DOI:
10.1039/D3RA02894A
(Paper)
RSC Adv., 2023,
13, 20342-20350
Photon avalanche assisted upconversion via customizing the green emission†
Received
2nd May 2023
, Accepted 5th June 2023
First published on 6th July 2023
Abstract
The developed SnWO4 phosphors incorporated with Ho3+, Yb3+ and Mn4+ ions have been explored under 980 nm laser irradiation. The molar concentration of dopants has been optimized to 0.5 Ho3+, 3.0 Yb3+ and 5.0 Mn4+ in SnWO4 phosphors. The upconversion (UC) emission from the codoped SnWO4 phosphors has been substantially amplified up to 13 times and described based on the energy transfer and charge compensation. On incorporating the Mn4+ ions in the Ho3+/Yb3+ codoped system the sharp green luminescence shifted to reddish broadband emission due to the photon avalanche mechanism. The processes accountable for the concentration quenching have been described based on critical distance. The interaction responsible for the concentration quenching in Yb3+ sensitized Ho3+ and Ho3+/Mn4+:SnWO4 phosphors is considered to be dipole-quadrupole and exchange interaction type, respectively. The activation energy 0.19 eV has been determined, and the phenomenon of thermal quenching is discussed using a configuration coordinate diagram.
1. Introduction
Manganese-based upconverting phosphors have recently been popular because of their extensive use in various promising applications, including solid-state lighting, displays, upconverters, temperature monitoring, plant growth and biological applications.1–3 Manganese (Mn) can be utilized as an activator in downshifting (DS) and upconversion (UC) phenomena.4–6 According to Blasse, the emission from Mn4+ lies in the deep red region, resulting from the 2E → 4A2 transition, which indicates that the Mn4+ incorporated phosphor can be a good candidate for red emission.7 Mn is accepted as a single luminescence center and can be paired with other atoms such as Bi, Cr, and rare earths (RE) such as Ce, Pr, Dy, Sm, Yb, Ho, Nd, Er and Tm.8–18 Researchers are interested in red-emitting Mn2+/Mn4+ ions in aggregation with Eu3+ ions.19,20 Codoping of manganese with lanthanide initiates a mutual energy transfer process, which enhances the photoluminescence properties. The production of dimers can sometimes improve the emission intensity.18 The optical characteristics of Mn can be easily influenced by the oxidation state (i.e., 2+, 4+) and the interaction of the crystal field. Besides the transition metals, fixed emissions can be obtained by doping the RE ions. On doping the lanthanides, the crystal field symmetry of the dopants breaks and due to the mixing of the 4fn and 4fn–15d electronic configurations having opposite parity, the electric dipole transitions become allowed.21–25
Among the various metal tungstates (MWO4), SnWO4 exits in two phases: α-SnWO4 is favoured at low synthesis temperature below 670 °C, whereas β-SnWO4 is commonly obtained beyond this temperature.26 The SnWO4 has a variety of applications, such as electrochemical performance, humidity sensing, photodynamic tumour therapy, photocatalytic degradation, water splitting, resistive switching memory, etc.27–30 The SnWO4 is a diamagnetic semiconductor with a large bandgap and low phonon energy that can be employed for upconverting applications.26,27,31
In the present work, Ho3+/Yb3+/Mn4+:SnWO4 phosphors have been prepared and investigated through several characterization techniques such as X-ray diffraction (XRD), field emission scanning electron microscopy (FESEM), Fourier transform infrared spectroscopy (FTIR), Raman spectroscopy, X-ray photon spectroscopy (XPS), UV-vis-NIR spectroscopy and frequency upconversion study. In the coactivated system, the green emission of Ho3+ ions has been tuned with manganese ions. The detailed study of concentration quenching, the thermal stability and thermal quenching have been performed based on the UC measurements.
2. Experimentation
2.1 Synthesis of phosphors
A variety of SnWO4 phosphors at varying concentration of Ho3+, Yb3+ and Mn4+ ions have been produced using the conventional solid-state synthesis technique. All stoichiometric raw materials SnO, WO4, Ho2O3, Yb2O3 and MnO2 were taken in high purity according to the following chemical expressions:
where, x = 0.1, 0.3, 0.5 and 0.7 mol%
(100 − x − y)SnWO4 + xHo2O3 + yYb2O3 |
where, x = 0.5; y = 1.0, 1.5, 3.0 and 4.0 mol%
(100 − x − y − z)SnWO4 + xHo2O3 + yYb2O3 + zMnO2 |
where, x = 0.5; y = 3.0; z = 1.0, 5.0, 10.0, 15.0 and 20.0 mol%
The accurately weighing precursors were mixed in the mortar pestle. After grinding for 90 minutes using volatile liquid acetone, the mixture was maintained at 1100 °C in a muffle furnace for three hours. The phosphors were retrieved and used for additional characterizations after naturally cooling to ambient temperature.
2.2 Phosphors characterization
To determine the crystal formation and lattice parameters, the optimized Ho3+/Yb3+/Mn4+:SnWO4 phosphors were analyzed by X-ray diffractometer in 10° ≤ 2θ ≤ 70° range. FESEM consisting of an airlock compartment has been used for surface morphological analysis and chemical components analyzed with EDAX. The vibrational bands have been detected with the FTIR and Raman spectroscopy. XPS confirms the valence states and binding energies of the elements in phosphors. Diffuse reflectance spectra (DRS) have been monitored in the UV-vis-NIR region. The developed phosphors have been irradiated with a continuous wave laser source to achieve frequency UC. The monochromator used for this purpose consists of a triple grating and a photomultiplier tube. The luminescence lifetimes measurements have been carried out by using HORIBA ‘Lifetime Spectrometer’ and micro second xenon pulse lamp excitation. Thermal stability experiments have been carried out using a multimeter, thermocouple, and a small heater. Calculations of CIE coordinates have been performed using the GoCIE software.
