0.016 mmol), EDCI (0.025 mmol), HOBt (0.041 mmol), DIPEA (0.038 mmol), 2-(3-(but-3-yn-1-yl)-3H-diazirin-3-yl)ethan-1-amine (0.021 mmol), dry DCM, rt, 3.5 h (80.6%). | |
Aaptamine
Aaptamine was found in marine sponge called Aaptos aaptos.88 Aaptamine has strong antimicrobial as well as antifungal properties. S. A. Dyshlovoy et al. showed the significant of anticancer activity of aaptamine where G2/M phase cell cycle would have arrested. When the concentration of aaptamin was increased, apoptosis was observed and 2D-gel based data expressed five unknown proteins (PEA15, TPT1, CTSD, CRABP2, CFL1) those are down regulated in aaptamin treated sample.89 However, two different isoforms of aaptamine, 33 and 34 (Fig. 4) had shown strong anti-tumor activity in cis-platin-resistant embryonal carcinoma cell line NT2. Dimethyl oxy aaptamine 33 and isoaaptamine 34 have equal activity in NT2 carcinoma cell line, mostly acts as apoptotic inducer including post transcriptional protein modification while many altered protein was observed in western blot analysis and ingenuity pathway analysis suggests that probe 33 and 34 will be target p53, myc and TNF.90 Hopefully clickable modified probe of aaptamine will give more precise information on target identification and pathway analysis to modulate tumor growth or precancerous stage.
Erinacine-A
Erinacine A is a diterpene compound extracted from hericium erinaceum (edible and medicinal mushroom) native in North America, Asia, and Europe.91 It was found that erinacine has prominent antimicrobial, antifungal, and anticancer activity. Therefore the effect of erinacine A in cancer cell was extensively studied and it gave the potential of erinacine-A especially in the TSGH-9201 cell line.91
In vivo analysis suggests that erinacine A could arrest the cell cycle at G1 phase in the human colorectal cancer cells, detailed investigation reveals that erinacin-A activates NF-κB/p70S6K signaling pathway which is evident for the generating of p21 and inactivation of cdK2/cyclin E and cdK4/cyclin D1.92 Again, Hsing-Chum Kud et al. observed that the effectiveness of erinacine A in gastric cancer cell line and proteomics workflow would suggest the generating of ROS, caspases activation and phosphorylation of KAH/AKT, p70S6K (ribosomal protein S6 kinase β-1) and PAK1 (p21-activated kinase-1) cumulatively provide a new pathway to suppress cancer activity in TSGH-9201 gastric cancer cell line.91 To elucidate the actual mechanistic overview of erinacine-A further investigation through chemical proteomics technique is required.
Costunolide and dehydrocostunolide
Costunolide is a sesquiterpene NP extracted from Saussurea lappa's root. These medicinal plants are native in India, China, and Japan. However, this lactone rich costunolide has potential anti-breast cancer activity which was discovered by Mu-Xiang.93
In recent years it has been found that the combination of Costunolide 35 and dehydrocostunolide 36 (Fig. 4) have remarkable anti-breast cancer activity in vitro and in vivo.94 To address the mechanistic pathway of costunolide in breast cancer, identification and quantification of newly expressed protein was performed by using iTRAQ (isobaric tags for relative and absolute quantification) based analysis.95 From bio-informatics analysis it has to consider that the combination of 35 and 36 can interfere in various apoptotic signaling pathways such as c-Myc dependent signaling pathway, 14–3–3 mediated and protein kinase-A (PKA) signaling pathway. Substantial proteomics approach reveals that the combinatorial dose-dependent (35 and 36) would increase the ratio of p53/c-Myc that relies on the up-regulation of p53 and down-regulation of c-Myc. This up-regulation of p53 was responsible for the increase in BAX and BCl-2 protein and this is directly related to the mitochondrial apoptosis in human breast cancer.96 The mechanism of p53 and c-Myc in human breast cancer are not fully understood, therefore the investigation of costunolide and its invasion is anticipated for effective therapeutic development.
Ingenol mebutate and target identification
The trade name of Ingenol Mebutate (IngMeb) is ‘Picato’, treated for ‘actinic keratosis’ refer to as pre-cancerous.97 IngMeb has been treated as a potential therapeutic agent for this disease. IngMeb is the exoteric nature-inspired diterpene that was found in Euphorbia peplus a tropical medicinal plant in Europe, West Asia, and North America.98
Due to the adverse effect of IngMeb, few european countries had withdrawn IngMeb from the market in February 2020.99 But it was constantly used as a remarkable therapeutic agent for the past few decades, mainly for skin cancer. It is required to understand the action of IngMeb at molecular level. In this perspective, a clickable probe was synthesized from 37, across the several difficult reaction steps, 41 was successively synthesized (Scheme 8) wherein covalent protein trapper photo-actively diazirine group and minimalist alkyne handle were incorporated for the feasibility of actual target identification of IngMeb. Subsequently, chemo-proteomics workflow was performed and 41 could reveal the principle target of IngMeb.
