DOI:
10.1039/D4QI01789G
(Review Article)
Inorg. Chem. Front., 2024,
11, 6218-6245
Recent progress of advanced electrocatalysts for hydrogen production via hydrazine-assisted water electrolysis
Received
16th July 2024
, Accepted 13th August 2024
First published on 14th August 2024
Abstract
Coupling the thermodynamically favorable hydrazine oxidation reaction (HzOR) with the hydrogen evolution reaction (HER) in a hybrid water electrolyzer is an effective strategy to improve the energy efficiency of large-scale high-purity H2 production, while achieving pollutant degradation. Recently, various advanced materials have been exploited as electrocatalysts for hydrazine-assisted water electrolysis, but a fundamental understanding of them and a comprehensive summary are lacking to date. In this review, we provide a comprehensive review of advanced electrocatalysts available for HzOR-assisted water electrolysis, as well as various regulatory strategies based on precious metals and non-noble metal-based materials, such as doping, heterostructures, single-atoms, and alloying. Moreover, the structure–activity relationship including electronic structure, surface properties, and catalytic performance of the electrocatalysts is systematically discussed. Given the importance and unique advantages of direct hydrazine hydrate-assisted seawater electrolysis and self-powered electrolyzers, we also present systematic summaries of material design, performance evaluation, and mechanism studies. Finally, several key challenges and future perspectives about hydrazine-assisted water electrolysis are discussed to offer insight into large-scale H2 production for energy-saving pathways.
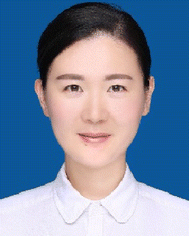 Yun Tong | Yun Tong is an associate professor at the School of Chemistry and Chemical Engineering at Zhejiang Sci-Tech University (ZSTU), China. She received her BS degree from Zhejiang Sci-Tech University in 2012 and obtained her Ph.D. in Inorganic Chemistry from the University of Science and Technology of China (USTC) in 2018. She joined ZSTU as a lecturer in 2018 and was promoted to associate professor in 2023. During this period, she received the China-Swiss Government Exchange Scholarship to conduct postdoctoral research at EPFL (2019–2021). Her research focuses on the development and application of inorganic materials for electrocatalysis. |
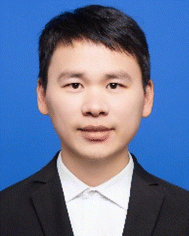 Pengzuo Chen | Pengzuo Chen is a professor at the School of Chemistry and Chemical Engineering in Zhejiang Sci-Tech University (ZSTU), China. He received his Ph.D. in Inorganic Chemistry from the University of Science and Technology of China (USTC) in 2018 and obtained a BS degree from Zhejiang Sci-Tech University in 2012. He worked as a postdoctoral researcher at École Polytechnique Fédérale de Lausanne (EPFL) from 2018 to 2021. He joined ZSTU as a full professor in 2021. His research interests include the controllable synthesis of low-dimensional inorganic solid materials for electrocatalysis. |
1. Introduction
The detrimental environmental pollution issues caused by the overdependence on fossil fuels have led to an urgent requirement for renewable, green, and alternative energy sources to alleviate the huge pressure on humanity. Hydrogen (H2) fuel is an important energy carrier and low-carbon energy system that has gained widespread attention as a renewable alternative energy resource due to its extremely high gravimetric energy density and pollution-free byproduct, water.1–5 Recently, the steam reforming of fossil resources has become the main method of producing most of the H2. However, the low conversion rate and high emission of greenhouse gases from this method result in high economic costs and environmental problems. In this regard, water electrolysis for the generation of hydrogen and oxygen has been considered a valuable alternative strategy to acquire high-purity H2 at a large scale with efficiency and zero-CO2 emission.6–10 The anodic oxygen evolution reaction (OER) and cathodic hydrogen evolution reaction (HER) are the basic half-reactions for water electrolysis.11–13 Particularly, the kinetically sluggish OER with a four-proton-coupled electron transfer process has been demonstrated as the key restraint for the conversion efficiency of water-to-hydrogen because of its high thermodynamic potential of 1.23 V. This high potential significantly hinders the commercialization of electrocatalytic water splitting technology.14–17
Over the past few years, plenty of advanced electrocatalytic oxidation reactions of small molecules, such as alcohol, urea, furfural, glucose, and hydrazine hydrate, have emerged as promising and effective substrates for the OER.18–25 The combination of the HER with favorable kinetics of the half-reactions, such as low theoretical potentials, is important to lower the overpotential at the anode, and thus, realize an energy-saving H2 production.26,27 Among these alternative reactions, the hydrazine oxidation reaction (HzOR) is regarded as an attractive option because of its small theoretical potential (−0.33 V vs. RHE) and high power density.28–30 On the one hand, hydrazine is regarded as an important feedstock for various applications, such as rocket fuel, pharmaceutical products, fuel cells, and agrochemicals.31–33 On the other hand, the excessive emissions of highly toxic hydrazine may cause irreversible effects on human health and ecosystem. Therefore, the construction of a HzOR-assisted HER system is beneficial to gain high-purity H2 in an energy-saving manner, while maintaining environmental sustainability.34,35 As early as 2001, T. I. Valdez and co-authors confirmed that the electrolysis of aqueous organic solutions is an effective way to generate H2.36 However, this phenomenon was only published as a United States patent. The related literature was lacking until Minoru Umeda's group reported the electrolysis of methanol to water solution and saved ∼60% electrical energy compared to direct water electrolysis.37 Subsequently, with the rapid development of nanostructure control strategies, more and more high-performance electrocatalysts have been developed for hydrazine hydrate-assisted electrolysis for hydrogen production.
Among the important material systems are precious metal-based electrocatalysts with high intrinsic activity, such as Pt, Rh, Ru, Pd, Ir, and Ag, and their alloys.38–46 However, the high prices and limited crustal reserves of these materials limit their wide-ranging applications. In addition, the formation of oxides/hydroxides of most precious metals on such materials’ surfaces limits the HzOR activity.47 Therefore, it is of great research value to achieve a high-quality utilization of noble metal catalysts to ensure high catalytic activity. Furthermore, improving the activity and stability of noble metal-based catalysts through nanostructure engineering is also a widely reported and important issue in the literature.48,49 On the other hand, non-precious metals, such as Fe, Co, Ni, Cu, and so on, have been widely used as alternatives to develop highly active electrocatalysts, including metal oxides, phosphides, nitrides, selenides, or their alloys for HzOR-assisted water electrolysis.50–60 Contrary to the precious metal catalysts, surface oxidation of these materials to active species with high valence states (oxyhydroxides/peroxides) plays a critical influence on their catalytic performance.61–63 However, the main challenge faced with non-precious metal materials is achieving noble metal-like activity, especially for the design of bifunctional electrocatalysts with high performance in both HER and HzOR.
Recently, inspired by pioneering works, various nanostructure engineering methods have been applied to rationally design efficient electrocatalysts. Great progress has been made in energy-saving H2 production by hydrazine-assisted water electrolysis.64–67 However, regardless of more and more significant achievements being reported, the comprehensive discussion and summary of research progress has been ignored. In this review, we focus on the nano-regulation strategies of advanced electrocatalysts based on precious metals, non-precious metals, or metal-free materials, to give a basic, but comprehensive, overview of the development of HzOR-assisted water electrolysis. Major nanostructure engineering approaches, such as doping, heterostructure, single-atom, and alloying, have been summarized to study the close relationship between the catalytic performance and the physicochemical properties of electrocatalysts. In addition, the promising applications of advanced electrocatalysts in various fields, including direct HzOR-assisted seawater electrolysis and self-powered electrolysis systems, have also been discussed. Finally, future challenges and opportunities have been proposed to encourage more in-depth study on this highly promising technology.
2. A fundamental understanding of hydrazine-assisted water electrolysis
2.1 The cathodic electrocatalytic hydrogen evolution reaction (HER)
The hydrogen evolution reaction (HER) is one of the most important cathodic half-reactions for traditional electrocatalytic water splitting. The HER process is related to the pH of the electrolyte, which is described by the following equations: 2H+ + 2e− → H2 in an acidic electrolyte and 4H2O + 4e− → 2H2 + 4OH− in an alkaline electrolyte.68 Normally, several possible principal steps have been reported to occur on the electrode surface during the HER process, whether it is for the reduction of H+ or the conversion of H2O in acidic and alkaline media, respectively. However, the adsorbed hydrogen atom (Hads) intermediate is always the key to the study of HER mechanisms. Firstly, the Volmer reaction is regarded as the first discharge step that yields Hads in an acidic electrolyte by receiving one electron on the electrode surface and reducing one proton at the same time.69 However, the concentration of proton is low under neutral/alkaline conditions, leading H2O to act as a Hads source by receiving the transferred electron. Furthermore, H2 gas can be produced by Heyrovsky or Tafel pathways, which is directly relative to the coverage ratio of Hads on the electrode surface. The HER takes place via the Heyrovsky reaction when the coverage ratio of Hads is low, leading to the preferable combination of proton/H2O and single Hads to form H2 with the help of an extra electron.70 On the contrary, the Tafel reaction of HER happens under a high coverage ratio of Hads, resulting in the formation of H2 by the combination of two neighboring Hads atoms. Regardless of the electrolyte used, the fast kinetics of the two-electron transfer process is a unique advantage of HER, but the efficiency of H2 generation via it is often limited by the anodic reaction.
2.2 The anodic electrocatalytic hydrazine oxidation reaction (HzOR)
Hydrazine (N2H4) fuel is not only a promising alternative to H2 due to its high energy density (5.40 kW h L−1, 1 atm), but it is also an attractive chemical as rocket fuel or reductant in organic synthesis. In the 1960s, direct hydrazine fuel cells (DHFCs) derived from hydrazine oxidation reaction (HzOR) emerged and gained attention because they benefited from the utilization of the energy-dense N2H4 fuel.71 HzOR process is a rapid four-electron transferred kinetics with an ultralow theoretical oxidation potential, which endows its high applicability and promising prospects. The fundamental reaction process of HzOR is directly related to the acidity and alkalinity of the electrolyte. Under acidic conditions, N2H4 is first converted predominantly to the hydrazinium (N2H4 + H+ + e− → N2H5+) ion and further oxidized to the nitrogen gas and protons by the following equation (N2H5+ → N2 + 5H+ + 4e−, E° = −0.23 V vs. SHE).72 Moreover, the hydrazine is relatively stable under alkaline conditions and can be further oxidized to harmless nitrogen gas and water according to a different reaction than the acidic pathway (N2H4 + 4OH− → N2 + 4H2O + 4e−, E° = −0.33 V vs. RHE, pH = 14).73 It is worth noting that the HzOR has small theoretical oxidation potentials in a pH universal range. This indicates that the HzOR is a promising half-reaction to couple with HER and can significantly reduce the required potential for water splitting.
2.3 The coupled overall reaction for energy-saving H2 generation
The traditional water electrolysis consists of HER/OER with a high thermodynamic voltage of 1.23 V, leading to low conversion efficiency and high energy consumption for the commercialization of H2 generation. To save energy and reduce the required voltage for water electrolysis, thermodynamically fast HzOR is a good alternative for the OER. It establishes a new electrochemical configuration for hydrazine-assisted H2 production (HER/HzOR), namely overall hydrazine splitting (OHzS), which is of significance for sustainable energy storage and conversion systems (Fig. 1).74 The HER and HzOR act as new cathodic and anodic reactions in this coupling system, and the overall reaction of OHzS can be presented as the following equations: anodic reaction is N2H4 + 4OH− → N2 + 4H2O + 4e−, while the cathodic reaction is 4H2O + 4e− → 2H2 + 4OH−, leading to the following overall reaction: N2H4 → N2 + 2H2, in alkaline system.75 However, although the coupled overall reactions of HER/HzOR have potential advantages, their catalytic performance must be improved further by designing highly active and robust electrocatalysts for large-scale H2 production. Recently, many electrocatalysts have been developed by using various regulation strategies that achieved significant progress for energy-saving H2 generation.
