DOI:
10.1039/D3NR05828J
(Communication)
Nanoscale, 2024,
16, 2310-2317
Light-controlled morphological development of self-organizing bioinspired nanocomposites†
Received
16th November 2023
, Accepted 9th January 2024
First published on 10th January 2024
Abstract
Nature's intricate biominerals inspire fundamental questions on self-organization and guide innovations towards functional materials. While advances in synthetic self-organization have enabled many levels of control, generating complex shapes remains difficult. Specifically, controlling morphologies during formation at the single micro/nanostructure level is the key challenge. Here, we steer the self-organization of barium carbonate nanocrystals and amorphous silica into complex nanocomposite morphologies by photogeneration of carbon dioxide (CO2) under ultraviolet (UV) light. Using modulations in the UV light intensity, we select the growth mode of the self-organization process inwards or outwards to form helical and coral-like morphologies respectively. The spatiotemporal control over CO2 photogeneration allows formation of different morphologies on pre-assigned locations, switching between different growth modes—to form for instance a coral on top of a helix or vice versa, and subtle sculpting and patterning of the nanocomposites during formation. These findings advance the understanding of these versatile self-organization processes and offer new prospects for tailored designs of functional materials using photochemically driven self-organization.
Introduction
Control over mineralization is essential for organisms to organize simple building blocks into complex morphologies with tailored functionalities.1–6 For instance, biominerals comprised of calcium carbonate or calcium phosphate such as observed in mollusk shells,7 skeletons,8,9 and microlenses.10 These biominerals can exhibit diverse morphologies that go beyond crystallographic symmetries, and are optimized in shape to fulfil specific mechanical, structural, or optical functions.11 Inspired by such examples, a wide range of synthetic self-organization strategies have been developed that are focused on morphological control.12–20
From this perspective, the nanocomposite morphologies that form during co-precipitation of barium carbonate (BaCO3) nanocrystals with amorphous silica (SiO2) are promising (Fig. 1).21–27 This bioinspired reaction yields a wide diversity of shapes and symmetries such as vases, helices and coral-like forms that can be further sculpted and patterned by controlling the reaction conditions.28–32 These morphologies show optical properties such as waveguiding and polarization.33 Moreover post-process surface functionalization,34–36 and ion exchange reactions have been developed towards a wide range of chemical compositions to introduce optoelectronic,37,38 catalytic,39 and magnetic40 functionalities with preservation of the initial morphology. Control over the morphology is essential for these functionalities: e.g. the helical shape turns nanocomposites into circular optical polarizers, while the coral-like forms offer large macroscale surfaces for maximum accessibility of the reagents and products that boosts catalytic performance.33,39,40
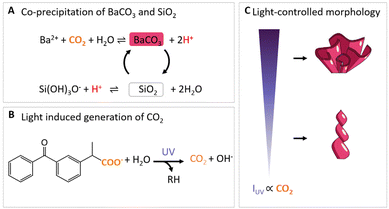 |
| Fig. 1 Light-controlled shaping of barium carbonate silica nanocomposites. (A) CO2 induces the acid-regulated coprecipitation of BaCO3 and SiO2. (B) Light-induced photodecarboxylation of ketoprofen into photoproduct RH and CO2.42 (C) Hypothesis for CO2 regulated morphological development in different growth modes. High IUV results in high CO2 production such that BaCO3/SiO2 composites grow outwards to the bulk solution in coral-like forms, while low values of IUV yield less CO2 such that growth occurs inwards away from the bulk solution to form helices. | |
Recently, more refined control over the precipitation of these mineral composites was achieved by local generation of carbonate using ultraviolet (UV) light-patterns (Fig. 1B).41 Specifically, photodecomposition of the organic molecule ketoprofen releases carbon dioxide (CO2), which in turn can onset the coprecipitation of BaCO3 and SiO2. Until now, light-induced morphological development of BaCO3/SiO2 has been focused on when and where carbonate is generated. What has not been explored, is if the morphological development can be steered by how much carbonate is generated.
So far, morphological development has been controlled between two growth modes: (i) growth away from the bulk solution to form sheets, globular structures and helices, which happens at the lower pH limit (ca. 10.8–11.3) and increased SiO2 precipitation; (ii) growth towards the bulk solution to form stems, vases and coral-like forms, which occurs at higher pH (ca. 11.8–12) and favors precipitation of BaCO3. Moreover, simple modulations in chemical conditions such as CO2 modulations already enable further patterning and sculpting of these shapes which can be further positioned into hierarchical tectonic architectures.23 The BaCO3 precipitation rate is directly determined by the concentration of CO2. Since the CO2-concentration can be controlled photochemically, this suggests that growth modes and patterns may be selected by modulating the light intensity (Fig. 1C).