3. Results and discussion
3.1 Structural characterization
3.1.1. XRD analysis. Fig. 1(a) displays the crystalline nature of the codoped SnWO4 phosphors. The diffraction patterns of phosphors are consistent with the JCPDS file 01-070-1497, of the β-SnWO4 system.26 β-SnWO4 has a cubic structure with space group number 198 and space group P
23. The unit cell consists of SnO6 octahedra and [WO4]2− tetrahedra with lattice constant a = b = c = 7.2989 Å, α = β = γ = 90°, volume = 388.84 Å3. According to the Debye–Scherrer equation, the crystallize size is given by,32 |
 | (1) |
where ‘λ’ implies the wavelength of X-ray, ‘β’ designates the full width at half maxima and ‘θ’ denotes Bragg's diffraction angle. The estimated crystalize sizes for SnWO4, Ho3+/Yb3+:SnWO4 and Ho3+/Yb3+/Mn4+:SnWO4 phosphors are determined to be 66.95 nm, 62.54 nm and 60.39 nm respectively. Further, the positive slope value obtained from the Williamson–Hall equation demonstrates the tensile strain created in the phosphors due to dopants ions.33 The decrease in strain followed by the decrease in slope values (0.017, 0.016, 0.009) with codoping may be the reason for the decrease in crystallite size.32,34
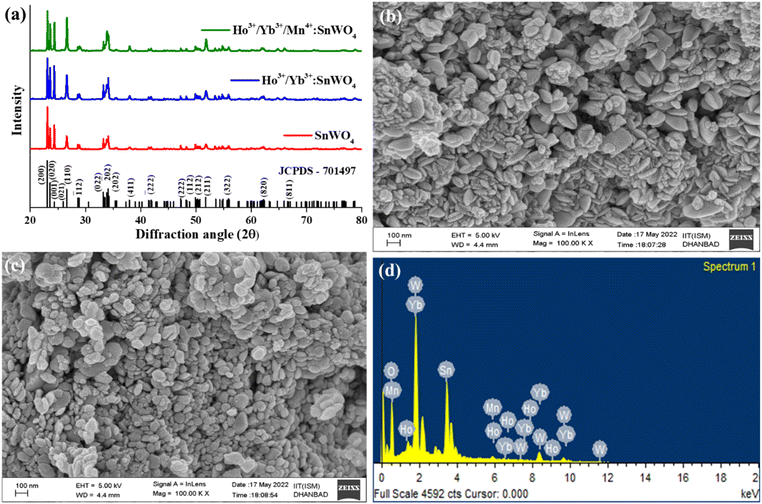 |
| Fig. 1 (a) XRD spectra of SnWO4 host, optimized Ho3+/Yb3+ and Ho3+/Yb3+/Mn4+:MgWO4 phosphors. The FESEM images of (b) Ho3+/Yb3+ (c) Ho3+/Yb3+/Mn4+:SnWO4 phosphors. (d) EDAX of Ho3+/Yb3+/Mn4+:SnWO4 phosphors. | |
The absence of any extra peak in the developed phosphors, indicates that there is no impurity phase. The intense diffraction peaks present in the phosphors correspond to (200), (020), (001), (110), (
12), (022), (
02), (202), (411), (
22), (222), (112), (212), (211), (322), (820) and (811) hkl planes.35 Again, using relation between the radius of the host cation ‘Rm(CN)’ and doped ion ‘Rd(CN)’, the acceptable percent of doping (Dr) can be confirmed as,32,34
|
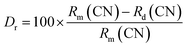 | (2) |
where the values of ionic radii for Sn
2+, W
6+, Ho
3+, Yb
3+ and Mn
4+ are 0.69 Å, 0.60 Å, 0.90 Å, 0.86 Å, 0.53 Å in VI coordination, respectively.
36 The
Dr value for Sn
2+ and Ho
3+/Yb
3+ is 30% and 24%, respectively. Also, for W
6+ and Mn
4+ ions, the
Dr value is 11%. The calculated
Dr values in the present case is less than 30%. This implies that the dopants successfully occupy the cationic sites of the host. The substitution of Sn
2+ by the RE ions creates Sn vacancy in the prepared Ho
3+:SnWO
4 and Ho
3+/Yb
3+:SnWO
4 phosphors, due to which the SnWO
4 system shows charge imbalance that can be demonstrated by using the following Kroger Vink notation given,
6,34,37
The vacancy of oxygen
in the SnWO4 sites is produced when Mn4+ is integrated into SnWO4 phosphors and W6+ is replaced by Mn4+. Thus, it can be applied to the method described below to compensate the Sn vacancy VSn:
3.1.2. FE-SEM and EDAX. The FESEM images {Fig. 1(b) and (c)} show the morphological properties of prepared phosphors. The image taken on 100 nm scale shows that the particles are agglomerated on the surface. Additionally, particle size appears to be decreasing from Ho3+/Yb3+ to Ho3+/Yb3+/Mn4+:SnWO4 phosphors. The presence of all elements viz., tin, tungsten, oxygen, holmium, ytterbium and manganese are confirmed by an elemental analysis using EDAX.
3.1.3. FTIR and Raman analysis. The vibrational features of the SnWO4 host have been accomplished using the FTIR spectrum over the 400–4000 cm−1 range {Fig. 2(a)}. The phonon energy corresponds to the maxima at 604 cm−1 and 668 cm−1. The asymmetric stretching bonds of the WO6 group are responsible for these vibrations. The peaks at 768 cm−1 and 850 cm−1 resemble the stretching vibration of W–W and W–O–W bonds. The stretching and bending mode of the hydroxyl (–OH) group appears at 1634 cm−1 and 3435 cm−1.35,38
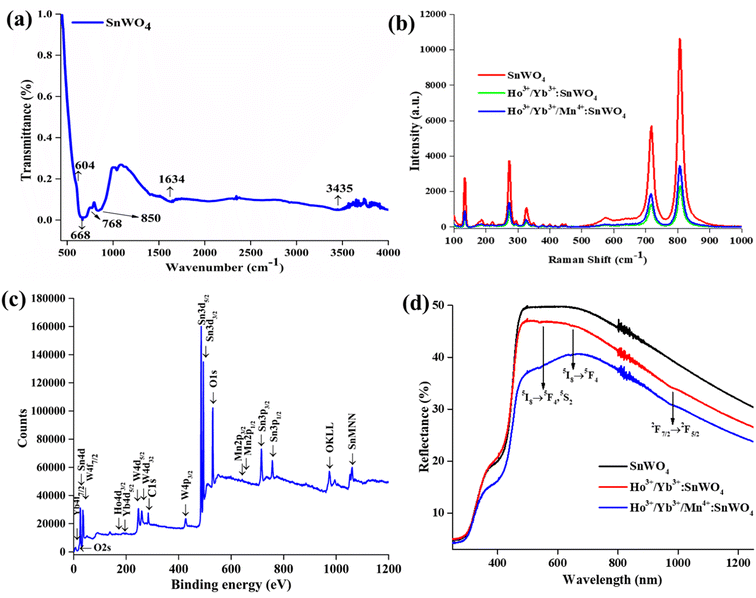 |
| Fig. 2 (a) FTIR spectrum of SnWO4 host, (b) Raman spectra of SnWO4, Ho3+/Yb3+ and Ho3+/Yb3+/Mn4+:SnWO4 phosphors, (c) XPS of Ho3+/Yb3+/Mn4+:SnWO4 phosphors (d) UV-vis spectra of SnWO4, Ho3+/Yb3+ and Ho3+/Yb3+/Mn4+:SnWO4 phosphors. | |