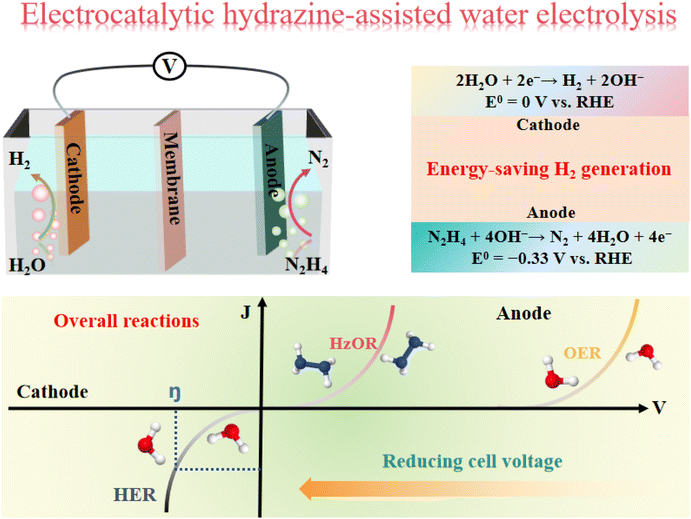 |
| Fig. 1 An illustration of the electrocatalytic hydrazine-assisted water electrolysis. | |
3. The design strategies of efficient electrocatalysts
3.1 Noble metal-based materials
Materials based on noble metals, including Pt, Au, Rh, Pd, and Ru, are promising electrocatalysts for various electrocatalysis reactions. However, the main obstacles to their practical application are still their high cost and scarcity. Therefore, devising feasible ways to improve the intrinsic mass activity of noble metal-based materials is urgent. Recently, the precious metal rhodium (Rh) emerged as a star material in the Pt-group metal family because of its unique and fascinating catalytic activity for energy-related electrocatalytic reactions.76–78 Rh-based materials have also been widely developed as excellent electrocatalysts for HzOR. Most of the work reported to date demonstrates that the regulation of a desirable morphology of the catalysts is directly related to the optimization of the catalytic activity targeting HzOR. For example, the porous single-metal Rh nanosheets (Rh-NSs) are developed by the in situ transformation of metastable trigonal RhO2 in an H2 atmosphere (Fig. 2a and b).79 The tunable lattice strain in Rh-NSs has been confirmed to be directly related to the reactivity of HzOR (Fig. 2c). The optimal Rh-NSs-300 shows better catalytic performance than pure Rh, which may be due to the reduced Gibbs free energy of the potential determining step on Rh-NSs as catalysts with tunable compressive strain (Fig. 2d and e). In addition, controlling the exposed crystal plane of the precious metal-based materials is another important means to improve the catalytic performance. For example, Rh nanocubes with specific exposed (100) planes are highly active bifunctional electrocatalysts for HzOR-assisted water splitting (Fig. 2f–h).80
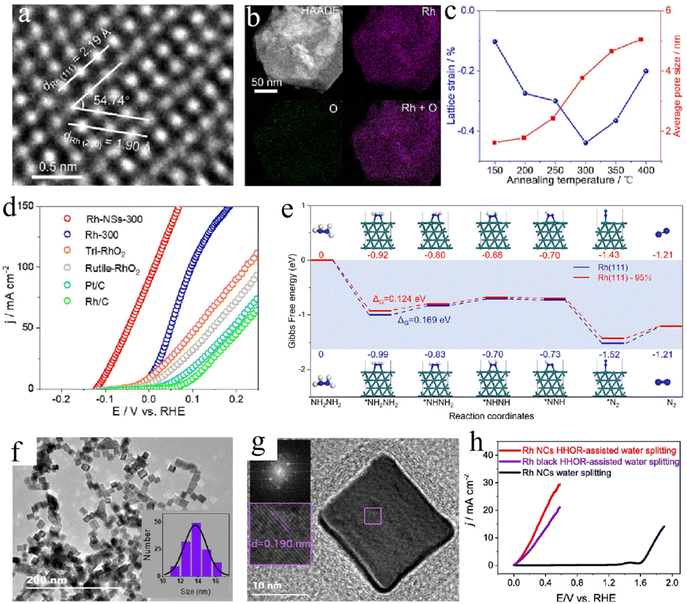 |
| Fig. 2 (a) The scanning transmission electron microscopy (STEM) and (b) energy dispersive spectroscopy (EDS) mapping images of Rh nanosheets. (c) Relationships among the lattice strain, average pore size, and annealing temperatures for Rh nanosheets. (d) HzOR polarization curves of various samples in 1.0 M KOH/0.5 M N2H4. (e) Density functional theory (DFT) calculations of Rh nanosheets for HzOR.79 Copyright 2023. Reproduced with permission from Elsevier. (f) Transmission electron microscopy (TEM) and (g) High-Resolution TEM (HRTEM) images of Rh nanocubes. (h) Polarization curves of HzOR-assisted water splitting for Rh nanocubes.80 Copyright 2021. Reproduced with permission from Elsevier. | |
However, according to the Sabatier principle, monometallic materials usually suffer from limited catalytic performance, as well as instability, due to the single linear adsorption behavior of reaction intermediates under practical operating conditions.81 This phenomenon has been confirmed experimentally for the d-band center of metals, where the reaction kinetics can be adjusted by doping foreign non-metallic atoms.82–84 For example, N-doped mesoporous Ru films and B-doped 2D Ir nanosheets array have been grown on nickel foam as excellent bifunctional electrocatalysts for HER and HzOR (Fig. 3a and b).85 The OHzS devices thus built exhibit low voltages and high stability for H2 production (Fig. 3c). On the other hand, metal cation doping is also an important strategy for regulating the electronic structure and catalytic activity of precious metals.86–89 For example, trace Co is doped into the Ru matrix to form a highly dispersed RuCo material, which exhibits significantly boosted bifunctional activity due to the optimization of the rate-determining energy barrier of HER and HzOR reactions.90 However, decreasing the amount of precious metal catalysts required is still a major concern in the field of electrocatalysis.
 |
| Fig. 3 (a) EDS mapping images and (b) the water contact angles of Ru–Cu2O. (c) Polarization curves of OHzS and OWS.85 Copyright 2023. Reproduced with permission from Elsevier. (d) The scanning electron microscopy (SEM) image of Ru–FeP4/IF. (e) The device of drainage method and (f) the generated volume of N2 and H2 gases at different times. Free energy diagrams of (g) HER and (h) HzOR on FeP4 and Ru–FeP4 samples.91 Copyright 2022. Reproduced with permission from Elsevier. | |
The introduction of precious metals as dopants into non-precious metal supports can not only reduce the usage of precious metals, but also maintain excellent electrocatalytic performance. Therefore, this has proved to be an effective strategy for the construction of high-performance electrocatalysts.92–94 For example, a 3D self-supported electrode decorated with Ru-doped Cu2O nanochains was fabricated and exhibited superior bifunctional performance for HER and HzOR.85 The improved reaction kinetics benefit from the unique porous structure, specific morphology with a hydrophilic surface, and Ru doping. Impressively, a low voltage of 17.4 mV was obtained at 10 mA cm−2 for an HzOR-assisted water electrolyzer. Similarly, Ru-doped FeP4 nanosheets are grown on iron foam as an efficient self-supporting bifunctional electrocatalyst for H2 production coupled with HzOR (Fig. 3d–f).91 The small voltage of 0.90 V at 1 A cm−2 and 100% faradaic efficiency of H2 release are obtained for the OHzS reaction. Density functional theory (DFT) calculations suggest that the modulated electronic structure and the improved adsorption-free energy of intermediates are synchronously achieved by trace Ru doping (Fig. 3g and h). Inspired by this, more and more materials doped with precious metals, such as defective Ru-doped α-MnO2 nanorods, Ru-doped VOx/Ni3S2 amorphous/crystalline heterostructure, Ru-doped oxygen-defective Co3O4 nanosheet array, and microsphere-like Ir-doped Ni/Fe-based MOF arrays, have been widely developed as high-performance electrocatalysts for hydrazine hydrate-assisted electrolysis.95–98
Alloying strategies have recently emerged as an effective way to rationalize the design of specific nanomaterials, which benefits from the increasing development of precision synthesis technology.99 Alloying various metals not only adjusts the morphology and structure of the final material, but also increases the intrinsic electrocatalytic activity and stability of electrocatalysts to avoid deactivation.100–102 For the HzOR-assisted water electrolysis, a series of advanced materials have been exploited via the alloying method. The design of ultrathin metallene may offer abundant favorable active sites and promote the utilization of metal atoms. For example, Wang and co-authors developed a trace Pt-doped Rhene by a simple solvothermal strategy (Fig. 4a).103 This kind of Pt–Rhene shows excellent bifunctional performance with an ultralow cell voltage of 0.28 V at 100 mA cm−2 for hydrazine-assisted water electrolysis, which mainly originates from the synergistic effect of dual active sites, the optimized d-band center, and improved reaction kinetics (Fig. 4b and c). In addition, similar bimetallic RhIr mesoporous nanospheres, AuPt alloys, and ultrafine RuPd alloy nanoparticles have been reported with superior electrocatalytic activity for OHzS.104–106
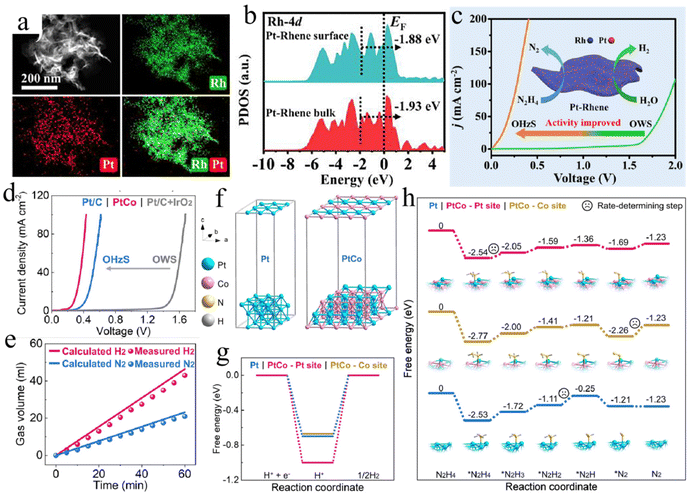 |
| Fig. 4 (a) EDS mapping images of Pt–Rhene. (b) The partial density of states (PDOS) of bulk and surface of Pt–Rhene. (c) Polarization curves of OHzS and OWS by using Pt–Rhene as catalysts.103 Copyright 2023. Reproduced with permission from Elsevier. (d) Two-electrode polarization curves of Pt/C, PtCo, and Pt/C + IrO2 for OHzS and OWS. (e) The volumes of N2 and H2 gases generated at different times. (f) Structural models of Pt and the PtCo alloy. (g) Free energy diagrams of (g) HER and (h) HzOR on various samples.107 Copyright 2022. Reproduced with permission from Elsevier. | |
In addition to binary precious metal alloys, the use of non-precious metals to build alloys is an ideal way to improve catalytic performance and reduce electrolysis costs. For example, hydrogen bubbles are generated in situ and used as dynamic templates to construct surface-cleaned trimetallic nanosponges of Pt53Ru39Ni8 and nanochain-like networks of PdNiRu alloy.108,109 Both electrocatalysts display dramatically enhanced catalytic performance. In addition, nanosheets of the PtCo alloy are developed as bifunctional electrocatalysts for a proton exchange membrane hydrazine electrolyzer (PEMHE) with acidic electrolytes (Fig. 4d and e).107 The optimized adsorption-free energy of H* and reduced dehydrogenation-free energy of N-based intermediates are realized by alloying Pt with Co (Fig. 4f–h). As a result, the PEMHE device achieves a high H2 yield rate of 1.87 mmol h−1 cm−2 at 0.6 V, 100% removal efficiency of hydrazine, as well as excellent stability over 60 h at 100 mA cm−2. In addition, there has been a rapid development of PdCo alloy nanoparticles, amorphous RhPb nanoflowers, Cu2O-derived PtCu nanoalloy, and Cu1Pd3/C and RuSb nanobranches as excellent electrocatalysts for HER and HzOR. These innovative materials indicate the feasibility of improving the electronic structure and catalytic properties of noble metal hosts by alloying them with non-noble metals.110–114
In recent years, high-entropy alloys have received extensive attention in the field of electrocatalysis due to their tunable multi-component and electronic structures.116–120 For example, Xia's group designed and synthesized extremely small NiCoMoPtRu alloy nanoclusters with only seven atomic layers (1.48 nm) and high entropy for HzOR-assisted electrolytic H2 generation (Fig. 5a and b).115 This high-entropy alloy exhibits a small overpotential of 9.5 mV at 10 mA cm−2 and superior mass activity of 12.85 A mg−1 of noble metals at −0.07 V. The OHzS electrolyzer thus constructed only needed a working voltage of 0.181 V to achieve 100 mA cm−2, which outperformed the other well-known chemical-assisted H2 generation systems (Fig. 5c–e). Theoretical calculations suggest that the fully active sites on high-entropy nanoclusters and some super-active sites are the key to achieving best-level performance (Fig. 5f and g).