Motivated by these insights we here steer the coprecipitation of BaCO3/SiO2 nanocomposites by modulating the UV light intensity (IUV) to generate local CO2 concentrations via photodecarboxylation. We demonstrate three levels of control: (1) switching between growth modes to form separate helical and coral-like structures; (2) dynamic transitioning between growth modes within a single structure; and (3) refined sculpting and patterning within one growth mode of a single structure. These results provide further insight in the role of reactions rates on morphological development of these nanocomposites, and open opportunities for tailoring self-organization towards bespoke designs of functional materials.
Results and discussion
Optical setup for light-controlled precipitation
Based on our previous work,41 we design an optical set-up that enables UV irradiation with spatiotemporal control while simultaneously monitoring the resulting mineralization process (Fig. 2). For the UV-irradiation, we select a light emitting diode (LED) with a wavelength of 275 nm or 365 nm, which are close to the absorption maximum of ketoprofen.42 Using a photomask and two lenses, the UV light can be focused in a light spot with a minimum radius up to 10 μm to irradiate a precursor solution in a reaction cell. The reaction cell—comprising two quartz plates that are separated by a Viton spacer—is positioned into a motorized and temperature-controlled sample holder. To monitor the mineralization process, an optical microscope containing a white LED, objective, and CMOS camera is positioned opposite to the UV-irradiation side.
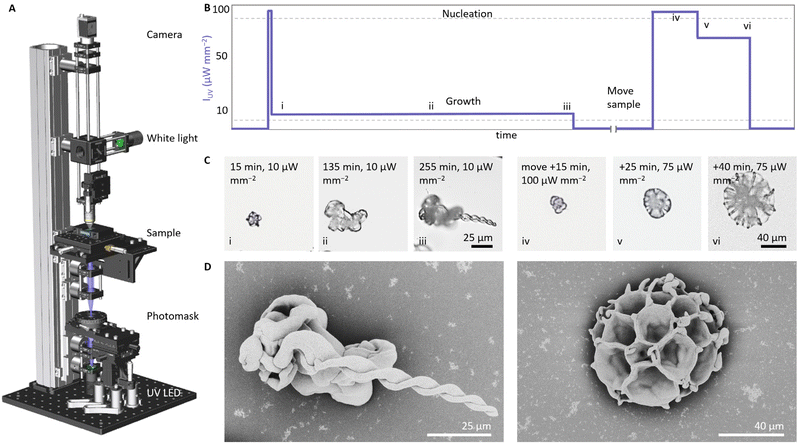 |
| Fig. 2 Light-controlled switching between helical and coral-like growth modes. (A) Optical setup in which UV light is focused on the reaction cell filled with the precursor solution to photochemically release CO2. Using white light, the process is monitored in situ. (B) Applied IUV schedule to yield a helix (left), and in a different location, a coral (right): (i) nucleation is induced at high IUV, (ii) growth at low IUV, and (iii) stopping the precipitation by switching of IUV. After moving the reaction cell to a new position, this illumination schedule is repeated: (iv) nucleation at high IUV (v) growth at slightly lower IUV, (v) stopping precipitation by switching off IUV. The proportions of time are indicative and not to true scale. (C) Timelapse of in situ optical microscope images showing the growth of a helix at low IUV, and growth of a coral at high IUV, the indices correspond the indices in (B). (D) SEM images of the helical (left) and coral-like (right) form that are grown at low and high IUV respectively. | |
Light-controlled switching between growth modes
We investigate if modulations in the UV light intensity IUV can determine the morphologies of the nanocomposites (Fig. 2). We fill the reaction cell with a precursor solution containing barium chloride (20 mM BaCl2), sodium metasilicate (9 mM Na2SiO3) ketoprofen (5 mM), and dodecyl trimethylammonium bromide (50 mM DTAB, a surfactant that is added to dissolve the photoproduct of ketoprofen). Using 0.1 M HCl we lower the pH of the solution to 10.9, which in previous experiments typically resulted in the formation of helices.41 Nucleation is induced by irradiating the precursor solution at high IUV of 100 μW mm−2 for 6 seconds (UV spot has a 250 μm radius). When the first nuclei are observed within the illuminated area, we lower IUV to 10 μW mm−2 (255 min) to enable growth while avoiding further nucleation (Fig. 2B). As expected, we observe the formation of helical architectures, which is consistent with the typical growth in solutions of this relatively low pH (Fig. 2C and D).