Further, the developed phosphors have been characterized with Raman spectra in 100–1000 cm−1 range {Fig. 2(b)}. The detected Raman peaks indicate that the cubic β-phase SnWO4 phosphors have been successfully formed. According to the group theory, β-SnWO4 consists of 36 vibration peaks, 18 of them are Raman, and rest of them are infrared active. In Mulliken notation, it is given by 6A, 6E, 6E, 18T.27 Several Raman shifts are detected at 135 cm−1, 275 cm−1, 327 cm−1, 718 cm−1 and 807 cm−1.28 The stretching vibration of W–O bonds in the WO6 octahedra appears at 718 cm−1 and 807 cm−1.35 The peak intensity decreases on doping Ho3+/Yb3+/Mn4+, which shows the successful incorporation of dopants. When the dopants are added, the bond distance between two atoms decreases, and there is a reduction in the phonon excitation probability.39
3.1.4. XPS analysis. The chemical analysis and binding energy of the elements present in the codoped phosphors have been confirmed with XPS spectroscopy. The spectrum (binding energy vs. count per second) has been recorded from 0–1200 eV {Fig. 2(c)}. The XPS spectrum shows that all dopants and host elements (Ho, Yb, Mn, Sn, W and O) are present in the phosphors. The peak observed at ∼284 eV due to C1s is used for binding energy calibration. The survey scan shows the characteristic peaks of Sn (4d) at 25 eV. The spin–orbit doublets are detected at 484 eV (3d5/2) and 495 eV (3d3/2).35 Additionally, the 3p3/2 and 3p1/2 states are responsible for the peaks at 715 eV and 756 eV. The XPS peaks for tungsten are found at 35 eV (4f7/2), 247 eV (4d5/2), 259 eV (4d3/2), 426 eV (4p3/2) and 495 eV (4p1/2).6,40 The XPS peaks of oxygen are observed at 21 eV (O2s), 530 eV (O 1s) and 974 eV (OKLL).6 As the concentration of dopants is very low in the developed SnWO4 phosphors, the intensity of Ho3+/Yb3+/Mn4+ is comparatively small. The 4d3/2 and 4d5/2 orbitals are responsible for the binding energy maxima, which are positioned at 159 eV and 184 eV respectively and at 7 eV due to the Yb (4f5/2).5 The +4-oxidation state of manganese has been identified with peaks at 642 eV and 654 eV. It corresponds to the 2p3/2 and 2p1/2 orbitals due to spin–orbit splitting.41
3.2 Optical characterization
3.2.1. Diffuse reflectance spectra and bandgap analysis. The SnWO4, codoped Ho3+/Yb3+ and Ho3+/Yb3+/Mn4+:SnWO4 phosphors have been characterized with UV-vis spectroscopy to find the absorption bands in the 250–1250 nm wavelength range {Fig. 2(d)}. The spectra were calibrated with the standard BaSO4 powder. There is no absorption peak for the SnWO4 host. The doped/codoped SnWO4 phosphors consist of absorption peaks at 538 nm and 640 nm that correspond to the 5I8 → 5F4, 5S2 and 5I8 → 5F5 transitions. The 970 nm peak shows the 2F7/2 → 2F5/2 absorption transition from Yb3+. Applying the following relationship between the Kubelka Monk function and Tauc, the bandgap has been calculated from the DRS spectra,25 |
[F(R∞)hν] = C(hν − Eg)n
| (3) |
where, F(R∞) stands for Kubelka–Munk function which satisfies the following relation, |
F(R∞) = (1 − R)2/2R = K/S
| (4) |
where ‘R’ stands for diffuse reflectance of the spectrum, ‘K’ stands for absorption coefficient and ‘S’ stands for scattering coefficient. F(R∞) depicts the reflection of the infinitely thick sample against a reference at each wavelength, ‘C’ is a constant, ‘hν’ stands for photon energy, ‘Eg’ for the bandgap and n = ½ for direct bandgap. The obtained values of bandgap are 3.60 eV, 3.54 eV and 3.66 eV, corresponding to SnWO4, Ho3+/Yb3+:SnWO4 and Ho3+/Yb3+/Mn4+:SnWO4 phosphors, respectively. The results show that with the doping of larger atoms in the Sn2+ sites, the bandgap decreases, and with the smaller manganese doping, the bandgap increases.26,34
3.2.2. Photoluminescence study and effect of Mn4+ codoping. Fig. 3(a) displays the UC emission of produced phosphors in the spectral range of 350–900 nm after excitation at 980 nm radiation. The emission from doped/codoped SnWO4 phosphors has been enhanced by altering the concentration of Ho3+ (0.1, 0.3, 0.5 and 0.7 mol%)/Yb3+ (1.0, 1.5, 3.0 and 7.0 mol%)/Mn4+ (1.0, 5.0, 10.0, 15.0 and 20.0 mol%). The luminescence peaks found at ∼541 nm and ∼645 nm, arising from Ho3+ ions, are responsible due to the 5F4, 5S2 → 5I8 and 5F5 → 5I8 transitions, respectively. Codoping of Yb3+ ions enhanced the obtained UC emission intensity. Due to the Yb3+ ion's sensitizing effect, the green emission is intensified by 9 times that of Ho3+:SnWO4 phosphors.