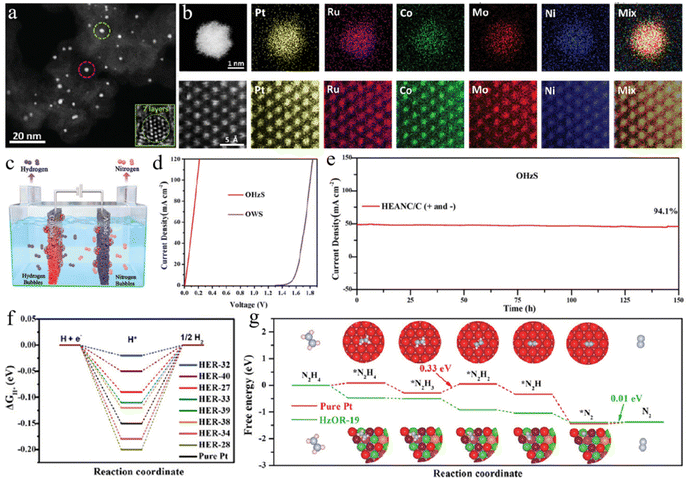 |
| Fig. 5 (a) High-angle annular-dark-field STEM (HAADF-STEM) image and (b) EDS elemental mapping images of high-entropy alloy nanoclusters (HEANCs). (c) Schematic illustration of the device and (d) polarization curves of OHzS and OWS by using HEANCs as catalysts. (e) The stability test of two-electrode electrolyzers. Free energy diagrams of (f) HER and (g) HzOR on various samples.115 Copyright 2024. Reproduced with permission from Wiley. | |
Interface engineering has been demonstrated as an effective strategy for material design to improve the performance of catalysts.123–125 The coupling of different components can promote electron transfer, expose catalytic active sites at the interface, as well as optimize the physical and chemical properties of the catalysts, which are closely related to the electrocatalytic performance.126 In recent years, carbon-based materials have been widely adopted as promising matrices to anchor metal-based catalysts.127,128 For example, N, S co-doped hollow carbon spheres are used as supporters to anchor Ru clusters or Rh nanoparticles by regulating the coordination environment surrounding the metal–S bonds, leading to superior bifunctional activity and stability for HER and HzOR reactions.129 In addition, other kinds of P, N-doped porous carbon or hollow dodecahedron carbons have also been developed for the decoration of RuP and Pd nanoparticles, respectively.130,131 Both materials achieve remarkable performance due to the optimized adsorption/desorption sites on the electrocatalyst. Similarly, N-doped porous carbon supports ultrafine Ir nanoclusters and Ru nanoparticles, Rh2S3/N-doped carbon material, Ag nanoparticles-decorated Fullerene, and the porous carbon microsheets anchored with RuP2 nanoparticles are synthesized by various chemical strategies; they show remarkable bifunctional performance for HER and HzOR.132–136 However, it is difficult to form a strong coupling heterogeneous interface between metals and the carbon carrier, resulting in limited performance in practical applications.137–139
Recently, oxide-supported metal catalysts have been used extensively to study the influence of metal–support interactions on catalytic performance.140 The oxygen atoms at the metal/oxide interface are proven to have uniquely high reactivity, while the synergistic effect of metal/oxide contributes to the optimization of the reaction energy barrier. Therefore, the modulation of the metal/oxide interface has an indispensable role in electrocatalysis.141 For example, Rh/RhOx nanosheets are synthesized by alkali-assisted synthesis, and the construction of highly active Rh–O–Rh interfaces endows ultra-low voltages in pH-universal conditions for H2 generation (Fig. 6a–c).121 In addition, fabric-like Rh2O3–NiWO4 nanosheets and RuO2/Co3O4 are also developed as superior electrocatalysts due to an abundance of exposed active sites on the heterostructure, as well as the enhanced metal–support interactions.52,142 Furthermore, the metal/metal interface is also important in regulating the binding energy of metal–hydrogen and N–N bond cleavage. For example, Duan's group reported Ru@Ag nanoparticles with abundant Ag–Ru interfaces, which acted as excellent bifunctional electrocatalysts for hydrazine-assisted water electrolysers (Fig. 6d).122 The optimized desorption energy of H2 and N2 and the higher barrier of N–N bond cleavage are confirmed in the Ru@Ag catalyst, leading to high electrocatalytic activity and selectivity (Fig. 6e–h). Meanwhile, the heterointerface Rh/Pd metallene and Au–Rh2P mesoporous nanotubes are confirmed to have a strong electronic effect, highly exposed active sites, as well as optimal adsorption energy of intermediates, contributing to the excellent performance for HzOR-assisted H2 production.143,144
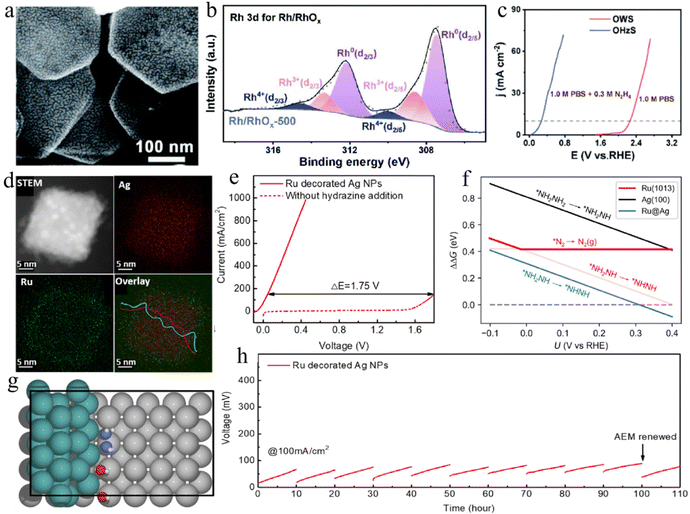 |
| Fig. 6 (a) SEM image, (b) X-ray photoelectron spectroscopy (XPS) spectra and, (c) polarization curves of OHzS and OWS for the Rh/RhOx-500 sample.121 Copyright 2022. Reproduced with permission from RSC. (d) EDS mapping images and (e) polarization curves of OHzS and OWS of Ru@Ag NPs. (f) DFT calculations of potential-determining steps for various catalysts. (g) Representative model of Ru@Ag. (h) Stability test of the Ru@Ag NPs‖Ru@Ag NPs electrolyser.122 Copyright 2024. Reproduced with permission from RSC. | |
An in-depth study and understanding of the reaction mechanism is significant for HzOR-assisted water electrolysis. A better knowledge in this field will drive the urgent requirement for precise design and construction of advanced electrocatalysts. In this regard, single-atom catalysts are undoubtedly an important model material due to the strong exposure of active metal atoms and the nearly 100% efficiency of atomic utilization, which boosts the development of electrochemical performance.145–147 Typically, carbon-based materials are an important support for single metal atoms. For example, a single atom of iridium anchoring the N-doped carbon layer and single atoms/clusters of Ru decorating N-doped carbon have been developed as robust electrocatalysts for HER and HzOR.148,149 However, the stability of such material remains an important challenge, especially in long-term electrolysis processes with high current density. Thus, metal-based oxides or sulfides have been exploited for the dispersion of single noble metal atoms. For example, the spinel Co3O4 with octahedral structure is adopted as a substrate for the lattice-confined Ru sites, causing a reduced energy barrier for the desorption of H* and the formation of N2H2* species on Ru–Co3O4 catalyst (Fig. 7a–d).150 Remarkably, a small potential of −0.024 V at 100 mA cm−2 and a high output of 0.48 kW h electricity per m3 H2 is realized (Fig. 7e–h). In addition, urchin-like oxygen vacancy-rich WO3 is also confirmed as an effective matrix for the immobilization of Ru single atoms, resulting in superior performance for pH-universal HzOR-assisted water splitting.151 The –O terminate Ti3C2Ox is regarded as a good electron promoter for enhancing the activity of heterojunctions. Luo and co-authors confirmed that the oxygen-functionalized Ti3C2Ox MXene is promising support for the decoration of highly dispersed single atoms of Rh. The Rh–SA/Ti3C2Ox catalyst achieves remarkable catalytic activities for pH-universal HER and HzOR reactions, as well as for the ultrahigh H2 generation rate of 45.77 mmol h−1.152
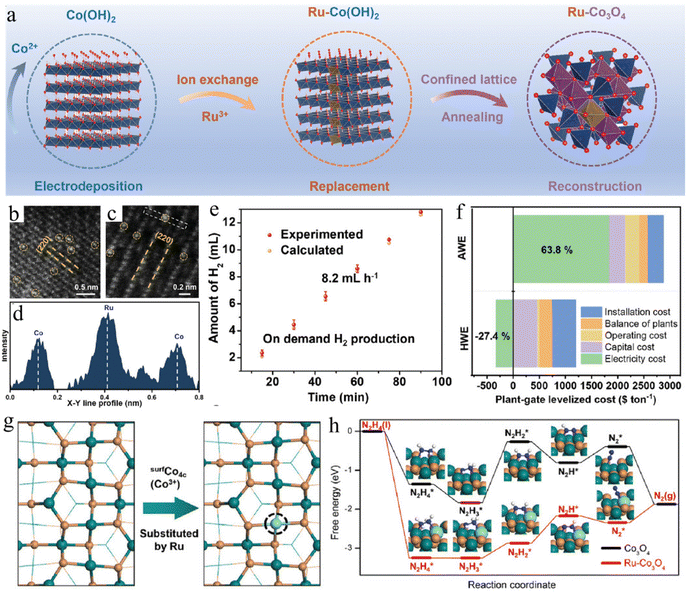 |
| Fig. 7 (a) Schematic illustration of the synthesis process, (b and c) HAADF-STEM images, and (d) corresponding intensity profiles of Ru–Co3O4. (e) The comparison of H2 volume between theoretical and experimental tests for HER. (f) Techno-economic analysis of various systems. (g) Calculated models of Ru–Co3O4 and (h) free energy diagrams of HzOR on Ru–Co3O4 and Co3O4 samples.150 Copyright 2024. Reproduced with permission from Wiley. | |
3.2 Non-noble metal-based materials
Considering some inherent shortcomings of precious metal-based catalysts, such as high prices, scarcity of reserves, and weak stabilities, researchers have devoted efforts toward exploiting non-noble metal-based materials for energy-saving H2 generation by HzOR-assisted water electrolysis. Therefore, the development of advanced electrocatalysts with lower cost and superior activity has achieved significant progress, including non-precious metal single-atom catalysts.154,155 For example, Yuan et al. developed a Ti3C2Tx–MXene rich in Ti vacancies as a trap that anchored substrates for the dispersion of individual Ni single atoms by a self-reduction strategy (Fig. 8a–c).153 The strong Ni–C interaction around three neighboring C atoms is realized on the Ni atoms that occupy the Ti vacancies. As a result, the Ni SACs/Ti3C2Tx exhibits a small onset potential of −0.03 V and excellent catalytic stability (Fig. 8d). DFT calculations suggest that hydrazine adsorption is promoted and the energy barrier of HzOR is optimized from the redistributed electronic density of states around the Ni–C bonds (Fig. 8e). In addition, single atomic Mn sites are embedded in a B, N co-doped carbon matrix, which is confirmed to have superior bifunctional performance for HER/HzOR reactions under alkaline conditions. This Mn–SA/BNC catalyst delivers low potentials of −51 mV at −10 mA cm−2 and 132 mV at 10 mA cm−2 for the HER and HzOR, respectively.156 In addition to the design of a single atom, non-noble metal-based alloys, such as Co–Mo alloy, nanotubular Ni–Cu alloy, Ni(Cu) cubic nanopore, and porous Ni(Cu) coatings, have also been developed via different chemical strategies; they are reported to demonstrate superior electrocatalytic activity for the HER and HzOR.157–160
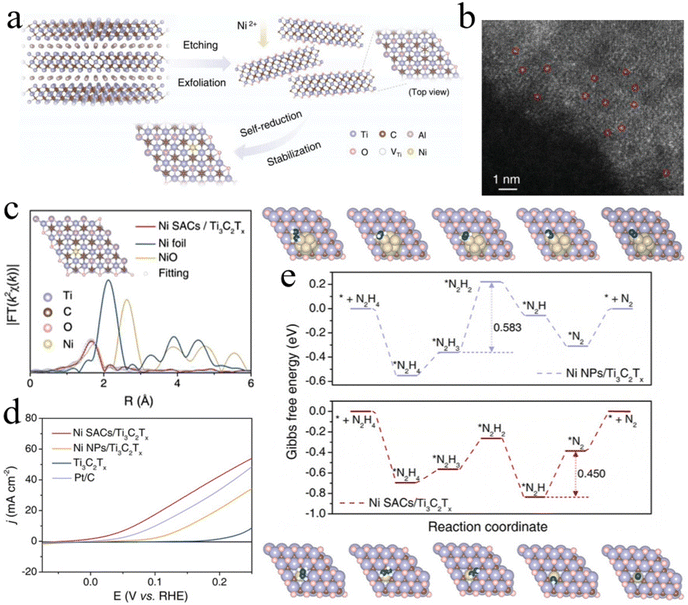 |
| Fig. 8 (a) Schematic diagram of the synthesis and (b) HAADF-STEM image of Ni SACs/Ti3C2Tx. (c) The k2-weighted Fourier-transform extended X-ray absorption fine structure (FT-EXAFS) spectra of Ni K-edge and (d) polarization curves of OHzS for various samples. (e) Free energy diagrams of HzOR on Ni SACs/Ti3C2Tx and Ni NPs/Ti3C2Tx samples.153 Copyright 2022. Reproduced with permission from Wiley. | |
In recent years, both anionic modification and cationic doping strategies have been widely used to design various electrocatalysts with unique advantages in regulating catalytic performance.161–164 Firstly, the anion modification strategy can achieve a synergistic optimization of the electronic structure and catalytic properties of the materials without the introduction of additional metal centers; it has been widely used in the field of HzOR-assisted H2 production. For example, N-doped M/CoO (Ni, Co, and Mn) heterostructure microspheres, P-doped CoCO3 nanosheets, S-doped tri-metallic NiCoFe phosphide, and S-rich MoS2 materials have been synthesized to study their catalytic performance in the HER or HzOR.58,165–167 On the other hand, metal cation doping can not only regulate the electronic structure of the supports but also introduce additional catalytic active centers, which has been considered to be an effective way to design high-performance bifunctional electrocatalysts. Recently, transition metal-based chalcogenides, such as sulfides and selenides, as well as other metal-based nitrides and phosphides, have gained extensive attention for various catalytic purposes due to their unique structural properties and intrinsic high stability.168–172 For example, a series of metal-doped transition metal-based catalysts, including Mn-doped CoS2, Fe-doped Ni2P–Co2P–Zn3P2 heterogeneous material, V-doped Ni3N nanosheet, porous Ce-doped Ni3N nanosheet arrays, Fe-doped Ni2P/CoP, Fe-doped Co3N, Ni0.6Co0.4Se, and Fe-doped CoS2 nanosheets have been developed and their remarkable advantages as bifunctional electrocatalysts in HzOR-assisted seawater splitting have been confirmed.173–180
Recently, a dual-modification strategy has emerged as an alternative way to further improve the performance of catalysts. The He group developed a dual-cation co-doping strategy to improve the HzOR and HER performance of Ni2P via control lattice strain engineering.182 The Cu1Co2 co-doped Ni2P induces an optimal compressive strain of −3.62% on the optimized d-band center and reduces the energy barrier of the catalytic process, thereby achieving superior bifunctional activity for both the HzOR and HER. In addition, Zhang and co-authors designed and fabricated an integrated electrode by growing P in situ and co-doping Co3N nanowire arrays with W on a nickel foam substrate (Fig. 9a–c).181 DFT calculations revealed that the co-doping of P/W not only endows the improved dehydrogenation of adsorbed *NH2NH2, but also optimizes the free energy of adsorbed H*, resulting in the highly efficient bifunctional activity with an ultra-low operation voltage of 277 mV at 200 mA cm−2 for the OHzS reaction (Fig. 9d–h).