We hypothesize that growth at higher IUV results in a higher precipitation of BaCO3, which in turn facilitates the formation of coral-like forms even though the low pH of the bulk solution typically favors the formation of helical shapes. To test this hypothesis, we move the sample to irradiate a new location in the reaction cell and induce new nucleation by increasing the IUV to 100 μW mm−2 for 20 minutes. We continue growth by lowering IUV to 75 μW mm−2 (20 min). This light intensity is 7.5 times higher than the previously used IUV for growing the helical structure, but sufficiently low to avoid undesired nucleation (Fig. 2B). We observe the formation of a coral-like architecture (Fig. 2C and D). Because IUV is much higher, more CO2 is generated and consequently the coral grows faster than the helix. Replicating the experiment consistently yields characteristic helical shapes for low values of IUV (5–10 μW mm−2) and coral-like shapes for high values of IUV (50–75 μW mm−2), independently of previous growth (see ESI Fig. S1 and S2†). Scanning electron microscopy (SEM) images of the corresponding morphologies confirm the development of the helical and coral-like forms. In addition, repeatedly switching between both growth modes is possible and consistently yields coral-like shapes for high IUV and helices for low IUV (see ESI, Fig. S2†). During this switching, we find a reduction of the nucleation rate over time, which we contribute to depletion of the precursor solution, accumulation of photoproduct, and oligomerization of silica. To compensate for this reduction in nucleation rate, we increase the photogeneration of CO2 by increasing the IUV. Hence, modulations of IUV enable switching between growth modes (Fig. 2D).
Switching growth modes within a single structure
The morphology of the growing composite is to a large extend independent of the underlying shape. This suggests that it may be possible to switch between different growth modes within a single structure by modulating IUV (Fig. 3). To explore this refined control over morphological development, we prepare a precursor solution (20 mM BaCl2, 9 mM Na2SiO3, 5 mM ketoprofen, 50 mM DTAB, pH 10.9). We initiate nucleation (100 μW mm−2, 18 s, 150 μm radius) and subsequently grow a coral shape at high IUV (75 μW mm−2) (Fig. 3A). Once the coral shape is formed after 20 min (Fig. 3B, see ESI Movie 1†), we decrease IUV to 10 μW mm−2 to reach the regime where we expect helical growth since less CO2 is generated. We continue growth in the helical growth mode for longer (ca. 130 min). Due to the lower concentration of CO2 most of the active growth sites on the coral shape stop growing as they become passivated with silica.43,44 The parts that continue growing bend towards each other, which is characteristic for precipitation in the helical growth mode. Inspection with SEM confirms that at the initial irradiation at high IUV a coral grows, which continues in helical growth upon decreasing of IUV (Fig. 3C).
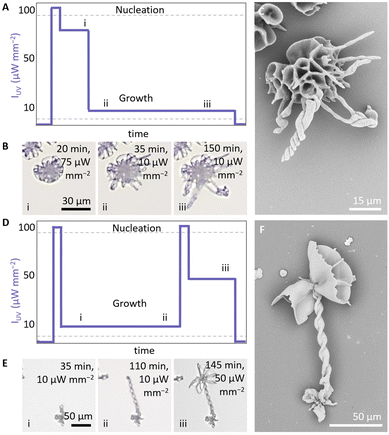 |
| Fig. 3 Light-controlled switching between growth modes within a single structure. (A) Indicative IUV schedule for switching from (i) coral to (ii and iii) helical growth. (B) Timelapse of in situ optical microscope images showing the switching from a coral growth into helical forms. (C) SEM of the resulting structure. (D) Indicative IUV schedule for switching from (i and ii) helical to (iii) coral growth. (E) Timelapse of in situ optical microscope images showing the switching from growth a helix into a coral. Indices correspond with the indices in IUV schedule. (F) SEM of the resulting structure. The figures show a zoom in of the illuminated area, for a complete overview see ESI (Fig. S2– and 3†). | |
To transition from helical growth to corals, we reverse the light intensity schedule (Fig. 3D). We prepare a precursor solution (20 mM BaCl2, 11 mM Na2SiO3, 5 mM ketoprofen, 50 mM DTAB, pH 11.3). After nucleation at high IUV (100 μW mm−2, 25 s, 250 μm radius), we induce helical growth at low IUV (10 μW mm−2, 110 min). Subsequently, we increase the CO2 concentration by increasing IUV to 100 μW mm−2 (30 s), followed by IUV to 50 μW mm−2 (35 min) to move into the coral growth mode. Indeed, time-lapse microscopy (Fig. 3E and ESI Movie 2†) and SEM (Fig. 3F) confirm that at low IUV initially a helix forms, that subsequently transitions into a coral-like form at high IUV. Modulations in IUV thus enable direct switching between growth modes within a single structure.
Light-controlled sculpting and patterning
The sensitivity of the co-precipitation process towards modulations in the CO2 concentration offers the opportunity for light-controlled shaping within a growth mode. We exemplify this capability by sculpting an architecture within the coral growth mode according to a user-defined pattern (Fig. 4). In previous research it was already shown that CO2 pulses in the bulk solution can result in sculpting and patterning.23 We now investigate if local generation of CO2 still enables such control.
