DOI:
10.1039/D2MH01207C
(Review Article)
Mater. Horiz., 2023,
10, 1105-1120
Liquid-precursor-intermediated synthesis of atomically thin transition metal dichalcogenides
Received
28th September 2022
, Accepted 9th December 2022
First published on 13th December 2022
Abstract
With the rapid development of integrated electronics and optoelectronics, methods for the scalable industrial-scale growth of two-dimensional (2D) transition metal dichalcogenide (TMD) materials have become a hot research topic. However, the control of gas distribution of solid precursors in common chemical vapor deposition (CVD) is still a challenge, resulting in the growth of 2D TMDs strongly influenced by the location of the substrate from the precursor powder. In contrast, liquid-precursor-intermediated growth not only avoids the use of solid powders but also enables the uniform distribution of precursors on the substrate through spin-coating, which is much more favorable for the synthesis of wafer-scale TMDs. Moreover, the spin-coating process based on liquid precursors can control the thickness of the spin-coated films by regulating the solution concentration and spin-coating speed. Herein, this review focuses on the recent progress in the synthesis of 2D TMDs based on liquid-precursor-intermediated CVD (LPI-CVD) growth. Firstly, the different assisted treatments based on LPI-CVD strategies for monolayer 2D TMDs are introduced. Then, the progress in the regulation of the different physical properties of monolayer 2D TMDs by substitution of the transition metal and their corresponding heterostructures based on LPI-CVD growth are summarized. Finally, the challenges and perspectives of 2D TMDs based on the LPI-CVD method are discussed.
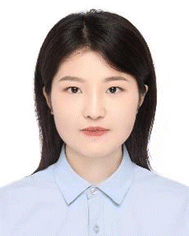
Huiyan Guan
| Huiyan Guan received her BS Degree in Functional Materials from the Henan Normal University in 2022. Currently, she is an MA student at the School of Physics of Southeast University under the supervision of Prof. Zhenhua Ni and Prof. Bei Zhao. Her research is focused on the controllable synthesis of two-dimensional materials and their applications in electronics, and optoelectronics. |
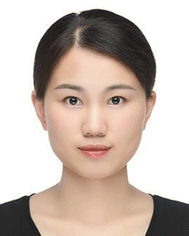
Bei Zhao
| Dr Bei Zhao received her BS Degree in Materials Chemistry from Shandong University of Technology in 2014, her MA Degree in Applied Chemistry from Southwest University in 2017, and her PhD in Physical Chemistry from Hunan University in 2021. She has been an Associate Professor in Physics Department at Southeast University since December 2022. Her research is focused on the controllable synthesis of two-dimensional materials and their applications in electronics, and optoelectronics. |
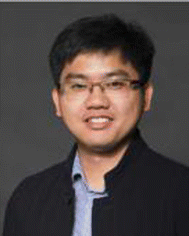
Zhenhua Ni
| Dr Zhenhua Ni received his BS Degree in Physics and a second BS Degree in Applied Electrical Techniques from Shanghai Jiaotong University in 2003 and PhD in Physics from the National University of Singapore (NUS) in 2007. Subsequently, he did postdoctoral research in the Department of Physics and Applied Physics at Nanyang Technological University (NTU) from 2007–2010. In 2009, he received the British Council “Researcher Exchange Programme Award” and worked as an academic visitor in Andre Geim's research group in the University of Manchester. He has been a Professor in Physics Department at Southeast University since May 2010. His current research interests include the fabrication, characterization, and optoelectronic application of graphene and other two-dimensional materials. |
1. Introduction
Two-dimensional (2D) materials have increasingly shown great potential for (opto)electronic,1 electrocatalytic,2 and energy storage3 applications due to their unique dimensionality, layer-dependent adjustable band gap, diverse crystal structures, and other properties. With the rapid development of integrated electronic device technology,4–9 the growth of large-area and high-quality 2D materials, especially TMD materials,5,10,11 limits the transformation from theoretical research in the laboratory to large-scale industrial applications such as electronic devices.12–14 Therefore, exploring controllable methods for the large-scale and industrial-scale synthesis of TMDs with uniform thickness becomes the key to facilitating scalable industrial applications for a range of electronic devices.15–17 In this case, CVD18 is one of the most promising method because of its low cost,19 scalable material domain size,20 and high controllability of thickness and uniformity,21 attracting significant attention from researchers.22 However, during the common CVD process,23 it is difficult to control the vapor phase distribution and reaction rate of the solid precursor powder in the growth chamber.24 The precursor concentration is not uniformly distributed on the substrate,19 which will lead to the formation of local aggregation or local sparse non-uniform distribution of nucleation sites on the substrate,25 resulting in the formation of undesirable nucleation and difficulty in controlling the nucleation density.26 Although the introduction of a solid salt powder during the CVD process effectively reduces the high melting point of transition metal precursor powder, the distribution of the precursor concentration on the substrate in the growth chamber is still difficult to control.27 Moreover, undesirable and inevitable byproducts or impurities will be introduced,28 seriously decreasing the quality and influencing the stability of ultra-thin TMDs, leading to a poor electrical and optical performance.29 Also, the byproducts and residual salts remain in the growth chambers and are difficult to remove,30 thereby increasing the time and cost of cleaning or replacing the crucibles and quartz tubes.31
Recently, the LPI-CVD strategy has attracted great interest for the uniform wafer-scale growth of TMDs with high reproducibility, efficiency and scalability.27 In the LPI-CVD process, the appropriate soluble precursor salt28 such as Na2WO4, Na2MoO4, NaVO3 and NaReO4 is firstly dissolved in a certain solvent to achieve a uniform mixture of one or more precursors at the molecular level to form a precursor solution.32 Subsequently, the precursor solution is spin-coated on the substrate by adjusting the solution concentration and spin-coating speed to form an ultrathin film with the uniform distribution of each precursor source on the substrate.33 Finally, as the growth temperature increases, the water in the spin-coated film gradually evaporates,34 and the remaining solvent is uniformly distributed on the substrate in the molten state due to its lower melting point (650–850 °C),35 making it easier to react with the gaseous sulfur precursors to produce uniform, high-quality and wafer-scale 2D TMD films such as MoS2,36 MoSe2,37 and WS2.38 Moreover, the amount of each precursor source in the precursor solution can be precisely controlled by simply mixing molten salt aqueous solutions in designated ratios,39 which is more conducive to achieve uniform doping to regulate the electrical and optical properties of TMDs.40 Notably, the use of precursor solutions in the LPI-CVD method allows the formation of precursor films with specific patterns on the substrate,41 which can then be templated to grow to form high-quality 2D TMDs arrays, which is difficult to achieve with powder precursors.42,43 In addition, precursor films with a precisely controlled composition or thickness reduce the production of some undesirable by-products and additional contaminants in the growth chamber during the growth process.44 Herein, a systematic understanding based on existing typical works needs to be further established to set the stage for the future development of the LPI-CVD method.
Herein, we present a comprehensive review of the recent advances in the synthesis of 2D TMDs based on LPI-CVD growth, and the following contents include four parts. Firstly, various assisted treatments that enable the controlled synthesis of atomically thin 2D TMDs, such as direct synthesis, molten-salt assisted, hydroxide-assisted, polymer-assisted, and the supply of novel chalcogen precursors are presented in detail. Then, the developed techniques for regulating the different properties of atomically doped monolayer 2D TMDs are discussed, which are capable of achieving uniform large-area doping in a controlled way. Furthermore, we summarize the progress on the synthesis of heterostructures based on 2D TMDs using the LPI-CVD strategy with particular emphasis on its advantages. Finally, the challenges and perspectives in the future of liquid-precursor-intermediated synthesis of 2D TMDs are prospected, showing the potential opportunities in realizing a technological revolution by LPI-CVD in TMD systems.
2 Synthesis of monolayer 2D TMDs materials
The LPI-CVD method allows the film thickness to be controlled by adjusting the solution concentration and spin-coating speed, which is a huge advantage for the growth of large-area 2D TMDs growth.27 The successful implementation of LPI-CVD requires the following conditions: (I) the precursor salts must have a suitable melting point and dissolve in suitable solvents (such as H2O). (II) Precursor salts that tend to form stable low vapor pressure melts at LPI-CVD growth temperatures. (III) The substrate must be hydrophilic, which can be treated by oxygen plasma, UV-O3, and piranha solution. Furthermore, various assisted techniques have been implemented to promote the lateral growth of 2D TMDs such as molten-salt assisted, hydroxide-assisted, polymer-assisted, and supply of novel chalcogen precursors, which will be summarized in this section.
2.1 Direct LPI-CVD growth
In the common CVD, a powder precursor such as MoO3, WO3, sulfur powder and selenium powder is used to obtain 2D TMDs.45–47 However, the gas atmosphere generated by the evaporation of the precursor powder in the growth chamber is difficult to control,23 causing the precursor concentration to be non-uniformly distributed on the substrate and the nucleation density uneven.48 The use of liquid precursors solves the problem of non-uniform distribution of the precursor concentration on the substrate and opens a new path for the growth of ultrathin 2D TMDs.49 In 2012, Liu et al. firstly demonstrated the production of large-area MoS2 films on insulating substrates by using (NH4)2MoS4 solution as the Mo source precursor.50 However, the synthesis of uniform monolayer MoS2 films was not achieved, and this dip-coating method only achieved the growth of triple MoS2 films with two layers appearing locally, as shown in Fig. 1(b). This can be attributed to the fact that the dip-coating process does not ensure a uniform distribution of the precursor solution on the substrate surface, and too many Mo sources on the substrate make it difficult to form a thinner MoS2 film (Fig. 1(a)).
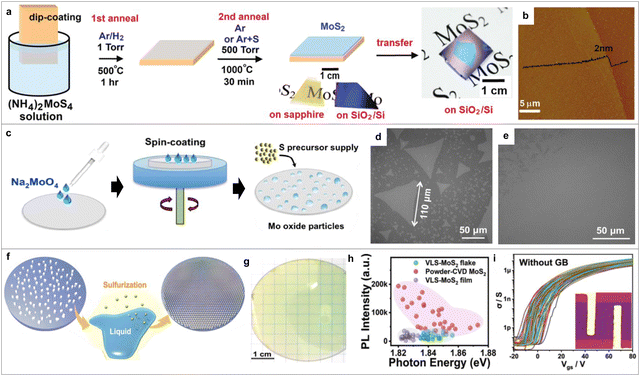 |
| Fig. 1 (a) Illustration of the synthesis of MoS2 layers. Inset is the optical image of MoS2 film.50 (b) AFM image of the triple MoS2.50 (c) Illustration of the synthesis of monolayer MoS2.36 (d) Optical image of MoS2 flake.36 (e) Optical image of continuous MoS2 film.36 (f) Illustration of the V-S-L growth mechanism for MoS2 films.52 (g) Optical image of monolayer MoS2 film on a sapphire substrate.52 (h) PL distribution of three types of MoS2 samples.52 (i) Transport curves of FETs based on MoS2 without the growth of GB.49 (a) and (b) Reprinted from ref. 50 Copyright 2012, the American Chemical Society. (c)–(e) Reprinted from ref. 36 Copyright 2019, Springer Nature. (f)–(i) Reprinted from ref. 52 Copyright 2019, The Royal Society of Chemistry. | |
In contrast, the spin-coating process has a huge advantage in ensuring that the precursor solution forms a uniform film thickness at all locations on the substrate surface by precisely controlling the spin rate, spin time and other parameters. Cun et al. successfully achieved the growth of wafer-scale monolayer MoS2 films by the spin-coating process with the uniform distribution of Mo nucleation sites (Fig. 1(c)–(e)).36 Similarly, NaMoO4 particles uniformly distributed on the substrate by spin coating will melt into liquid, while high-quality MoS2 films with high electrical performance (Fig. 1(f)–(i)) will be synthesized when the sulfur vapor is saturated with liquid droplets.51,52 Furthermore, other 2D materials such as 2-inch continuous WS2 films38 and continuous Bi2O2Se films53 with the thickness of few atomic layers have also been successfully obtained via the LPI-CVD method. Furthermore, industrial inkjet-printer was used in the LPI-CVD system to prepare monolayer TMDs such as MoSe2 and MoS2 with millimeter size, in which an aqueous solution of (NH4)6Mo7O24–4H2O was used as the precursor solution.54 Therefore, the LPI-CVD method is still feasible for the large-area ultrafast and patterned growth of high-quality TMDs under suitable conditions, with good prospects for industrialization, which requires more research in the future.