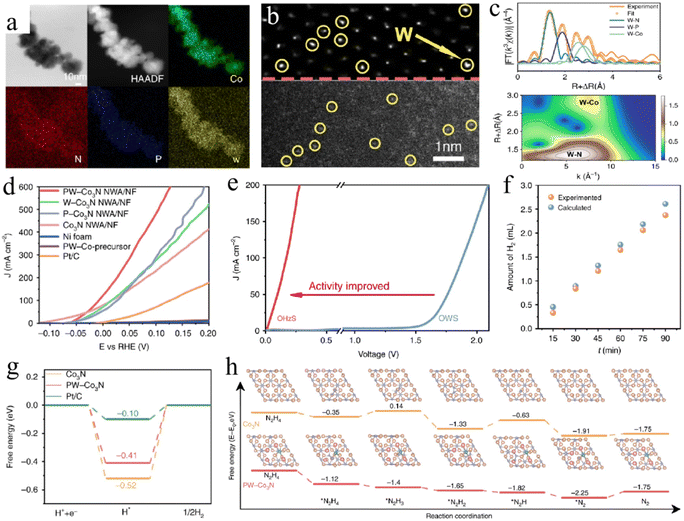 |
| Fig. 9 (a) The EDS mapping images and (b) HAADF-STEM images of PW-Co3N nanowires. (c) W L3-edge extended XAFS (EXAFS) fitting curves and wavelet-transformed (WT) contour plots of PW-Co3N sample. (d) HzOR polarization curves of various samples. (e) Polarization curves of OHzS and OWS with PW-Co3N as catalysts. (f) The comparison of H2 volume between calculations and experimental tests. Free energy diagrams of (g) HER and (h) HzOR on various samples.181 Copyright 2020. Reproduced with permission from Nature. | |
In the past few decades, the design of heterostructures of non-precious metal-based materials has been an important research field. For HzOR-assisted water electrolysis, a variety of heterogeneous materials have been widely designed and studied for their catalytic performance. The application of transition metal oxides is limited by their relatively weak intrinsic conductivity. Therefore, heterogeneous interface manipulation based on non-noble metal oxides is an ideal way to design high-performance electrocatalysts.195 Recently, various heterogeneous materials, such as Co(OH)2/MoS2 nanosheets, MoO2/Co, MoOx/MoS2 core–shell nanowires, NiOOH@cobalt copper carbonate hydroxide, SNiC2O4–Nb2O5, FeWO4–WO3, Co3O4/Co, Co/amorphous LaCoOx, and Ni(OH)2/Ni2P, have been developed as highly effective electrocatalysts for energy-saving H2 generation via the HzOR-assisted process.189,196–203 In addition, transition metal nitrides/phosphides have emerged as promising models for heterostructure engineering because of their versatile advantages, such as high stability and high conductivity. For example, CoP/Co Mott–Schottky material, Ni2P/Zn–Ni–P nanosheet, 1D/3D CoN–Co2N, Ni(Cu)@NiFeP, NiCoP@CoFeP, and porous Ni3N–Co3N nanosheets have been assessed and confirmed to have superior bifunctionality toward HER and HzOR.64,184,188,191,204–206 However, the lattice mismatch for heterojunction engineering is a universal challenge for many heterostructures, which hinders electron transfer at the interface, leading to limited bifunctional performance. Thus, the development of lattice-matched dual-phase materials with abundant interfaces is required to boost the electron transfer efficiency.
Recently, Yang and co-authors constructed a heterojunction with biphasic metal nitrides (Ni3N–Co3N) containing highly lattice-matched interfaces (Fig. 10a).191 The electron redistribution at the enhanced local electric field between electron-rich Ni3N and electron-deficient Co3N is triggered by Mn doping (Fig. 10b and c). As a result, this Mn@Ni3N–Co3N/NF bifunctional electrocatalyst achieves a small voltage of 0.49 V at 500 mA cm−2 and at least 53.3% energy consumption of H2 generation compared to traditional water splitting (Fig. 10d–f). On the one hand, DFT simulations indicate that highly active Ni species and electron-deficient Co centers contribute to the optimization of the adsorption energy of H2O, H*, and N2H4. On the other hand, researchers have confirmed that heterostructures designed using materials with intrinsically high HER activity can simultaneously improve the bifunctional activity of the HER and HzOR. In this regard, the NiMo alloy, being a typical high-efficiency HER material, has been widely studied.207–210 For example, hierarchical NiMo/Ni2P ohmic contact heterojunction was developed by the Shen group to achieve a low cell voltage of 343 mV at high current densities of 500 mA cm−2 for the OHzS electrolyzer (Fig. 10g–i).187 Moreover, an abundance of NiCo/MoNi4 heterostructure interfaces and metallic hierarchical Co/MoNi heterostructures have been demonstrated to be highly active centers for both the HER and HzOR.185,211 Multiple modulation strategies to optimize the electrocatalytic activity of materials have been proven to be feasible. For example, both heterostructure interfaces and heteroatom substitution have been adopted for the design and synthesis of Mo–Ni3N/Ni, N–Ni5P4@CoP, and Fe/F–Ni2P electrocatalysts for the OHzS systems.190,212,213
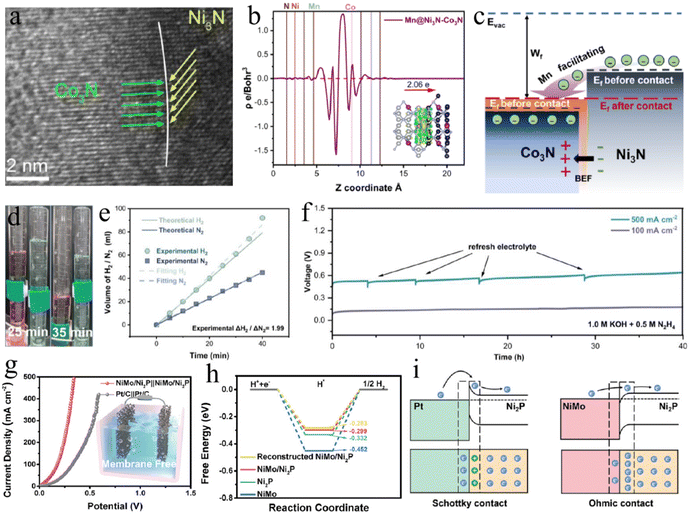 |
| Fig. 10 (a) HRTEM image and (b) average charge density difference of Mn@Ni3N–Co3N. (c) Schematic illustration of the enhanced charge transfer by Mn. (d) The picture of the gas-collection device and (e) the volumes of H2 and N2 at different times. (f) The stability tests of OHzS by Mn@Ni3N–Co3N/NF.191 Copyright 2024. Reproduced with permission from Wiley. (g) Polarization curves of OHzS with NiMo/Ni2P and Pt/C as catalysts. (h) Free energy diagrams of HER. (i) The difference of energy bands between Schottky and ohmic contacts.187 Copyright 2024. Reproduced with permission from Wiley. | |
As mentioned above, carbon supports are common materials for supported metal catalysts. As a typical representative, reduced graphene oxide is widely used to improve the catalytic activity and stability of catalysts due to its ultra-thin two-dimensional characteristics and high conductivity.214 For example, N-doped NiZnCu-layered double hydroxides have been grown in situ on the reduced graphene oxide (N–NiZnCu LDH/rGO), representing a promising bifunctional catalyst for HER and HzOR.215 Moreover, the active Ni–P species are cathodically deposited onto reduced graphene oxide nanosheets, achieving improved conductivity for excellent stability and catalytic activity for HzOR-assisted HER system.216 On the other hand, direct pyrolysis of precursors to form carbon-supported metal catalysts is a widely used approach. The Li group utilized dual-heteroatom-containing metal–organic framework sheets to synthesize Co nanoparticles embedded with B and N co-doped carbon matrix to achieve a small voltage of 0.617 V at 100 mA cm−2 for the OHzS electrolyzer.217 However, for carbon-supported catalysts, to the accurate construction of the catalytic active sites and interface structure is key to improving their bifunctional activity and stability. Xie and co-authors confirmed that the controllable construction of dual nanoislands on Ni/C nanosheet array is an effective way to integrate dual active sites for both HER and HzOR (Fig. 11a–c).193 DFT results suggest that a more thermoneutral H* adsorption on the C sites and favorable N2H4 dehydrogenation kinetics on the surface can expose the Ni centers, and thus, are the main factors for the boosted bifunctionality. Remarkably, this catalyst delivers low potentials of −37 mV and −20 mV at 10 mA cm−2 for HER and HzOR, respectively (Fig. 11d–i).