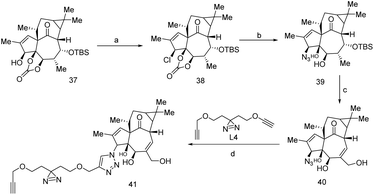 |
| Scheme 8 (a) (1) Compound 37 (0.283 mmol), TEA (0.358 mmol), MsCl (0.358 mmol), dry DCM, 0 °C, 1 h; (2) TBACl (0.714 mmol), DMF, 75 °C; (b) LiN3 (20 wt%, 0.295 mmol), dry DMF, 80 °C, 8 h; (c) (1) compound 39 (0.087 mmol), TASF (0.174 mmol), dry DMF, rt, 22 h; (2) Martin's sulfurane anhydrous (0.5 ml), CHCl3, reflux, 1 h; (3) SeO2 (0.25 mmol), formic acid (0.3 ml), dioxan (0.6 ml), 80 °C, 6 h; (d) compound 40 (0.04 mmol), CuSO4, 5H2O (0.008 mmol), sodium ascorbate (0.40 mmol), L4 (0.20 mmol), dry MeOH, rt, 20 h, (37%). | |
SLC25A20 (mitochondrial acylcarnitine transporter) was identified as the primary target such that it inhibits mitochondrial dysfunction in cancer cells (HeLa cell line) but earlier it was seen that Protein kinase-C (PKC) used to interacts with other proteins for the localized inflammatory response.100 Interestingly, recombinant SLC25A20 does not affect IngMeb cytotoxic activity, that means mechanistic approach of IngMeb follows SLC25A20 independent biological pathway. Author seems that remodel of probe 41 is required for future studies.100
Curcumin
Curcumin is a polyphenolic natural product and it is the key component in Curcuma longa, trade name turmeric traditionally used in Indian cuisines and remedy from several diseases. Recent study shows that curcumin controls several tumor growths effectively and uses as anti-cancer therapeutic agent. However, it is involved in several apoptotic signaling pathways such as MAPK, NF-κB, p53/p21, P13-K-AKT.101,102 We anticipated the curcumin engineered probe to elucidate mitochondrial dysfunction and several signaling pathway. Thereby, to assess their remarks, a cell-permeable clickable probe 42 was synthesized by ‘–OH’ alkylation using propargyl bromide and potassium carbonate in dry DMF solvent (Scheme 9) which can identify specific binding partners of curcumin in the colon cancer cell line (HCT116). However, the comparative study suggested the activity of probe 42 is unaltered with parent curcumin, thereby 42 is the preferable probe for investigation. Subsequently iTRAQ and TMT based proteomics analysis followed by LCMS/MS was performed and it shows 370 new proteins were found. Also, curcumin hit protein network agreed with EIF2, eIF4/p70S6K and mTOR signaling pathway, throughout this pathway cellular protein synthesis in colon cancer cell might be inhibited.103 To owing for improvement of curcumin in various cancer application, several derivatives have emerged as the potential therapeutic candidate, such as 4-arylidene curcumin 43 (Fig. 4) effectively arrest tumor cell cycle by inhibiting the proteasome, protein 14–3–3, Hsp90 also it can modulate G1/S cell cycle by recognition of cell cycle modulating protein such as p21, p27, cyclin D1, cyclin E etc. Since 43 has diverse molecular target similar to curcumin, appeared to be more vulnerable to the target site.104
 |
| Scheme 9 Synthesis of activity based curcumin probe. | |
Vioprolide-A
Vioprolide is a cyclic oligopeptide based natural biosynthetic product found in cystobacter violaceus. Several classes of vioprolide have found in nature based on heterocyclic ring in their peptidolide skeleton, these are vioprolide A, B, C, and D. Recent study has found that vioprolide is a potential nature-inspired anticancer agent; also it was found that vioprolide would be effective for immunomodulation.105 Therefore target profiling should be required to investigate which vioprolide is more crucial for antiproliferation. Subsequent broad-spectrum proteomics and biochemical method would suggest that vioprolide-A is effective in Jurkat cancer cells and data provides the probable target of vioprolide-A in nuclear protein 14 (NOP14).106 Thereby, ribosome biosynthesis was inhibited by vioprolide-A, since NOP14 was the key substance for ribosome biosynthesis. Further studies of vioprolide will be expected due to their adequate anti-cancer activity in various cancer cell lines.