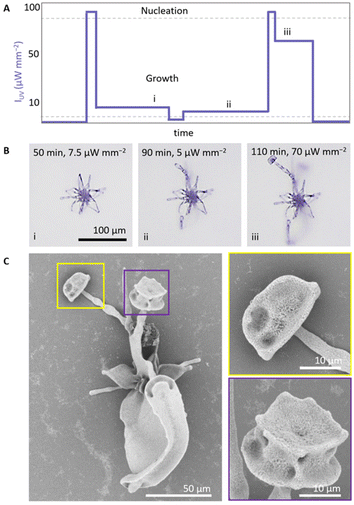 |
| Fig. 4 Dynamic light modulation for refined sculpting within a growth mode. (A) Indicative IUV schedule. (B) Timelapse of in situ optical microscope images of growing architectures, showing (i) initial growth of a coral shape followed by (ii) the narrowing of the growth front to give stems at reduced IUV, that subsequently (iii) open in vase-like shapes upon increasing IUV, indices correspond the with the indices in illumination schedule. (C) SEM images of the grown structure. | |
To demonstrate sculpting, we first induce nucleation (IUV = 100 μW mm−2, 3 s, 250 μm radius) and growth of a coral-like structure in a precursor solution (20 mM BaCl2, 9 mM Na2SiO3, 2.5 mM ketoprofen, 25 mM DTAB, pH 11.5). Once a coral form grows (IUV = 7.5 μW mm−2, 55 min), we adjust the carbonate supply by reducing IUV (2 μW mm−2, 3 min), resulting in the development of thin stems. To prevent complete passivation of growth sites by silica, we slightly increase IUV (5 μW mm−2, 45 min), and achieve a balance between carbonate supply and consumption such that stem growth is sustained. Then, we boost the local CO2 concentration and thereby trigger the splitting of the stems by applying a high intensity pulse (100 μW mm−2, 4 s). During the splitting, the nanocrystals arrange with a wider splay, which results in an increase of the surface, as shown in Fig. 4C. Importantly, even though this high light intensity initially was also used to induce nucleation, now no new nucleation is observed as the already active growth sites deplete the local CO2 concentration. Finally, growth is continued at lower IUV (70 μW mm−2) for 10 minutes to continue growth into vase-like forms, showing that dynamic modulations in light-intensity enable formation of complex forms.
We demonstrate patterning by forming equidistantly spaced bands on a structure. To this aim we perform short rhythmic modulations in IUV using a 365 nm UV light to illuminate a small area of 100 μm radius (Fig. 5). We prepare a precursor solution (20 mM BaCl2, 9 mM Na2SiO3, 5 mM ketoprofen, 50 mM DTAB, pH 11.5). We induce nucleation using a high IUV for 5 seconds (175 μW mm−2) and enable growth for 75 minutes at low IUV (15 μW mm−2). To selectively enable growth on one side, we maintain the UV spot on one side of the growth front by moving the sample stage with a pace that matches the growth rate of the architecture (Fig. 5B). To form equidistantly spaced bands, we take advantage of the fact that the growth rate is approximately linear in time. To form a band, we apply a short 5 seconds pulse in IUV (175 μW mm−2) and subsequently enable 12 minutes of growth with IUV back to the original low IUV (15 μW mm−2) before we apply another two sets of 5 seconds high IUV (175 μW mm−2) followed by 12 minutes low IUV (15 μW mm−2). This rhythmic light schedule of three short pulses equally paced in time results in three well-defined bands that are equally distanced in space (Fig. 5C), hence demonstrating the spatiotemporally control that can be achieved over self-organization.
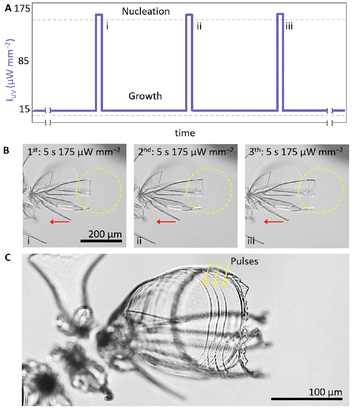 |
| Fig. 5 Dynamic light modulation for rhythmic patterning. (A) Selection of the indicative IUV schedule, in which three short periods of 5 seconds pulses of high IUV (365 nm) with 12 minutes of low IUV is used to pattern a growing structure. (B) Timelapse of the growing structure after each pulse of high IUV. The dashed yellow circle represents the UV spot. The red arrow indicates the direction in which the sample is moved. Indices corresponds with indices in (A). (C) Light microscope image of grown structure. The yellow arrows indicate the moments of pulses in IUV. | |
Mechanistic insights in coprecipitation reaction
Our results provide insights in the formation mechanism of BaCO3/SiO2 nanocomposites. In well-studied configurations of BaCO3/SiO2 coprecipitation,23,44 CO2 diffuses from the air into the reaction solution. In such open systems, switching from helical to coral growth typically requires an increase of the pH from 11 to 12. Increasing the pH from 11 to 12 results in an approximate tenfold increase in CO2 that is taken up from the air into the solution.45 In contrast, in the closed reaction cell that we study here, CO2 is only generated photochemically in the solution. Although we are not able to determine the exact concentration of CO2 that is generated, we find that an approximate tenfold increase in IUV results in switching from helices to corals. Hence both in open and closed systems an approximate tenfold increase in either CO2 uptake or IUV is required to switch from helical to coral growth modes, which emphasizes the importance of the CO2 concentration in the morphological development.