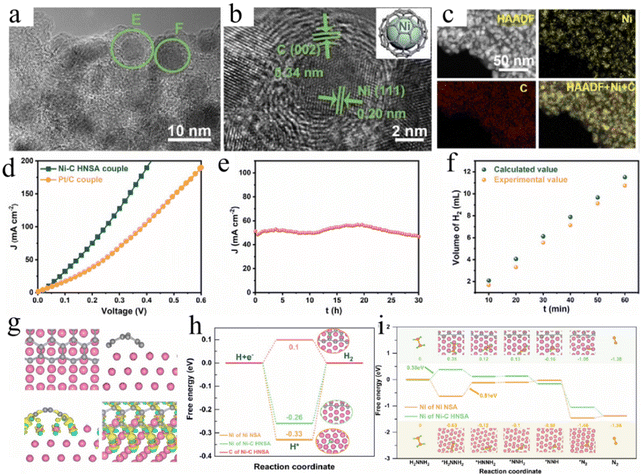 |
| Fig. 11 (a–c) The TEM, HRTEM, and EDS mapping images of Ni/C hybrid nanosheet array. (d) Polarization curves of OHzS by using Ni/C and Pt/C as catalysts. (e) The stability tests of OHzS and (f) the generated H2 volume with different times. (g) A structural model of Ni/C, and free energy diagrams of (h) HER and (i) HzOR on various samples.193 Copyright 2022. Reproduced with permission from Wiley. | |
The highly reactive metal species in metal-based catalysts are generally considered to be the main catalytically active centers of HER and HzOR. Therefore, most of the reported catalysts are based on noble or non-precious metal materials to develop high-performance electrocatalysts. Recently, some studies have shown that non-metallic materials can also be used for HzOR-assisted H2 production, although the catalytic performance of such materials is limited. For example, the You group reported the fabrication of a metal-free pyridinic nitrogen-rich carbon paper (N-CP-800) by electropolymerization of aniline via an annealing treatment (Fig. 12a–e).192 The abundant pyridinic-N exists as zig and arm configurations in N-CP-800, allowing the favorable rate-limiting proton desorption of reaction intermediates for HzOR. As a result, this N-CP-800 catalyst shows a low potential of only 0.78 V at 10 mA cm−2 for HzOR under neutral conditions (Fig. 12f–h). However, although the coupling of N-CP-800 and CoP can help achieve a small cell voltage of 1.56 V at 10 mA cm−2 for HzOR-assisted seawater electrolysis, the potential application of this kind of metal-free material is still challenging.
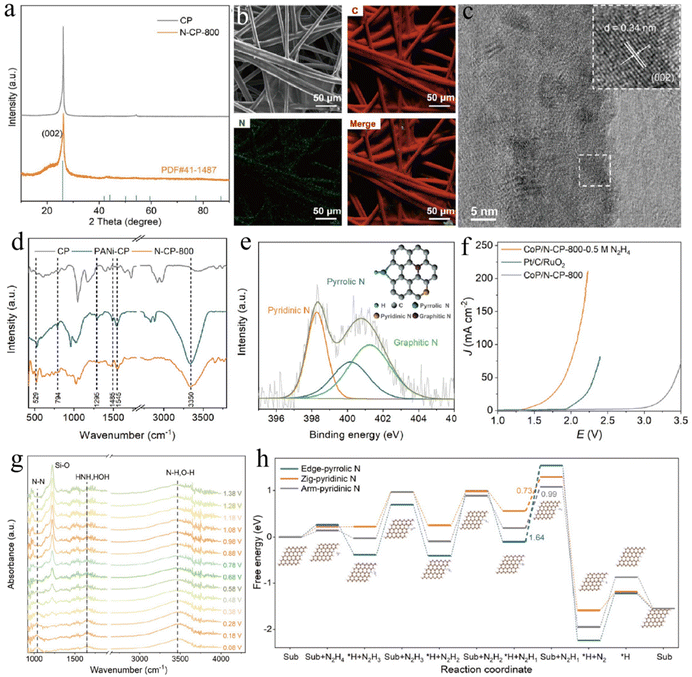 |
| Fig. 12 (a) The X-ray diffraction (XRD) patterns, (b) SEM mapping images, (c) TEM and HRTEM images of N-CP-800. (d) Fourier transform infrared spectroscopy (FTIR) spectra of CP, PANi-CP, and N-CP-800 samples. (e) XPS spectrum of N 1s for N-CP-800. (f) Polarization curves of various samples and (g) in situ FTIR spectra for OHzS. (h) Free energy diagrams of HzOR on different N-sites.192 Copyright 2024. Reproduced with permission from Springer. | |
To sum up, the improvement in performance of advanced electrocatalysts by designing heterostructures, doping, or alloying, may additionally benefit from their optimized electronic interaction and synergistic effects (Table 1). However, building precise structural models of these catalysts to study the structure–activity relationship is challenging. Moreover, the single-atom strategy is a promising model for studying the catalytic mechanism of HzOR-assisted water electrolysis. However, their catalytic activity and stability during long-term testing have been unsatisfactory. Therefore, the development of advanced synthesis methods and characterization techniques remains important for the development of high-performance electrocatalysts for HzOR and HER reactions.
Table 1 Performance comparison of representative non-noble metal-based electrocatalysts for HzOR-assisted water electrolysis
Catalysts |
HzOR |
HER |
OHzS |
Potential at 10 mA cm−2 (mV) |
Tafel slope (mV dec−1) |
η
10 (mV) |
Tafel slope (mV dec−1) |
Voltage at 10 mA cm−2 (mV) |
P, W co-doped Co3N181 |
−55 |
14 |
41 |
40 |
28 |
Ce–Ni3N/NF178 |
92 |
47 |
— |
— |
256 |
Fe–CoNiP@NC175 |
280@1 A cm−2 |
54.7 |
490@1 A cm−2 |
86.09 |
560@1 A cm−2 |
Cu1Co2–Ni2P/NF182 |
−52 |
14.4 |
51 |
52.3 |
160 |
NiCoMoPtRu/C115 |
50@3.26 A mg−1 |
32 |
9.5 |
29.8 |
25 |
CoH–CoPV@CFP183 |
−61 |
59.9 |
77 |
75.2 |
230@0.5 A cm−2 |
CoN–Co2N@NF184 |
−110 |
42 |
71 |
84 |
53 |
NiCo–MoNi4 HMNAs/NF185 |
−30 |
28.5 |
68 |
67.5 |
630@0.25 A cm−2 |
(P–Co/Ni3P)A3/NF55 |
−79 |
1.8 |
10 |
45 |
50@0.3 A cm−2 |
B–CoNS@CuPD186 |
−146 |
21.7 |
70 |
76.6 |
60 |
NiMo/Ni2P187 |
−17 |
35.24 |
15 |
29.45 |
181@0.1 A cm−2 |
Ni3N–Co3N188 |
−88 |
21.6 |
43 |
35.1 |
71 |
Ni(OH)2/Ni2P/NF189 |
−14 |
22.0 |
72 |
82.7 |
80 |
Mo–Ni3N/Ni/NF190 |
−0.3 |
45 |
45 |
48 |
55 |
Mn@Ni3N–Co3N/NF191 |
1.65 V@0.5 A cm−2 |
19.3 |
310@0.5 A cm−2 |
117.2 |
490@0.5 A cm−2 |
CoP/N-CP-800 (neutral condition)192 |
780 |
99 |
140 |
108 |
780 |
Ni–C HNSA193 |
−20 |
16.2 |
37 |
31.9 |
140@50 mA cm−2 |
ZIF67@CoNiSe-3194 |
130@0.4 A cm−2 |
44.3 |
49 |
41.4 |
450@0.1 A cm−2 |
Ni SACs/Ti3C2Tx 153 |
−30 |
62 |
— |
— |
— |
Mn–SA/BNC156 |
132 |
75.9 |
51 |
51.2 |
0.41 |
4. Hydrazine-assisted seawater splitting
Although hydrazine-assisted H2 production by water electrolysis outperforms traditional petrochemical techniques due to its capacity as renewable, high processing efficiency, and environmental friendliness, the critical concerns focus on the massive requirement for high-purity water. In this regard, seawater has been regarded as a sustainable water resource to generate high-purity H2 due to its great volume, i.e., ∼97% of all water on our planet. However, direct seawater electrolysis faces huge challenges in its application for large-scale hydrogen generation because of the complex ionic chemistry of seawater. An important problem is the competitive chlorine evolution reaction (CER) with OER on the anode.220 The electrolysis efficiency and sustainability are largely hindered due to anodic dissolution and contamination from the generation of toxic and corrosive chlorine species.221 Another challenge in maintaining high electrolysis efficiency is preventing blocked active sites by the formed solid precipitates. Therefore, suppressing the CER process by reducing the overpotential of OER below 0.48 V in an alkaline medium is important. However, the demands of industry-level criteria for seawater electrolysis are far beyond this overpotential, forcing researchers to develop high-performance electrocatalysts or effective modification strategies to hinder the CER.
The alternative HzOR process has a far lower oxidation potential than CER, which contributes to eliminating the notorious problems of chlorine chemistry based on high current density and H2-yielding efficiency (Fig. 13a).218 Therefore, the coupling of HzOR with HER in a costless seawater electrolyte can become a promising and valuable avenue to achieve environmental sustainability and cost-effectiveness of H2 generation. In recent years, researchers have spent a lot of effort to develop high-performance electrocatalysts for HzOR-assisted seawater electrolysis. For example, the Qiu group reported a super aerophobic–hydrophilic NiCo/MXene-based electrode for chlorine-free H2 production. The hybrid hydrazine-assisted seawater electrolyzer only needs an ultrasmall voltage of 0.7 V, high H2 yields of 9.2 mol h−1 gcat−1, as well as high stability over 140 h at a high current density of 500 mA cm−2 (Fig. 13b).218 In addition, not only the chlorine-induced hazards on cell performance of NiCo/MXene-based electrolyzer can be effectively reduced, but also the consumption of electricity is significantly decreased by 30–52% at 500 mA cm−2 compared to the traditional alkaline water electrolysis (Fig. 13c). The excellent bifunctional performance of NiCo/MXene materials is attributed to the improved water/hydrazine adsorption capability, interfacial conductivity, strong structure stability, and gas-releasing pattern at large current densities. Moreover, a cobalt/nitrogen co-doped carbon nanosheet array (CC@CoNC) is developed by an in situ pyrolysis strategy of metal–organic framework (MOF).219 The hydrazine-assisted seawater electrolysis system delivers a small cell voltage of 0.557 V, as well as a low electricity expense of 1.22 kW h per cubic meter of H2 at 200 mA cm−2 (Fig. 13d–g). To improve the catalytic activity and stability of HzOR-assisted seawater electrolyzers, more and more regulatory strategies have been exploited to optimize the surface properties and electronic structure of electrocatalysts. For example, the noble metal-doped materials (Ru–Ni(OH)2 nanowire network, Ru–(Ni/Fe)C2O4, NiRh–MOF), non-noble metal-doped materials (Cu–CoFe/Co/NC, Fe/P co-doped NiMoO4, FeCo–Ni2P@MIL–FeCoNi), and heterostructured materials (CoP/Ni2P, MoNi/MoO2, bimetallic Ni4Mo/Ni4W nanoalloys) have emerged as bifunctional electrocatalysts with remarkable catalytic performance for HzOR-assisted seawater splitting.222–230
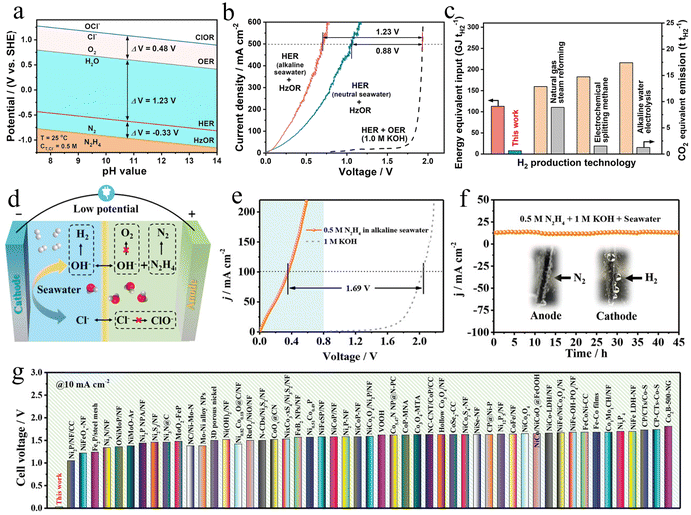 |
| Fig. 13 (a) The Pourbaix diagram of different reactions on artificial seawater. (b) Polarization curves of HER//HzOR and HER//OER in neutral or alkaline seawater media. (c) Energy equivalent input and CO2 equivalent emission of different H2 generation techniques.218 Copyright 2021. Reproduced with permission from Nature. (d) The schematic diagram of CC@CoNC-600 catalyst during seawater electrolysis. (e) Polarization curves of OHzS and OWS, and (f) the stability tests of OHzS using CC@CoNC-600 as a catalyst. (g) The comparison of the cell voltages between different catalysts.219 Copyright 2023. Reproduced with permission from Wiley. | |
5. Self-powered systems for hydrazine-assisted H2 production
Recently, considering the requirement of large-scale renewable energy systems for traditional seawater electrolysis, self-powered systems for hydrazine-assisted H2 production have attracted more and more attention due to the low electricity consumption of HzOR-assisted seawater electrolysis. Small-scale renewable energy systems (fuel cells or solar cells) are feasible for integration with hydrazine-based hybrid electrolyzers. For example, the Wang group developed hydrazine-assisted self-powered seawater electrolysis by coupling direct hydrazine fuel cell (DHzFC) with OHzS system for the production of high-purity H2.231 This self-powered device delivers a maximum power density of 37.06 mW cm−2 at 98.31 mA cm−2 and high H2 yield of 0.90 mmol h−1 with 1.0 M KOH + seawater + 0.5 M N2H4 electrolyte without an external power source (Fig. 14a–e). In addition, the DHzFC-powered OHzS device is built by using 6W–O–CoP/NF as the anode and features a maximal power density of 142 mW cm−2 and a superior H2 production rate of 3.53 mmol cm−2 h (Fig. 14f–h).232 The performance of self-powered systems can further be improved by using catalysts decorated with noble metals. For example, Ru1–NiCoP and RuFe–Ni2P have been further developed to achieve a maximum power density of 60.1 mW cm−2 at 157.8 mA cm−2 and 176 mW cm−2 at 0.40 V, confirming the feasibility of the application of self-powered H2 production.233,234