Conclusion and future prospective
To summarize, the study of chemical proteomics technique in various cancer cells is well established. Nature inspired anticancer drugs were engineered by incorporating photo-actively functional moiety and a suitable linker enabling unaltered parent drug information. Moreover, the proteomics analysis including 2D-gel electrophoresis, iTRAQ and TMT followed by LCMS/MS analysis and statistical data analysis using swiss-port, mascot and NCBI data-base assisting to find out the new proteins involved in protein network. Therefore, chemo-proteomics technique has an extreme application to precisely characterize and pathway analysis of ANPs treated sample. Several targets (see Table 1) namely CD147, SLC3A2, p53, SLC25A20, 14–3–3, HSP90, Bcl2, CDK1, CDK2, GPX4, ATP1A1, PGK1, AIFM1 etc., and signalling pathways such as EIF2, eIF4/p70S6K, mTOR, MAPK, NF-κB, p53/p21, P13-K-AKT were dissected for the natural products betulinic acid, staurosporine-A, arenobufagin, pseudoloric acid, costanolide, ingenol-mebutate, curcumin and vioprolide using chemical proteomics approach. As per current status, most of the cases PAL have been introduced to the ANPs, probably for their small size diazirine (DA) and flexible linker. Now days no such tools are available to investigate the MOA of ANPs in cellular environment, chemical proteomics reveals ANPs have multiple targets in cellular environment instead of single target, but the important of these targets in biological pathway is still elusive. The most advantage of chemical proteomics approach is the sensitivity and reliability towards low abundance protein because fully engineered probe can covalently trap to the target protein. Another important advantage LCMS/MS spectrometry has been used to determine peptide sequences according to their m/z ratio. Despite of powerful, reliable and straightforward method, it has few limitations that photo-reactive functionality in chemical probe is generally be used in chemical proteomics study that could not allow identifying pharmacologically cognate proteins. Moreover, ANPs is generally a complex molecule, such example as vioprolide, a macrocyclic polypeptide which carried multiple functional moieties. Design of chemical probe is challenging process where synthesis of chemical probe is highly tedious, since, ANPs have multiple targets, new strategies are required to identify and unravel molecular level targets of ANPs with high degree of target selectivity should focus newly founded off target of ANPs to make a new inhibitory scaffold. Undoubtedly, chemical proteomics approach remains a key player in identifying new MOAs for therapeutically important NPs and play crucial role in the drug discovery process.
Table 1 Summary of identified target proteins, off target proteins and varied chemical probes
Parent ANPs |
Chemical probes (Fig. 4) |
Molecular target/off target identification (gene symbol) |
Cancer cell line (type of cancer cells) |
Ref. |
Betulinic acid |
6 and 7 |
Bcl-2 family of protein, p53-protein, AIFM1 (target), PFKFB1, C3, PGK1, APOL2, PEX16 (off target) |
Glioblastoma cells, MCF-7 (tumor cells) |
37 and 38 |
Staurosporine-A |
10, 11 and 17 |
Bcl-2 protein family, CDK1, CDK2 (target), RPS6KA6, PFKM, CKB, PRPS2, ADK, PRPS1, PKM, PRKDC, PRKAR1A, FYN (off target) |
HepG2 (liver cancer cells) |
47, 48 and 107 |
Zerumbone |
20 |
Bcl-2, cytochrome-c, COX-2, iNOS (target), DFNA5, CDA, UVRAG, LCMT1, NT5DC1, CPPED1, NT5CD2, FAM114A1, GCLC, MLKL, NR3C1, ATXN10, TMPO, RPS6KA1, BRAT1, MCMBP, MAGED2, UQCRC1, SYNCRIP, DUT (off target) |
Liver cancer cells |
54–56 |
Collismycin-A |
CMA |
ALDOA, PGAMI, PGK1, TPI1 (target) |
HeLa cells |
60 |
Artemisinin |
26 |
GPX4 (target), GSTK1, GSTP1, GST3 (off target), GSTO1 (non specific target) |
HeLa cells |
65–67 |
Gambogic acid |
27 |
RPS27A, S1P, SPTSSB, Hsp90 (target) |
Live HeLa cells, K562 cells, MCF-7 cells |
73 and 77 |
Arenobufagin |
29, 30 and 31 |
Na/K ATpase α-subunit, ATP1A1 (target), PARP1, NOP2, DDX5, ERGIC1, CPNE1 (off target) |
MDA-MD 436 (breast cancer cells), MCF-7 |
78 and 81 |
Pseudoloric acid |
PAB and 32 |
VDAC1, VDAC2, CD147, MMP-7, MMP-2, MMP-9 (target), SLC3A2, TUBB, TUBB4B, IPO7, CSE1L, TNPO1, HSP90AB1 (off target) |
SMMG-7721 (multidrug-resistance human liver cancer cells) |
87 |
Aaptamine |
33 and 34 |
PEA15, TPT1, CTSD, CRABP2, CFL1 (off target) |
NT2 cell line (cis-platin resistance embryonal carcinoma cells) |
89 and 90 |
Erinacine-A |
Erinacine-A |