2.2 Molten-salt-assisted LPI-CVD growth
In general, by using alkali metal halide mixed directly with solid source powders as precursors, TMD films will inevitably grow in the vapor–solid–solid (VSS) growth mode, sometimes accompanied by the vapor–liquid–solid (VLS) growth mode. In comparison, a promising feature of the molten salt-assisted approach is that the molten salt and the transition metal source can be homogeneously mixed in the liquid state at the molecular level, which ensures their uniform distribution on the substrate surface by spin-coating, ink-jet printing, and other processes, efficient interaction with the transition metal source and stable synthesis of TMDs in a single VLS growth mode. For example, Ji et al. successfully synthesized millimeter-size monolayer MoS2 films by using (NH4)2MoO4 and KI as precursors via the LPI-CVD method (Fig. 2(a)).55 The presence of KI in the growth lower the MoS2 reaction barrier. According to the edge passivation-substitution (EPS) theoretical growth model shown in Fig. 2(b), this reduction in the reaction potential barrier is attributed to the passivation of the Mo atom zigzag edges by halogen atoms. The Mo–X bond will be replaced by an Mo–S bond during the sulfuration reaction, decreasing the reaction potential barrier compared to the oxygen atom passivation-substitution process, which greatly facilitates the growth of millimeter-size MoS2 monolayer (Fig. 2(c)). For common alkali metal halides such as NaCI and KI, their alkali metals can liquefy and stabilize transition metal oxides (TMOs), their halogen elements are capable of reacting with TMOs to form metal oxychlorides with low melting points, and the synergistic effect of the two can significantly reduce the formation energy of the material. The addition of KI produced uniform and continuous high-quality MoSe2 films (Fig. 2(d)) in which KI significantly reduced the energy barrier of chalcogenization and promoted the lateral growth of MoSe2 along the in-plane.34 Besides MoS2 and MoSe2, the KI-assisted LPI-CVD method can also be used for the growth of WS2 (Fig. 2(e) and WSe2 (Fig. 2(f)).56
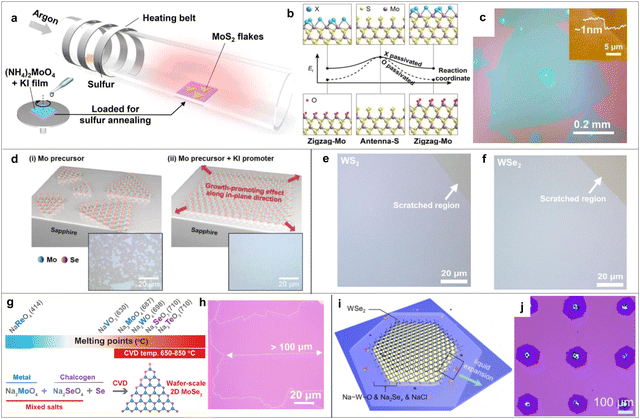 |
| Fig. 2 (a) Schematic of the synthesis of MoS2 flakes.55 (b) Energy barrier diagram for MoS2 growth with (top) and without (bottom) KI and the edge structure.55 (c) Optical image of MoS2 flakes, inset is the AFM height image of MoS2 edge.55 (d) Illustration of MoS2 growth with and without KI, inset is the optical image of MoS2 film.34 Optical image of continuous WS2 (e) and WSe2 film (f).56 (g) Illustration of the melting points of some precursors, and schematic of the synthesis of MoSe2 film.57 (h) Optical image of monolayer MoSe2 film with grain boundaries.57 (i) Illustration of the self-expanding molten salt-driven mechanism for growing WSe2 films.58 (j) Optical image of WSe2 array.58 (a)–(c) Reprinted from ref. 55 Copyright 2021, American Association for the Advancement of Science. (d) Reprinted from ref. 34 Copyright 2022, Wiley-VCH. (e) and (f) Reprinted from ref. 56 Copyright 2021, the American Chemical Society. (g) and (h) Reprinted from ref. 57 Copyright 2021, the American Chemical Society. (i) and (j) Reprinted from ref. 58 Copyright 2022, the American Chemical Society. | |
In addition, besides the common alkali metal halide, some molten salts with the chemical formula AxMOy (where A is an alkali metal and M is a transition metal or sulfur group element) not only can serve as precursors for the growth of 2D TMDs by themselves, such as Na2SeO3 and Na2TeO3, but can also effectively reduce the formation energies of TMDs (Fig. 2(g) and (h)), making it a new salt-assisted LPI-CVD method. For example, Li et al. synthesized continuous-monolayer MoSe2 films with a grain size of 100–250 μm by mixing Na2MoO4–Na2SeO3.57 Compared with only Na2MoO4 molten salt, the mixed molten salt formed by adding Na2SeO3 molten salt not only made the produced film more homogeneous but also significantly lowered the reaction potential and promoted the domain size of the monolayer MoSe2. With an increase in temperature, Na+ can stabilize MoO42− and promote the growth of MoSe2 in concert with SeO32−. Similarly, ReSe2 and WTe2 with improved yield have also been synthesized by using NaReO4–Na2SeO3 and Na2WO4–Na2TeO3 as precursors, respectively.
As research progresses, the molten-salt-assisted growth mechanism is increasingly being understood and the growth behavior is also becoming more controllable. Recently, Jiang et al. reported a new self-expanding molten salt-driven growth mechanism and successfully achieved the patterned growth of highly crystalline TMD arrays.58 At a lower temperature, the Na2Sex (formed by Se with NaCl) reacts with Na–W–O intermediates (produced by WO3 and NaCl) to form WSe2 nuclei at designed sites, which then continuously extend horizontally and vertically. The molten salt in the interlayer between the TMD edge and the substrate confines the reaction sites (Fig. 2(i)). Due to the non-wetting effect of the TMDs, the molten salt keeps spreading to the edge and provides a reaction source, and the monolayer TMDs keep expanding until the molten salt is depleted. The obtained TMD arrays (Fig. 2(j)) present new ideas for the preparation of wafer-scale and single-crystal TMDs. The deeper exploration of the molten-salt assisted method and its development and application deserve more efforts for further research.
2.3 Hydroxide-assisted LPI-CVD growth
The novel design of precursor solutions can open up new ideas for the growth of 2D TMD films. Unlike other promoters that produce unavoidable residues, the introduction of hydroxyl groups not only can be effectively removed by temperature regulation, but also shows amazing advantages in promoting the monolayer growth of TMDs. In 2019, Zhu et al. reported an MoS2–OH bilayer-mediated method to successfully grow one-inch uniform monolayer MoS2 films.59 The added KOH forms homogeneous mixture of solutions, which can generate –OH, the key to hydroxide-assisted growth. When the –OH group is not present in the precursor solution (Fig. 3(a)), the Mo atoms directly combine with sulfur atoms on the substrate to form multilayer MoS2. When the –OH group is introduced in the precursor solution, as shown in Fig. 3(b), the Mo atoms and sulfur atoms are combined in the substrate to firstly form monolayer MoS2, and then the –OH group will be adsorbed on the monolayer MoS2 (100) surface to form an MoS2–OH bilayer structure. The presence of the –OH group layer on the MoS2 (100) surface makes the Mo atoms and sulfur atoms adsorbed only on the edge of the first MoS2 layer, preventing the growth of MoS2 along the [001] axis to form multilayers and promoting its growth in the lateral direction, leading to the preferential formation of ultra-large monolayer MoS2. Fig. 3(c) clearly demonstrates the uniformity of the monolayer MoS2 films with full coverage on 1 × 1 and 3 × 3 cm2 sapphire obtained by the hydroxide-assisted LPI-CVD method. In addition, the binding energy of MoS2 is −0.24 eV with the introduction of –OH groups, which is lower than the corresponding binding energy without –OH groups (Fig. 3(d)), confirming that the introduction of –OH groups reduces the energy barrier for the growth of monolayer MoS2.
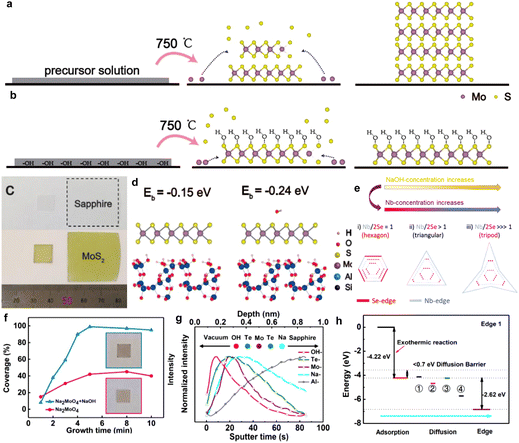 |
| Fig. 3 Illustration of MoS2 growth with (a) and without NaOH (b).59 (c) Optical micrograph of MoS2 on sapphire substrate.59 (d) Atomic structure of MoS2 with and without NaOH and the corresponding calculated binding energies.59 (e) Transformation diagram of NbSe2 morphology at different NaOH or Nb concentrations.60 (f) MoTe2 coverage at different precursor solutions and inset is the optical image of MoTe2 films.61 (g) Time-of-flight secondary ion mass spectrometry (ToF-SIMS) of MoTe2 growth with NaOH.61 (h) Calculated reaction energy diagram of MoTe2 molecule adsorbed on the surface of MoTe2 monolayer.61 (a)–(d) Reprinted from ref. 59 Copyright 2019, the American Chemical Society. (e) Reprinted from ref. 60 Copyright 2020, the American Chemical Society. (f)–(h) Reprinted from ref. 61 Copyright 2021, the American Chemical Society. | |
Similar to the effect produced in promoting the growth of MoS2 monolayers, the introduction of hydroxyl groups can also have an effect on the modulation of TMD shape. The presence of the OH− could modulate the morphology of NbSe2, which could anchor the Nb precursor.60 With an increase in the –OH and Nb concentration, the edge growth rate of the Se terminal was faster than that of the Nb terminal, and thus the shape of NbSe2 changed from hexagonal to triangular, tripod-like, and herringbone-like (Fig. 3(e)). In addition, a centimeter-sized monoclinic MoTe2 monolayer with almost 100% coverage (Fig. 3(f)) has also been successfully achieved using the hydroxide-assisted LPI-CVD method.61 The time-of-flight secondary ion mass spectrometry (ToF-SIMS) images showed that the –OH introduced from the precursor solution could adsorb on the surface (Fig. 3(g)) of the material to form an –OH layer, which inhibited the growth of the material in the vertical direction. DFT calculations showed that the diffusion potential of less than 0.7 eV for different diffusion paths of MoTe2 molecules from the surface to the edge and the adsorption energy at the edges is smaller than that at the surface (Fig. 3(h)), which indicates that MoTe2 molecules can diffuse on the surface and adsorb more preferentially at the edge sites, leading to the lateral growth of MoTe2. This success, which has the same effect as the promotion of monolayer MoS2 growth described above, indicates that the hydroxide-assisted LPI-CVD growth method has the potential to be extended to the monolayer of other TMDs growth and can provide a simple and universal idea for the growth of ultrathin TMDs.
2.4 Polymer-assisted LPI-CVD growth
Besides water, organic solutions have been also used as the basic solvent, such as dimethylformamide (DMF), n-butylamine, ethylenediaminetetraacetic acid (EDTA), and dimethyl sulfoxide (DMSO), for the growth of TMDs. By adding complexing agents, hydrogen and coordination bonds are formed with the soluble salt in solution, which in turn form stable polymers to improve the controllability and homogeneity of the precursor films during spin coating. In 2015, Yang et al. successfully synthesized 2-inch wafer-scale homogeneous MoS2 films with excellent thickness controllability (Fig. 4(a)) by optimizing the solvent for dissolving (NH4)2MoS4.62 When the solvent contained only DMF (Fig. 4(b)), the MoS42− group could not be completely dissolved, leaving a precursor film with de-wetted areas and pinholes. When 2-aminoethanol was added in DMF solvent (Fig. 4(c)), its functional group hydroxide group could join with the MoS42− group to form hydrogen bonds, which allowed the MoS42− group to be uniformly distributed in the solution, and eventually form a uniform precursor film, while it still had certain micro-scale defects. When n-butylamine was added to the achieved solvent (Fig. 4(d)), an almost defect-free MoS42− group film was achieved. In addition, as shown in Fig. 4(e) and (f), the linear-poly(ethylenimine) (L-PEI) in the precursor solution is ionically bonded to the anhydrous ammonium tetrathiomolybdate (ATM) to form a complex precursor, which facilitates uniform spin coating of large areas and reduces the de-wetting areas,63 and therefore 6-in wafer-scale MoS2 films were more readily available. Noteworthy, L-PEI can be decomposed directly without carbon residue at relatively low temperature, which avoids contamination during the growth process. Compared to the use of DMF and L-PEI, the introduction of other organic solvents such as chelating agents not only can reduce the dehumidification of precursor films on the substrate surface, but also interact with the precursor source in solution to increase the coverage of the precursor on the substrate surface, which is also conducive to achieve the growth of wafer-scale MoS2 films.64 In the precursor solution, EDTA coordinates with the MoS42− anion in DMSO to form hydrogen bonds and produce a complex precursor solution (Fig. 4(g)). When spin-coating the precursor solution, each carboxyl group from EDTA interacts with the hydroxyl group of the substrate, which anchors the precursor to the surface. Upon heating, DMSO first evaporates and releases H2S and NH3 gases, leaving equal amounts of MoS3 to form ligand bonds with equal amounts of EDTA (Fig. 4(h)). Then, EDTA evaporates at high temperature, causing MoS3 to be uniformly distributed on the surface with hydroxyl terminations, and finally MoS3 is reduced to crystalline MoS2 films. This provides a new approach to synthesize large-area monolayer TMDs; however, the selection of the polymers used still needs further updating to better achieve the targeted and uniform distribution of precursors on the substrate surface. Recently, Kim et al. used colloidal nanoparticles with the uniform distribution of precursors on a nanometer scale as precursors to achieve the generation of wafer-scale MoS2 films.65 The MoO2 nanoparticle precursors were formed via the polyvinyl-pyrrolidone (PVP)-assisted hydrothermal method, which were uniformly distributed on the substrate by the spin-coating process. At the beginning of sulfur supply growth, polycrystalline MoO2 nanoparticles start to unfold laterally (Fig. 4(i)) and continuously transform into nanocrystals with single domains. Then, monolayers of MoS2 nucleate at the bottom of MoO2 nanoparticles with the lowest energy orientation angle (Fig. 4(j)). Finally, until the MoO2 particles are depleted, the wafer-scale MoS2 monolayer is synthesized on the substrate. It is worth noting that some appropriate organic solvents can evaporate without leaving residues during the ramp-up process, effectively avoiding the generation of contaminants. However, there are still many organic solvents with unavoidable residual problems, and therefore the selection of better organic solvents that effectively reduce the dehumidification of the precursor films and can more precisely control the coverage of the precursors on the substrate surface is worth further exploration and research.
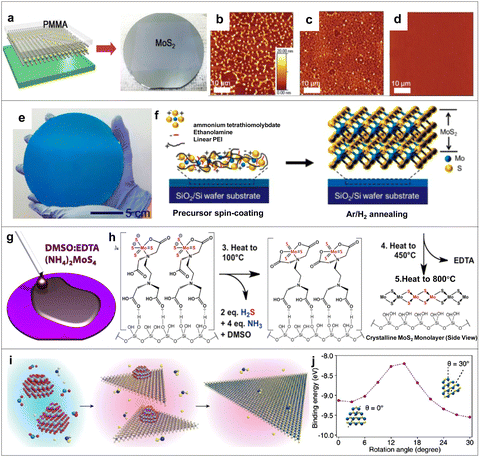 |
| Fig. 4 (a) Transfer process of MoS2 film.62 (b)–(d) AFM images of different precursor films: (b) only DMF, (c) DMF : 2-aminoethanol (9 : 1), and (d) DMF : n-butylamine : 2-aminoethanol in a volume ratio of 4.5 : 4.5 : 1.62 (e) Optical image of MoS2 film.63 (f) Schematic of the synthesis of MoS2 films.63 (g) Schematic of the synthesis of MoS2 film.64 (h) Growth mechanism of MoS2 films.64 (i) Growth mechanism of MoS2 films.65 (j) Binding energy curves of MoS2 and MoO2 at different rotation angles.65 (a)–(d) Reprinted from ref. 62 Copyright 2015, The Royal Society of Chemistry. (e) and (f) Reprinted from ref. 63 Copyright 2017, The American Chemical Society. (g) and (h) Reprinted from ref. 64 Copyright 2017, Springer Nature. (i) and (j) Reprinted from ref. 65 Copyright 2021, the American Chemical Society. | |
2.5 Novel chalcogen precursor-supplied LPI-CVD growth
The transition metal precursor powder can be replaced by the corresponding soluble salt in the LPI-CVD method, allowing the transition metal precursor sources to be uniformly distributed on the substrate. However, the gas distribution of the chalcogen source in the chamber is still difficult to control. Therefore, it is equally critical to design a uniform and appropriate amount of chalcogen to control the flow on the substrate surface. In 2017, Boandoh et al. proposed a novel method to synthesize large-area MoS2 films by precisely controlling the bubbling systems of the supply of chalcogen organics in the LPI-CVD method.66 When Ar is passed through a bubbler into liquid dimethyl disulfide (DMDS), the DMDS enters the growth chamber in the form of bubbles, which contains Mo(CO)6 to supply the Mo source (Fig. 5(a)) and promote the growth of the Mo terminal edges. DMDS continuously decomposes into methyl mercaptan (CH3SH), ethylene (CH2CH2), and hydrogen sulfide (H2S) and other sulfides during the growth process, while Mo(CO)6 decomposes into Mo radicals and undergoes the sulfidation reaction (Fig. 5(b)). The amount of sulfur precursors can be the precisely controlled by controlling the flow rate of DMDS, resulting in the formation of large-area MoS2 monolayer films (Fig. 5(c)). Similarly, dodecyl mercaptan (C12H25SH) could also be used as the sulfur source to synthesize uniform MoS2 monolayers through LPI-CVD with a bubbling system.67 Thiol (C12H25SH) as the sulfur source could precisely control the precursor concentration during the growth process (Fig. 5(d)), and also effectively repair the sulfur vacancies in the MoS2 material (Fig. 5(d)–(f)). This novel sulfur supply method opens new horizons for the introduction of sulfur in common CVD systems with certain scalability as well as reproducibility. It effectively avoids the difficulty in controlling the gas-phase distribution generated by conventional solid sulfur sources.
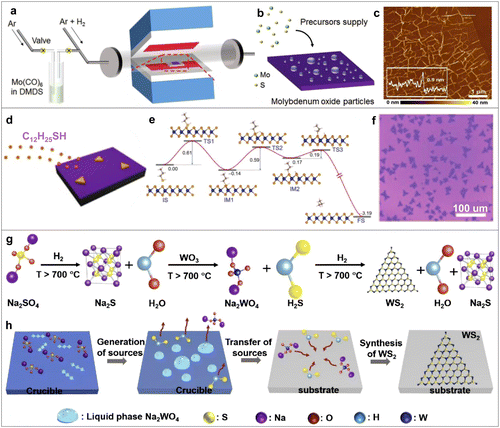 |
| Fig. 5 (a) Illustration of the bubbling system.66 (b) Schematic of the synthesis of MoS2 film.66 (c) AFM image of the MoS2 film, where the white line shows the height of the film.66 (d) Diagram showing the process of supplying thiol molecules.67 (e) Potential diagram of thiol molecule repairing sulfur vacancy process.67 (f) Optical images of MoS2 films.67 (g) Illustration of three-step chemical reaction process.68 (h) Illustration of WS2 growth process.68 (a)–(c) Reprinted from ref. 66 Copyright 2017, Wiley-VCH. (d) and (f) Reprinted from ref. 67 Copyright 2020, Wiley-VCH. (g) and (h) Reprinted from ref. 68 Copyright 2020, the American Chemical Society. | |
In addition to the use of a bubbler, the use of temperature to control the decomposition of raw materials for the sulfur supply, such as Na2SO4 could regulate the supply of sulfur to successfully synthesize WS2, MoS2 and ZrS2.68 At controlled temperatures above 700 °C, the Na2S generated by the decomposition of Na2SO4 under a reducing atmosphere reacted with WO3 on the substrate to form liquid Na2WO4 and gaseous H2S as a transition metal source and sulfur source (Fig. 5(g)). The gaseous H2S is incorporated into the liquid Na2WO4, causing it to be released slowly to ensure a stable supply of sulfur source, and eventually some of the vaporized Na2WO4 and H2S are transferred to the surface of the substrate to react with each other, synthesizing monolayer WS2 (Fig. 5(h)). Furthermore, high-quality monolayer TMDs can also be obtained by designing new LPI-CVD growth methods to control the gas distribution of the solid chalcogen precursors. By placing the substrate in a one-end-closed inner quartz tube, the sulfur precursor pressure in the growth chamber changes from the gradient distribution presented in the common CVD process to a quasi-static distribution, whose distribution concentration of sulfur precursors on the surface of the substrate changes from high to low. The sulfur atoms will migrate to the energy favorable sites with low potential energy under the guidance of the substrate structure, resulting in the highly oriented growth of TMD domains with excellent electrical properties.69 By precise controlling the precursor mass flux, these ingenious designs provide extremely important references for the future exploration of novel methods for the controlled growth of 2D TMDs.
3. Transition-metal substitution of 2D TMD materials
In the common CVD, it is often difficult to precisely control the mixture of vaporized host precursor with dopant, making the synthesis of high-quality and uniformly doped 2D TMDs a great challenge.70 Alternatively, the LPI-CVD method has the potential to overcome the difficulties of gas-phase control based solid powder and provides a new pathway. The liquid precursor allows precise control of the doping concentration71 and enables uniform mixing of the dopant at the molecular level in the precursor solution, which in turn can be uniformly doped into 2D TMDs. Besides, it results in minimal contamination to the reaction chamber,72 eliminating the influence of circulation residue.
In this section, we summarize the recent advances of atomic doping of 2D TMD monolayer by the LPI-CVD method.
3.1 Regulation of the electrical properties of 2D TMDs
Substitutional doping, where metal cations are replaced by directly introducing different conducting foreign metal atoms, can modulate the electrical properties of TMDs. In the case of the LPI-CVD method, the preparation of liquid-phase precursors plays an important role in precisely controlling the doping concentration, which can be controlled by precisely controlling the concentration of the doping elements in solution to change the electrical properties of 2D TMD materials. In 2019, Qin et al. successfully obtained Nb-doped monolayer WS2 with a transformation from n-type to p-type by tailoring the doping concentrations of Nb in the LPI-CVD method, whose doping concentrations could reach up to >1014 cm−2 (>10% Nb) in WS2 monolayer.73 The scanning transmission electron microscopy (STEM) image of WS2 with 10.3% Nb doping displayed some darker dots, occupying the positions of the W atoms compared to that of the undoped WS2 (Fig. 6(a) and (b)), which confirms that the Nb doping is carried out randomly with in situ atom substitution. Notably, the undoped WS2 FET exhibited distinct n-type behavior (Fig. 6(c)) and 10.3% Nb-doped WS2 FET (Fig. 6(d)) showed a clear indication of hole doping, which proved that the Nb dopants were the ionized acceptors at room temperature. Compared to the Nb element, the V element, as the doping cation, can also adjust the electrical properties of WSe2 monolayers by the LPI-CVD method.74 In contrast to the bipolar transport characteristics exhibited in the WSe2 monolayer, the V-WSe2 monolayer threshold voltage shifted to a positive value and exhibited significant p-type transport characteristics (Fig. 6(e)) with an increase in the V-doping concentration from 1% to 10%, and the charge mobility as well as the hole density increased with an increase in the V-doping concentration. The current switching ratio of the V-WSe2 monolayer was as high as 105 with an increase in the V doping concentration to 4% (Fig. 6(f)).
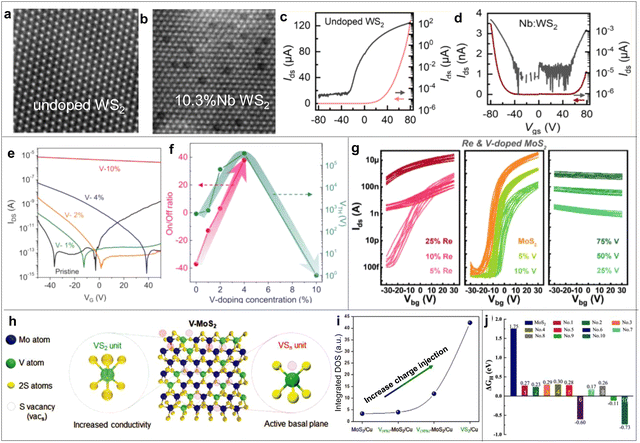 |
| Fig. 6 HAADF-STEM images of undoped (a) and 10.3% Nb-doped (b) WS2 flakes.73 (c) I–V curve of FET based on undoped WS2.73 (d) I–V curve of FET based on 10.3% Nb-doped WS2.73 (e) I–V curve of V-doped WSe2 transistors under VDS = 0.5 V.74 (f) On/off ratio and threshold voltage as a function of V-doping concentration.74 (g) I–V curves of FETs based on Re-doped and V-doped TMDs.75 (h) Illustration of V-MoS2 with VS2 and VSn units.77 (i) Integrated density of states plots for MoS2 at different V-doping.77 (j) Hydrogen adsorption free energy for different defect configurations.78 (a)–(d) Reprinted from ref. 73 Copyright 2019, the American Chemical Society. (e) and (f) Reprinted from ref. 74 Copyright 2019, Wiley-VCH. (g) Reprinted from ref. 75 Copyright 2021, Wiley-VCH. (h)–(i) Reprinted from ref. 77 Copyright 2021, Wiley-VCH. (j) Reprinted from ref. 78 Copyright 2022, Wiley-VCH. | |
Besides Nb and V, other metal atoms such as Re and Fe can be effectively doped in monolayer 2D TMDs to tune their electrical properties.75–78 The Re atom was doped in MoS2 as an electron donor. As the Re doping concentration gradually increased to 25%, the conductivity increased significantly and the threshold voltage shifted sharply to a more negative gate bias, exhibiting n-type transport characteristics (Fig. 6(g)).75 In addition to changing the type of transport properties of 2D TMDs, the introduction of suitable doping atoms in the host material can also improve the electrocatalytic properties of 2D TMDs. Furthermore, metal-atom-doped TMDs can generate additional active sites to regulate the bandgap, manipulating the electrical properties, such as carrier concentration and mobility, which ultimately enhance the hydrogen evolution reaction (HER) catalytic activity. In 2021, Agyapong-Fordjour et al. doped V atoms into semiconducting MoS2 to form nanodispersed metallic vanadium sulfide units via a one-step LPI-CVD method,77 and the resulting V-doped MoS2 exhibited excellent HER catalytic activity. During the growth process, the Mo atoms were replaced by V atoms to form VS2 groups to enhance the electrical conductivity of V-doped MoS2 materials, while V vacancies were created to form VSn groups to introduce additional active sites in the less reactive substrate planes of the MoS2 materials to enhance the adsorption hydrogen (Fig. 6(h)). The DFT calculations yielded a Gibbs free energy of −0.02 eV for VSn groups in V-MoS2 monolayers on copper substrates, which is almost close to 0 eV. This indicates that the V-MoS2 monolayer containing VSn groups has excellent catalytic properties for the HER. In addition, the density of states near the Fermi energy level associated with the active site increases with an increase in V-doping concentration (Fig. 6(i)), which illustrates the increase in the charge transfer rate of V-MoS2, ultimately yielding catalytic properties almost comparable to that of the Pt metal. Similarly, Liu et al. achieved the in situ doping of V atoms in MoS2 monolayers via the LPI-CVD method with the lowest hydrogen adsorption free energy at the defective configuration of three V-doped atoms and S vacancies (Fig. 6(j)), which synergistically improved the catalytic performance of V-MoS2 monolayers for the HER.78 Therefore, the LPI-CVD method offers significant advantages for in situ doping with precisely controlled doping concentrations, in which Mo or W can be replaced by an electron donor and acceptor, leading to a change in electronic structure, carrier types, and conductivity of TMD monolayers. We can envisage the extension of this approach to the introduction of a variety of other doping elements, making it possible to systematically study the fantastic modulation of various electrical properties.
3.2 Regulation of the optical properties of 2D TMDs
Due to their similar atomic structure, the doping concentration of 2D TMD materials can be varied over the entire composition range, thus modulating the energy band structure of 2D TMD materials. An outstanding advantage of the LPI-CVD method over the commonly used CVD preparation methods is the avoidance of inhomogeneous precursor vapor pressure generation. In 2019, Lee et al. successfully achieved the growth of Mo1−xWxS2 monolayer with controlled composition by adjusting the ratio of MoO3 and WO3 using the LPI-CVD method.79 The synthesized Mo1−xWxS2 monolayer had a homogeneous chemical composition. The resulting PL mapping at 1.90 eV showed that the Mo1−xWxS2 monolayers possessed a uniform chemical composition and structural characteristics (Fig. 7(a)). With an increase in the W composition ratio, the PL of the Mo1−xWxS2 monolayers was blue-shifted (Fig. 7(b)). The variation in free excitons in the Mo1−xWxS2 monolayer with temperature agrees well with the Varshni equation, while the bound excitons produce a turning point at 75 K (Fig. 7(c)).
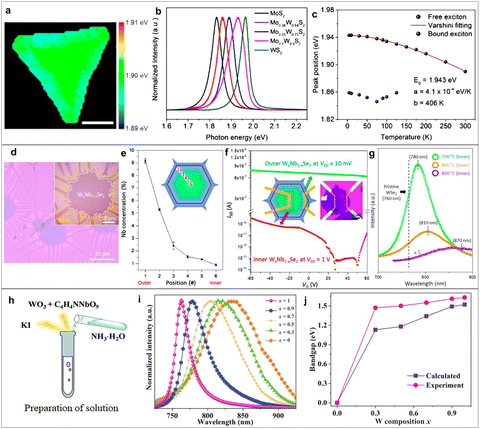 |
| Fig. 7 (a) PL position mapping of Mo1−xWxS2.79 (b) PL spectrum of Mo1−xWxS2.79 (c) Curves of peak position of the free excitons and the bound excitons with temperature.79 (d) Optical image of the NbSe2/WxNb1−xSe2 heterostructure.60 (e) Variation curve of Nb doping concentration with position from edge to inside.60 (f) I–V curve of the outer and the inner WxNb1−xSe2 FET, where the insets show a schematic and optical images of the device.60 (g) PL spectra of the inner WxNb1−xSe2 region at different temperatures.60 (h) Process for the preparation process of precursor solution.39 (i) PL intensity of Nb1−xWxSe2 alloys with different W compositions.39 (j) Curves of band gap variation with the ratio of W components.39 (a)–(c) Reprinted from ref. 79 Copyright 2019, the American Chemical Society. (d)–(g) Reprinted from ref. 60 Copyright 2020, the American Chemical Society. (h)–(j) Reprinted from ref. 39 Copyright 2022, Springer Nature. | |
Polygonal WxNb1−xSe2 domains around 40 μm were successfully synthesized using the LPI-CVD method, which possessed a heterogeneous structure with the outer sea urchin-like NbSe2 domains (Fig. 7(d)–(g)).60 The emission peak of the PL spectrum of the internal WxNb1−xSe2 redshifted to ∼780 nm (Fig. 7(g)) compared to that of pure WSe2. In addition, the Nb atoms act as electron acceptors equivalent to p-type doping. The Fermi energy level moved below the valence band maximum as the proportion of Nb component increased, and the normalized PL peak position shifted continuously from 760 nm to 845 nm, which indicates that the energy band gap of the synthesized monolayer decreased continuously from 1.63 eV to 1.47 eV (Fig. 7(h)–(j)).39 Therefore, the LPI-CVD method is a concise and effective approach for the preparation of alloys with continuously tunable band gaps. However, whether the universal preparation of multiple types of alloys can be achieved requires more efforts in the future.