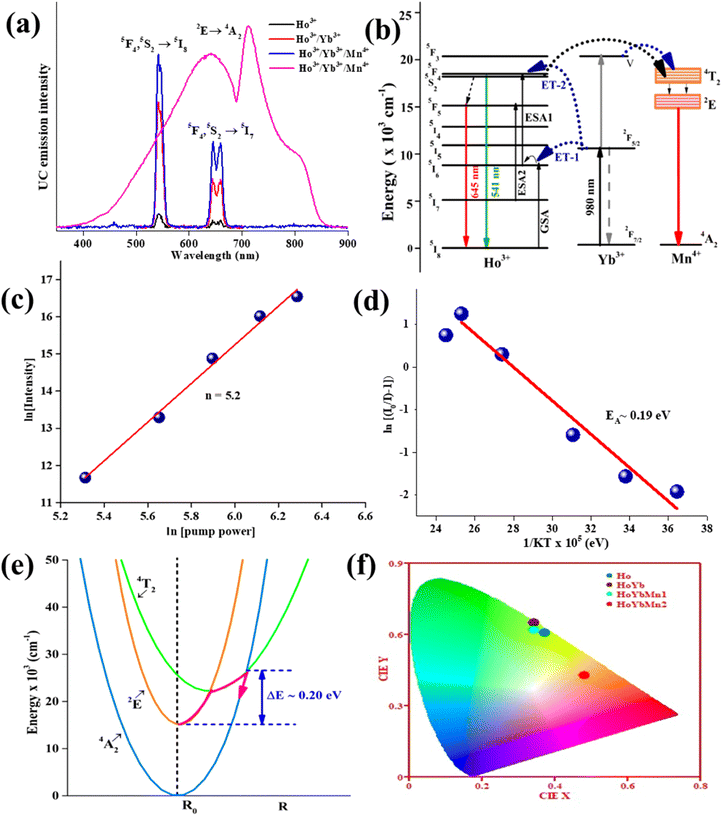 |
| Fig. 3 (a) UC emission spectra of Ho3+, Ho3+/Yb3+, Ho3+/Yb3+/Mn4+:SnWO4 phosphors upon 980 nm excitation, (b) schematic energy level diagram of Ho3+/Yb3+/Mn4+ (c) slope value ‘n’ for broadband in Ho3+/Yb3+/Mn4+:SnWO4 phosphors., (d) activation energy of Ho3+/Yb3+/Mn4+:SnWO4 phosphors, (e) configuration coordinate diagrams showing thermal quenching in Ho3+/Yb3+/Mn4+:SnWO4 phosphors (f) CIE coordinates for Ho3+, Ho3+/Yb3+, Ho3+/Yb3+/Mn4+:SnWO4 phosphors. | |
The UC emission intensity (I) and pump power (p) is correlated by the expression I α pn, where n is the number of pump photons needed to populate the emitting levels. The calculated ‘n’ value for the 5F4, 5S2 → 5I8 transition is 1.3. The decreased slope value in the Ho3+/Yb3+ doped SnWO4 system is due to upconversion saturation at high power density, which is a consequence of the involvement of processes like energy transfer and non-radiative relaxation.42–44 The energy level diagram has been used to explain excitation and emission mechanisms of the Ho3+, Yb3+ and Mn4+ dopants in SnWO4 {Fig. 3(b)}. The luminescence from Ho3+/Yb3+ is the result of ESA, GSA and ET processes.5,45,46 The electrons are stimulated to the 5I6 level by taking the 980 nm excitation energy through GSA, and the 5F4, 5S2 levels of Ho3+ ions are then populated by absorbing the second photon (ESA1). Again, the 5F4 and 5S2 levels are populated by the energy transfer from Yb3+ ions through ET-1 and ET-2 processes. The 5F4, 5S2 → 5I8 transition results in the green emission at 541 nm. A part of the population at 5F4, 5S2 levels relaxes non-radiatively (involving 5 phonons) to populate the next lower 5F5 level. Also, the ESA2 from the 5I7 level populates the 5F5 level from where the 5F5 → 5I8 transition corresponding to the red emission at 645 nm occurs.
Further, on introducing the Mn4+ ions in the Ho3+/Yb3+:SnWO4 phosphors, some fascinating results have been obtained. Here, Mn4+ acts as an intensity enhancer at low pump photon density and a spectra modifier on tuning the pump power density. In the phosphors, after codoping Mn4+, the UC emission intensity of the green band is enhanced by 1.4 times and 13 times compared to the Ho3+/Yb3+ and Ho3+, respectively. On increasing the pump power (<23.6 W cm−2) the UC emission intensity enhances, and at higher pump power (≥23.6 W cm−2) there is a change in the sharp UC peak to broadband ranging from 400–900 nm due to the 2E→ 4A2 transition. At 45.1 W cm−2 visible broadband occurs, and the intensity goes on increasing on a further increase of pump power density. The broadband emitting Ho3+/Yb3+/Mn4+:SnWO4 phosphors is found with slope value 5.2 {Fig. 3(c)}, demonstrating the role of the photon avalanche (PA), which is supported by the cross-relaxation process.47–51 The single Mn4+:SnWO4 is not excited with 980 nm excitation because there is no equivalent energy level. Hence, the energy transfer from either Ho3+ or Yb3+ or both is the only possible way to populate the energy levels of Mn4+. The energy transfer from Ho3+/Yb3+ ions to ground state 4A2 of Mn4+ becomes prominently active when the pump photon number increases sufficiently. The two photons trigger other two-photons and further to four and sixteen photons and so on, simultaneously for a photon avalanche process, which populates the excited 4T2 level of Mn4+. Mn4+ ions are populated in the 2E level through the non-radiative relaxation from the 4T2 level. Owing to the ligand field dependency of Mn4+ ions, the broadband spectra corresponding to the 2E → 4A2 transition has two overlapped peaks at 640 and 710 nm, which is located in the red and deep red region. The peak at 710 nm arises in the broad spectra because of the simultaneous generation and annihilation of phonons caused by the electronic states of 3d electrons and lattice vibrations.52–56
To further reveal the spectroscopic properties, the luminescence lifetimes measurements of Ho3+/Yb3+ and Ho3+/Yb3+/Mn4+:SnWO4 phosphors have been performed upon 361 nm excitation. The ESI Fig. S(a) and S(b)† show the rise and decay time of green (530 nm) and red (645 nm) emissions corresponding to the 5F4, 5S2 → 5I8 and 5F5 → 5I8 transitions, respectively, in Ho3+/Yb3+ and Ho3+/Yb3+/Mn4+:SnWO4 phosphors. The decay curves are fitted to the exponential rise and decay function given by [I = I0[exp(−) − exp(−t/τd)]] where ‘I’ and ‘I0’ are emission intensity at time ‘t’ and ‘0’, ‘τr’, ‘τd’ are the rise and decay times.57–59 The rise time is found to be 0.92 μs and 1.41 μs for green emission and 0.73 μs and 1.12 μs for red emission for Ho3+/Yb3+ and Ho3+/Yb3+/Mn4+:SnWO4 phosphors. The decay time for Ho3+/Yb3+ and Ho3+/Yb3+/Mn4+:SnWO4 phosphors are found to be 5.83 μs and 5.79 μs for green emission and 5.75 μs and 5.81 μs for red emission, respectively. There is a slight change in decay time has been observed. The decay time is the lifetime of the excited levels of the rare earth ions involved in the emission process. However, there is an increase in rise time for both 530 nm and 645 nm emissions has been observed, which shows an increment in the efficient energy transfer process among the dopant ions.
3.2.3. Concentration quenching and energy transfer interaction. On increasing the percentage of activator ions beyond 0.5 mole percent of Ho3+ and 5.0 mole percent of Mn4+ the decrease in luminescence intensity is recorded. Because the distance among dopant ions becomes smaller, and the sensitizer ions are insufficient to transfer the energy to such a smaller distance. In the Ho3+/Yb3+:SnWO4 phosphors, the mean distance among Ho3+ ions have a significant influence on the energy transfer between them. As a result, the critical distance has been calculated using the Blesse equation,34 |
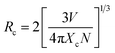 | (5) |
where ‘V’ stands for unit cell volume, ‘Xc’ for critical concentration, and ‘N’ for the quantity of activator ions occupying each lattice site. In present case, V = 388.84 Å3, Xc is 0.5 mol%, N = 4. After calculation, the Rc value is found to be 7.18 Å. If Rc is more than 5, it means that rare earth interactions are not the result of exchange interactions.34,46 Hence, the multipolar interaction precedes the mechanism, which may be established by the relationship given by Dexter and Van Uitert,60 |
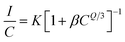 | (6) |
where ‘I’ stands for the emission intensity corresponding to each concentration and ‘C’ stands for the dopant ion concentration. ‘K’ and ‘β’ are constants, while ‘Q’ denotes the nature of the interaction between the quenching center and luminescence center. The exchange interaction, electric dipole–dipole, electric dipole-quadrupole, and electric quadrupole–quadrupole interactions are represented by the distinct values of ‘Q’ = 3, 6, 8, and 10. Since the value of Q/3 has been determined to be 2.60, Q is roughly equivalent to 8. This implied that the concentration quenching of Ho3+ ions in the SnWO4 phosphors is caused by electric dipole-quadrupole interaction.53,61 However, by considering the concentration of Mn4+ ions, the energy transfer process from Ho3+ to Mn4+ can be identified. The found value of Rc is 3.2 Å, which is less than 5 Å. This shows that Ho3+ transfers energy to Mn4+ via short range exchange interaction in the Ho3+/Yb3+/Mn4+:SnWO4 phosphors.62 The efficient energy migration is decided by the critical distance. It becomes important as the dopant's concentration increases. Beyond the critical concentration, further increase in the dopant's concentration brings the dopants too close (i.e., below critical distance) and the attractive potential rises. The obtained value of critical distance confirms that at high concentration (5.0 mol% Mn4+), i.e., when there is a small distance between Mn4+ ions, the excitation energy effectively moves among Mn4+ ions till it hits a killing site and then decays non-radiatively. Thus, the concentration quenching decreases the UC emission intensity.
3.2.4. Thermal stability, configuration coordinates and CIE diagram. The temperature dependent UC spectra of Ho3+/Yb3+/Mn4+:SnWO4 phosphors have been recorded over 303–473 K temperature range to determine the thermal stability. Temperature is a measurable parameter that controls the dynamics of the UC process. Thus, it becomes necessary to check any optical behavior change, such as spectral position, lifetime, CIE, CCT, etc., before the device fabrication. As temperature increases, the intensity of UC emission decreases to 46% at 423 K compared to the initial intensity.5,6,62–65 Thermal quenching at higher temperatures is because of the non-radiative multiphonon relaxation caused by lattice vibrations. The relevant relation between phonon density (n), energy gap (ℏw), Boltzmann constant (K) and the absolute temperature (T) can be given by equation
.5,53 The activation energy can be determined by using the following Arrhenius equation,58,66 |
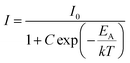 | (7) |
The calculated value of activation energy EA for the broad emitting Ho3+/Yb3+/Mn4+:SnWO4 phosphors is 0.19 eV {Fig. 3(d)}. Greater the activation energy of phosphors, more is the thermal stability. The activation energy and thermal quenching due to increased temperature can be illustrated by using the configuration coordinate {Fig. 3(e)}. The figure shows the 4A2, 2E and 4T2 energy level parabola in terms of potential curve equilibrium position or internuclear distance (R). According to the configuration coordinate, the parabolas of 4A2 and 2E energy states do not cross each other. Thus, the quenching of UC intensity is not possible through 4A2 and 2E crossover. With an increase in temperature, thermal quenching is caused by the wide splitting of the 4T2 and 4T1 spin-quartet states of Mn4+. As a result, there is horizontal displacement of the 4T2 parabola. The 2E, 2T1, 2T2 and 4A2 levels are originated from the same t32 electronic orbital and the 4T1 and 4T2 levels are formed from another t22e orbital. Therefore, the ground state 4A2 and excited state 4T2 (or 4T1) parabolas exhibit a significant lateral displacement. A greater displacement indicates a more intense electron–phonon interaction.67 The lowest sublevel originating from the 4T2 state is shifted nearer to the emitting 2E state; due to that, the nonradiative transitions from 2E to 4T2 are promoted, which probably returns to the 4A2 ground state.68 Thus, thermal quenching occurs in the phosphors owing to thermally generated crossover from the 4T2 excited state to the 4A2 ground state.
To visualize the color emission, the CIE coordinates of all developed phosphors have been determined {Fig. 3(f)}. The found CIE coordinates are (0.37, 0.61), (0.34, 0.65), (0.34, 0.62) and (0.48, 0.43) for Ho3+, Ho3+/Yb3+, Ho3+/Yb3+/Mn4+ and broad emitting Ho3+/Yb3+/Mn4+ codoped SnWO4 phosphors, respectively. The observation shows that upon Yb3+ ions codoping in Ho3+:SnWO4 phosphors, the CIE coordinates shifted towards a more greenish region, i.e., the incorporation of Yb3+ enhances the purity. However, due to the codoping of Mn4+, the CIE coordinates shift towards reddish region. Also, the CCT values have been calculated for all the phosphors, and the calculated values are 4937 K, 5403 K, 5395 K and 2579 K, respectively. The observation shows that from single Ho3+ doped to Ho3+/Yb3+/Mn4+ codoped phosphors, the CCT value shifted from cool light to warm light region.69 Thus, the present phosphors can be used in green and broad visible upconverters.
4. Conclusion
The green and broad emitting Ho3+/Yb3+/Mn4+:SnWO4 phosphors synthesized effectively by the solid-state reaction approach have been structurally and optically studied. Charge compensation boosted the green emission of Ho3+ in codoped SnWO4 phosphors by 13 times after incorporating Mn4+ ions. The broad UC emission is induced by the photon avalanche energy transfer process. Critical distance has been used to demonstrate the concentration quenching owing to exchange interaction in the Ho3+/Yb3+/Mn4+:SnWO4 phosphors. The temperature-dependent luminescence quenching of the broad emitting Ho3+/Yb3+/Mn4+:SnWO4 phosphors has been described by using a configuration coordinate diagram. From these above experimental investigations, it can be inferred that the generated phosphors may be utilized for broadband visible optical devices.
Conflicts of interest
There are no conflicts to declare.
Acknowledgements
Ms Manisha Prasad is thankful to IIT(ISM), Dhanbad for providing the research fellowship.
References
- H. D. Nguyen and R. S. Liu, Narrow-band red-emitting Mn4+-doped hexafluoride phosphors: synthesis, optoelectronic properties, and applications in white light-emitting diodes, J. Mater. Chem. C, 2016, 4, 10759–10775 RSC
. - M. Prasad and V. K. Rai, Frequency Upconversion in UCNPs Containing Transition Metal Ions, Upconverting Nanoparticles: From Fundamentals to Applications, 2022, pp. 141–169 Search PubMed
. - R. Cao, F. Zhang, C. Cao, X. Yu, A. Liang, S. Guo and H. Xue, Synthesis and luminescence properties of CaAl2O4: Mn4+ phosphor, Opt. Mater., 2014, 38, 53–56 CrossRef CAS
. - X. Xu, J. Ren, N. Huang, H. Zeng, G. Chen, D. Kong and H. Tao, Broadly tunable emission from Mn-doped zinc gallogermanate phosphors through composition modification, Opt. Mater. Express, 2014, 4, 2433–2440 CrossRef CAS
. - M. Prasad and V. K. Rai, Influence of transition metal ions: Broad band upconversion emission in thermally stable phosphors, J. Alloys Compd., 2020, 837, 155289 CrossRef CAS
. - M. Prasad and V. K. Rai, Simultaneous effects of synthesis temperature and dopants on MgWO4 UC phosphors, Methods Appl. Fluoresc., 2022, 10, 034004 CrossRef PubMed
. - L. Dong, L. Zhang, Y. Jia, Y. Xu, S. Yin and H. You, ZnGa2–yAlyO4: Mn2+, Mn4+ Thermochromic Phosphors: Valence State Control and Optical Temperature Sensing, Inorg. Chem., 2020, 59, 15969–15976 CrossRef CAS PubMed
. - L. Dong, L. Zhang, Y. Jia, B. Shao, W. Lü, S. Zhao and H. You, Site occupation and luminescence of novel orange-red Ca3M2Ge3O12: Mn2+, Mn4+ (M= Al, Ga) phosphors, ACS Sustainable Chem. Eng., 2020, 8, 3357–3366 CrossRef CAS
. - H. Zhou, N. Guo, M. Zhu, J. Li, Y. Miao and B. Shao, Photoluminescence and ratiometric optical thermometry in Mn4+/Eu3+ dual-doped phosphor via site-favorable occupation, J. Lumin., 2020, 224, 117311 CrossRef CAS
. - L. Li, Y. Pan, Z. Chen, S. Huang and M. Wu, Tunable luminescence and energy transfer properties of Bi3+ and Mn4+ co-doped Ca14Al10Zn6O35 phosphors for agricultural applications, RSC Adv., 2017, 7, 14868–14875 RSC
. - H. Zhang, Y. Chen, X. Zhu, K. Liu, H. Zhou, X. Sun and Y. Feng, et al. The deep red Ca2YZr2Al3O12: Mn4+ phosphor and enhanced emission by Bi3+ doping, J. Lumin., 2021, 236, 118131 CrossRef CAS
. - Q. Sai, C. Xia, H. Rao, X. Xu, G. Zhou and P. Xu, Mn, Cr-co-doped MgAl2O4 phosphors for white LEDs, J. Lumin., 2011, 131, 2359–2364 CrossRef CAS
. - X. Zhang and M. Gong, Single-phased white-light-emitting NaCaBO3: Ce3+, Tb3+, Mn2+ phosphors for LED applications, Dalton Trans., 2014, 43, 2465–2472 RSC
. - W. Liang and Y. Wang, Energy transfer between Pr3+ and Mn2+ in K2YZr (PO4)3: Pr, Mn phosphor, Mater. Chem. Phys., 2011, 127, 170–173 CrossRef CAS
. - X. Qu, L. Cao, W. Liu, G. Su and P. Wang, Luminescence properties of CdSiO3: Mn2+, RE3+ (RE= Sm, Dy, Eu) phosphors, J. Alloys Compd., 2009, 487, 387–390 CrossRef CAS
. - R. Valiente, O. S. Wenger and H. U. Güdel, Near-infrared-to-visible photon upconversion process induced by exchange interactions in Yb3+-doped RbMnCl3, Phys. Rev. B: Condens. Matter Mater. Phys., 2001, 63, 165102 CrossRef
. - Z. T. Chen, E. H. Song, M. Wu, S. Ding, S. Ye and Q. Y. Zhang, Bidirectional energy transfer induced single-band red upconversion emission of Ho3+ in KZnF3: Mn2+, Yb3+, Ho3+ nanocrystals, J. Alloys Compd., 2016, 667, 134–140 CrossRef CAS
. - Y. Lv, Y. Jin, T. Sun, J. Su, C. Wang, G. Ju and Y. Hu, et al. Visible to NIR down-shifting and NIR to visible upconversion luminescence in Ca14Zn6Ga10O35: Mn4+, Ln3+ (Ln = Nd, Yb, Er), Dyes Pigm., 2019, 161, 137–146 CrossRef CAS
. - J. Liao, Q. Wang, H. R. Wen, H. Yuan, S. J. Liu, J. Fu and B. Qiu, First observation of mutual energy transfer of Mn4+–Er3+ via different excitation in Gd2ZnTiO6: Mn4+/Er3+ phosphors, J. Mater. Chem. C, 2017, 5, 9098–9105 RSC
. - E. H. Song, S. Ding, M. Wu, S. Ye, Z. T. Chen, Y. Y. Ma and Q. Y. Zhang, Tunable white upconversion luminescence from Yb3+-Tm3+-Mn2+ tri-doped perovskite nanocrystals, Opt. Mater. Express, 2014, 4, 1186–1196 CrossRef
. - X. Qin, X. Liu, W. Huang, M. Bettinelli and X. Liu, Lanthanide-activated phosphors based on 4f-5d optical transitions: theoretical and experimental aspects, Chem. Rev., 2017, 117, 4488–4527 CrossRef CAS PubMed
. - K. Ogasawara, S. Watanabe, Y. Sakai, H. Toyoshima, T. Ishii, M. G. Brik and I. Tanaka, Calculations of complete 4fn and 4fn-1 5d1 energy level schemes of free trivalent rare-earth ions, Jpn. J. Appl. Phys., 2004, 43, L611 CrossRef CAS
. - I. Gupta, S. Singh, S. Bhagwan and D. Singh, Rare earth (RE) doped phosphors and their emerging applications: A review, Ceram. Int., 2021, 47, 19282–19303 CrossRef CAS
. - G. Liu, Advances in the theoretical understanding of photon upconversion in rare-earth activated nanophosphors, Chem. Soc. Rev., 2015, 44, 1635–1652 RSC
. - M. Prasad and V. K. Rai, Thermally stable upconverting Na3Zr2(SiO4)2PO4: Er3+/Yb3+ phosphors for displays and optical thermometry, J. Alloys Compd., 2022, 911, 164968 CrossRef CAS
. - W. Jeitschko and A. W. Sleight, Synthesis, properties and crystal structure of β-SnWO4, Acta Crystallogr., Sect. B: Struct. Crystallogr. Cryst. Chem., 1972, 28, 3174–3178 CrossRef CAS
. - J. L. Solis, J. Frantti, V. Lantto, L. Häggström and M. Wikner, Characterization of phase structures in semiconducting SnWO4 powders by Mössbauer and Raman spectroscopies, Phys. Rev. B: Condens. Matter Mater. Phys., 1998, 57, 13491 CrossRef CAS
. - F. A. Alharthi, M. A. Alsaiari, M. S. Jalalah, M. Shashank, A. A. Alghamdi, J. S. Algethami and N. Ganganagappa, Combustion synthesis of β-SnWO4-rGO: Anode material for Li-ion battery and photocatalytic dye degradation, Ceram. Int., 2021, 47, 10291–10300 CrossRef CAS
. - C. Seidl, J. Ungelenk, E. Zittel, T. Bergfeldt, J. P. Sleeman, U. Schepers and C. Feldmann, Tin tungstate nanoparticles: a photosensitizer for photodynamic tumor therapy, ACS Nano, 2016, 10, 3149–3157 CrossRef CAS PubMed
. - P. Han, B. Sun, S. Cheng, F. Yu, B. Jiao and Q. Wu, An optoelectronic resistive switching memory behavior of Ag/α-SnWO4/FTO device, J. Alloys Compd., 2016, 681, 516–521 CrossRef CAS
. - M. Zhang, Z. Cui, R. Song, B. Lv, Z. Tang, X. Meng and W. Bu, et al. SnWO4-based nanohybrids with full energy transfer for largely enhanced photodynamic therapy and radiotherapy, Biomaterials, 2018, 155, 135–144 CrossRef CAS PubMed
. - L. Mukhopadhyay, V. K. Rai, R. Bokolia and K. Sreenivas, 980 nm excited Er3+/Yb3+/Li+/Ba2+: NaZnPO4 upconverting phosphors in optical thermometry, J. Lumin., 2017, 187, 368–377 CrossRef CAS
. - P. Ghosh, J. Oliva, E. D. L. Rosa, K. K. Haldar, D. Solis and A. Patra, Enhancement of upconversion emission of LaPO4: Er@ Yb core− shell nanoparticles/nanorods, J. Phys. Chem. C, 2008, 112, 9650–9658 CrossRef CAS
. - S. Pattnaik and V. K. Rai, Impact of charge compensation on optical and thermometric behaviour of titanate phosphors, Mater. Res. Bull., 2020, 125, 110761 CrossRef CAS
. - S. R. Ede and S. Kundu, Microwave synthesis of SnWO4 nanoassemblies on DNA scaffold: a novel material for high performance supercapacitor and as catalyst for butanol oxidation, ACS Sustainable Chem. Eng., 2015, 3, 2321–2336 CrossRef CAS
. - R. D. Shannon, Revised effective ionic radii and systematic studies of interatomic distances in halides and chalcogenides, Acta Crystallogr., Sect. A: Cryst. Phys., Diffr., Theor. Gen. Crystallogr., 1976, 32, 751–767 CrossRef
. - S. Wang, Y. J. Han, L. Shi, M. X. Jia, Y. L. Tong, N. N. Bao and S. L. Niu, et al. Charge compensation assisted enhanced photoluminescence derived from Al3+-codoped NaLaMgWO6: Mn4+ phosphors for plant growth lighting applications, J. Lumin., 2020, 226, 117438 CrossRef CAS
. - H. N. Sumedha, M. A. Alsaiari, M. S. Jalalah, M. Shashank, F. A. Alharthi, N. Ahmad and N. Ganganagappa, et al. Rapid Microwave Synthesis of β-SnWO4 Nanoparticles: An Efficient Anode Material for Lithium Ion Batteries, Crystals, 2021, 11, 334 CrossRef CAS
. - T. Badapanda, V. Senthil, S. Panigrahi and S. Anwar, Diffuse phase transition behavior of dysprosium doped barium titanate ceramic, J. Electroceram., 2013, 31, 55–60 CrossRef CAS
. - S. Wang, H. Gao, C. Chen, Q. Li, C. Li, Y. Wei and L. Fang, Effect of phase transition on optical and photoluminescence properties of nano-MgWO4 phosphor prepared by a gamma-ray irradiation assisted polyacrylamide gel method, J. Mater. Sci.: Mater. Electron., 2019, 30, 15744–15753 CrossRef CAS
. - F. Grote, R. S. Kühnel, A. Balducci and Y. Lei, Template assisted fabrication of free-standing MnO2 nanotube and nanowire arrays and their application in supercapacitors, Appl. Phys. Lett., 2014, 104, 053904 CrossRef
. - A. Nadort, J. Zhao and E. M. Goldys, Lanthanide upconversion luminescence at the nanoscale: fundamentals and optical properties, Nanoscale, 2016, 8, 13099–13130 RSC
. - M. Pollnau, D. R. Gamelin, S. R. Lüthi, H. U. Güdel and M. P. Hehlen, Power dependence of upconversion luminescence in lanthanide and transition-metal-ion systems, Phys. Rev. B: Condens. Matter Mater. Phys., 2000, 61, 3337 CrossRef CAS
. - M. K. Mahata, T. Koppe, T. Mondal, C. Brüsewitz, K. Kumar, V. K. Rai and U. Vetter, et al. Incorporation of Zn2+ ions into BaTiO3: Er3+/Yb3+ nanophosphor: an effective way to enhance upconversion, defect luminescence and temperature sensing, Phys. Chem. Chem. Phys., 2015, 17, 20741–20753 RSC
. - M. Mondal and V. K. Rai, Ho3+-Yb3+: YMoO4 core@ shell nanoparticles for enhanced visible upconversion and security applications, J. Alloys Compd., 2018, 750, 304–311 CrossRef CAS
. - Z. T. Chen, E. H. Song, M. Wu, S. Ding, S. Ye and Q. Y. Zhang, Bidirectional energy transfer induced single-band red upconversion emission of Ho3+ in KZnF3: Mn2+, Yb3+, Ho3+ nanocrystals, J. Alloys Compd., 2016, 667, 134–140 CrossRef CAS