P70S6K, PAK1 (target) |
TSGH-9201 cell line (gastric cancer cells) |
91 and 92 |
Costunolide |
35 and 36 |
P53, c-myc, Bcl-2 (target) |
Breast cancer cells |
96 |
Ingenol mebutate |
37 and 41 |
SLC25A20 (target), SCCPDH, PON2, NUCB1 (off target) |
HeLa cancer cells, HEK293T cells |
100 |
Curcumin |
42 |
Protein 14–3–3, Hsp90, cyclin D1, cyclin E, TNF-α, Bcl-2 (target), PRDX1, TUBB, HS90, GAPDH, FASN (off target) |
HCT116 cell line (colon cancer cells) |
103 and 104 |
Abbreviations
NPs | natural products |
ANPs | anticancer natural products |
MOA | mechanism of action |
ERα | estrogen receptor-α |
BTK | Bruton's tyrosine kinase |
DARTS | drug affinity responsive target |
TPP | thermal proteome profiling |
CETSA | cellular thermal shift assay |
SAR | structure–activity relationship |
ABPs | activity-based probes |
AfBP | affinity based probes |
PAL | photo-affinity labeling |
BA | betulinic acid |
ROS | reactive oxygen species |
PFKFB1 | 6-phosphofructo-2-kinase |
C3 | human complement component C3 |
A1FM1 | apoptosis inducing factor-1 |
PGK1 | phosphoglycerate kinase-1 |
APOL2 | apolipoprotein L2 |
PEX16 | peroxisomal membrane protein |
STS | staurosporine |
PKA | protein kinase A |
CCMS | capture compound mass spectrometry |
SILAC | stable isotope labelling with amino acid |
cSrc | SRC proto oncogene, non receptor tyrosine kinase |
COX-2 | cyclooxygenase-2 |
iNOS | inducing nitric oxide synthase |
HIF-1α | hypoxia inducible factor 1-alpha |
CMA | collimycin-A |
ALDOA | fructose-biphosphate aldolase |
PGAMI | phosphoglycerate mutase 1 |
PGK1 | phosphoglycerate kinase 1 |
TPI1 | triosephospahate isomerise |
ART | artemisinin |
GPX4 | glutathione peroxidise 4 |
GST | glutathione S-transferase |
GSTP1 | glutathione S-transferase P (protein) |
GSTK1 | Glutathione S-transferase κ-1 (protein) |
GSTO1 | Glutathione S-transferase omega-1 (protein) |
Hsp90 | heat-shock protein 90 |
Hsp90β | heat-shock protein 90-β |
TAMRA-N3 | tetramethylrhodamine azide |
AzTB | azido-TAMRA-biotin |
PARP1 | poly(ADP-ribose)polymerase 1 |
ATP1A1 | sodium/potassium-transporting ATPase alpha-1 |
PAB | pseudoloric acid-B |
ERK | extracellular-signal regulated kinase |
MAPK | mitogen-activated protein kinase |
NF-κB | nuclear factor kappa-light chain-enhancer activated B-cell |
VDAC1 | voltage dependent anion selective channel 1 |
VDAC2 | voltage dependent anion channel 2 |
CD147 | cluster of differentiation 147 |
SLC3A2 | solute carrier family-3 member-2 |
MMP-7 | matrix metallopeptidase 7 |
MMP-2 | matrix metallopeptidase 2 |
MMP-9 | matrix metallopeptidase 9 |
PEA15 | proliferation and apoptosis adaptor protein-15 |
TPT1 | tumor protein, translationally controlled-1 |
CTSD | cathepsin D |
CRABP2 | cellular retinoic acid binding protein-2 |
CFL1 | cofilin 1 |
Myc | MYC proto-oncogene |
BHLH | transcription factor |
TNF | tumor necrosis factor |
CDK2 | cyclin dependent kinase 2 |
AKT | threonine kinase 1 |
PKA1 | p21-activated kinase-1 |
P70S6K | ribosomal protein S6 kinase β-1 |
iTRAQ | isobaric tags for relative and absolute quantification |
PKA | protein kinase-A |
C-myc | regulatory gene, locate in chromosome 8 |
BAX | BCL2 associated X-protein |
BCL2 | B-cell lymphoma 2 |
IngMeb | ingenol mebutate |
SLC25A20 | solute carrier family-25 member-20 |
EIF2 | eukaryotic translation initiation factor-2 |
EIF4 | eukaryotic translation ignition factor 4 |
p70S6K | ribosomal protein S6 kinase B1 |
mTOR | mechanistic target of ramamycin kinase |
NOP14 | nuclear protein 14 |
RPS27A | 40S ribosomal protein |
S1P | sphingosine-1-phosphate |
SPT | serin palmitoyltransferase |
SPTSSA | serine palmitoyltransferase complex-A |
SPTSSB | serine palmitoyltransferase complex-B |
HCT116 | colon cancer cells |
JURKAT | T-lymphocyte cells |
NT2 | cis-platin-resistant embryonal carcinoma cells |
TSGH-9201 | gastric cancer cells |
SMMG-7721 | liver cancer cells |
HSO578T | breast cancer cells |
P388 | lymphocytic leukemia cells |
HepG2 | liver cancer cells |
MOLT-4 | human acute lymphoblastic leukemia cells |
HL-60 | human leukemia cells |
MCF-7 | human breast cancer cells |
Conflicts of interest
There was no conflict of interest.
Acknowledgements
The authors acknowledge ICT-IOC, Bhubaneswar for providing necessary support. Rambabu Dandela thanks DST-SERB for Ramanujan fellowship (SB/S2/RJN-075/2016), Core research grant (CRG/2018/000782) and ICT-IOC startup grant. Pushparathinam Gopinath thanks DST-start-up research grant from DST-SERB (SRG/2019/001133).
References
- Press release N° 263, WHO, 12-sept, 2018.
- H. Sung, J. Ferlay, R. L. Siegel, M. Laversanne, I. Soerjomataram, A. Jemal and F. Bray, Ca-Cancer J. Clin., 2021, 71(3), 209–249 CrossRef PubMed
. - A. R. Omran, Milbank Meml. Fund Q., 1971, 49, 509–538 CrossRef CAS
. - M. Greenwell and P. K. S. M. Rahman, Int. J. Pharma Sci. Res., 2015, 6(10), 4103 CAS
. - P. Banerjee, J. Erehman, B. O. Gohlke, T. Wilhelm, R. Preissner and M. Dunkel, Nucleic Acids Res., 2015, 43(D1), D935–D939 CrossRef CAS PubMed
. - D. J. Newman and G. M. Cragg, J. Nat. Prod., 2012, 75, 311–335 CrossRef CAS PubMed
. - D. Dias, S. Urban and U. Roessner, Metabolites, 2012, 2(2), 303–336 CrossRef CAS PubMed
. - X. Wang, H. Zhang and X. Chen, Cancer Drug Resistance, 2019, 2(2), 141–160 Search PubMed
. - P. Fan and V. C. Jordan, Cancer Drug Resistance, 2019, 2, 198 Search PubMed
. - X. Zhao, T. Lwin, A. Silva, B. Shah, J. Tao, B. Fang and H. Jiang, Nat. Commun., 2017, 8(1), 1–15 CrossRef PubMed
. - G. Drewes and S. Knapp, Trends Biotechnol., 2018, 36(12), 1275–1286 CrossRef CAS PubMed
. - S. A. Sieber and B. F. Cravatt, Chem. Commun., 2006, 22, 2311–2319 RSC
. - P. Yang and K. Liu, Chem. Bio. Chem., 2015, 16(5), 712–724 CrossRef CAS PubMed
. - B. Lomenick, R. Hao, N. Jonai, R. M. Chin, M. Aghajan, S. Warburton and J. A. Wohlschlegel, Proc. Natl. Acad. Sci., 2009, 106(51), 21984–21989 CrossRef CAS PubMed
. - B. Lomenick, G. Jung, J. A. Wohlschlegel and J. Curr, Protoc. Chem. Biol., 2011, 3(4), 163–180 CrossRef PubMed
. - P. D. DeArmond, Y. Xu, E. C. Strickland, K. G. Daniels and M. C. Fitzgerald, J. Proteome Res., 2011, 10(11), 4948–4958 CrossRef CAS PubMed
. - D. M. Molina, R. Jafari, M. Ignatushchenko, T. Seki, E. A. Larsson, C. Dan and P. Nordlund, Science, 2013, 341(6141), 84–87 CrossRef CAS PubMed
. - J. M. Baskin, J. A. Prescher, S. T. Laughlin, N. J. Agard, P. V. Chang, I. A. Miller and C. R. Bertozzi, Proc. Natl. Acad. Sci., 2007, 104(43), 16793–16797 CrossRef CAS PubMed
. - C. E. Keohane, A. D. Steele, C. Fetzer, J. Khowsathit, D. Van Tyne, L. Moynié and W. M. Wuest, J. Am. Chem. Soc., 2018, 140(5), 1774–1782 CrossRef CAS PubMed
. - P. P. Geurink, L. M. Prely, G. A. Van der Marel, R. Bischoff and H. S. Overkleeft, Photoaffinity labeling in activity-based protein profiling, Springer, Berlin, Heidelberg, 2011, pp. 85–113 Search PubMed
. - B. Lee, W. Sun, H. Lee, H. Basavarajappa, R. S. Sulaiman, K. Sishtla and S. Y. Seo, Bioorg. Med. Chem. Lett., 2016, 26(17), 4277–4281 CrossRef CAS PubMed
. - J. R. Hill and A. A. Robertson, J. Med. Chem., 2018, 61(16), 6945–6963 CrossRef CAS PubMed
. - J. Wang, L. Gao, Y. M. Lee, K. A. Kalesh, Y. S. Ong, J. Lim and Q. Lin, Pharmacol. Ther., 2016, 162, 10–22 CrossRef CAS PubMed
. - M. H. Wright and S. A. Sieber, Nat. Prod. Rep., 2016, 33(5), 681–708 RSC
. - X. Chen, Y. Wang, N. Ma, J. Tian, Y. Shao, B. Zhu and J. Wang, Signal Transduction Targeted Ther., 2020, 5(1), 1–13 CrossRef PubMed
. - P. Gehrtz and N. London, Trends Pharmacol. Sci., 2021, 44(6), 434–447 CrossRef PubMed
. - W. P. Heal, T. T. Dang and E. W. Tate, Chem. Soc. Rev., 2011, 40(1), 246–257 RSC
. - N. C. Sadler and A. T. Wright, Curr. Opin. Chem. Biol., 2015, 24, 139–144 CrossRef CAS PubMed
. - S. M. Hacker, K. M. Backus, M. R. Lazear, S. Forli, B. E. Correia and B. F. Cravatt, Nat. Chem., 2017, 9(12), 1181 CrossRef CAS PubMed
. - S. Sakamoto and I. Hamachi, Anal. Sci., 2018, 18R003 Search PubMed
. - A. J. van der Zouwen, J. Lohse, L. H. Wieske, K. F. Hohmann, R. van der Vlag and M. D. Witte, Chem. Commun., 2019, 55(14), 2050–2053 RSC
. - A. E. Speers and B. F. Cravatt, Curr. Protoc. Mol. Biol., 2009, 1(1), 29–41 CrossRef PubMed
. - E. Pisha, H. Chai, I. S. Lee, T. E. Chagwedera, N. R. Farnsworth, G. A. Cordell and J. M. Pezzuto, Nat. Med., 1995, 1(10), 1046–1051 CrossRef CAS PubMed
. - Y. Tan, R. Yu and J. M. Pezzuto, Clin. Cancer Res., 2003, 9(7), 2866–2875 CAS
. - L. Huang, P. Ho, K. H. Lee and C. H. Chen, Bioorg. Med. Chem., 2006, 14(7), 2279–2289 CrossRef CAS PubMed
. - H. W. Cui, Y. He, J. Wang, W. Gao, T. Liu, M. Qin and Z. Yi, Eur. J. Med. Chem., 2015, 95, 240–248 CrossRef CAS PubMed
. - H. Guo, J. Xu, P. Hao, K. Ding and Z. Li, Chem. Commun., 2017, 53(69), 9620–9623 RSC
. - S. Fulda, Int. J. Mol. Sci., 2008, 9(6), 1096–1107 CrossRef CAS PubMed
. - S. Omura, Y. Iwai, A. Hirano, A. Nakagawa, J. Awaya, H. Tsuchiya and R. Asuma, J. Antibiot., 1977, 30(4), 275–282 CrossRef CAS PubMed
. - R. P. Joyce, J. A. Gainor and S. M. Weinreb, J. Org. Chem., 1987, 52(7), 1177–1185 CrossRef CAS
. - R. Bertrand, E. Solary, P. O'Connor, K. W. Kohn and Y. Pommier, Exp. Cell Res., 1994, 211(2), 314–321 CrossRef CAS PubMed
. - S. C. Meyer, C. D. Shomin, T. Gaj and I. Ghosh, J. Am. Chem. Soc., 2007, 129(45), 13812–13813 CrossRef CAS PubMed
. - W. P. Jencks, Proc. Natl. Acad. Sci., 1981, 78(7), 4046–4050 CrossRef CAS PubMed
. - A. Ricouart, J. C. Gesquiere, A. Tartar and C. Sergheraert, J. Med. Chem., 1991, 34(1), 73–78 CrossRef CAS PubMed
. - M. C. De Moraes, C. L. Cardoso and Q. B. Cass, Front. Chem., 2019, 7, 752 CrossRef CAS PubMed
. - J. J. Fischer, O. Y. Graebner, C. Dalhoff, S. Michaelis, A. K. Schrey, J. Ungewiss and M. Sefkow, J. Proteome Res., 2010, 9(2), 806–817 CrossRef CAS PubMed
. - H. Shi, X. Cheng, S. K. Sze and S. Q. Yao, Chem. Commun., 2011, 47(40), 11306–11308 RSC
. - X. Cheng, L. Li, M. Uttamchandani and S. Q. Yao, Chem. Commun., 2014, 50(22), 2851–2853 RSC
. - J. W. Tan, D. A. Israf and C. L. Tham, Front. Pharmacol., 2018, 9, 652 CrossRef PubMed
. - Y. Nakamura, C. Yoshida, A. Murakami, H. Ohigashi, T. Osawa and K. Uchida, FEBS Lett., 2004, 572(1), 245–250 CrossRef CAS PubMed
. - H. O. Hemn, H. Hazilawati, M. M. Noordin, S. R. Heshu and A. B. Zuki, Open. Conf. Proc. J., 2013, 4(1), 61–64 CrossRef CAS
. - A. Ghasemzadeh, H. Z. Jaafar, A. Rahmat and M. K. Swamy, Chem. Cent. J., 2017, 11(1), 1–10 CrossRef PubMed
. - T. Sithara, B. P. Dhanya, K. B. Arun, S. Sini, M. Dan, R. Kokkuvayil Vasu and P. Nisha, J. Agric. Food Chem., 2018, 66(3), 602–612 CrossRef CAS PubMed
. - S. Girisa, B. Shabnam, J. Monisha, L. Fan, C. E. Halim, F. Arfuso and A. B. Kunnumakkara, Molecules, 2019, 24(4), 734 CrossRef CAS PubMed
. - K. Ohnishi, K. Irie and A. Murakami, Biosci., Biotechnol., Biochem., 2009, 73(8), 1905 CrossRef CAS PubMed
. - K. A. Kalesh, J. A. Clulow and E. W. Tate, Chem. Commun., 2015, 51(25), 5497–5500 RSC
. - N. Tsuge, K. Furihata, K. Shin-Ya, Y. Hayakawa and H. Seto, J. Antibiot., 1999, 52(5), 505–507 CrossRef CAS PubMed
. - S. V. Torti and F. M. Torti, Nat. Rev. Cancer, 2013, 13(5), 342–355 CrossRef CAS PubMed
. - A. Lawen and D. Lane, Antioxid. Redox Signaling, 2013, 18(18), 2473–2507 CrossRef CAS PubMed
. - M. Kawatani, M. Muroi, A. Wada, G. Inoue, Y. Futamura, H. Aono and H. Osada, Sci. Rep., 2016, 6, 38385 CrossRef CAS PubMed
. - Y. Y. Tae, Travel around near Seoul, 2005, Nexus BOOKS, Seoul, p. 131 Search PubMed
. - J. Yang, Y. He, Y. Li, X. Zhang, Y. K. Wong, S. Shen and J. Wang, Pharmacol. Ther., 2020, 107697 CrossRef CAS PubMed
. - Y. Tu, Nat. Med., 2011, 17(10), 1217–1220 CrossRef CAS PubMed
. - A. K. Das, Ann. Med. Health. Sci. Res., 2015, 5(2), 93–102 CrossRef CAS PubMed
. - G. Q. Chen, F. A. Benthani, J. Wu, D. Liang, Z. X. Bian and X. Jiang, Cell Death Differ., 2020, 27(1), 242–254 CrossRef CAS PubMed
. - T. Eichhorn, S. Schloissnig, B. Hahn, A. Wendler, R. Mertens, W. D. Lehmann and T. Efferth, Mol. BioSyst., 2012, 8(4), 1311–1318 RSC
. - Y. Zhou, W. Li and Y. Xiao, ACS Chem. Biol., 2016, 11(4), 882–888 CrossRef CAS PubMed
. - X. Zhang, X. Li, H. Sun, X. Wang, L. Zhao, Y. Gao and S. Zeng, J. Med. Chem., 2013, 56(1), 276–292 CrossRef CAS PubMed
. - L. J. Yang and Y. Chen, Acta Pharmacol. Sin., 2013, 34(2), 191–198 CrossRef CAS PubMed
. - K. H. Yim, T. L. Prince, S. Qu, F. Bai, P. A. Jennings, J. N. Onuchic and L. Neckers, Proc. Natl. Acad. Sci., 2016, 113(33), E4801–E4809 CrossRef CAS PubMed
. - Q. Yue, L. Feng, B. Cao, M. Liu, D. Zhang, W. Wu and D. Guo, Mol. Cell. Proteomics, 2016, 15(1), 26–44 CrossRef CAS PubMed
. - N. Kudo, N. Matsumori, H. Taoka, D. Fujiwara, E. P. Schreiner, B. Wolff and S. Horinouchi, Proc. Natl. Acad. Sci., 1999, 96(16), 9112–9117 CrossRef CAS PubMed
. - Y. Zhou, W. Li, X. Zhang, H. Zhang and Y. Xiao, Chem. Commun, 2016, 52(97), 14035–14038 RSC
. - N. Shabek and A. Ciechanover, Cell Cycle, 2010, 9(3), 523–530 CrossRef CAS PubMed
. - A. H. Merrill, J. Biol. Chem., 2002, 277(29), 25843–25846 CrossRef CAS PubMed
. - G. Han, S. D. Gupta, K. Gable, S. Niranjanakumari, P. Moitra, F. Eichler and T. M. Dunn, Proc. Natl. Acad. Sci., 2009, 106(20), 8186–8191 CrossRef CAS PubMed
. - D. G. Hoch, D. Abegg, J. T. Hannich, D. Pechalrieu, A. Shuster, B. G. Dwyer and A. Adibekian, Cell Chem. Biol., 2020, 27(5), 586–597 CrossRef CAS PubMed
. - Q. Yue, H. Zhen, M. Huang, X. Zheng, L. Feng, B. Jiang and D. Guo, PLoS One, 2016, 11(7), e0159034 CrossRef PubMed
. - Z. Li, L. L. L. Qian, J. S. Bernhammer, J. C. Huynh, H. V. Lee and S. Q. Yao, Angew. Chem., Int. Ed., 2016, 55, 2002–2006 CrossRef CAS PubMed
. - T. Helleday, E. Petermann, C. Lundin, B. Hodgson and R. A. Sharma, Nat. Rev. Cancer, 2008, 8(3), 193–204 CrossRef CAS PubMed
. - N. Ma, Z. M. Zhang, J. S. Lee, K. Cheng, L. Lin, D. M. Zhang and Z. Li, ACS Chem. Biol., 2019, 14(12), 2546–2552 CrossRef CAS PubMed
. - J. X. Yao and X. Y. Lin, Acta Chim. Sin., 1982, 40(5), 385–393 CAS
. - M. O. Hamburger, H. L. Shieh, B. N. Zhou, J. M. Pezzuto and G. A. Cordell, Magn. Reson. Chem., 1989, 27(11), 1025–1030 CrossRef CAS
. - T. Li, V. K. W. Wong, X. Q. Yi, Y. F. Wong, H. Zhou and L. Liu, J. Cell. Biochem., 2009, 108(1), 87–95 CrossRef CAS PubMed
. - J. H. Yu, H. J. Wang, X. R. Li, S. I. Tashiro, S. Onodera and T. Ikejima, Acta Pharmacol. Sin., 2008, 29(9), 1069–1076 CrossRef CAS PubMed
. - T. Sarkar, T. L. Nguyen, Z. W. Su, J. Hao, R. Bai, R. Gussio and E. Hamel, Biochem. Pharmacol., 2012, 84(4), 444–450 CrossRef CAS PubMed
. - Y. Zhou, Z. Di, X. Li, Y. Shan, W. Li, H. Zhang and Y. Xiao, Chem. Commun., 2017, 53(62), 8671–8674 RSC
. - H. Nakamura, J. I. Kobayashi, Y. Ohizumi and Y. Hirata, Tetrahedron Lett., 1982, 23(52), 5555–5558 CrossRef CAS
. - S. A. Dyshlovoy, I. Naeth, S. Venz, M. Preukschas, H. Sievert, C. Jacobsen and M. Krepstakies, J. Proteome Res., 2012, 11(4), 2316–2330 CrossRef CAS PubMed
. - S. A. Dyshlovoy, S. Venz, L. K. Shubina, S. N. Fedorov, R. Walther, C. Jacobsen and F. Honecker, J. Proteomics, 2014, 96, 223–239 CrossRef CAS PubMed
. - H. C. Kuo, Y. R. Kuo, K. F. Lee, M. C. Hsieh, C. Y. Huang, Y. Y. Hsieh and C. C. Chen, Cell. Physiol. Biochem., 2017, 43(1), 195–208 CrossRef CAS PubMed
. - C. C. Lu, W. S. Huang, K. F. Lee, K. C. Lee, M. C. Hsieh, C. Y. Huang and S. Y. Tung, J. Funct. Foods, 2016, 21, 474–484 CrossRef CAS
. - Z. X. Peng, Y. Wang, X. Gu, Y. Xue, Q. Wu, J. Y. Zhou and C. Yan, Metabolomics, 2015, 11(3), 636–656 CrossRef CAS
. - Z. X. Peng, Y. Wang, X. Gu, Y. Y. Wen and C. Yan, Biomed. Chromatogr., 2013, 27(12), 1759–1766 CrossRef CAS PubMed
. - L. R. Zieske, J. Exp. Bot., 2006, 57(7), 1501–1508 CrossRef CAS PubMed
. - Z. Peng, Y. Wang, J. Fan, X. Lin, C. Liu, Y. Xu and C. Su, Sci. Rep., 2017, 7(1), 1–16 CrossRef PubMed
. - V. Prajapati and B. Barankin, Can. Fam. Physician, 2008, 54(5), 699 Search PubMed
. - R. S. Fallen and M. Gooderham, Skin Therapy Lett., 2012, 17(2), 1–3 Search PubMed
. - Withdrawal of the marketing authorisation in the European Union. 20 February 2020 EMA/91080/2020 EMEA/H/C/002275.
- C. G. Parker, C. A. Kuttruff, A. Galmozzi, L. Jørgensen, C. H. Yeh, D. J. Hermanson and B. Nørremark, ACS Cent. Sci., 2017, 3(12), 1276–1285 CrossRef CAS PubMed
. - M. Salem, S. Rohani and E. R. Gillies, RSC Adv., 2014, 4(21), 10815–10829 RSC
. - J. Ravindran, S. Prasad and B. B. Aggarwal, AAPS J., 2009, 11(3), 495–510 CrossRef CAS PubMed
. - J. Wang, J. Zhang, C. J. Zhang, Y. K. Wong, T. K. Lim, Z. C. Hua and Q. Lin, Sci. Rep., 2016, 6(1), 1–8 CrossRef PubMed
. - H. Liu, Y. Z. Liu, F. Zhang, H. S. Wang, G. Zhang, B. H. Zhou and J. Du, Mol. BioSyst., 2014, 10(6), 1320–1331 RSC
. - D. Chauhan, E. Bartok, M. M. Gaidt, F. J. Bock, J. Herrmann, J. M. Seeger and R. Müller, Cell Rep., 2018, 25(9), 2354–2368 CrossRef CAS PubMed
. - V. C. Kirsch, C. Orgler, S. Braig, I. Jeremias, D. Auerbach, R. Müller and S. A. Sieber, Angew. Chem., Int. Ed., 2020, 59(4), 1595–1600 CrossRef CAS PubMed
. - M. Malsy, D. Bitzinger, B. Graf and A. Bundscherer, Eur. J. Med.Res., 2019, 24(1), 1–8 CrossRef PubMed
.
|
This journal is © The Royal Society of Chemistry 2021 |
Click here to see how this site uses Cookies. View our privacy policy here.