The controlled local photochemical generation of CO2 also gives insights into the role of the solution pH and silica polymerization during the coprecipitation. In open systems CO2 diffusion from the air results in notable acidification of the bulk solution (typically from pH 11.8 to pH 11.5 in 2 hours). This acidification not only reduces the CO2 uptake from the air, but also induces the polymerization of silica species. The resulting silica oligomers can act as slow-diffusing species that buffer the locally acid environment around the reaction front where the co-precipitation occurs.29,30,44 In the closed reaction cell, CO2 is only generated near the precipitating structures, and therefore we observe no significant change in the pH of the bulk solution. Consequently, in the closed system we need to either systematically lower the pH of the bulk (pH 10.5–10.9) or increase the silica content in the solution to access both growth modes. These findings imply that both the silica polymerization and the CO2 concentration determine the morphological development. Overall, these findings emphasize the intricate interplay between the various components in this self-organization processes and highlight how photochemical processes can help to disentangle these processes for gaining mechanistic understanding.
Conclusions
In this study, we show how photogeneration of CO2 can steer the morphological development of BaCO3/SiO2 nanocomposites. With simple modulations in the light intensity, we steer the balance in the precipitation rates to switch between different growth modes, such that helical and coral-like forms can be developed in separate structures next to each other, or integrated into single structures. Moreover, we demonstrate that rational modulations in the photogeneration of CO2 enable local sculpting and patterning of structures within a single growth mode by decorating corals with thin stems that subsequently open as vases and applying rhythmic patterning. This refined level of control provides mechanistic insights and expands the possibilities for steering self-organization processes into user-defined shapes.
We foresee that these results can also impact the development of carbonate–silica nanocomposites as a platform for functional materials. Such artificial self-organization processes are still rare. However, we envisage that light-modulations can be used to steer the self-organization of components in a desired shape, after which complete chemical modification with preservation of the original morphology is possible by ion-exchange. These shape-preserving ion-exchange reactions already given access to a vast and continuously growing library of chemical compositions, which currently provide conversion routes to more than fifty different chemical compositions including perovskites, metal chalcogenides and metals.38–40,46,47,51 Moreover, the catalytic, photochemical, and electronic functionalities of these bioinspired architectures have already been used, for instance as catalysts for the dry reforming of butanol at low temperatures and the Fischer-Tropsch reaction with tunable product distribution.37,38,40,46,47 These results highlight the potential of combining light-controlled precipitation and conversion reactions to independently optimize shape and composition for self-organizing functional components. We also project that other self-organization processes such as Liesegang-inspired patterning or chemical gardens can be controlled using light-driven reactions.48–50 As next step, integration of these self-organization and conversion principles are currently being developed.
Experimental
Preparation precursor solution
Typically, the precursor solution is prepared by dissolving 12.6 mg (6 mM) ketoprofen (KP) (Fluorochem) and 175.0 mg (60 mM) DTAB (VWR) in an 8 mL aqueous solution containing 10.6 mg (11 mM) sodium metasilicate (Na2SiO3) (Sigma Aldrich). Two minutes of sonication is used to enhance the dissolution of KP. In another vial, 49.5 mg barium chloride dihydrate (BaCl2·2H2O) (Sigma Aldrich) is dissolved in 2 mL degassed water (100 mM). The final precursor solution is made by adding the KP–DTAB–Na2SiO3 solution to the BaCl2 solution. The precursor solution (20 mM BaCl2, 9 mM Na2SiO3, 5 mM KP, and 50 mM DTAB) is directly injected in a closed reaction cell. All solutions are kept in nitrogen during preparation to avoid any uptake of carbonate species from the air. For higher concentrations KP, a concentration ratio of 1
:
10 KP
:
DTAB is used to assure that both KP and the photoproduct remain dissolved.
Preparation reaction cell
The reaction cell is composed of a custom-made holder that has two quartz slides and a Viton® spacer (2–3 mm, 1.3–1.9 mL volume). The substrates are plasma cleaned for 10 minutes. Injection of the precursor solution is performed using a syringe with needle by puncturing through the Viton® spacer.
UV-light irradiation
To induce photodecarboxylation, the sample is irradiated using UV-light irradiation of 275 nm and 365 nm and a radius of 100–250 μm. Selection of the light intensity depends on the concentration of precursors, absorption wavelength, illumination area, and initial pH.
UV-irradiation microscope
In the custom-built setup used for the photodecarboxylation process, we identify three main parts: the photolithography part, the sample holder stage, and the imaging part. In the irradiation part, a lens (Edmund Optics 84-337, focal length f = 20.0 mm) collects the light from a 275 nm mounted LED (Thorlabs M275L4, 80 mW output power) or a 365 nm mounted LED (Thorlabs M365L3, 1290 mW output power). The UV light intensity (IUV in μW mm−2) is determined by collecting the UV light on a handheld power meter (Thorlabs PM160T) and dividing the recorded power by the area of the sensor. Custom-made photomasks are placed after the collector lens and a light pattern is projected with the help of two UV anti-reflection coated plano-convex lenses (Thorlabs LA4148-UV, f = 50.0 mm) on the sample. To control the light intensity, neutral density (ND) filters (Thorlabs NDUV-B) are used, as well as adjustments of the LED driving current. The sample holder stage consists of a custom-made temperature cell on top of a motorized translation table. The temperature is controlled by a bath and circulation thermostat (Huber CC-K6). Motion control is provided by piezo inertia actuators (Thorlabs PIAK10) that had a typical step size of 20 nm. In the imaging part, a cold white light mounted LED (Thorlabs MCWHL5) is collected by an aspheric lens (Thorlabs ACL2520U-DG6-A, f = 20.0 mm). A 10
:
90 beamsplitter (Thorlabs BSN10R) directs the light through a 10×/0.30 magnification objective (Nikon Plan Fluor) to the sample. The reflected images are collected with the same objective lens and transmitted through the beamsplitter. With help of a tube lens (Thorlabs AC254-200-A-ML, f = 200.0 mm), images are recorded by a CMOS camera (Basler Ace acA1920-40gc).
Post-process analysis
For post-process analysis, the cell is disassembled in degassed water by carefully separating the substrates from the spacer. The substrates are slowly pulled out of the water, while sprinkled with acetone, and subsequently dried to air. SEM imaging of the substrates is performed using a FEI Verios 460.
Author contributions
The idea, design and concept of the research were developed by M. H. B., A. M. B. and W. L. N. The methodology was developed by M. H. B. and W. L. N. The experimental set-up was developed by M. H. B., M. K., and H. S. The experiments were performed by M. H. B. and N. T. H. The data was analyzed by M. H. B., N. T. H., and W. L. N. The project and research were managed and supervised by W. L. N. The manuscript was written and edited by M. H. B. and W. L. N.
Conflicts of interest
The authors declare no competing interests.
Acknowledgements
The authors thank Dr Hans Hendrikse for drawing the schematics in Fig. 1. This work is part of the Vernieuwingsimpuls Vidi research program “Shaping up materials” with project number 016.Vidi.189.083, which is partly financed by the Dutch Research Council (NWO).
References
-
H. A. Lowenstam and S. Weiner, On Biomineralization, Oxford University Press, New York, USA, 1989 Search PubMed
.
- T. Yang, H. Chen, Z. Jia, Z. Deng, L. Chen, E. M. Peterman, J. C. Weaver and L. Li, Science, 2022, 375, 647–652 CrossRef CAS PubMed
.
- A. Akiva, M. Kerschnitzki, I. Pinkas, W. Wagermaier, K. Yaniv, P. Fratzl, L. Addadi and S. Weiner, J. Am. Chem. Soc., 2016, 138, 14481–14487 CrossRef CAS PubMed
.
- W. Huang, D. Restrepo, J. Jung, F. Y. Su, Z. Liu, R. O. Ritchie, J. McKittrick, P. Zavattieri and D. Kisailus, Adv. Mater., 2019, 31, 1–37 Search PubMed
.
- P. U. P. A. Gilbert, K. D. Bergmann, N. Boekelheide, S. Tambutté, T. Mass, F. Marin, J. F. Adkins, J. Erez, B. Gilbert, V. Knutson, M. Cantine, J. O. Hernández and A. H. Knoll, Sci. Adv., 2022, 8, eabl9653 CrossRef CAS PubMed
.
- E. Beniash, C. A. Stifler, C.-Y. Sun, G. S. Jung, Z. Qin, M. J. Buehler and P. U. P. A. Gilbert, Nat. Commun., 2019, 10, 4383 CrossRef PubMed
.
- M. Connors, T. Yang, A. Hosny, Z. Deng, F. Yazdandoost, H. Massaadi, D. Eernisse, R. Mirzaeifar, M. N. Dean, J. C. Weaver, C. Ortiz and L. Li, Nat. Commun., 2019, 10, 5413 CrossRef CAS PubMed
.
- Y. Cui, H. Li, Y. Li and L. Mao, Nanoscale Adv., 2022, 4, 334–352 RSC
.