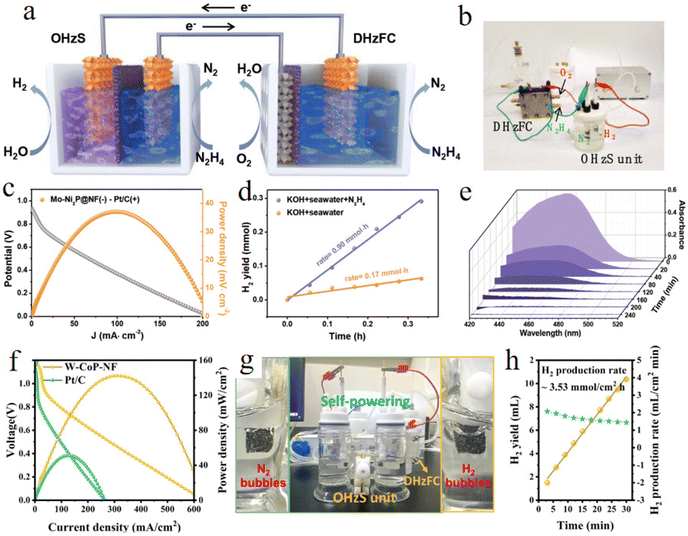 |
| Fig. 14 (a) Schematic illustration and (b) digital photograph of self-powered H2 production device integrated by driving the OHzS electrolyzer with DHzFC as power supply. (c) Discharge curve and power density of DHzFC. (d) The generation rates of H2 in different electrolytes. (e) Ultraviolet-visible (UV-vis) spectra of the industrial N2H4 wastewater at different test times.231 Copyright 2023. Reproduced with permission from Wiley. (f) Discharge curve and power density of W-CoP/NF and Pt/C for DHzFC. (g) Digital photograph of the self-powered device. (h) The yield and generation rate of H2 by using a DHzFC-powered system.232 Copyright 2023. Reproduced with permission from Springer. | |
In addition to the DHzFC-powered OHzS system, the solar cell is also applied to couple with OHzS by photovoltaic electrolysis (PV–EC) system. For example, Yong and co-authors demonstrate that the perovskite solar cells (PSC) can be used as a power source to drive the OHzS system.235 This kind of self-powered PV–EC system realizes excellent performance with a high photocurrent density of 23 mA cm−2 and a considerable H2 amount for hydrazine-assisted H2 production. Furthermore, the Chen group recently developed a pre-protonated vanadium hexacyanoferrate (p-VHCF)–N2H4 liquid battery, which can power the decoupled electrolysis of H2 generation and hydrazine oxidation at nighttime, providing a new idea for the renewables-to-hydrogen conversion.236
6. Conclusions and outlooks
In summary, this comprehensive review discusses state-of-the-art research progress on the cost-effective and efficient electrocatalysts for HzOR-assisted water electrolysis based on noble, non-noble metal-based, and metal-free materials to date. In terms of precious metals, many efforts have been devoted to optimizing the utilization efficiency through designing specific morphology, exposing high-active planes, or incorporating other low-cost transition metals or carbon materials to achieve a highly homogeneous dispersion of precious metal-based active sites. On the other hand, non-noble metal-based materials, such as oxides, phosphides, alloys, nitrides, and chalcogenides, as well as single-atom structures feature breakthroughs. Some of the advanced materials exhibit comparable performance to the benchmark noble metals. Specifically, the nanostructure engineering of electronic structure and surface properties of the advanced catalysts have been highlighted: (i) constructing specific structure or morphology to improve the exposed number of surface active sites, (ii) designing coupled heterogeneous interfaces or combining with highly conductive matrix to speed up electron transfer, thereby reducing the barriers to kinetic reactions, (iii) introducing heteroatoms to optimize the electronic configuration and improve the thermodynamic adsorption/desorption of reaction intermediates on the surface of catalysts. Although there are relatively few examples of metal-free catalysts, doping and design of defect structures are feasible ways to improve catalytic activity. With the development of various bifunctional electrocatalysts and the better catalytic performance of HzOR-assisted water electrolysis for H2 production, significant progress has been made toward practical application-oriented systems, such as with seawater electrolyte or self-powered systems, that represent an important research direction in this field for future studies.
However, despite the significant progress made for the coupling of sustainable and energy-saving H2 generation via small-molecule oxidation reactions with HER, there is still a long journey to continue before HzOR-assisted water electrolysis can be achieved for commercial and industrial applications. The co-existence of opportunities and challenges creates many possibilities for future studies as proposed below: (i) most of the reported synthetic strategies focus on studying the relationship between structure and activity while ignoring the enhanced promotion of industrial applications. Therefore, based on maintaining high activity and stability, preparing high-quality electrocatalysts or electrodes on a large scale and realizing the leap from the laboratory scale to the industrial level is of significance. (ii) Despite the coupling of thermodynamically favorable HzOR reactions that lower the cell voltage of a hybrid water electrolyzer, the anode feedstock of hydrazine is expensive, unstable, and toxic compared to the traditional water splitting. Moreover, the worthless oxidation products of HzOR also increase the separation costs. Therefore, the use of industrial wastewater with highly toxic hydrazine hazards instead of pure hydrazine hydrate solution is a more feasible option. Although some materials have been used in this research, the application of direct energy-saving electrolysis of industrial hydrazine hydrate wastewater for hydrogen production is rarely reported. (iii) Achieving high operational stability of electrocatalysts at large current density is a formidable task. Although the oxidation products (N2) from HzOR can avoid the safety issues generally associated with the H2/O2 mixture, the membrane electrodes assembly (MEA) devices are still necessary to achieve the direct separation of high-purity H2. Therefore, more high-performance electrocatalysts should be directly used for the application of actual MEA devices. (iv) More research efforts have been made to develop direct HzOR-assisted seawater electrolysis. However, most of the research is still based on evaluating performance in simulated alkaline seawater electrolytes. It is necessary to reveal whether some existing nanostructure-engineered electrocatalysts can be used for H2 production from HzOR-assisted real seawater electrolysis.
Data availability
No primary research results, software or code have been included, and no new data were generated or analysed as part of this review.
Conflicts of interest
The authors declare no conflict of interest.
Acknowledgements
This work was financially supported by the National Natural Science Foundation of China (22205205), the Zhejiang Provincial Natural Science Foundation of China (LQ22B030008), and the Science Foundation of Zhejiang Sci-Tech University (ZSTU) under Grant No. 21062337-Y.
References
- J. Chen, S. Huang, Y. Yang, Z. Li, S. Liu, Z. Zhuo, N. Lu, X. Zhou, Y. Liu, H. Li and T. Zhai, Interlayer Delocalized Electrons Activate Basal Plane for Ultrahigh-Current-Density Hydrogen Evolution, Nano Lett., 2024, 24(26), 8063–8070 CrossRef PubMed.
- D. Ding, Y. Liu and F. Xia, Interface Engineering via Molecules/Ions/Groups for Electrocatalytic Water Splitting, Nano Res., 2024, 17, 7864–7879 CrossRef.
- Q. Wen, J. Duan, W. Wang, D. Huang, Y. Liu, Y. Shi, J. Fang, A. Nie, H. Li and T. Zhai, Engineering a Local Free Water Enriched Microenvironment for Surpassing Platinum Hydrogen Evolution Activity, Angew. Chem., Int. Ed., 2022, 61(35), e202206077 CrossRef CAS PubMed.
- Q. Wen, K. Yang, D. Huang, G. Cheng, X. Ai, Y. Liu, J. Fang, H. Li, L. Yu and T. Zhai, Schottky Heterojunction Nanosheet Array Achieving High-Current-Density Oxygen Evolution for Industrial Water Splitting Electrolyzers, Adv. Energy Mater., 2021, 11(46), 2102353 CrossRef CAS.
- Y. Zhao, Y. Wu, Q. Wen, D. Huang, R. Yang, H. Wang, Y. Xu, M. Sun, Y. Liu, J. Fang, T. Zhai and L. Yu, Operando-Reconstructed Polyatomic Ion Layers Boost the Activity and Stability of Industrial Current-Density Water Splitting, Sci. Bull., 2024 DOI:10.1016/j.scib.2024.07.003.
- J. Wang, H. Yang, F. Li, L. Li, J. Wu, S. Liu, T. Cheng, Y. Xu, Q. Shao and X. Huang, Single-Site Pt-Doped RuO2 Hollow Nanospheres with Interstitial C for High-Performance Acidic Overall Water Splitting, Sci. Adv., 2024, 8(9), eabl9271 CrossRef PubMed.
- L. Wan, Z. Xu, Q. Xu, M. Pang, D. Lin, J. Liu and B. Wang, Key Components and Design Strategy of the Membrane Electrode Assembly for Alkaline Water Electrolysis, Energy Environ. Sci., 2023, 16(4), 1384–1430 RSC.
- H. Zhang, A. Chen, Z. Bi, X. Wang, X. Liu, Q. Kong, W. Zhang, L. Mai and G. Hu, MOF-on-MOF-Derived Ultrafine Fe2P-Co2P Heterostructures for High-Efficiency and Durable Anion Exchange Membrane Water Electrolyzers, ACS Nano, 2023, 17(23), 24070–24079 CrossRef CAS PubMed.
- L. Quan, H. Jiang, G. Mei, Y. Sun and B. You, Bifunctional Electrocatalysts for Overall and Hybrid Water Splitting, Chem. Rev., 2024, 124(7), 3694–3812 CrossRef CAS PubMed.
- A. Kumar, S. K. Purkayastha, A. K. Guha, M. R. Das and S. Deka, Designing Nanoarchitecture of NiCu Dealloyed Nanoparticles on Hierarchical Co Nanosheets for Alkaline Overall Water Splitting at Low Cell Voltage, ACS Catal., 2023, 13(16), 10615–10626 CrossRef CAS.
- X. Lin, W. Hu, J. Xu, X. Liu, W. Jiang, X. Ma, D. He, Z. Wang, W. Li, L.-M. Yang, H. Zhou and Y. Wu, Alleviating OH Blockage on the Catalyst Surface by the Puncture Effect of Single-Atom Sites to Boost Alkaline Water Electrolysis, J. Am. Chem. Soc., 2024, 146(7), 4883–4891 CrossRef CAS PubMed.
- N. Du, C. Roy, R. Peach, M. Turnbull, S. Thiele and C. Bock, Anion-Exchange Membrane Water Electrolyzers, Chem. Rev., 2022, 122(13), 11830–11895 CrossRef CAS PubMed.
- J.-T. Ren, L. Chen, H.-Y. Wang, W.-W. Tian and Z.-Y. Yuan, Water Electrolysis for Hydrogen Production: From Hybrid Systems to Self-Powered/Catalyzed Devices, Energy Environ. Sci., 2024, 17(1), 49–113 RSC.
- L. Chen, Y. Liu, H. Cong, Q. Ge, W. Zhao, N. Jiang and Q. Zhang, Recent Progress and Perspective for Oxygen Evolution Reaction under Acidic Environments, Mater. Chem. Front., 2024, 8(4), 986–1014 RSC.
- H. Zhong, Q. Zhang, J. Yu, X. Zhang, C. Wu, Y. Ma, H. An, H. Wang, J. Zhang, X. Wang and J. Xue, Fundamental Understanding of Structural Reconstruction Behaviors in Oxygen Evolution Reaction Electrocatalysts, Adv. Energy Mater., 2023, 13(31), 2301391 CrossRef CAS.