. - G. Bilir, G. Ozen, M. Bettinelli, F. Piccinelli, M. Cesaria and B. Di Bartolo, Broadband Visible Light Emission From Nominally Undoped and Cr3+ Doped Garnet Nanopowders, IEEE Photonics J., 2014, 6, 1–11 Search PubMed
. - Y. Zhu, W. Xu, C. Li, H. Zhang, B. Dong, L. Xu and H. Song, et al. Broad white light and infrared emission bands in YVO4: Yb3+, Ln3+ (Ln3+= Er3+, Tm3+, or Ho3+), Appl. Phys. Express, 2012, 5, 092701 CrossRef
. - L. Marciniak, W. Strek, D. Hreniak and Y. Guyot, Temperature of broadband anti-Stokes white emission in LiYbP4O12: Er nanocrystals, Appl. Phys. Lett., 2014, 105, 173113 CrossRef
. - X. Chen, W. Xu, Y. Zhu, P. Zhou, S. Cui, L. Tao and H. Song, et al. Nd2O3/Au nanocomposites: upconversion broadband emission and enhancement under near-infrared light excitation, J. Mater. Chem. C, 2014, 2, 5857–5863 RSC
. - W. Xu, X. Min, X. Chen, Y. Zhu, P. Zhou, S. Cui and H. Song, et al. Ag-SiO2-Er2O3 nanocomposites: Highly effective upconversion luminescence at high power excitation and high temperature, Sci. Rep., 2014, 4, 5087 CrossRef PubMed
. - D. Chen, Y. Zhou and J. Zhong, A review on Mn4+ activators in solids for warm white light-emitting diodes, RSC Adv., 2016, 6(89), 86285–86296 RSC
. - Upconverting Nanoparticles: From Fundamentals to Applications, ed. V. K. Rai, John Wiley & Sons, 2022 Search PubMed
. - K. Sankarasubramanian, B. Devakumar, G. Annadurai, L. Sun, Y. J. Zeng and X. Huang, Novel SrLaAlO4: Mn4+ deep-red emitting phosphors with excellent responsiveness to phytochrome PFR for plant cultivation LEDs: synthesis, photoluminescence properties, and thermal stability, RSC Adv., 2018, 8, 30223–30229 RSC
. - T. Hu, H. Lin, Y. Cheng, Q. Huang, J. Xu, Y. Gao and Y. Wang, et al. A highly-distorted octahedron with a C 2v group symmetry inducing an ultra-intense zero phonon line in Mn4+-activated oxyfluoride Na2WO2F4, J. Mater. Chem. C, 2017, 5, 10524–10532 RSC
. - Y. Lv, Y. Jin, T. Sun, J. Su, C. Wang, G. Ju and Y. Hu, et al. Visible to NIR down-shifting and NIR to visible upconversion luminescence in Ca14Zn6Ga10O35: Mn4+, Ln3+ (Ln= Nd, Yb, Er), Dyes Pigm., 2019, 161, 137–146 CrossRef CAS
. - J. Grigorjevaite and A. Katelnikovas, Up-Converting K2Gd(PO4)(WO4): 20% Yb3+, Ho3+ Phosphors for Temperature Sensing, Materials, 2023, 16, 917 CrossRef CAS PubMed
. - M. Prasad and V. K. Rai, Coactivated cyan emitting phosphors in optical thermometry using thermally and non-thermally coupled levels, Mater. Res. Bull., 2022, 160, 112116 CrossRef
. - B. Bondzior, D. Stefańska, T. H. Q. Vũ, N. Miniajluk-Gaweł and P. J. Dereń, Red luminescence with controlled rise time in La2MgTiO6: Eu3+, J. Alloys Compd., 2021, 852, 157074 CrossRef CAS
. - M. Prasad, M. Mondal, L. Mukhopadhyay, S. Pattnaik and V. K. Rai, Photoluminescence investigation in tungstate-based materials, Mater. Today: Proc., 2021, 46, 6388–6391 CAS
. - Z. Jia and M. Xia, Blue-green tunable color of Ce3+/Tb3+ coactivated NaBa3La3Si6O20 phosphor via energy transfer, Sci. Rep., 2016, 6, 1–9 CrossRef PubMed
. - W. H. Du, N. Zhang, N. Z. Zhuo, L. Y. Xie, T. Jiang, Y. H. Zhu and H. B. Wang, et al. Photoluminescence properties and energy transfer of apatite-type Sr3LaNa(PO4)3F: Ce3+, Tb3+ phosphors, Mater. Res. Express, 2019, 6, 126209 CrossRef CAS
. - K. Sankarasubramanian, B. Devakumar, G. Annadurai, L. Sun, Y. J. Zeng and X. Huang, Novel SrLaAlO4: Mn4+ deep-red emitting phosphors with excellent responsiveness to phytochrome P FR for plant cultivation LEDs: synthesis, photoluminescence properties, and thermal stability, RSC Adv., 2018, 8, 30223–30229 RSC
. - L. Mukhopadhyay and V. K. Rai, Thermally stable red emitting xenotime phosphate nanophosphors for displays, Mater. Res. Bull., 2020, 121, 110628 CrossRef CAS
. - H. Chen, H. Lin, Q. Huang, F. Huang, J. Xu, B. Wang and Y. Wang, et al. A novel double-perovskite Gd2ZnTiO6:Mn4+ red phosphor for UV-based w-LEDs: structure and luminescence properties, J. Mater. Chem. C, 2016, 4, 2374–2381 RSC
. - S. Pattnaik and V. K. Rai, Tailoring of upconversion luminescence of Al3+ engineered titanate phosphor for non-invasive thermometry, Methods Appl. Fluoresc., 2022, 10, 034002 CrossRef PubMed
. - B. Henderson and G. F. Imbusch, Optical spectroscopy of inorganic solids, Oxford University Press, 2006, vol. 44 Search PubMed
. - T. Senden, R. J. van Dijk-Moes and A. Meijerink, Quenching of the red Mn4+ luminescence in Mn4+-doped fluoride LED phosphors, Light: Sci. Appl., 2018, 7, 1–13 CrossRef CAS PubMed
. - J. Dutta and V. K. Rai, Zirconia based Ho3+–Yb3+ codoped upconverting nanophosphors for green light emitting devices applications, Methods Appl. Fluoresc., 2018, 6, 025003 CrossRef PubMed
.
|
This journal is © The Royal Society of Chemistry 2023 |
Click here to see how this site uses Cookies. View our privacy policy here.