- T. Mass, A. J. Giuffre, C. Y. Sun, C. A. Stifler, M. J. Frazier, M. Neder, N. Tamura, C. V. Stan, M. A. Marcus and P. U. P. A. Gilbert, Proc. Natl. Acad. Sci. U. S. A., 2017, 114, E7670–E7678 CrossRef CAS PubMed
.
- J. Aizenberg, A. Tkachenko, S. Weiner, L. Addadi and G. Hendler, Nature, 2001, 412, 819–822 CrossRef CAS PubMed
.
- F. C. Meldrum and H. Cölfen, Nat. Chem., 2023, 15, 1196 CrossRef CAS PubMed
.
- M. Nakayama, S. Kajiyama, A. Kumamoto, Y. Ikuhara and T. Kato, Nanoscale Adv., 2020, 2, 2326–2332 RSC
.
- A. Arakaki, K. Shimizu, M. Oda, T. Sakamoto, T. Nishimura and T. Kato, Org. Biomol. Chem., 2015, 13, 974–989 RSC
.
- E. S. Turali-Emre, A. E. Emre, D. A. Vecchio, U. Kadiyala, J. S. VanEpps and N. A. Kotov, Adv. Mater., 2023, 35, 1–17 Search PubMed
.
- U. G. K. Wegst, H. Bai, E. Saiz, A. P. Tomsia and R. O. Ritchie, Nat. Mater., 2015, 14, 23–36 CrossRef CAS PubMed
.
- G. Isapour, B. H. Miller and M. Kolle, Adv. Photonics Res., 2022, 3, 1–7 Search PubMed
.
- O. Nahi, A. N. Kulak, S. Zhang, X. He, Z. Aslam, M. A. Ilett, I. J. Ford, R. Darkins and F. C. Meldrum, Adv. Sci., 2023, 10, 1–12 Search PubMed
.
- D. Nepal, S. Kang, K. M. Adstedt, K. Kanhaiya, M. R. Bockstaller, L. C. Brinson, M. J. Buehler, P. V. Coveney, K. Dayal, J. A. El-Awady, L. C. Henderson, D. L. Kaplan, S. Keten, N. A. Kotov, G. C. Schatz, S. Vignolini, F. Vollrath, Y. Wang, B. I. Yakobson, V. V. Tsukruk and H. Heinz, Nat. Mater., 2023, 22, 18–35 CrossRef CAS PubMed
.
- M. H. Himel, B. Sikder, T. Ahmed and S. M. Choudhury, Nanoscale Adv., 2022, 5, 596–614 RSC
.
- Z. Xu, L. Shi, D. Hu, B. Hu, M. Yang and L. Zhu, RSC Adv., 2016, 6, 76426–76433 RSC
.
- J. M. García-Ruiz, E. Melero-García and S. T. Hyde, Science, 2009, 323, 362–365 CrossRef PubMed
.
- M. Montalti, G. Zhang, D. Genovese, J. Morales, M. Kellermeier and J. M. Garciá-Ruiz, Nat. Commun., 2017, 8, 1–6 CrossRef PubMed
.
- W. L. Noorduin, A. Grinthal, L. Mahadevan and J. Aizenberg, Science, 2013, 340, 832–837 CrossRef CAS PubMed
.
- C. N. Kaplan, W. L. Noorduin, L. Li, R. Sadza, L. Folkertsma, J. Aizenberg and L. Mahadevan, Science, 2017, 355, 1395–1399 CrossRef CAS PubMed
.
- M. Kellermeier, H. Cölfen and J. M. García-Ruiz, Eur. J. Inorg. Chem., 2012, 5123–5144 CrossRef CAS
.
- P. Knoll and O. Steinbock, Isr. J. Chem., 2018, 58, 682–692 CrossRef CAS
.
- E. Nakouzi and O. Steinbock, Sci. Adv., 2016, 2, e1601144 CrossRef PubMed
.
- P. Knoll, D. S. D'Silva, D. I. Adeoye, M. G. Roper and O. Steinbock, ChemSystemsChem, 2021, 3, e2000061 CrossRef CAS
.
- T. Terada, S. Yamabi and H. Imai, J. Cryst. Growth, 2003, 253, 435–444 CrossRef CAS
.
- E. Bittarello and D. Aquilano, Eur. J. Mineral., 2007, 19, 345–351 CrossRef CAS
.
- J. Opel, M. Kellermeier, A. Sickinger, J. Morales, H. Cölfen and J. M. García-Ruiz, Minerals, 2018, 8, 1–12 CrossRef
.
- M. Kellermeier, F. Glaab, A. M. Carnerup, M. Drechsler, B. Gossler, S. T. Hyde and W. Kunz, J. Cryst. Growth, 2009, 311, 2530–2541 CrossRef CAS
.
- L. Helmbrecht, M. Tan, R. Röhrich, M. H. Bistervels, B. O. Kessels, A. F. Koenderink, B. Kahr and W. L. Noorduin, Adv. Funct. Mater., 2020, 30, 1–5 Search PubMed
.
- J. Opel, J. Brunner, R. Zimmermanns, T. Steegmans, E. Sturm, M. Kellermeier, H. Cölfen and J. M. García-Ruiz, Adv. Funct. Mater., 2019, 29, 1–7 CrossRef
.
- J. Opel, F. P. Wimmer, M. Kellermeier and H. Cölfen, Nanoscale Horiz., 2016, 1, 144–149 RSC
.
- J. Opel, L. C. Rosenbaum, J. Brunner, A. Staiger, R. Zimmermanns, M. Kellermeier, T. Gaich, H. Cölfen and J. M. García-Ruiz, J. Mater. Chem. B, 2020, 8, 4831–4835 RSC
.
- B. C. Batista and O. Steinbock, Chem. Commun., 2022, 58, 12736–12739 RSC
.
- T. Holtus, L. Helmbrecht, H. C. Hendrikse, I. Baglai, S. Meuret, G. W. P. Adhyaksa, E. C. Garnett and W. L. Noorduin, Nat. Chem., 2018, 10, 740–745 CrossRef CAS PubMed
.
- H. C. Hendrikse, A. Aguirre, A. Van Der Weijden, A. S. Meeussen, F. Neira D'Angelo and W. L. Noorduin, Cryst. Growth Des., 2021, 21, 4299–4304 CrossRef CAS PubMed
.
- H. C. Hendrikse, A. van der Weijden, M. Ronda-Lloret, T. Yang, R. Bliem, N. R. Shiju and M. van Hecke, Adv. Mater., 2020, 32, 2003999 CrossRef PubMed
.
- M. H. Bistervels, M. Kamp, H. Schoenmaker, A. M. Brouwer and W. L. Noorduin, Adv. Mater., 2022, 34, 2107843 CrossRef CAS PubMed
.
- G. Cosa, L. J. Martínez and J. C. Scaiano, Phys. Chem. Chem. Phys., 1999, 1, 3533–3537 RSC
.
- J. Opel, M. Hecht, K. Rurack, J. Eiblmeier, W. Kunz, H. Cölfen and M. Kellermeier, Nanoscale, 2015, 7, 17434–17440 RSC
.
- M. Kellermeier, E. Melero-García, F. Glaab, J. Eiblmeier, L. Kienle, R. Rachel, W. Kunz and J. M. García-Ruiz, Chem. – Eur. J., 2012, 18, 2272–2282 CrossRef CAS PubMed
.
- W. J. Cai, Y. Y. Xu, R. A. Feely, R. Wanninkhof, B. Jönsson, S. R. Alin, L. Barbero, J. N. Cross, K. Azetsu-Scott, A. J. Fassbender, B. R. Carter, L. Q. Jiang, P. Pepin, B. Chen, N. Hussain, J. J. Reimer, L. Xue, J. E. Salisbury, J. M. Hernández-Ayón, C. Langdon, Q. Li, A. J. Sutton, C. T. A. Chen and D. K. Gledhill, Nat. Commun., 2020, 11, 1–13 CrossRef PubMed
.
- H. C. Hendrikse, S. Hémon-Charles, L. Helmbrecht, E. P. Van Dam, E. C. Garnett and W. L. Noorduin, Cryst. Growth Des., 2021, 21, 4500–4505 CrossRef CAS PubMed
.
- Q. Wang and O. Steinbock, Phys. Chem. Chem. Phys., 2022, 24, 14538–14544 RSC
.
- B. C. Batista, A. Z. Morris and O. Steinbock, Proc. Natl. Acad. Sci. U. S. A., 2023, 120, e2305172120 CrossRef CAS PubMed
.
- C. T. van Campenhout, H. Schoenmaker, M. van Hecke and W. L. Noorduin, Adv. Mater., 2023, 2305191, 1–8 Search PubMed
.
- L. M. Barge, S. S. S. Cardoso, J. H. E. Cartwright, G. J. T. Cooper, L. Cronin, A. De Wit, I. J. Doloboff, B. Escribano, R. E. Goldstein, F. Haudin, D. E. H. Jones, A. L. Mackay, J. Maselko, J. J. Pagano, J. Pantaleone, M. J. Russell, C. I. Sainz-Díaz, O. Steinbock, D. A. Stone, Y. Tanimoto and N. L. Thomas, Chem. Rev., 2015, 115, 8652–8703 CrossRef CAS PubMed
.
- van der Weijden, Contraction and Expansion of Nanocomposites during Ion Exchange Reactions, Cryst. Growth Des., 2022, 22, 2289–2293, DOI:10.1021/acs.cgd.1c01364
.
|
This journal is © The Royal Society of Chemistry 2024 |
Click here to see how this site uses Cookies. View our privacy policy here.