- Z. Feng, C. Dai, P. Shi, X. Lei, R. Guo, B. Wang, X. Liu and J. You, Seven Mechanisms of Oxygen Evolution Reaction Proposed Recently: A Mini Review, Chem. Eng. J., 2024, 485, 149992 CrossRef CAS.
- E. Fabbri and T. J. Schmidt, Oxygen Evolution Reaction-The Enigma in Water Electrolysis, ACS Catal., 2018, 8(10), 9765–9774 CrossRef CAS.
- Z.-J. Chen, J. Dong, J. Wu, Q. Shao, N. Luo, M. Xu, Y. Sun, Y. Tang, J. Peng and H.-M. Cheng, Acidic Enol Electrooxidation-Coupled Hydrogen Production with Ampere-Level Current Density, Nat. Commun., 2023, 14(1), 4210 CrossRef CAS PubMed.
- Y. Guo, X. Yang, X. Liu, X. Tong and N. Yang, Coupling Methanol Oxidation with Hydrogen Evolution on Bifunctional Co-Doped Rh Electrocatalyst for Efficient Hydrogen Generation, Adv. Funct. Mater., 2023, 33(2), 2209134 CrossRef CAS.
- Y. Guo, Y. Tong, G. Zhou, J. He, X. Ren, L. Chen and P. Chen, Oxygen-Deficient NiCo2O4 Porous Nanowire for Superior Electrosynthesis of Ammonia Coupling with Valorization of Ethylene Glycol, Chem. Eng. J., 2024, 496, 154220 CrossRef CAS.
- J. He, Y. Tong, Z. Wang, G. Zhou, X. Ren, J. Zhu, N. Zhang, L. Chen and P. Chen, Oxygenate-Induced Structural Evolution of High-Entropy Electrocatalysts for Multifunctional Alcohol Electrooxidation Integrated with Hydrogen Production, Proc. Natl. Acad. Sci. U. S. A., 2024, 121(30), e2405846121 CrossRef PubMed.
- X. Lin, H. Zhong, W. Hu and J. Du, Nickel–Cobalt Selenide Electrocatalytic Electrode toward Glucose Oxidation Coupling with Alkaline Hydrogen Production, Inorg. Chem., 2023, 62(26), 10513–10521 CrossRef CAS.
- W.-J. Liu, Z. Xu, D. Zhao, X.-Q. Pan, H.-C. Li, X. Hu, Z.-Y. Fan, W.-K. Wang, G.-H. Zhao, S. Jin, G. W. Huber and H.-Q. Yu, Efficient Electrochemical Production of Glucaric Acid and H2 via Glucose Electrolysis, Nat. Commun., 2020, 11(1), 265 CrossRef PubMed.
- X. Ren and Y. Tong, The Self-Reconstruction of Co-Modified Bimetallic Hydroxysulfide Nanosheet Arrays for Efficient Hydrazine Assisted Water Splitting, Int. J. Hydrogen Energy, 2024, 49, 489–497 CrossRef CAS.
- T. Wang, Z. Huang, T. Liu, L. Tao, J. Tian, K. Gu, X. Wei, P. Zhou, L. Gan, S. Du, Y. Zou, R. Chen, Y. Li, X.-Z. Fu and S. Wang, Transforming Electrocatalytic Biomass Upgrading and Hydrogen Production from Electricity Input to Electricity Output, Angew. Chem., Int. Ed., 2022, 61(12), e202115636 CrossRef CAS PubMed.
- T. Wang, X. Cao and L. Jiao, Progress in Hydrogen Production Coupled with Electrochemical Oxidation of Small Molecules, Angew. Chem., Int. Ed., 2022, 61(51), e202213328 CrossRef.
- H. Sun, X. Xu, L. Fei, W. Zhou and Z. Shao, Electrochemical Oxidation of Small Molecules for Energy-Saving Hydrogen Production, Adv. Energy Mater., 2024, 2401242 CrossRef.
- R. Guo, Y. Zhang, X. Zhang, H. Yang and T. Hu, CoFe2O4 Nanosheets Grown in Situ on Nickel Nanowires for Efficient Hydrazine Oxidation-Boosted Hydrogen Production, Chem. Eng. J., 2024, 491, 152028 CrossRef.
- R.-Q. Li, S. Guo, X. Wang, X. Wan, S. Xie, Y. Liu, C. Wang, G. Zhang, J. Cao, J. Dai, M. Ge and W. Zhang, Dual-Strategy Engineered Nickel Phosphide for Achieving Efficient Hydrazine-Assisted Hydrogen Production in Seawater, Chem. Sci., 2024, 15, 10084–10091 RSC.
- X. Ren, C. Lin, G. Zhou, J. He, Y. Tong and P. Chen, Pt-Decorated Spinel MnCo2O4 Nanosheets Enable Ampere-Level Hydrazine Assisted Water Electrolysis, J. Colloid Interface Sci., 2024, 676, 13–21 CrossRef.
- Z. Feng, H. Meng, Y. Fu, L. Ren, B. Gao and W. Liu, Modulation of Charge Redistribution in Heterogeneous CoSe-Ni0.95Se Coupling with Ti3C2Tx MXene for Hydrazine-Assisted Water Splitting, Small, 2024, 2403270 CrossRef.
- M. Tasleem, V. Singh, A. Tiwari, V. Ganesan and M. Sankar, Electrocatalysis Using Cobalt Porphyrin Covalently Linked with Multi-Walled Carbon Nanotubes: Hydrazine Sensing and Hydrazine-Assisted Green Hydrogen Synthesis, Small, 2024, 2401273 CrossRef.
- Y. Li, Y. Jiao, H. Yan, C. Tian, A. Wu and H. Fu, Recent Progress in Synergistic Electrocatalysis for Generation of Valuable Products Based on Water Cycle, Nano Res., 2023, 16(5), 6444–6476 CrossRef.
- Y. Li, S. Niu, P. Liu, R. Pan, H. Zhang, N. Ahmad, Y. Shi, X. Liang, M. Cheng, S. Chen, J. Du, M. Hu, D. Wang, W. Chen and Y. Li, Ruthenium Nanoclusters and Single Atoms on α-MoC/N-Doped Carbon Achieves Low-Input/Input-Free Hydrogen Evolution via Decoupled/Coupled Hydrazine Oxidation, Angew. Chem., Int. Ed., 2024, 63(30), e202316755 CrossRef PubMed.
- H.-Y. Wang, S. Zhai, H. Wang, F. Yan, J.-T. Ren, L. Wang, M. Sun and Z.-Y. Yuan, Taking Advantage of Potential Coincidence Region: Insights into Gas Production Behavior in Advanced Self-Activated Hydrazine-Assisted Alkaline Seawater Electrolysis, ACS Nano, 2024, 18(30), 19682–19693 CAS.
-
T. Sato, US Pat., 5861366, 2020 Search PubMed.
- T. Take, K. Tsurutani and M. Umeda, Hydrogen Production by Methanol–Water Solution Electrolysis, J. Power Sources, 2007, 164(1), 9–16 CrossRef CAS.
- X. Yang, B. Ouyang, L. Zhao, Q. Shen, G. Chen, Y. Sun, C. Li and K. Xu, Ultrathin Rh Nanosheets with Rich Grain Boundaries for Efficient Hydrogen Oxidation Electrocatalysis, J. Am. Chem. Soc., 2023, 145(49), 27010–27021 CrossRef CAS PubMed.
- M.-G. Kim, H. J. Lee, T. K. Lee, E. Lee, H. Jin, J.-H. Park, S. Y. Cho, S. Lee, H. C. Ham and S. J. Yoo, Iridium Selenium Oxyhydroxide Shell for Polymer Electrolyte Membrane Water Electrolyzer with Low Ir Loading, ACS Energy Lett., 2024, 2876–2884 CrossRef CAS.
- Y. Li, X. Wei, R. Pan, Y. Wang, J. Luo, L. Li, L. Chen and J. Shi, PtAu Alloying-Modulated Hydroxyl and Substrate Adsorption for Glycerol Electrooxidation to C3 Products, Energy Environ. Sci., 2024, 17, 4205–4215 RSC.
- L.-Y. Jiang, F.-M. Tian, X.-Y. Chen, X.-X. Ren, J.-J. Feng, Y. Yao, L. Zhang and A.-J. Wang, Cu2+-Regulated One-Pot Wet-Chemical Synthesis of Uniform PdCu Nanostars for Electrocatalytic Oxidation of Ethylene Glycol and Glycerol, J. Colloid Interface Sci., 2023, 649, 118–124 CrossRef CAS PubMed.
- K. Deng, Z. Lian, W. Wang, J. Yu, H. Yu, Z. Wang, Y. Xu, L. Wang and H. Wang, Lattice Strain and Charge Redistribution of Pt Cluster/Ir Metallene Heterostructure for Ethylene Glycol to Glycolic Acid Conversion Coupled with Hydrogen Production, Small, 2024, 20(1), 2305000 CrossRef CAS PubMed.
- H. Lv, Y. Mao, H. Yao, H. Ma, C. Han, Y.-Y. Yang, Z.-A. Qiao and B. Liu, Ir-Doped CuPd Single-Crystalline Mesoporous Nanotetrahedrons for Ethylene Glycol Oxidation Electrocatalysis: Enhanced Selective Cleavage of C–C Bond, Angew. Chem., Int. Ed., 2024, 63(15), e202400281 CrossRef CAS PubMed.
- Y. Hu, J. Liu, W. Luo, J. Dong, C. Lee, N. Zhang, M. Chen, Y. Xu, D. Wu, M. Zhang, Q. Zhu, E. Hu, D. Geng, L. Zhong and Q. Yan, Alloying Pd with Ru Enables Electroreduction of Nitrate to Ammonia with ∼100% Faradaic Efficiency over a Wide Potential Window, Chem. Sci., 2024, 15(21), 8204–8215 RSC.
- K. Qin, H. Yu, W. Zhu, Y. Zhou, Z. Guo, Q. Shao, Y. Wu, X. Wang, Y. Li, Y. Ji, F. Liao, Y. Liu, Z. Kang and M. Shao, 1D Monoclinic IrxRu1−xO2 Solid Solution with Ru-Enhanced Electrocatalytic Activity for Acidic Oxygen Evolution Reaction, Adv. Funct. Mater., 2024, 2402226 CrossRef.
- J. Xu, X. Wang, X. Mao, K. Feng, J. Xu, J. Zhong, L. Wang, N. Han and Y. Li, Prominent Electronic Effect in Iridium-Alloy-Skinned Nickel Nanoparticles Boosts Alkaline Hydrogen Electrocatalysis, Energy Environ. Sci., 2023, 16(12), 6120–6126 RSC.
- D. A. Finkelstein, R. Imbeault, S. Garbarino, L. Roué and D. Guay, Trends in Catalysis and Catalyst Cost Effectiveness for N2H4 Fuel Cells and Sensors: A Rotating Disk Electrode (RDE) Study, J. Phys. Chem. C, 2016, 120(9), 4717–4738 CrossRef CAS.
- Y. Tong and P. Chen, Nanostructure Engineering of Ruthenium-Modified Electrocatalysts for Efficient Electrocatalytic Water Splitting, J. Mater. Chem. A, 2024, 12(7), 3844–3878 RSC.
- K. Chen, J. Xiang, Y. Guo, X. Liu, X. Li and K. Chu, Pd1Cu Single-Atom Alloys for High-Current-Density and Durable NO-to-NH3 Electroreduction, Nano Lett., 2024, 24(2), 541–548 CrossRef PubMed.
- H.-M. Yang, H.-Y. Wang, S. Zhai, J.-T. Ren and Z.-Y. Yuan, Aluminum-Induced Electron Structure on Nickel Phosphide For effective Hydrazine-assisted Water Splitting at Large Current Density, Chem. Eng. J., 2024, 489, 151236 CrossRef CAS.
- K. Li, Y. Tong, J. He, X.-Y. Liu and P. Chen, Anion-Modulated CoP Electrode as Bifunctional Electrocatalyst for Anion-Exchange Membrane Hydrazine-Assisted Water Electrolyser, Mater. Horiz., 2023, 10(11), 5277–5287 RSC.
- R. A. Senthil, S. Jung, A. Min, C. J. Moon and M. Y. Choi, Unveiling the Origin of Activity in RuCoOx-Anchored Nitrogen-Doped Carbon Electrocatalyst for High-Efficiency Hydrogen Production and Hydrazine Oxidation Using Raman Spectroscopy, Chem. Eng. J., 2023, 475, 146441 CrossRef.
- Y. Zhu, Y. Chen, Y. Feng, X. Meng, J. Xia and G. Zhang, Constructing Ru-O-TM Bridge in NiFe-LDH Enables High Current Hydrazine-Assisted H2 Production, Adv. Mater., 2024, 2401694 CrossRef PubMed.
- X. Cheng and Y. Tong, Hierarchical Cobalt–Nickel Phosphide/Molybdenum Disulfide Hybrid Electrocatalyst Triggering Efficient Hydrogen Generation in a Wider pH Range, ACS Appl. Energy Mater., 2023, 6(18), 9577–9584 CrossRef.
- K. Li, G. Zhou, Y. Tong, Y. Ye and P. Chen, Interface Engineering of a Hierarchical P-Modified Co/Ni3P Heterostructure for Highly Efficient Water Electrolysis Coupled with Hydrazine Degradation, ACS Sustainable Chem. Eng., 2023, 11(38), 14186–14196 CrossRef CAS.
- H. Hu, Z. Xu, Z. Zhang, X. Yan, X. Wang, Y. Zhu, J. Wang and M. Yang, Optimizing the Dehydrogenation Kinetics of Metal Nitrides for Energy-Efficient Seawater Hydrogen Production at 2 A cm−2, Adv. Funct. Mater., 2024, 2403863 CrossRef.
- K. Li, J. He, X. Guan, Y. Tong, Y. Ye, L. Chen and P. Chen, Phosphorus-Modified Amorphous High-Entropy CoFeNiCrMn Compound as High-Performance Electrocatalyst for Hydrazine-Assisted Water Electrolysis, Small, 2023, e2302130 CrossRef.
- S. Gopi and K. Yun, Synergistic Sulfur-Doped Tri-Metal Phosphide Electrocatalyst for Efficient Hydrazine Oxidation in Water Electrolysis: Toward High-Performance Hydrogen Fuel Generation, J. Alloys Compd., 2024, 986, 174044 CrossRef CAS.
- A. B. Ghosh, R. Banerjee, S. Roy, S. N. Bhaduri, D. K. Chanda, P. Biswas and A. Bandyopadhyay, Synthesis of Poly (3-bromo Thiophene) Supported Cobalt Molybdate Bifunctional Catalyst: Manifestation of Overall Water Splitting and Hydrazine Assisted Water Splitting, Electrochim. Acta, 2024, 475, 143521 CrossRef CAS.
- H. Liu, Y. Liu, M. Li, X. Liu and J. Luo, Transition-Metal-Based Electrocatalysts for Hydrazine-Assisted Hydrogen Production, Mater. Today Adv., 2020, 7, 100083 CrossRef CAS.
- Q. Jiang, S. Wang, C. Zhang, Z. Sheng, H. Zhang, R. Feng, Y. Ni, X. Tang, Y. Gu, X. Zhou, S. Lee, D. Zhang and F. Song, Active Oxygen Species Mediate the Iron-Promoting Electrocatalysis of Oxygen Evolution Reaction on Metal Oxyhydroxides, Nat. Commun., 2023, 14(1), 6826 CrossRef CAS PubMed.
- B. Zhang, L. Wang, Z. Cao, S. M. Kozlov, F. P. García de Arquer, C. T. Dinh, J. Li, Z. Wang, X. Zheng, L. Zhang, Y. Wen, O. Voznyy, R. Comin, P. De Luna, T. Regier, W. Bi, E. E. Alp, C.-W. Pao, L. Zheng, Y. Hu, Y. Ji, Y. Li, Y. Zhang, L. Cavallo, H. Peng and E. H. Sargent, High-Valence Metals Improve Oxygen Evolution Reaction Performance by Modulating 3d Metal Oxidation Cycle Energetics, Nat. Catal., 2020, 3(12), 985–992 CrossRef CAS.
- H. Wang, T. Zhai, Y. Wu, T. Zhou, B. Zhou, C. Shang and Z. Guo, High-Valence Oxides for High Performance Oxygen Evolution Electrocatalysis, Adv. Sci., 2023, 10(22), 2301706 CrossRef CAS.
- Y. Li, X. Yu, J. Gao and Y. Ma, Hierarchical Ni2P/Zn–Ni–P Nanosheet Array for Efficient Energy-Saving Hydrogen Evolution and Hydrazine Oxidation, J. Mater. Chem. A, 2023, 11(5), 2191–2202 RSC.
- S. Zhao, Y. Sun, H. Li, S. Zeng, Q. Yao, R. Li, H. Chen and K. Qu, Highly Bifunctional Rh2P on N,P-Codoped Carbon for Hydrazine Oxidation Assisted Energy-Saving Hydrogen Production, Chem. Commun., 2024, 60(46), 5928–5931 RSC.
- Q. Quan, X. Li, C. Song, Q. Jia, H. Lu, X. Cui, G. Liu, X. Chen and L. Jiang, Polyoxometalate-Derived Bi-Functional Crystalline/Amorphous Interfaces with Optimized d-Electron Configuration for Efficient Self-Powered Hydrazine-Seawater Splitting, Chem. Eng. J., 2024, 488, 150897 CrossRef CAS.
- M. Zhang, B. Zhou, Y. Gong, M. Shang, W. Xiao, J. Wang, C. Dai, H. Zhang, Z. Wu and L. Wang, Regulating Mo-Based Alloy-Oxide Active Interfaces for Efficient Alkaline Hydrogen Evolution Assisted by Hydrazine Oxidation, J. Colloid Interface Sci., 2024, 667, 73–81 CrossRef CAS.
- N. Mahmood, Y. Yao, J.-W. Zhang, L. Pan, X. Zhang and J.-J. Zou, Electrocatalysts for Hydrogen Evolution in Alkaline Electrolytes: Mechanisms, Challenges, and Prospective Solutions, Adv. Sci., 2018, 5(2), 1700464 CrossRef.
- F. Bao, E. Kemppainen, I. Dorbandt, R. Bors, F. Xi, R. Schlatmann, R. van de Krol and S. Calnan, Understanding the Hydrogen Evolution Reaction Kinetics of Electrodeposited Nickel-Molybdenum in Acidic, Near-Neutral, and Alkaline Conditions, ChemElectroChem, 2021, 8(1), 195–208 CrossRef CAS.
- F. Cheng, X. Peng, L. Hu, B. Yang, Z. Li, C.-L. Dong, J.-L. Chen, L.-C. Hsu, L. Lei, Q. Zheng, M. Qiu, L. Dai and Y. Hou, Accelerated Water Activation and Stabilized Metal-Organic Framework via Constructing Triangular Active-Regions for Ampere-Level Current Density Hydrogen Production, Nat. Commun., 2022, 13(1), 6486 CrossRef CAS PubMed.
- G. E. Evans and K. V. Kordesch, Hydrazine-Air Fuel Cells, Science, 1967, 158(3805), 1148–1152 CrossRef CAS.
- T. Y. Burshtein, Y. Yasman, L. Muñoz-Moene, J. H. Zagal and D. Eisenberg, Hydrazine Oxidation Electrocatalysis, ACS Catal., 2024, 14(4), 2264–2283 CrossRef CAS.
- T. Y. Burshtein, K. Tamakuwala, M. Sananis, I. Grinberg, N. R. Samala and D. Eisenberg, Understanding Hydrazine Oxidation Electrocatalysis on Undoped Carbon, Phys. Chem. Chem. Phys., 2022, 24(17), 9897–9903 RSC.
- A. Badreldin, E. Youssef, A. Djire, A. Abdala and A. Abdel-Wahab, A Critical Look at Alternative Oxidation Reactions for Hydrogen Production from Water Electrolysis, Cell Rep. Phys. Sci., 2023, 4(6), 101427 CrossRef CAS.
- Y. Huang, X. Zhang, L. Li, M. Humayun, H. Zhang, X. Xu, S. P. Anthony, Z. Chen, J. Zeng, D. V. Shtansky, K. Huo, H. Song, C. Wang and W. Zhang, Mott–Schottky Barrier Enabling High-Performance Hydrazine-Assisted Hydrogen Generation at Ampere-Level Current Densities, Adv. Funct. Mater., 2024, 2401011 CrossRef.
- J. Ge, P. Yin, Y. Chen, H. Cheng, J. Liu, B. Chen, C. Tan, P.-F. Yin, H.-X. Zheng, Q.-Q. Li, S. Chen, W. Xu, X. Wang, G. Wu, R. Sun, X.-H. Shan, X. Hong and H. Zhang, Ultrathin Amorphous/Crystalline Heterophase Rh and Rh Alloy Nanosheets as Tandem Catalysts for Direct Indole Synthesis, Adv. Mater., 2021, 33(9), 2006711 CrossRef CAS.
- Y. Gao, Y. Xue, L. Qi, C. Xing, X. Zheng, F. He and Y. Li, Rhodium Nanocrystals on Porous Graphdiyne for Electrocatalytic Hydrogen Evolution from Saline Water, Nat. Commun., 2022, 13(1), 5227 CrossRef CAS.
- Y. Gao, Y. Xue, F. He and Y. Li, Controlled Growth of a High Selectivity Interface for Seawater Electrolysis, Proc. Natl. Acad. Sci. U. S. A., 2022, 119(36), e2206946119 CrossRef CAS PubMed.
- J. Shi, Q. Sun, W. Zhu, T. Cheng, F. Liao, M. Ma, J. Yang, H. Yang, Z. Fan and M. Shao, Lattice Stain Dominated Hydrazine Oxidation Reaction in Single-Metal-Element Nanosheet, Chem. Eng. J., 2023, 463, 142385 CrossRef.
- X. Huang, Y. Wang, Q. Zhu, K. Zhou, H. Zhi and J. Yang, High Quality Synthesis of Rh Nanocubes and Their Application in Hydrazine Hydrate Oxidation Assisted Water Splitting, Inorg. Chem. Commun., 2021, 134, 109023 CrossRef.
- Z. W. Chen, J. Li, P. Ou, J. E. Huang, Z. Wen, L. Chen, X. Yao, G. Cai, C. C. Yang, C. V. Singh and Q. Jiang, Unusual Sabatier Principle on High Entropy Alloy Catalysts for Hydrogen Evolution Reactions, Nat. Commun., 2024, 15(1), 359 CrossRef PubMed.
- Z. Wang, X. Zhang, W. Tian, H. Yu, K. Deng, Y. Xu, X. Wang, H. Wang and L. Wang, Nitrogen-Doped Ru Film for Energy-Saving Hydrogen Production Assisted with Hydrazine Oxidation, Chem. Commun., 2022, 58(74), 10424–10427 RSC.
- Z. Duan, T. Ren, Y. Xu, Z. Wang, H. Yu, K. Deng, L. Wang and H. Wang, Boron-Doped Iridium Nanosheets Array for Energy-Saving Hydrogen Production by Hydrazine-Assisted Water Electrolysis, Int. J. Hydrogen Energy, 2023, 48(95), 37045–37052 CrossRef CAS.
- X. Zhang, S. Wang, C. Wu, H. Li, Y. Cao, S. Li and H. Xia, Synthesis of S-Doped AuPbPt Alloy Nanowire-Networks as Superior Catalysts towards the ORR and HER, J. Mater. Chem. A, 2020, 8(45), 23906–23918 RSC.
- P. Shen, B. Zhou, Z. Chen, W. Xiao, Y. Fu, J. Wan, Z. Wu and L. Wang, Ruthenium-Doped 3D Cu2O Nanochains as Efficient Electrocatalyst towards Hydrogen Evolution and Hydrazine Oxidation, Appl. Catal., B, 2023, 325, 122305 CrossRef CAS.
- H. Chen, J. Yu, L. Liu, R.-T. Gao, Z. Gao, Y. Yang, Z. Chen, S. Zhan, X. Liu, X. Zhang, H. Dong, L. Wu and L. Wang, Modulating Pt-N/O Bonds on Co-Doped WO3 for Acid Electrocatalytic Hydrogen Evolution with Over 2000 h Operation, Adv. Energy Mater., 2024, 14(19), 2303635 CrossRef CAS.
- W. Zhong, Z. Wang, N. Gao, L. Huang, Z. Lin, Y. Liu, F. Meng, J. Deng, S. Jin, Q. Zhang and L. Gu, Coupled Vacancy Pairs in Ni-Doped CoSe for Improved Electrocatalytic Hydrogen Production Through Topochemical Deintercalation, Angew. Chem., Int. Ed., 2020, 59(50), 22743–22748 CrossRef CAS.
- J. Wang, Y. Wang, C. Cai, Y. Liu, D. Wu, M. Wang, M. