DOI:
10.1039/D2MH01056A
(Communication)
Mater. Horiz., 2023,
10, 908-917
A variable-stiffness and healable pneumatic actuator†
Received
25th August 2022
, Accepted 15th December 2022
First published on 15th December 2022
Abstract
Pneumatic-powered actuators are receiving increasing attention due to their widespread applications. However, their inherent low stiffness makes them incompetent in tasks requiring high load capacity or high force output. On the other hand, soft pneumatic actuators are susceptible to damage caused by over-pressuring or punctures by sharp objects. In this work, we designed and synthesized a coordination adaptable network (PETMP-AIM-Cu) with high mechanical rigidity (Young's modulus of 1.9 GPa and elongation <2% before fracturing) as well as excellent variable stiffness property (soft-rigid switching ability σ as high as 3
268
000 when ΔT = 90 °C). Combining PETMP-AIM-Cu with a self-healing elastomer based on dynamic disulfide bonds (LP-PDMS), we fabricated a new pneumatic actuator which shows high load capacity at room temperature, but can also easily deform upon heating and thus can be actuated pneumatically. Benefiting from the excellent self-healing ability of PETMP-AIM-Cu and LP-PDMS, the entire pneumatic actuator can still be actuated after being cut and healed. Such a variable-stiffness and healable pneumatic actuator would be useful for complex environmental applications.
New concepts
Soft robotics, which are inherently safe and adapt well to unstructured environments due to their softness, are expected to satisfy the needs of robots that interact safely with humans. However, for soft robotic arms, they are prone to various damage types and poorly suited for tasks requiring high load capacity or high force output. In this work, we fabricated a new pneumatic robotic arm by combining a coordination-adaptive network with high mechanical rigidity as well as excellent variable stiffness property and a self-healing elastomer based on disulfide bonds. The pneumatic robotic arm shows high load capacity at room temperature but can be actuated pneumatically upon heating, thus can bridge the gap between traditional rigid robotics and emerging soft robotics. Moreover, it can be thermally healed after damaging, thus can prolong its service time. The combination of variable-stiffness and self-healing properties will greatly broaden the application of soft robotics in complex environments.
|
Introduction
Robots are widely used in different fields from industries to daily life, aiming to boost production speed, minimize human error, avoid accidents, etc. Traditional robots are made of stiff materials actuated by motors, cables, and linear actuators, thus they are fast, precise, and capable of applying high forces. However, they are unable to deform elastically and adapt their shape to external constraints and obstacles. On the other hand, soft robots made of intrinsically soft and/or flexible materials have gained great attention due to their capability of adapting to complex environments and building safe interactions with humans.1–4 They have found various applications in robotic manipulators,5,6 crawling and swimming robots,7,8 minimally invasive surgery devices,9 and other biomedical systems.10–12 Pneumatic-powered robotics, which are actuated by air pressurization or inflation, are particularly important due to their small-form-factor and high-force capabilities as well as their low costs compared to servo-electrics. However, two main challenges remain to be addressed for pneumatic robotics. First, the inherent low stiffness of their constituent materials (for example, silicone elastomers) makes pneumatic robotic systems incompetent in tasks requiring high load capacity or high force output such as grasping and manipulation of heavy objects,13,14 hand rehabilitation,15 and precise surgical operations.9,12,16 Second, pneumatic robotics are vulnerable to sharp objects or over-pressure.17 The resulting damages cause leaking of the gas and lead to decrease in actuation efficiency and performance, and eventually to failure of the component at a limited lifetime.
Many stiffness-tunable soft robots that show high load capacity as well as shape adaptability, and thus can fulfill tasks in diverse environments, have been reported.18 A series of materials, including shape memory polymers (SMPs),19,20 electrorheological materials,21 low melting point alloys (LMPAs),22,23 granular or laminar jamming structures,24 and elastomers filled with electro/magneto-active liquids,25 have been utilized in soft robotics. These materials can change from rigid to soft state under external stimuli, thus bridging the gap between traditional rigid robotics and emerging soft robotics. In spite of these successes, the design and building of adaptable robots are still not satisfactory due to the difficulty in synthesizing polymeric materials that simultaneously possess high rigidity at room temperature and excellent soft-rigid switching ability upon gentle heating. Generally, high rigidity at room temperature requires the crosslinking interactions to be strong enough to overcome the external force without breaking, while an excellent soft-rigid switching ability demands dynamic chemistry that will exhibit sensitive response to temperature change in a suitable range. Therefore, most of the present materials are either very rigid at room temperature but do not show plasticity or only soften at very high temperature, or can be readily softened at mild conditions but show low stiffness at room temperature. Moreover, realizing healing in rigid materials is difficult due to the slow diffusion dynamics. Therefore, although soft robotics that are able to heal microscopic and macroscopic damage have been demonstrated by utilizing self-healing elastomers,17,26–28 developing both variable-stiffness and healable pneumatic robotic is still very challenging.
In order to combine rigidity, healability and soft-rigid switching ability in a single polymer, it is important to choose a dynamic bond that maintains a high binding constant at room temperature, while upon heating, the equilibrium of the reversible binding reactions will sensitively move to its dissociated state, decreasing the crosslink density and making the material soft and easy to process. However, there is a trade-off problem between mechanical rigidity and soft-rigid switching temperature. Weaker bonds usually can dissociate at lower temperature, but they cannot form polymers with satisfactory mechanical rigidity. Stronger bonds can form highly rigid polymers, but they tend to dissociate at higher temperature. In previous studies by us and Aida et al.,29,30 it has been shown that low-molecular-weight polymers, when cross-linked by dense hydrogen bonds, yield mechanically robust materials. Owning to the dissociation of hydrogen bonds which generate the flexible low-molecular-weight monomers, the polymer turned into soft and viscoelastic state with very low viscosity, thus exhibiting excellent variable stiffness property and efficient thermal healing behavior. We therefore envisage that if the hydrogen bonds are substituted by stronger coordination bonds, polymer networks with higher mechanical strength can be obtained. Meanwhile, the coordination bonds are natively reversible and will undergo dissociation at elevated temperature, thus showing better variable stiffness property and favorable for variable-stiffness and healable robotics.
Herein, we report a coordination adaptable network obtained by crosslinking a low-molecular-weight imidazole-containing monomer with Cu(II). The as-prepared coordination adaptable network (denoted as PETMP-AIM-Cu) shows high mechanical rigidity (Young's modulus of 1.9 GPa and elongation <2% before fracturing) as well as excellent variable stiffness property. The soft–rigid switching ability σ, defined as
, can reach 3
268
000 when ΔT = 90 °C. By combining PETMP-AIM-Cu with a self-healing elastomer base on dynamic disulfide bonds (LP-PDMS), we fabricated a new pneumatic actuator. The pneumatic actuator shows high load capacity (3–4 times compared to the self-weight) at room temperature, but can also easily deform upon heating and thus can be actuated pneumatically. Upon tunning the electric voltages, the stiffness of the pneumatic actuator can be changed in a wide range, thus the different force output can be realized for grasping, picking, and manipulation of both soft and hard objects. As both the variable-stiffness PETMP-AIM-Cu and elastomer LP-PDMS have excellent self-healing ability, the entire pneumatic actuator can still be actuated after being cut and healed at 70 °C. Such a variable-stiffness and healable pneumatic actuator would be useful for various applications.
Results and discussion
Materials design and characterization
Generally, the mechanical strength of a polymer is dependent on the bond energy and density of crosslinking sites. Strong crosslinking interactions can lead to stiff polymers but their stiffness are hard to change due to the poor reversibility. Weak and dynamic crosslinking interactions are efficient for constructing variable-stiffness materials but often bear the disadvantage of low mechanical strength. According to the previous study from our group and others,29,30 decreasing the chain segment length, or even crosslinking low-molecular-weight monomers instead of a long polymer chain can lead to dynamic polymers with high stiffness due to the high crosslinking density. Regarding the soft-rigid switching ability, adopting low-molecular-weight monomers can lead to another advantage: the low-molecular-weight ligands have lower entanglements, thus the mechanical strength of the resulting polymer is dominantly determined by the crosslinking interactions. Upon heating, the equilibrium of the dynamic coordination bond moves to its dissociated state, and thus the low-molecular-weight monomer can be de-crosslinked and released from the network, making the material softer and softer. Upon cooling, the monomers are re-crosslinked and thus recovering the material stiffness. Therefore, we selected pentaerythritol tetra (3-mercaptopropionate) (PETMP) as our design owing to its flexibility and multi-functionality.31,32 Imidazole group was used as the coordination group because of its ability to form dynamic coordination bonds with many metal ions.33,34 Some studies have proved that the coordination bonds between imidazole and Cu(II) show dynamic kinetics.35,36 Therefore, we choose imidazole and Cu(II) as our design for dynamic chemistry.
PETMP was first modified with imidazole group by thiol-ene click reaction to generate PETMP-AIM.37 By reacting PETMP-AIM with Cu(BF4)2·6H2O, we obtained a coordination adaptable network containing Cu(II)-imidazole interactions (Fig. 1a and Fig. S1, ESI†). More detailed synthesis and characterizations can be found in the ESI† (Fig. S2–S4). From the test results of isothermal calorimetric titration (ITC) on PETMP-AIM-Cu, the association constant (Ka) was 1.78 × 106 M−1 (Fig. 1b and Table S1, ESI†), which is quite small compared to general coordination bonds but is still higher than hydrogen bond.38,39 Mass spectrum of model complexes of 1-methylimidazole (L1) or 1-allylimidazole (L2) and Cu(II) were performed. As shown in Fig. 1c, d and Fig. S5, S6 (ESI†), after mixing [Cu(L1)4](BF4)2 and [Cu(L2)4](BF4)2 in 1
:
1 molar ratio in water at 70 °C, a series of intermediate products from ligand exchange reaction between [Cu(L1)4](BF4)2 and [Cu(L2)4](BF4)2 were observed, confirming the dynamics of the coordination bonds between imidazole and Cu(II). The crosslinked PETMP-AIM-Cu polymer was obtained as solid powder and it can be hot-pressed into various block samples (Fig. 1e). According to the FT-IR spectra (Fig. S7, ESI†) and the crystal structures of the Cu(II)-imidazole complexes reported in the literature,40,41 the coordination complexes formed by Cu(II) with four N atoms from four imidazole groups ligands are the dominant crosslinking sites within the polymer system. Furthermore, as shown in Table S1 (ESI†), the tendency of Cu(II) binding to PETMP-AIM ligand to form a 1
:
1 complex was confirmed by ITC titrations (the complex stoichiometry N = 1.10). Meanwhile, the results of X-ray photoelectron spectroscopy (XPS) suggested that Cu(II) tended to interact with N atoms instead of O atoms (Fig. S8, ESI†). The small-angle X-ray scattering (SAXS) analysis (Fig. S9, ESI†) and energy dispersive X-ray spectroscopy (EDS) data (Fig. S10, ESI†) showed that there was no apparent aggregation of the Cu(II)-imidazole complexes.
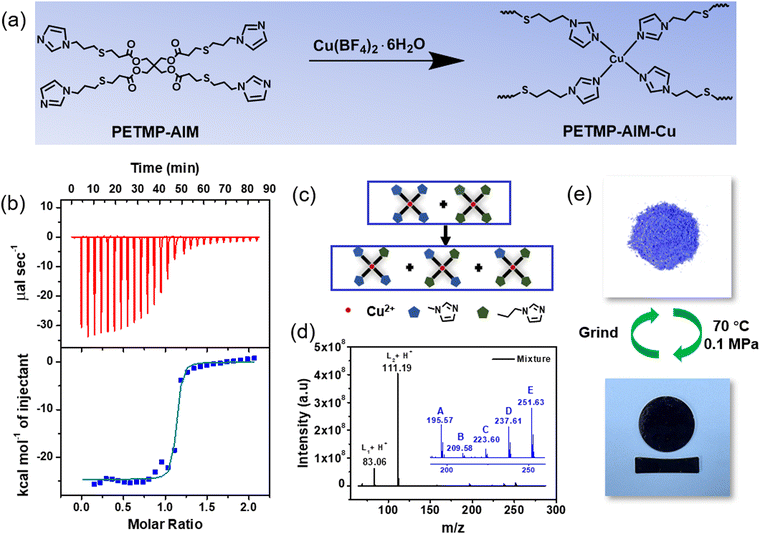 |
| Fig. 1 Preparation and characterizations of PETMP-AIM-Cu and model complexes. (a) Synthesis and structure of PETMP-AIM-Cu. (b) The ITC titration data of the PETMP-AIM with Cu(BF4)2·6H2O in anhydrous ethanol at 25 °C. (c) Scheme diagram of the ligand exchange process of Cu(II)-imidazole complexes. (d) ESI-MS spectrum for the mixture of [Cu(L1)4](BF4)2 and [Cu(L2)4](BF4)2 in 1 : 1 molar ratio. The inserted picture is partial enlarged view from m/z = 190 to m/z = 260. (A–E) Represent Cu(L1)42+, Cu[(L1)3(L2)]2+, Cu[(L1)2(L2)2]2+, Cu[(L1) (L2)3]2+, Cu(L2)42+, respectively. (e) Picture showing that the powder of PETMP-AIM-Cu can be reversibly hot-pressed into various blocks at 70 °C. | |
The differential scanning calorimetry (DSC) curve showed that the glass-transition temperature (Tg) of the polymer was about 56 °C (Fig. S11, ESI†). No melting point was found in the DSC curve (−10 to 115 °C), indicating an amorphous structure, which was consistent with the result of X-ray diffraction (XRD) (Fig. S12, ESI†). According to the thermal gravimetric analysis (TGA) data, the polymer was stable below 200 °C (Fig. S13, ESI†). Dissolution experiments demonstrated that the polymer had good solvent resistance to most solvents (Fig. S14, ESI†). These features indicate that the polymer behaves like rigid thermoset polymer at room temperature.
Rigid-soft switching properties
The temperature dependence of the mechanical properties of PETMP-AIM-Cu was investigated. As shown in Fig. 2a and Table S2 (ESI†), the material owns a high flexural modulus (1859 ± 178 MPa) at room temperature. Only slight flexural strain (1.52 ± 0.05%) before fracturing was observed under flexural stress of 23.58 ± 3.09 MPa, manifesting the rigid feature. Increasing temperature can significantly influence the mechanical properties. Results from tensile-stress tests show that the tensile modulus and the tensile strength of the material decrease while the elongation of the material increases upon heating (Fig. S15, ESI†). At 60 °C, the material can withstand more than 10% elongation before fracturing (Fig. 2a). The temperature-dependent rheological measurements show that the storage modulus begins to decrease dramatically as the temperature increases to 50 °C and reaches a plateau as the temperature approaches 110 °C (Fig. 2b). The storage modulus is 621 MPa at 30 °C and 190 Pa at 120 °C, corresponding to a soft-rigid switching ability σ over six orders of magnitude (3
268
000). Moreover, as shown in Fig. 2c, we performed two heating and cooling cycles for the temperature-sweep rheology measurements. The four curves almost overlapped, indicating that the change of mechanical properties with temperature is fully reversible. Fig. S16 (ESI†) shows the frequency-sweep rheology results at different temperatures. The material features a classic dissociative network rheological behavior. The increases in temperature over 60 °C result in a dramatic decrease of the plateau shear modulus owing to the decrease in crosslink density,42,43 which can be further confirmed by the stress relaxation tests for PETMP-AIM-Cu (Fig. S17, ESI†). The mechanism behind the excellent soft-rigid switching ability of the material is illustrated in Fig. 2d. At room temperature, the mobility of molecular segments is limited and the bulk material is hard and shows a very high mechanical strength due to the dense and strong crosslinking coordination bonds between imidazole and Cu(II). The coordination bonds will be dynamic and exchangeable as temperature elevates, making the material soft. Upon further heating, the coordination bonds fully dissociate and the low-molecular-weight ligands of PETMP-AIM will be released from the coordination adaptable network and the materials will then turn into viscous state. When the temperature cools down, the equilibrium of the coordination reaction between imidazole and Cu(II) shifts to the associated state. Thus the dissociated network at high temperature can perfectly convert back to its previous crosslinked state without impairing the network integrity. By utilizing moderate but dense coordination bonds to crosslinking low-molecular-weight ligands, here we achieved a combination of high rigidity at room temperature and a large change in mechanical strength upon gentle heating. Such performance is superior among literatures (Fig. 2e and Table S3, ESI†).
 |
| Fig. 2 Mechanical properties of PETMP-AIM-Cu. (a) Three-point flexural stress–strain curves of PETMP-AIM-Cu under various temperature. (b) Temperature-dependent rheology measurements of PETMP-AIM-Cu. (c) Cyclic temperature-sweep rheology measurements of PETMP-AIM-Cu (with the temperature changing rate of 2 °C min−1). (d) Schematic diagram of the polymer network at different temperatures. (e) A comparison of the maximum storage modulus at room temperature and the soft-rigid switching ability σ for stiffness-variable materials in the literature and in our work. References to the existing materials are listed in Table S3 (ESI†). (f) Flexural stress–strain curves of PETMP-AIM-Cu before and after healing at 70 °C for different time. (g) Construction of a podium through thermal healing of bulk samples. | |
The excellent reversibility of the coordination adaptable network leads to various dynamic properties of PETMP-AIM-Cu. As shown in Fig. S18 and Table S2 (ESI†), the rigid material is reprocessable and can still maintain similar flexural properties (including flexural strain before breaking, flexural strength, and flexural modulus) to the pristine one, even after being reprocessed for four times. As the materials turn into viscous state upon heating and then turn back into rigid networks upon cooling, the polymer can be 3D printed into various shapes (such as cross and NJU, see Fig. S19, ESI†), thus fabricating complicated structures or devices through 3D-printing is possible. Moreover, benefiting from the fully reversible dynamic network, the material also possesses good thermal healing properties in mild conditions. As shown in Fig. 2f and Fig. S20 (ESI†), after being placed at 70 °C for 6 h, the damaged material can be healed and recover its original mechanical properties with over 96% healing efficiency (Table S4, ESI†). Optical microscopic images (Fig. S21, ESI†) evidence the disappearance of crack on the damaged material after being healed at 70 °C for 6 h. Fig. S22 and Table S5 (ESI†) show that the self-healing efficiency increases with increasing healing temperature. Heating to 60 °C and 70 °C significantly enhances the healing efficiency, which agrees well with the temperature-dependent rheological measurements, in which at 60 °C, the modulus begins to decrease, and at 70 °C, the material shows a much significant decrease in storage modulus compared with that at 60 °C. The thermal healing ability can also be used to connect different samples and construct functional architectures. As shown in Fig. 2g, three pieces of specimens can be thermally healed together to construct a podium, which can easily withstand 500 grams of weight.
Fabrication of pneumatic robotic actuators
A key component of the pneumatic actuator is the elastic body which can be actuated upon air pressurization or inflation. As PETMP-AIM-Cu is rigid at room temperature and viscous upon heating, it is not suitable for functioning as the body material.
Therefore, another elastic and healable polymer was needed. For an ideal body material, the viscoelastic behavior should be suppressed to avoid undesired phenomena like stress–strain hysteresis and creep, which have a negative effect on the energy efficiency and the dynamics of the soft robotic components. Hence, the elastic recovery should be fast and complete. However, fully elastic and self-healing polymers are rare in the literature,44–46 as dynamic bonds favorable for self-healing generally break upon stretching but need time to reform after release. Here, we choose the polymer obtained from the reaction between thiol-terminated polysulfide (LP3) and epoxy-terminated polydimethylsiloxane (epoxy-PDMS), as disulfide is a well-known dynamic bond and the reaction between thiol/hydroxyl and epoxy can lead to three-dimensional crosslinked networks which is able to improve the elasticity (Fig. 3a). The resulting polymer (denoted as LP-PDMS) was characterized by IR spectroscopy (Fig. S23, ESI†). LP-PDMS shows good elasticity and small stress–strain hysteresis (Fig. 3b), which should be due to the three-dimensional crosslinked structure as it can maintain the network integrity even when part of the disulfide bonds are broken. To further investigate the antifatigue behavior and reliability, the LP-PDMS elastomer was subjected to 100 consecutive loading–unloading cycles at a constant strain of 50% (Fig. 3c). It also shows impressive puncture-resistant behavior with a maximum load stress of 8.9 N at puncture speed of 50 mm min−1 (Fig. 3d). The puncture energy is calculated to be 68.35 ± 0.62 mJ according to the literature.47 When damaged by cutting, LP-PDMS can self-heal upon gentle heating. As shown in Fig. 3e and Fig. S24 and S25 (ESI†), the healing efficiency was 97.65% after 4 h at 70 °C and the healed sample can bear a load of 500 g, suggesting excellent self-healing behavior. According to the stress relaxation curves (Fig. S26, ESI†), the elastomers relaxed faster at higher temperatures, demonstrating that the network possesses much better dynamic exchanges upon heating. Meanwhile, the linear correlation of ln(τ) with diverse temperatures was constructed and the apparent activation energy (Ea) of the LP-PDMS elastomer was calculated according to the Arrhenius equation.48 An apparent activation energy (Ea) of 49.14 kJ mol−1 was obtained, which is relatively low as compared to common thermal healing polymers,32 confirming the excellent self-healing behavior upon gentle heating. Such features are favorable for the body material of pneumatic actuator.
 |
| Fig. 3 Fabrication of the pneumatic actuator. (a) Synthesis and structure of LP-PDMS elastomer. (b) Successive tensile loading–unloading curves of the LP-PDMS elastomer as stretching to different strains. (c) Successive loading–unloading curves of the LP-PDMS elastomer under the strain of 50% for 100 cycles. (d) Puncture curves under the deformation rate of 50 mm min−1 (the thickness of the film is 0.8 mm). (e) Stress–strain curves of the original and self-healed specimens after different healing times at 70 °C. (f) Schematic diagram of the structure and assembly of the pneumatic actuator. (g) Picture showing the actual pneumatic actuator obtained after splicing. | |
With the elastic and self-healing body material, a variable-stiffness pneumatic actuator was then fabricated. As depicted in Fig. 3f, the pneumatic actuator was constructed by integrating five main parts: inflatable chambers that will expand when inflated, the main body containing an airway that accepts inflatable chambers, a strain-limiting layer that also acts as a gas barrier, a variable-stiffness layer composed of PETMP-AIM-Cu, and a heating layer sprayed with copper paste. These components were stacked together and spliced through thermal healing at 70 °C for 4 h. The interfacial toughness between LP-PDMS and PETMP-AIM-Cu is not high (36.3 J m−2). Actually, PETMP-AIM-Cu can be dislodged from LP-PDMS if they are simply affixed together. However, in our device, the PETMP-AIM-Cu was encapsulated in LP-PDMS matrix, so even if the interfacial toughness is not high, the material will not be displaced or peeled off and will not affect the normal work of the actuator. Compared to the common molding process for fabricating pneumatic actuators, our method through self-healing provides more degree of freedom to design personalized structures. The actual actuator obtained by splicing is described below as shown in Fig. 3g. Meanwhile, another pneumatic actuator without the variable-stiffness layer was fabricated for comparison.
Performance of variable-stiffness and healable pneumatic actuator
Due to the presence of rigid PETMP-AIM-Cu polymer, the pneumatic actuators can withstand a heavy load (1600 g, Fig. 4a) at room temperature. In contrast, the pneumatic actuators without the rigid layer will severely deform upon loading (Fig. 4b). However, the pneumatic actuator with rigid layer cannot be actuated through air pressurization or inflation. This is the dilemma for most actuators as they are either rigid but undeformable or environment adaptive but cannot provide high force output. Here, with our variable-stiffness materials, we can combine both rigid and soft features into a single pneumatic actuators and thus bridge the gap between traditional rigid robotics and emerging soft robotics. As shown in Fig. 4c, we can control the temperature of the pneumatic actuators by controlling the voltage. Under the voltage of 1 V, the temperature can hardly rise, but when the voltage reaches 4 V, the maximum temperature can reach 100 °C. At 5 V, the temperature of the heating layer can reach more than 100 °C within 13 seconds. The different temperature upon applying different voltages endows the pneumatic actuators with different rigidity. As a result, the actuator can be actuated at different voltages for different purposes. We first heat the pneumatic actuators for 1 minute at 5 V and make the variable-stiffness layer turn into soft state. When the pneumatic actuators are pressurized, the chambers of the main body begin to expand, the inextensible strain-limiting layer then drives the embedded variable-stiffness layer to bend and deform. In this condition, the actuator can pick up light and soft objects such as eggs or tennis balls, just like common robotic arms (Fig. 4d). In addition, when the air pressure is removed, the pneumatic actuators can return to its original shape due to the elasticity of LP-PDMS. However, it is difficult for the actuator to pick up heavy objects because the variable-stiffness layer is too soft (Movie S1, ESI†). If we cool down the variable-stiffness layer in the bending state, the shape of the actuator can be fixed even after pressurization. The actuator can overcome the gravity of heavy objects through the rigidity of the variable stiffness layer, so the actuator can pull up heavy objects. Since the actuator has different stiffness at different temperature, different force output can be realized by heating voltage and heating time. As shown in Fig. 4d, the actuators can pick up a cola, an apple, and a 500 g weight (Movie S2, ESI†). Principally, such a variable-stiffness pneumatic actuator can be used to perform different tasks in different environments. The speed of the temperature-tuned stiff-to-soft transition in grasping objects with different weight is a problem to be solved. Further research to accelerate the heating and cooling process is necessary to make such pneumatic actuators useful in practical applications.
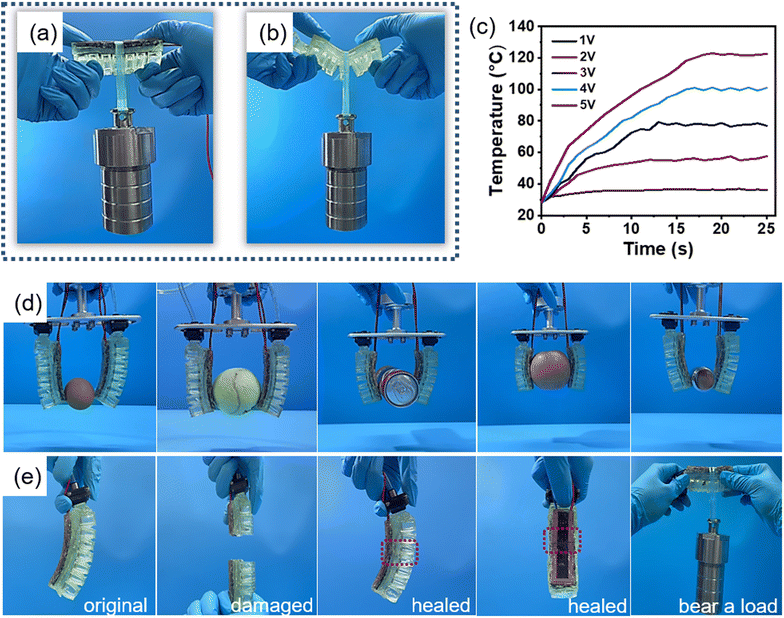 |
| Fig. 4 Performance of Variable-stiffness and healable Pneumatic actuator. (a) Picture showing that the pneumatic actuator with the variable-stiffness layer can withstand a heavy load (1600 g). (b) Picture showing that the pneumatic actuator without the variable-stiffness layer will severely deform upon loading. (c) The temperature change of pneumatic actuator upon applying different voltages for different time. (d) Pictures showing that the pneumatic actuator can pick up different objects with different weights. (e) Pictures showing that the pneumatic actuator can be healed upon cutting integrally. | |
As both the variable-stiffness PETMP-AIM-Cu polymer and elastic LP-PDMS polymer have excellent self-healing ability, the entire pneumatic actuator can be healed upon damaging. In order to better show the integral repair ability of the device, we cut the pneumatic actuator into two segments (Fig. 4e and Movie S3, ESI†). The two segments were then brought together and placed into a 70 °C oven for 6 hours for self-healing. Although minor scars were still visible after healing, the repaired pneumatic actuator can drive normally like the pristine one. It can still carry a weight of 1600 g when variable-stiffness layer is in a hard state. For common soft robotic arms, the elastic bodies are highly strained and thinned, thus susceptible to tearing and puncturing. Even if there is no external damage, repeated actuation will also lead to weakening, bulging of the pneumatic channel, and rupture of the internal wall. Therefore, our new materials with good self-healing properties would greatly improve the pneumatic actuator's service life.
Conclusions
In summary, in order to realize simultaneously high load capacity and environmental adaptability of the pneumatic actuators, and to suppress their susceptibility to damages, here we designed and synthesized a new coordination adaptable network (PETMP-AIM-Cu) that can balance rigidity, healability and soft-rigid switching ability. By utilizing moderate but dense dynamic coordination bonds to crosslink low-molecule-weight ligands, we achieved a combination of high rigidity (Young's modulus of 1.9 GPa and elongation <2% before fracturing) at room temperature and large change (soft-rigid switching ability up to six orders of magnitude) in mechanical strength upon gentle heating. We also obtained a self-healing elastomer based on dynamic disulfide bonds (LP-PDMS) and combined it with PETMP-AIM-Cu to fabricate a new pneumatic actuator. Since the pneumatic actuator is rigid at room temperature but can deform upon heating, both high load capacity and pneumatic actuation can be achieved simultaneously. The stiffness of the pneumatic actuators can be adjusted in a wide range by tuning the voltage to achieve different force outputs for grasping, picking, and manipulating soft and hard objects. In addition, due to the excellent self-healing ability of both the variable-stiffness layer PETMP-AIM-Cu and the elastomer body LP-PDMS, the entire pneumatic actuator can still restore its function after being cutting and healing. Such a variable-stiffness and healable pneumatic actuators would be useful for complex environmental applications.
Experimental section
Materials and general measurements
Pentaerythritol tetra(3-mercaptopropionate) (PETMP, 95%) and copper(II) tetrafluoroborate hexahydrate (Cu(BF4)2·6H2O, 98%) were purchased from Energy Chemical. 1-Methylimidazole (MIM, 99%), 1-propylimidazole (PIM, 99%) and 2,2-bimethoxy-2-phenylacetophenone (DMPA, 99%) were obtained from Aladdin Reagent Co. 1-Allylimidazole (AIM, 98%) and the remaining reagents and solvents were purchased from Macklin. Liquid thiol-terminated polysulfide oligomer (LP3, Mn = 1000) was supplied by Thiokol Co., Ltd, Japan. (Epoxypropoxypropyl)dimethoxysilyl terminated polydimethylsiloxane (epoxy-PDMS, 80–120 cSt.) was purchased from Gelest. Tris(dimethylaminomethyl)phenol (DMP-30, 80%) was purchased from Shanghai Bide Pharmaceutical Technology Co., Ltd. All commercially obtained chemicals were used as received without further purification. 1H NMR (500 MHz) and 13C NMR (125 MHz) spectra were collected with a Bruker DRX 500 NMR spectrometer in dimethyl sulfoxide-d6 with tetramethylsilane as an internal standard (1H) at room temperature (25 °C). ESI-mass spectra (ESI-MS) were recorded by direct injection on an Agilent mass spectrometer with the methanol as solvent and mobile phase. The test was conducted under the positive ion mode. Thermogravimetric analysis (TGA) was carried out on a simultaneous SDT 2960 thermal analysis system from 30 to 600 °C with a heating rate of 10 °C min−1 under the nitrogen atmosphere. Differential scanning calorimetry (DSC) experiments were performed using a Mettler-Toledo DSC1 STARe analyzer under a dry N2 atmosphere (50 mL min−1), and the speed of heating and cooling was 10 °C min−1. The rheological behaviors were evaluated on a TA Instruments DHR-2 system. Temperature and frequency sweeps were all performed with a 8 mm parallel plates on circular samples with a diameter of 8 mm. Temperature sweeps were run from 30 °C to 130 °C (or from 130 °C to 30 °C in the cyclic test mode) at a rate of 2 °C min−1 and a frequency of 1 Hz, and the strain was set at 0.05% ± 0.02% by the instrument to keep the measured torque at a reasonable value when the sample was softened. Contact with the sample was maintained by the auto-compression feature set to 0.2 ± 0.15 N. Frequency sweeps were performed from 0.01 to 400 rad s−1 with a constant 0.05% strain. FT-IR spectra were recorded on a vector 22 Bruker spectrophotometer ranging from 4000 to 400 cm−1. X-ray powder diffraction (XRD) spectrum was obtained with a Bruker D8 ADVANCE X-ray diffractometer at 40 kV.
Synthesis of PETMP-AIM
The PETMP-AIM was synthesized by the thiol-ene click reaction.37 PETMP (50.00 g, 102 mmol), AIM (48.68 g, 450 mmol) and DMPA (1.05 g, 4.09 mmol) were dissolved in 150 mL THF and added into a 500 mL eggplant-shaped bottle equipped with a magnetic stirrer. Then the mixture was stirred at room temperature for 2 h under an argon atmosphere and irradiated with 365 nm UV light. After the reaction, the solvent was removed by rotary evaporation. The crude product was re-dissolved in 50 mL THF and precipitated in 1 L water, washed with water for three times to remove the unreacted AIM. After removing the solvents by vacuum evaporation, the PETMP-AIM was obtained as a light-yellow transparent viscous liquid (84.25 g, yield: 89.3%). Molecular weight according to ESI-MS: found m/z = 921.3450 [M + H+]. 1H NMR (500 MHz, DMSO-d6, δ, ppm): 7.60 (s, 4H), 7.15 (s, 4H), 6.89 (s, 4H), 4.13 (s, 8H), 4.01 (t, J = 6.9 Hz, 8H), 2.69 (t, J = 7.1 Hz, 8H), 2.60 (t, J = 7.1 Hz, 8H), 2.41 (t, J = 7.2 Hz, 8H), 1.96–1.91 (m, 8H). 13C NMR (125 MHz, DMSO-d6, δ, ppm): 170.98, 137.21, 128.45, 119.18, 44.74, 41.81, 39.62, 34.10, 30.32, 27.70, 26.01. The disappearance of stretching vibration at 2568 cm−1 of PETMP and 1645 cm−1 of AIM also confirmed the successful synthesis of PETMP-AIM.
Synthesis of model Cu(II) complex
MIM (L1) (1.00 g, 12.18 mmol) was dissolved in 5 mL of anhydrous ethanol and Cu(BF4)2·6H2O in ethanol (0.20 g mL−1) (5.26 mL, 3.04 mmol) was slowly added into the solution with stirring. The reaction mixture was stirred overnight at room temperature. The mixture was filtered and then washed three times with ethanol, yielding lavender solid powder (1.98 g, yield 96.5%). ESI-MS: found m/z = 195.57 [Cu(L1)4]2+. Complexes [Cu(L2)4]2+ was synthesized in the same procedure, but the target product was obtained by removing the solvent by rotary evaporation, yielding purple solid powder (1.75 g, 98.3%). ESI-MS: found m/z = 251.63 [Cu(L2)4]2+.
Synthesis of the PETMP-AIM-Cu
The highly crosslinked PETMP-AIM-Cu were prepared by reacting PETMP-AIM with Cu(BF4)2·6H2O in ethanol. The general procedure for the preparation of PETMP-AIM-Cu film is as follows. PETMP-AIM (50.00 g, 54.26 mmol) was dissolved in 100 mL ethanol and Cu(BF4)2·6H2O in ethanol (93.65 mL, 0.20 g mL−1) was added to the solution dropwise under stirring for 12 h at room temperature. After the reaction, the mixture was concentrated by rotary evaporation to afford the PETMP-AIM-Cu as a navy-blue solid powder. The solid powder was then hot pressed into block samples of different dimensions for the different tests. As shown in Fig. S7 (ESI†), the vibration peak of the imidazole ring at 906 cm−1 in PETMP-AIM disappeared, and the coordination of Cu(II) and imidazole appeared at 950 cm−1 in PETMP-AIM-Cu.49
Isothermal titration calorimetry (ITC)
The titration was performed using a MicroCal VP-ITC apparatus at 298 K. The solutions for the titrations were prepared in anhydrous ethanol. The Cu(II) solution (3 mM Cu(BF4)2·6H2O) was added into the solution of PETMP-AIM (0.3 mM) during the titrations. Blank titrations in anhydrous ethanol were performed, and the result was subtracted from the corresponding titrations to account for the effect of the dilution. The fitting was performed by using Origin software provided by Microcal and the first two data points were removed due to excessive errors.
Mechanical and self-healing tests
For PETMP-AIM-Cu, mechanical flexural strain–stress was performed using an Instron 3343 instrument under the three-point flexural mode. For all the tests, samples’ size were 30 mm length × 5 mm width × 1 mm height and span of 13 mm, and three samples were used for each test. The temperature-dependent flexural strain–stress test was performed at the speed of 10 mm min−1. As for the self-healing tests, each sample was cut into two pieces and then put together, healing at different temperature for different durations. The healed samples were then tested following the same procedure to obtain the flexural strain–stress curves. The mechanical healing efficiency, η, is defined as the ratio between the maximal strength restored relative to the original maximal strength. For LP-PDMS elastomer, samples’ size was 30 mm length × 2 mm width × 1 mm height and the stress–strain tests were conducted at a strain rate of 50 mm min−1.
3D printing for PETMP-AIM-Cu
A fused deposition modeling (FDM) 3D printer (Regenovo, China) with temperature and gas pressure controllers was used in the 3D-printing experiments. The structures of the samples were designed using Pro/ENGINEER 5.0 (Parametric Technology Corporation, USA). Then the powder of PETMP-AIM-Cu was loaded into a syringe that was preheated to 130 °C for 10 min. The filamentous samples were extruded through a nozzle with a diameter of 400 μm under 0.5 MPa of air pressure to prepare the designed structure.
Fabrication of actuators
The structure and assembly of the pneumatic actuator are detailed in Fig. 3f. The desired structures and shapes were first printed out of commercial resins by using a commercial LCD 3D printer (Jiangsu Wiiboox Technology Co., Ltd) to obtain the resin molds, which were then placed into uncured pre-mixed Ecoflex 00-30 elastomer A and B. When the silicone was cured, the resin mold was taken out and the silicone mold for pouring our resin mixture was available. Next, LP3, epoxy -PDMS and DMP-30 were mixed in a mass ratio of 5
:
2
:
0.07 and then poured into a silicone mold, and cured at room temperature for 6 h to obtain the parts to be assembled. Finally splicing each part through the self-healing property of the material, then a complete pneumatic actuator with good hermeticity was obtained.
Author contributions
H.-Q. W., Z.-Y. H. and C.-H. L. conceived, designed and directed the project; H.-Q. W., Z.-Y. H. performed the experiments and analyzed the data. D.-W.Y. and F.-Z. W. helped to discuss the structure and assembly of the pneumatic actuator. H.-Q. W., Z.-Y. H. and C.-H. L. wrote the paper. All authors discussed the results and commented on the manuscript.
Conflicts of interest
There are no conflicts to declare.
Acknowledgements
The authors would like to thank Prof. Jian Jiao from Peng Cheng Laboratory for the useful discussion on robotics. This work was supported by the National Natural Science Foundation of China (Grant No. 22271139 and 21771100).
Notes and references
- D. Rus and M. T. Tolley, Nature, 2015, 521, 467–475 CrossRef CAS PubMed.
- P. S. Lee and M. Kaltenbrunner, Adv. Mater., 2021, 33, 2007638 CrossRef CAS PubMed.
- M. Wehner, R. L. Truby, D. J. Fitzgerald, B. Mosadegh, G. M. Whitesides, J. A. Lewis and R. J. Wood, Nature, 2016, 536, 451–455 CrossRef CAS PubMed.
- M. Gao, Y. Meng, C. Shen and Q. Pei, Adv. Mater., 2022, 34, 2109798 CrossRef CAS PubMed.
- R. V. Martinez, J. L. Branch, C. R. Fish, L. Jin, R. F. Shepherd, R. M. Nunes, Z. Suo and G. M. Whitesides, Adv. Mater., 2013, 25, 205–212 CrossRef CAS PubMed.
- T. J. Jones, E. J. Puillet, J. Marthelot and P. T. Brun, Nature, 2021, 599, 229–233 CrossRef CAS PubMed.
- R. F. Shepherd, F. Ilievski, W. Choi, S. A. Morin, A. A. Stokes, A. D. Mazzeo, X. Chen, M. Wang and G. M. Whitesides, Proc. Natl. Acad. Sci. U. S. A., 2011, 108, 20400–20403 CrossRef CAS PubMed.
- A. D. Marchese, C. D. Onal and D. Rus, Soft Rob., 2014, 1, 75–87 CrossRef PubMed.
- M. Cianchetti, T. Ranzani, G. Gerboni, T. Nanayakkara, K. Althoefer, P. Dasgupta and A. Menciassi, Soft Rob., 2014, 1, 122–131 CrossRef.
- E. T. Roche, R. Wohlfarth, J. T. B. Overvelde, N. V. Vasilyev, F. A. Pigula, D. J. Mooney, K. Bertoldi and C. J. Walsh, Adv. Mater., 2014, 26, 1200–1206 CrossRef CAS PubMed.
- Y. Qiu, E. Askounis, F. Guan, Z. Peng, W. Xiao and Q. Pei, ACS Appl. Polym. Mater., 2020, 2, 2008–2015 CrossRef CAS.
- M. Cianchetti, C. Laschi, A. Menciassi and P. Dario, Nat. Rev. Mater., 2018, 3, 143–153 CrossRef.
- H. K. Yap, H. Y. Ng and C. H. Yeow, Soft Rob., 2016, 3, 144–158 CrossRef.
- C. Y. Chu and R. M. Patterson, J. Neuroeng. Rehabil., 2018, 15, 1–14 CrossRef PubMed.
- Y. Chi, Y. Tang, H. Liu and J. Yin, Adv. Mater. Technol., 2020, 5, 2000370 CrossRef CAS.
- M. Runciman, A. Darzi and G. P. Mylonas, Soft Rob., 2019, 6, 423–443 CrossRef PubMed.
- S. Terryn, J. Brancart, D. Lefeber, G. V. Assche and B. Vanderborght, Sci. Rob., 2017, 2, eaan4268 CrossRef PubMed.
- L. Wang, Y. Yang, Y. Chen, C. Majidi, F. Iida, E. Askounis and Q. Pei, Mater. Today, 2018, 21, 563–576 CrossRef CAS.
- Y. Yang, Y. Chen, Y. Li, M. Z. Q. Chen and Y. Wei, Soft Rob., 2017, 4, 147–162 CrossRef PubMed.
- Y.-F. Zhang, N. Zhang, H. Hingorani, N. Ding, D. Wang, C. Yuan, B. Zhang, G. Gu and Q. Ge, Adv. Funct. Mater., 2019, 29, 1806698 CrossRef.
- A. Tonazzini, A. Sadeghi and B. Mazzolai, Soft Rob., 2016, 3, 34–41 CrossRef.
- I. M. Van Meerbeek, B. C. Mac Murray, J. W. Kim, S. S. Robinson, P. X. Zou, M. N. Silberstein and R. F. Shepherd, Adv. Mater., 2016, 28, 2801–2806 CrossRef CAS PubMed.
- Y. Hao, T. Wang, Z. Xie, W. Sun, Z. Liu, X. Fang, M. Yang and L. Wen, J. Micromech. Microeng., 2018, 28, 024004 CrossRef.
- S. Y. Narang, J. J. Vlassak and D. R. Howe, Adv. Funct. Mater., 2018, 28, 1707136 CrossRef.
- E. Acome, S. K. Mitchell, T. G. Morrissey, M. B. Emmett, C. Benjamin, M. King, M. Radakovitz and C. Keplinger, Science, 2018, 359, 61–65 CrossRef CAS PubMed.
- S. Terryn, J. Langenbach, E. Roels, J. Brancart, C. Bakkali-Hassani, Q. A. Poutrel, A. Georgopoulou, T. G. Thuruthel, A. Safaei, P. Ferrentino, T. Sebastian, S. Norvez, F. Iida, A. W. Bosman, F. Tournilhac, F. Clemens, G. V. Assche and B. Vanderborght, Mater. Today, 2021, 47, 187–205 CrossRef CAS.
- E. Roels, S. Terryn, F. Iida, A. W. Bosman, S. Norvez, F. Clemens, G. V. Assche, B. Vanderborght and J. Brancart, Adv. Mater., 2022, 34, 2104798 CrossRef CAS PubMed.
- S. Terryn, E. Roels, J. Brancart, G. V. Assche and B. Vanderborght, Actuators, 2020, 9, 34 CrossRef.
- Y. Yanagisawa, Y. Nan, K. Okuro and T. Aida, Science, 2018, 359, 72–76 CrossRef CAS PubMed.
- M.-H. Zhang, C.-H. Li and J.-L. Zuo, Cell Rep. Phys. Sci., 2020, 1, 100144 CrossRef CAS.
- Y. Tao, S. Liu, Y. Zhang, Z. Chi and J. Xu, Polym. Chem., 2018, 9, 878–884 RSC.
- W. Li, H.-Q. Wang, W.-T. Gao, Z. Wang, P. Xu, H. Ma and C.-H. Li, CCS Chem., 2022, 1–17 Search PubMed.
- S. C. Grindy, R. Learsch, D. Mozhdehi, J. Cheng, D. G. Barrett, Z. Guan, P. B. Messersmith and N. Holten-Andersen, Nat. Mater., 2015, 14, 1210–1216 CrossRef CAS PubMed.
- S. Tang and B. D. Olsen, Macromolecules, 2016, 49, 9163–9175 CrossRef CAS.
- D. Mozhdehi, S. Ayala, O. R. Cromwell and Z. Guan, J. Am. Chem. Soc., 2014, 136, 16128–16131 CrossRef CAS PubMed.
- D. E. Fullenkamp, L. He, D. G. Barrett, W. R. Burghardt and P. B. Messersmith, Macromolecules, 2013, 46, 1167–1174 CrossRef CAS PubMed.
- J. Shi, N. Zhao, D. Yan, J. Song, W. Fu and Z. Li, J. Mater. Chem. A, 2020, 8, 5943–5951 RSC.
- Y. V. Griko, Biophys. Chem., 1999, 79, 117–127 CrossRef CAS PubMed.
- E. A. Archer and M. J. Krische, J. Am. Chem. Soc., 2002, 124, 5074–5083 CrossRef CAS PubMed.
- L. S. Liebeskind, P. Gangireddy and M. G. Lindale, J. Am. Chem. Soc., 2016, 138, 6715–6718 CrossRef CAS PubMed.
- J. Wang, M. Zhang, X. Xu, J. Feng, Y. Wang, M. Zhang, W. Han, Y. Chen and G. Tian, Chem. Res. Chin. Univ., 2018, 34, 8–12 CrossRef CAS.
- A. Jourdain, R. Asbai, O. Anaya, M. M. Chehimi, E. Drockenmuller and D. Montarnal, Macromolecules, 2020, 53, 1884–1900 CrossRef CAS.
- C. J. Kloxin and C. N. Bowman, Chem. Soc. Rev., 2013, 42, 7161–7173 RSC.
- P.-C. Zhao, W. Li, W. Huang and C.-H. Li, Molecules, 2020, 25, 597 CrossRef CAS PubMed.
- S. Chen, L. Sun, X. Zhou, Y. Guo, J. Song, S. Qian, Z. Liu, Q. Guan, E. M. Jeffries, W. Liu, Y. Wang, C. He and Z. You, Nat. Commun., 2020, 11, 1–8 CrossRef PubMed.
- L. Zhang, J. Liang, C. Jiang, Z. Liu, L. Sun, S. Chen, H. Xuan, D. Lei, Q. Guan, X. Ye and Z. You, Natl. Sci. Rev., 2021, 8, nwaa154 CrossRef CAS PubMed.
- X. Chen, Q. Zhong, C. Cui, L. Ma, S. Liu, Q. Zhang, Y. Wu, L. An, Y. Cheng, S. Ye, X. Chen, Z. Dong, Q. Chen and Y. Zhang, ACS Appl. Polym. Mater., 2020, 12, 30847–30855 CrossRef CAS PubMed.
- W. A. Ogden and Z. Guan, J. Am. Chem. Soc., 2018, 140, 6217–6220 CrossRef CAS PubMed.
- J. A. Welleman, F. B. Hulsbergen, J. Verbiest and J. Reedijk, J. Inorg. Nucl. Chem., 1978, 40, 143–147 CrossRef CAS.
|
This journal is © The Royal Society of Chemistry 2023 |
Click here to see how this site uses Cookies. View our privacy policy here.