3.3 Regulation of the magnetic properties of 2D TMDs
Materials with both ferromagnetic and semiconducting properties are called dilute magnetic semiconductors, which have great potential applications in spintronics and magnetoelectrics.80,81 The doping of magnetic atoms such as Fe, Co, Ni, Mn, and V will produce ferromagnetism in non-ferromagnetic 2D TMDs.82–84 Thus, the LPI-CVD method has become one of the most effective methods for the controllable synthesis of substitutional magnetic transition metal-doped atomically thin TMDs. In 2020, Zhang et al. successfully synthesized high-quality V-doped monolayers of WS2 with a wide range of V concentrations from 0 to 12% via the LPI-CVD method (Fig. 8(a) and (b)), which exhibited ferromagnetism at room temperature.85 The ferromagnetism of the V-doped WS2 increased with an increase in V concentration, and the maximum coercivity and saturation magnetization of the V-doped WS2 was obtained when the V doping concentration reached 2% (Fig. 8(c)–(e)).
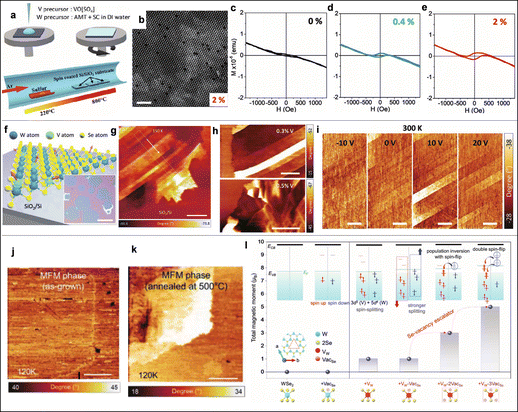 |
| Fig. 8 (a) Schematic of the synthesis of Fe-doped MoSe2.85 (b) HAADF-STEM images of V-doped MoSe2 at 2% concentration.85 (c)–(e) Magnetization versus field loops for pristine (c) and V-doped WS2 at 0.4% (d) and 2% (e) concentration.85 (f) Synthetic schematic of V-doped WSe2, where the inset shows the optical image of V-doped WSe2.86 MFM images of the magnetic domains of V-doped WSe2 with 0.1% (g) and 0.3% and 0.5% (h) concentrations.86 (i) Gate-dependent MFM images for 0.1% V-doped WSe2.86 MFM phase images measured at 120 K in as-grown (j) and annealed (k) V-doped WSe2.40 (l) Image of the variation in the total magnetic moment by DFT for different doping and vacancy binding types.40 (a)–(e) Reprinted from ref. 85 Copyright 2020, Wiley-VCH. (f)–(i) Reprinted from ref. 86 Copyright 2020, Wiley-VCH. (j)–(l) Reprinted from ref. 40 Copyright 2022, Wiley-VCH. | |
V doping produces a more pronounced change in the magnetic properties of WSe2 materials compared to that of V-doped WS2. For example, Yun et al. reported that V-doped WSe2 possessed long-range ordered ferromagnetic domains at room-temperature (Fig. 8(f)).86 The V-WSe2 monolayer exhibited striped magnetic domains at a V doping concentration of 0.1% according to magnetic force microscopy (MFM) tests (Fig. 8(g)). As the doping concentration increased to 5%, the stripe-like domains of the V-WSe2 monolayer clearly changed to polygonal shape and the domain area gradually decreases (Fig. 8(h)). The MFM phase test of 0.1% V-WSe2 at different gate voltages showed that at a gate voltage of −10 V, 0.1% V-WSe2 had almost no magnetic domains (Fig. 8(i)). With a positive shift in the gate voltage to 20 V, domains gradually appeared with stripes, which indicates that the domains of the 0.1% V-WSe2 monolayer can be regulated by the gate and the 0.1% V-WSe2 monolayer is a dilute magnetic semiconductor. Recently, the ferromagnetic order of V-doped WSe2 synthesized by the LPI-CVD method was enhanced through post-annealing treatment.40 Compared to the unannealed V-WSe2 monolayers, the V-WSe2 monolayers clearly produced polygonal-shaped magnetic domains after annealing at 500 °C (Fig. 8(j) and (k)). Before annealing, the doping of the V atom caused spin splitting, and the hybridization of the V-3d and W-5d orbitals produced emerging defect states, which increased the magnetic moment of V-WSe2 by 1 μB, while the paired configuration of VW–VacSe after annealing intensified the spin splitting (Fig. 8(l)), and the strong spin splitting caused by the formation of VW–2VacSe with an increase in the concentration of sulfur vacancies caused population inversion. Additionally, the magnetic moment increased to 3 μB, and in the VW–3VacSe configuration, the magnetic moment increased to 5 μB to reach saturation. Except for V atoms, other magnetic atoms such as Fe, Co, and Ni can also dope the 2D TMDs and modulate the ferromagnetic properties of the materials.87
4. Heterostructures based on 2D TMDs
High-quality lateral heterostructures require atomic-level sharpness at the interface of different 2D materials.88 Controlling the supply sequence of precursors, and thus the orderly growth of different materials in a heterojunction is an important idea for the synthesis of high-quality heterostructures in the LPI-CVD method. High-quality MoS2/WS2 lateral heterostructures have been comprehensively reported using the LPI-CVD method.79,88,89 For example, Chen et al. synthesized large-area MoS2/WS2 lateral heterostructures using H2 as the reducing agent.89 This chemical vapor deposition combined with hydrogen-triggered method could accurately control the precursor source supply period due to the different volatilities between feedstocks. During the growth process, the MoS2 monolayer was firstly grown due to due to the higher volatility of the Mo source compared to WO3 under Ar gas, followed by the reduction of WO3 to highly volatile WO3−x after the introduction of H2, and WS2 started to grow seamlessly around MoS2, forming a high-quality MoS2/WS2 lateral heterostructure (Fig. 9(a)). It is clear that the MoS2/WS2 lateral heterostructure consisted of seamlessly stitched MoS2 and WS2 regions with atomically sharp interfaces (Fig. 9(b)–(d)). Controlling the supply sequence of different precursor sources by using the difference in the volatility of precursor raw materials is one of the common methods for growing heterostructures currently; however, controlling the key parameters in CVD growth is equally important for the synthesis of heterostructure. Consequently, lateral heterostructures (MoS2/WS2, MoS2/alloy, and alloy/WS2) have been successfully synthesized by precisely controlling key factors such as the relative supersaturation degree of the precursor, the sulfur vaporization temperature, and the growth temperature (Fig. 9(e)–(h)).79 Depending on the physical or chemical properties of the precursor source, the order of precursor supply is regulated by strictly controlling the growth temperature to selectively grow high-quality lateral/vertical heterostructures.90 In the LPI-CVD method, the growth temperature can regulate the adsorption or dissociation state of OH− ions on the monolayer MoS2 surface.91 When the growth temperature reached 780 °C (Fig. 9(i)), OH− ions were fully adsorbed on the surface of the monolayer MoS2, and the formed OH− ion layer passivated the MoS2 surface, preventing the W atoms from nucleating on the MoS2 surface for vertical growth, and eventually, the W atoms nucleated at the edge of MoS2 to form the WS2/MoS2 lateral heterostructure with atomically sharp interfaces (Fig. 9(j)). As the temperature increased, the OH− ions dissociated from the surface of monolayer MoS2, leading to the exposure of surface of monolayer MoS2. To reduce the total energy of the WS2/MoS2 heterostructure, WS2 preferentially nucleated on the surface of MoS2, resulting in high-quality WS2/MoS2 vertical heterostructure (Fig. 9(k)).
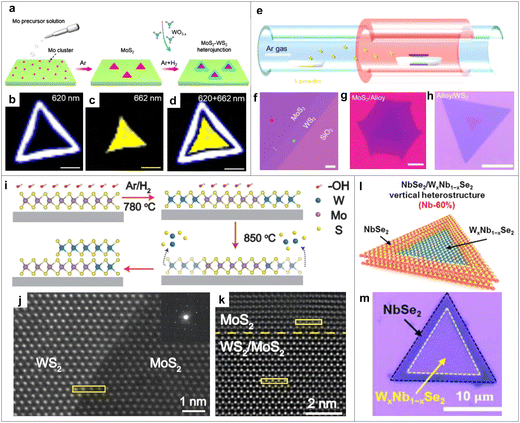 |
| Fig. 9 (a) Synthetic schematic of MoS2/WS2 lateral heterostructures.89 (b)–(d) PL intensity mapping of MoS2/WS2 lateral heterostructures.89 (e) Synthetic schematic of heterostructures and alloy.79 (f)–(h) Optical images between MoS2/WS2 (f), MoS2/alloy (g), alloy/WS2 and (h) lateral heterostructures interfaces.79 (i) Schematic of selective growth of WS2/MoS2 lateral and vertical heterostructures.91 (j) HAADF-STEM image of WS2/MoS2 lateral heterostructures.91 (k) HAADF-STEM image of WS2/MoS2 vertical heterostructures.91 (l) Atomic structure model of NbSe2/WxNb1−xSe2 vdWHs with 60% concentration.93 (m) Optical microscopy of NbSe2/WxNb1−xSe2 vdWHs with 60% concentration.93 (a)–(d) Reprinted from ref. 89 Copyright 2021, the American Chemical Society. (e)–(h) Reprinted from ref. 79 Copyright 2019, the American Chemical Society. (i)–(k) Reprinted from ref. 91 Copyright 2020, the American Chemical Society. (l)–(m) Reprinted from ref. 93 Copyright 2021, the American Chemical Society. | |
Changing the growth temperature can affect the thermodynamic tendency of growth during the synthesis of heterostructures, for example, Bai et al. reported a two-step heating process to grow MoS2 monolayers at lower temperatures, while growing WS2 monolayers on the surface of MoS2 at high temperatures due to the large energy potential barrier by the LPI-CVD method, resulting in the formation of stable MoS2/WS2 vertical heterostructures with an orientation difference of 60°.92 Recently, NbSe2/WxNb1−xSe2 van der Waals vertical heterostructures (vdWHs) were synthesized using a one-step LPI-CVD growth method.93 As the temperature increased, the Nb precursor evaporated and absorbed enough energy to overcome the substrate surface energy barrier, preferentially nucleating the growth at the edge of the monolayer Nb-doped WSe2 (Fig. 9(l)), followed by extension to the middle part, and finally completing coverage to achieve the growth of NbSe2/WxNb1−xSe2 van der Waals vertical heterostructures, and the optical images are shown in Fig. 9(m). Notably, the energy band structure of the constructed vertical/lateral heterostructures can be modulated by changing the type of 2D materials, and thus the electrical properties of the constructed electronic devices. To expand the application of these materials in electronic and optoelectronic electronic devices, more efforts are needed to develop more generalized LPI-CVD methods for the synthesis of heterostructures.70,94
5. Summary and outlook
In this review, we mainly overviewed the recent research on the LPI-CVD growth of 2D TMDs, including monolayer 2D TMDs, with doping and heterostructures. The precursor solution in the LPI-CVD process enables the homogeneous mixing of multiple precursors at the molecular level to facilitate the growth of wafer-scale 2D TMDs.95 For the controllable growth of larger area and higher quality TMDs with more excellent physical performance, various assisted treatments based on LPI-CVD strategies were implemented mainly including molten-salt assisted, hydroxide-assisted, polymer-assisted, and supply of novel chalcogen precursor LPI-CVD. In addition, the recent progress of atomically doped monolayer 2D TMDs was summarized. Furthermore, we further elaborated the latest progress on the growth of heterostructures based on LPI-CVD. Notably, although the LPI-CVD method has shown great potential for the high-quality growth of atomically thin 2D TMDs, doped 2D TMDs and heterostructures, the growth of 2D TMDs via LPI-CVD is still in infancy and faces some challenges, as follows:
(I) At present, in the huge TMD family, only a small number of TMDs such as MoS2, MoSe2, and WSe2 has been successfully grown in high quality by the LPI-CVD method. However, the LPI-CVD method is strongly dependent on the precursor salts of each element, as shown in the Table 1, which can be used as precursors to grow more 2D TMDs. Therefore, more efforts need to be devoted to finding various assisted treatments such as organic solvents (Fig. 10(a)) and other co-solvents to promote the dissolution and reaction of salts for the growth of more 2D TMDs.
Table 1 Periodic table of soluble salts for the synthesis of 2D TMDs
IVB |
VB |
VIB |
VIIB |
VIIIB |
IB |
IIIA |
IVA |
VA |
VIA |
22Ti (d2s2) |
23V (d3s2) |
24Cr (d5s1) |
25Mn (d5s2) |
26Fe (d6s2) |
27Co (d7s2) |
28Ni (d8s2) |
29Cu (d10s1) |
31Ga (s2p1) |
32Ge (s2p2) |
|
16S (s2p4) |
TiOSO4 |
VOSO4·xH2O |
Na2CrO4 |
MnSO4 |
C6H12FeN3O12·3H2O |
Co(NH2SO3)2·xH2O |
NiC4H6O4·4H2O |
Cu(NO3)2 |
Ga2(SeO4)3·16H2O |
Ge(OH)4 |
|
(NH)2SO4 |
C4K2O9Ti·2H2O |
NH4VO3 |
|
MnCl2 |
|
C4H6CoO4·4H2O |
Ni(SO3NH2)2·4H2O |
Cu(ClO3)2 |
|
|
|
|
NaVO3 |
|
|
|
|
|
|
|
|
|
|
40Zr (d2s2) |
41Nb (d4s1) |
42Mo (d5s1) |
|
|
|
46Pd (d10) |
|
49In (s2p1) |
50Sn (s2p2) |
51Sb (s2p3) |
34Se (s2p4) |
Zr(SO4)2·4H2O |
C10H5NbO20·xH2O |
H24Mo7N6O24·4H2O |
|
|
|
Pd(C2H3O2)2 |
|
InBr3 |
SnF2 |
SbF3 |
Na2SeO3 |
C4H4NNbO9·nH2O |
Na2Mo2O7 |
|
|
|
Pd(NO3)2 |
|
InCl3 |
SnSO4 |
SbCl3 |
Nb(HC2O4)5 |
Na2MoO4 |
|
|
|
|
|
|
|
|
|
K2MoS4 |
|
|
|
|
|
|
|
|
|
(NH4)6Mo7O24·4H2O |
|
|
|
|
|
|
|
|
72Hf (d2s2) |
73Ta (d3s2) |
74W (d4s2) |
75Re (d5s2) |
|
|
78Pt (d9s1) |
|
|
|
83Bi (s2p3) |
52Te (s2p4) |
Hf(OH)3 |
TaCl5 |
Na2WO4 |
NaReO4 |
|
|
H2PtCl6 |
BiI3 |
Na2TeO3 |
Na2W4O13 |
|
|
Bi(OH)3 |
(NH4)10W12O41 |
|
|
Bi(NO3)3·5H2O |
(NH4)6W12O39·2H2O |
|
|
|
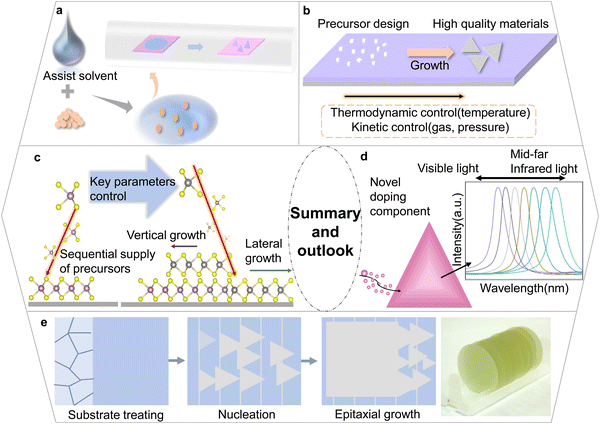 |
| Fig. 10 (a) Growth process using other assistant solvents or promoters. (b) Application of precursor design and multi-condition to regulate the growth process. (c) Control of key parameters regulating the sequential supply of precursors and selection for the growth of vertical or lateral heterostructures. (d) Doping with other novel elements to modulate the alloy band gap from visible to mid- and far-infrared light range. (e) Pre-treating substrates to control domain unidirectional growth and seamlessly stitch together wafer-scale monolayer films. (e) Reprinted from ref. 96 Copyright 2017, the American Chemical Society. | |
(II) The quality of 2D TMDs obtained by LPI-CVD growth is still inferior compared to that produced by mechanical exfoliation. For example, excessive or insufficient spin coating of the precursor solutions can lead to a dense nucleation density or insufficient source supply during growth, producing unavoidable defects. Therefore, more precise precursor design (Fig. 10(b)) and the reaction processes of serial reactions should be drastically implemented to improve the high-quality growth of 2D TMDs.
(III) The mechanism of controlled lateral/vertical heterostructure growth remains unclear, although that produced by LPI-CVD methods is advantageous. The regulation of key parameters such as the growth temperature and gas flow during growth is essentially the regulation of the growth thermodynamics and growth kinetic competition between the precursor materials. The intrinsic growth mechanism (Fig. 10(c)) should be understood in depth to develop new synthetic methods that can precisely control the order of precursor introduction to achieve the growth of multiple high-quality lateral heterostructures.
(IV) Controllable doping of 2D TMDs still needs further exploration. Although controllable doping such as WxNb1−xSe2 can effectively regulate the electrical and optical properties of TMDs, the range of band gap regulation is limited such as 1.47–1.63 eV. The LPI-CVD method plays an important role in growing doped 2D TMDs, and therefore exploring novel doping components (such as Re, In, and Te) for the LPI-CVD method is urgently required to control the growth of lateral composition-graded atomic-layered 2D TMD nanosheets with tunable bandgaps from the visible to mid-to-far infrared range (Fig. 10(d)).
(V) The growth of large-area continuity, large domain size, and thickness uniformity 2D semiconductors, as the prerequisite of industrial optoelectronic integration application, is still very challenging.96 Although the LPI-CVD method has great potential for the growth of 2D TMDs, the preparation of wafer-scale size especially large single crystals of 2D TMDs still in its infancy. Therefore, given the centimeter-scale epitaxy of graphene and MoS2 on annealed copper foil or sapphire, searching for substrates designed for good contact with the precursor solutions, and annealing or other treatment can enable the seamless stitching of unidirectionally aligned domains into wafer-scale single-crystal films (Fig. 10(e)) by suppressing the nucleation rate and edge induction as a path worth exploring.
Conflicts of interest
There are no conflicts to declare.
Acknowledgements
This work was supported by the National Key Research and Development Program of China (2021YFA1200700), the National Natural Science Foundation of China (62225404, 61927808, 62174026, 6507024076), and the Strategic Priority Research Program of Chinese Academy of Sciences (XDB30000000).
Notes and references
- Z. Wang, H.-H. Wu, Q. Li, F. Besenbacher, X. C. Zeng and M. Dong, Nanoscale, 2018, 10, 18178–18185 RSC.
- Z. Wang, H.-H. Wu, Q. Li, F. Besenbacher, Y. Li, X. C. Zeng and M. Dong, Adv. Sci., 2020, 7, 1901382 CrossRef CAS PubMed.
- Z. Hu, X. Kuai, J. Chen, P. Sun, Q. Zhang, H.-H. Wu and L. Zhang, ChemSusChem, 2020, 13, 1485–1490 CrossRef CAS PubMed.
- K. S. Novoselov, A. K. Geim, S. V. Morozov, D. Jiang, Y. Zhang, S. V. Dubonos, I. V. Grigorieva and A. A. Firsov, Science, 2004, 306, 666–669 CrossRef CAS PubMed.
- H. J. Conley, B. Wang, J. I. Ziegler, R. F. Haglund, Jr., S. T. Pantelides and K. I. Bolotin, Nano Lett., 2013, 13, 3626–3630 CrossRef CAS PubMed.
- S. Mouri, Y. Miyauchi and K. Matsuda, Nano Lett., 2013, 13, 5944–5948 CrossRef CAS PubMed.
- I. Datta, S. H. Chae, G. R. Bhatt, M. A. Tadayon, B. Li, Y. Yu, C. Park, J. Park, L. Cao, D. N. Basov, J. Hone and M. Lipson, Nat. Photonics, 2020, 14, 256–262 CrossRef CAS.
- S. B. Desai, S. R. Madhvapathy, A. B. Sachid, J. P. Llinas, Q. Wang, G. H. Ahn, G. Pitner, M. J. Kim, J. Bokor and C. Hu, Science, 2016, 354, 99 CrossRef CAS PubMed.
- Y. Zhang, Y.-W. Tan, H. L. Stormer and P. Kim, Nature, 2005, 438, 201–204 CrossRef CAS PubMed.
- Q. Li, X. Zhao, L. Deng, Z. Shi, S. Liu, Q. Wei, L. Zhang, Y. Cheng, L. Zhang, H. Lu, W. Gao, W. Huang, C. W. Qiu, G. Xiang, S. J. Pennycook, Q. Xiong, K. P. Loh and B. Peng, ACS Nano, 2020, 14, 4636–4645 CrossRef CAS PubMed.
- B. Zhao, Z. Wan, Y. Liu, J. Xu, X. Yang, D. Shen, Z. Zhang, C. Guo, Q. Qian, J. Li, R. Wu, Z. Lin, X. Yan, B. Li, Z. Zhang, H. Ma, B. Li, X. Chen, Y. Qiao, I. Shakir, Z. Almutairi, F. Wei, Y. Zhang, X. Pan, Y. Huang, Y. Ping, X. Duan and X. Duan, Nature, 2021, 591, 385–390 CrossRef CAS PubMed.
- S. B. Desai, G. Seol, J. S. Kang, H. Fang, C. Battaglia, R. Kapadia, J. W. Ager, J. Guo and A. Javey, Nano Lett., 2014, 14, 4592–4597 CrossRef CAS PubMed.
- J. Xia, X. Huang, L. Z. Liu, M. Wang, L. Wang, B. Huang, D. D. Zhu, J. J. Li, C. Z. Gu and X. M. Meng, Nanoscale, 2014, 6, 8949–8955 RSC.
- X. Chen, Y. Qiu, G. Liu, W. Zheng, W. Feng, F. Gao, W. Cao, Y. Fu, W. Hu and P. Hu, J. Mater. Chem. A, 2017, 5, 11357–11363 RSC.
- C. Xie, C. Mak, X. Tao and F. Yan, Adv. Funct. Mater., 2017, 27, 1603886 CrossRef.
- S. Y. Wang, T. S. Ko, C. C. Huang, D. Y. Lin and Y. S. Huang, Jpn. J. Appl. Phys., 2014, 53, 04EH07 CrossRef CAS.
- X. Lin, W. Yang, K. L. Wang and W. Zhao, Nat. Electron., 2019, 2, 274–283 CrossRef CAS.
- W. S. Yun and J. D. Lee, Phys. Chem. Chem. Phys., 2014, 16, 8990–8996 RSC.
- B. Zhao, W. Dang, Y. Liu, B. Li, J. Li, J. Luo, Z. Zhang, R. Wu, H. Ma, G. Sun, Y. Huang, X. Duan and X. Duan, J. Am. Chem. Soc., 2018, 140, 14217–14223 CrossRef CAS PubMed.
- Z. Cai, B. Liu, X. Zou and H. M. Cheng, Chem. Rev., 2018, 118, 6091–6133 CrossRef CAS PubMed.
- C. H. Naylor, W. M. Parkin, J. Ping, Z. Gao and A. T. C. Johnson, Nano Lett., 2016, 16, 4297–4304 CrossRef CAS PubMed.
- X. Tong, K. Liu, M. Zeng and L. Fu, InfoMat, 2019, 1, 460–478 CrossRef CAS.
- J. H. Lee, E. K. Lee, W. J. Joo, Y. Jang, B. S. Kim, J. Y. Lim, S. H. Choi, S. J. Ahn, J. R. Ahn, M. H. Park, C. W. Yang, B. L. Choi, S. W. Hwang and D. Whang, Science, 2014, 344, 286–289 CrossRef CAS PubMed.
- T. Zhang and L. Fu, Chem, 2018, 4, 671–689 CAS.
- T. Li, W. Guo, L. Ma, W. Li, Z. Yu, Z. Han, S. Gao, L. Liu, D. Fan, Z. Wang, Y. Yang, W. Lin, Z. Luo, X. Chen, N. Dai, X. Tu, D. Pan, Y. Yao, P. Wang, Y. Nie, J. Wang, Y. Shi and X. Wang, Nat. Nanotechnol., 2021, 16, 1201–1207 CrossRef CAS PubMed.
- J. C. Shaw, H. Zhou, Y. Chen, N. O. Weiss, Y. Liu, Y. Huang and X. Duan, Nano Res., 2015, 7, 511–517 CrossRef.
- S. H. Choi, S. Boandoh, Y. H. Lee, J. S. Lee, J. H. Park, S. M. Kim, W. Yang and K. K. Kim, ACS Appl. Mater. Interfaces, 2017, 9, 43021–43029 CrossRef CAS PubMed.
- S. Li, iScience, 2021, 24, 103229 CrossRef CAS PubMed.
- P. Braeuninger-Weimer, B. Brennan, A. J. Pollard and S. Hofmann, Chem. Mater., 2016, 28, 8905–8915 CrossRef CAS PubMed.
- P. Yang, S. Zhang, S. Pan, B. Tang, Y. Liang, X. Zhao, Z. Zhang, J. Shi, Y. Huan, Y. Shi, S.
J. Pennycook, Z. Ren, G. Zhang, Q. Chen, X. Zou, Z. Liu and Y. Zhang, ACS Nano, 2020, 14, 5036–5045 CrossRef CAS PubMed.
- C. Chang, W. Chen, Y. Chen, Y. H. Chen, Y. Chen, F. Ding, C. H. Fan, H. J. Fan, Z. X. Fan, C. Gong, Y. J. Gong, Q. Y. He, X. Hong, S. Hu, W. D. Hu, W. Huang, Y. Huang, W. Ji, D. H. Li, L. J. Li, Q. Li, L. Lin, C. Y. Ling, M. H. Liu, N. Liu, Z. Liu, K. P. Loh, J. M. Ma, F. Miao, H. L. Peng, M. F. Shao, L. Song, S. Su, S. Sun, C. L. Tan, Z. Y. Tang, D. S. Wang, H. Wang, J. L. Wang, X. Wang, X. R. Wang, A. T. S. Wee, Z. M. Wei, Y. E. Wu, Z. S. Wu, J. Xiong, Q. H. Xiong, W. G. Xu, P. Yin, H. B. Zeng, Z. Y. Zeng, T. Y. Zhai, H. Zhang, H. Zhang, Q. C. Zhang, T. R. Zhang, X. Zhang, L. D. Zhao, M. T. Zhao, W. J. Zhao, Y. X. Zhao, K. G. Zhou, X. Zhou, Y. Zhou, H. W. Zhu, H. Zhang and Z. F. Liu, Acta. Phys.-Chim. Sin., 2021, 37(12), 2108017 Search PubMed.
- T. Carey, S. Cacovich, G. Divitini, J. Ren, A. Mansouri, J. M. Kim, C. Wang, C. Ducati, R. Sordan and F. Torrisi, Nat. Commun., 2017, 8, 1202 CrossRef PubMed.
- X. Gao, G. Bian and J. Zhu, J. Mater. Chem. C, 2019, 7, 12835–12861 RSC.
- Y. Jeon, J. Seo, J. Kim, D. Rhee, M. Jung, H. Park and J. Kang, Adv. Opt. Mater., 2022, 10, 2101492 CrossRef CAS.
- B. Zhao, D. Shen, Z. Zhang, P. Lu, M. Hossain, J. Li, B. Li and X. Duan, Adv. Funct. Mater., 2021, 31, 2105132 CrossRef CAS.
- H. Cun, M. Macha, H. Kim, K. Liu, Y. Zhao, T. LaGrange, A. Kis and A. Radenovic, Nano Res., 2019, 12, 2646–2652 CrossRef CAS.
- D. Zhang, C. Wen, J. B. McClimon, P. Masih Das, Q. Zhang, G. A. Leone, S. V. Mandyam, M. Drndić, A. T. C. Johnson and M. Q. Zhao, Adv. Electron. Mater., 2021, 7, 2001219 CrossRef CAS PubMed.
- H. Liu, G. Qi, C. Tang, M. Chen, Y. Chen, Z. Shu, H. Xiang, Y. Jin, S. Wang, H. Li, M. Ouzounian, T. S. Hu, H. Duan, S. Li, Z. Han and S. Liu, ACS. Appl. Mater. Interfaces, 2020, 12, 13174–13181 CrossRef CAS PubMed.
- B. An, Y. Ma, F. Chu, X. Li, Y. Wu, C. You, W. Deng, S. Li and Y. Zhang, Nano Res., 2022, 15, 2608–2615 CrossRef CAS.
- S. J. Yun, B. W. Cho, T. Dinesh, D. H. Yang, Y. I. Kim, J. W. Jin, S.-H. Yang, T. D. Nguyen, Y.-M. Kim, K. K. Kim, D. L. Duong, S.-G. Kim and Y. H. Lee, Adv. Mater., 2022, 34, 2106551 CrossRef CAS PubMed.
- S. Wang, Y. Zhang, D. Zhao, J. Li, H. Kang, S. Zhao, T. Jin, J. Zhang, Z. Xue, Y. Wang, Y. Sui, Z. Chen, S. Peng, Z. Jin, X. Liu, J. Wang, Y. Chen and G. Yu, 2D Mater., 2021, 9, 015016 CrossRef.
- O. Lopez-Sanchez, D. Lembke, M. Kayci, A. Radenovic and A. Kis, Nat. Nanotechnol., 2013, 8, 497–501 CrossRef CAS PubMed.
- Z. Yan, J. Lin, Z. W. Peng, Z. Z. Sun, Y. Zhu, L. Li, C. S. Xiang, E. L. Samuel, C. Kittrell and J. M. Tour, ACS Nano, 2013, 7, 2872 CrossRef CAS.
- S. Li, S. Wang, D.-M. Tang, W. Zhao, H. Xu, L. Chu, Y. Bando, D. Golberg and G. Eda, Appl. Mater. Today., 2015, 1, 60–66 CrossRef.
- U. G. Lee, W.-B. Kim, D. H. Han and H. S. Chung, Symmetry, 2019, 11, 1183 CrossRef CAS.
- Y. Zuo, W. Yu, C. Liu, X. Cheng, R. Qiao, J. Liang, X. Zhou, J. Wang, M. Wu, Y. Zhao, P. Gao, S. Wu, Z. Sun, K. Liu, X. Bai and Z. Liu, Nat. Nanotechnol., 2020, 15, 987–991 CrossRef CAS PubMed.
- Q. Wang, N. Li, J. Tang, J. Zhu, Q. Zhang, Q. Jia, Y. Lu, Z. Wei, H. Yu, Y. Zhao, Y. Guo, L. Gu, G. Sun, W. Yang, R. Yang, D. Shi and G. Zhang, Nano Lett., 2020, 20, 7193–7199 CrossRef CAS PubMed.
- C. Song, G. Noh, T. S. Kim, M. Kang, H. Song, A. Ham, M.-K. Jo, S. Cho, H.-J. Chai, S. R. Cho, K. Cho, J. Park, S. Song, I. Song, S. Bang, J. Y. Kwak and K. Kang, ACS Nano, 2020, 14, 16266–16300 CrossRef CAS PubMed.
- J. Wang, T. Li, Q. Wang, W. Wang, R. Shi, N. Wang, A. Amini and C. Cheng, Mater. Today. Adv., 2020, 8, 100098 CrossRef.
- K. K. Liu, W. Zhang, Y. H. Lee, Y. C. Lin, M. T. Chang, C. Y. Su, C. S. Chang, H. Li, Y. Shi, H. Zhang, C. S. Lai and L. J. Li, Nano Lett., 2012, 12, 1538–1544 CrossRef CAS PubMed.
- A. S. George, Z. Mutlu, R. Ionescu, R. J. Wu, J. S. Jeong, H. H. Bay, Y. Chai, K. A. Mkhoyan, M. Ozkan and C. S. Ozkan, Adv. Funct. Mater., 2014, 24, 7461–7466 CrossRef CAS.
- S. Li, Y. C. Lin, X. Y. Liu, Z. Hu, J. Wu, H. Nakajima, S. Liu, T. Okazaki, W. Chen, T. Minari, Y. Sakuma, K. Tsukagoshi, K. Suenaga, T. Taniguchi and M. Osada, Nanoscale, 2019, 11, 16122–16129 RSC.
- C. Zhang, J. Wu, Y. Sun, C. Tan, T. Li, T. Tu, Y. Zhang, Y. Liang, X. Zhou, P. Gao and H. Peng, J. Am. Chem. Soc., 2020, 142, 2726–2731 CrossRef CAS PubMed.
- X. Wan, X. Miao, J. Yao, S. Wang, F. Shao, S. Xiao, R. Zhan, K. Chen, X. Zeng, X. Gu and J. Xu, Adv. Mater., 2021, 33, e2100260 CrossRef PubMed.
- Q. Ji, C. Su, N. Mao, X. Tian, J.-C. Idrobo, J. Miao, W. A. Tisdale, A. Zettl, J. Li and J. Kong, Sci. Adv., 2021, 7, eabj3274 CrossRef CAS PubMed.
- M. Kim, J. Seo, J. Kim, J. S. Moon and H. Park, ACS Nano, 2021, 15, 3038–3046 CrossRef CAS PubMed.
- S. Li, Y.-C. Lin, J. Hong, B. Gao, H. Lim, X. Yang, S. Liu, Y. Tateyama, K. Tsukagoshi, Y. Sakuma, K. Suenaga and T. Taniguchi, Chem. Mater., 2021, 33, 7301–7308 CrossRef CAS.
- D. Jiang, X. Wang, R. Chen, J. Sun, H. Kang, D. Ji, Y. Liu and D. Wei, J. Am. Chem. Soc., 2022, 144, 8746–8755 CrossRef CAS PubMed.
- J. Zhu, H. Xu, G. Zou, W. Zhang, R. Chai, J. Choi, J. Wu, H. Liu, G. Shen and H. Fan, J. Am. Chem. Soc., 2019, 141, 5392–5401 CrossRef CAS PubMed.
- S. Park, S. J. Yun, Y. I. Kim, J. H. Kim, Y. M. Kim, K. K. Kim and Y. H. Lee, ACS Nano, 2020, 14, 8784–8792 CrossRef CAS PubMed.
- L. Ma, J. Zhu, W. Li, R. Huang and G. Zou, J. Am. Chem. Soc., 2021, 143, 13314–13324 CrossRef CAS PubMed.
- J. Yang, Y. Gu, E. Lee, H. Lee, S. H. Park, M. H. Cho, Y. H. Kim, Y. H. Kim and H. Kim, Nanoscale, 2015, 7, 9311–9319 RSC.
- H. Yang, A. Giri, S. Moon, S. Shin, J.-M. Myoung and U. Jeong, Chem. Mater., 2017, 29, 5772–5776 CrossRef CAS.
- R. Ionescu, B. Campbell, R. Wu, E. Aytan, A. Patalano, I. Ruiz, S. W. Howell, A. E. McDonald, T. E. Beechem, K. A. Mkhoyan, M. Ozkan and C. S. Ozkan, Sci. Rep., 2017, 7, 6419 CrossRef PubMed.
- J. Kim, H. Seung, D. Kang, J. Kim, H. Bae, H. Park, S. Kang, C. Choi, B. K. Choi, J. S. Kim, T. Hyeon, H. Lee, D. H. Kim, S. Shim and J. Park, Nano Lett., 2021, 21, 9153–9163 CrossRef CAS PubMed.
- S. Boandoh, S. H. Choi, J. H. Park, S. Y. Park, S. Bang, M. S. Jeong, J. S. Lee, H. J. Kim, W. Yang, J. Y. Choi, S. M. Kim and K. K. Kim, Small, 2017, 13, 1701306 CrossRef PubMed.
- S. Feng, J. Tan, S. Zhao, S. Zhang, U. Khan, L. Tang, X. Zou, J. Lin, H. M. Cheng and B. Liu, Small, 2020, 16, 2003357 CrossRef CAS PubMed.
- Y. Jin, M. Cheng, H. Liu, M. Ouzounian, T. S. Hu, B. You, G. Shao, X. Liu, Y. Liu, H. Li, S. Li, J. Guan and S. Liu, Chem. Mater., 2020, 32, 5616–5625 CrossRef CAS.
- J. Seo, J. Lee, S. Baek, W. Jung, N. K. Oh, E. Son and H. Park, ACS Appl. Electron. Mater., 2021, 3, 5528–5536 CrossRef CAS.
- J. Zou, Z. Cai, Y. Lai, J. Tan, R. Zhang, S. Feng, G. Wang, J. Lin, B. Liu and H. M. Cheng, ACS Nano, 2021, 15, 7340–7347 CrossRef CAS PubMed.
- J. Zhang, Y. Zhu, M. Tebyetekerwa, D. Li, D. Liu, W. Lei, L. Wang, Y. Zhang and Y. Lu, ACS Appl. Nano. Mater., 2020, 4, 769–777 CrossRef.
- K. Zhang, B. M. Bersch, J. Joshi, R. Addou, C. R. Cormier, C. Zhang, K. Xu, N. C. Briggs, K. Wang, S. Subramanian, K. Cho, S. Fullerton-Shirey, R. M. Wallace, P. M. Vora and J. A. Robinson, Adv. Funct. Mater., 2018, 28, 1706950 CrossRef.
- Z. Qin, L. Loh, J. Wang, X. Xu and G. Eda, ACS Nano, 2019, 13, 10768–10775 CrossRef CAS PubMed.
- S. Fan, S. J. Yun, W. J. Yu and Y. H. Lee, Adv. Sci., 2020, 7, 1902751 CrossRef CAS PubMed.
- S. Li, J. Hong, B. Gao, Y. C. Lin, H. E. Lim, X. Lu, J. Wu, S. Liu, Y. Tateyama, Y. Sakuma, K. Tsukagoshi, K. Suenaga and T. Taniguchi, Adv. Sci., 2021, 8, e2004438 CrossRef PubMed.
- T. Zhang, K. Fujisawa, F. Zhang, M. Liu, M. C. Lucking, R. N. Gontijo, Y. Lei, H. Liu, K. Crust, T. Granzier-Nakajima, H. Terrones, A. L. Elias and M. Terrones, ACS Nano, 2020, 14, 4326–4335 CrossRef CAS PubMed.
- F. O. Agyapong-Fordjour, S. J. Yun, H. J. Kim, W. Choi, B. Kirubasankar, S. H. Choi, L. A. Adofo, S. Boandoh, Y. I. Kim, S. M. Kim, Y. M. Kim, Y. H. Lee, Y. K. Han and K. K. Kim, Adv. Sci., 2021, 8, e2003709 CrossRef PubMed.
- X. Liu, X. Jiang, G. Shao, H. Xiang, Z. Li, Y. Jin, Y. Chen, H. Jiang, H. Li, J. Shui, Y. Feng and S. Liu, Small, 2022, 18, 2200601 CrossRef CAS PubMed.
- J. Lee, S. Pak, Y. W. Lee, Y. Park, A. R. Jang, J. Hong, Y. Cho, B. Hou, S. Lee, H. Y. Jeong, H. S. Shin, S. M. Morris, S. Cha, J. I. Sohn and J. M. Kim, ACS Nano, 2019, 13, 13047–13055 CrossRef CAS PubMed.
- L. Yang, H. Wu, L. Zhang, G. Zhang, H. Li, W. Jin, W. Zhang and H. Chang, ACS Appl. Mater. Interfaces, 2021, 13, 31880–31890 CrossRef CAS PubMed.
- B. Li, T. Xing, M. Zhong, L. Huang, N. Lei, J. Zhang, J. Li and Z. Wei, Nat. Commun., 2017, 8, 1958 CrossRef PubMed.
- L. Yang, H. Wu, L. Zhang, W. Zhang, L. Li, T. Kawakami, K. Sugawara, T. Sato, G. Zhang, P. Gao, Y. Muhammad, X. Wen, B. Tao, F. Guo and H. Chang, Adv. Funct. Mater., 2021, 31, 2008116 CrossRef CAS.
- T. Dietl and H. Ohno, Rev. Mod. Phys., 2014, 86, 187–251 CrossRef CAS.
- X. L. Fan, Y. R. An and W. J. Guo, Nanoscale Res. Lett., 2016, 11, 154 CrossRef PubMed.
- F. Zhang, B. Zheng, A. Sebastian, D. H. Olson, M. Liu, K. Fujisawa, Y. T. H. Pham, V. O. Jimenez, V. Kalappattil, L. Miao, T. Zhang, R. Pendurthi, Y. Lei, A. L. Elias, Y. Wang, N. Alem, P. E. Hopkins, S. Das, V. H. Crespi, M.-H. Phan and M. Terrones, Adv. Sci., 2020, 7, 2001174 CrossRef CAS PubMed.
- S. J. Yun, D. L. Duong, D. M. Ha, K. Singh, T. L. Phan, W. Choi, Y.-M. Kim and Y. H. Lee, Adv. Sci., 2020, 7, 1903076 CrossRef CAS PubMed.
- D. Shen, B. Zhao, Z. Zhang, H. Zhang, X. Yang, Z. Huang, B. Li, R. Song, Y. Jin, R. Wu, B. Li, J. Li and X. Duan, ACS Nano, 2022, 16, 10623–10631 CrossRef CAS PubMed.
- K. Chen, X. Wan, W. Xie, J. Wen, Z. Kang, X. Zeng, H. Chen and J. Xu, Adv. Mater., 2015, 27, 6431–6437 CrossRef CAS PubMed.
- C. Chen, Y. Yang, X. Zhou, W. X. Xu, Q. N. Cui, J. B. Lu, H. M. Jing, D. Tian, C. X. Xu, T. Y. Zhai and H. Xu, ACS Appl. Nano Mater., 2021, 4, 5522–5530 CrossRef CAS.
- A. Y. Lu, H. Zhu, J. Xiao, C. P. Chuu, Y. Han, M. H. Chiu, C. C. Cheng, C. W. Yang, K. H. Wei, Y. Yang, Y. Wang, D. Sokaras, D. Nordlund, P. Yang, D. A. Muller, M. Y. Chou, X. Zhang and L. J. Li, Nat. Nanotechnol., 2017, 12, 744–749 CrossRef CAS PubMed.
- J. Zhu, W. Li, R. Huang, L. Ma, H. Sun, J. H. Choi, L. Zhang, Y. Cui and G. Zou, J. Am. Chem. Soc., 2020, 142, 16276–16284 CrossRef CAS PubMed.
- X. Bai, S. Li, S. Das, L. Du, Y. Dai, L. Yao, R. Raju, M. Du, H. Lipsanen and Z. Sun, Nanoscale, 2021, 13, 4537–4542 RSC.
- V. T. Vu, T. T. H. Vu, T. L. Phan, W. T. Kang, Y. R. Kim, M. D. Tran, H. T. T. Nguyen, Y. H. Lee and W. J. Yu, ACS Nano, 2021, 15, 13031–13040 CrossRef CAS PubMed.
- C. Li, J. Zhu, W. Du, Y. Huang, H. Xu, Z. Zhai and G. Zou, Nanoscale Res. Lett., 2021, 16, 123 CrossRef CAS PubMed.
- Y. J. Lai, J. y Tan, Z. y Cai, R. Zhang, C. Teng, S. Zhao, J. Lin and B. Liu, APL Mater., 2021, 9, 051123 CrossRef CAS.
- H. Yu, M. Liao, W. Zhao, G. Liu, X. J. Zhou, Z. Wei, X. Xu, K. Liu, Z. Hu, K. Deng, S. Zhou, J.-A. Shi, L. Gu, C. Shen, T. Zhang, L. Du, L. Xie, J. Zhu, W. Chen, R. Yang, D. Shi and G. Zhang, ACS Nano, 2017, 11, 12001–12007 CrossRef CAS PubMed.
Footnote |
† These authors contributed equally to this work. |
|
This journal is © The Royal Society of Chemistry 2023 |
Click here to see how this site uses Cookies. View our privacy policy here.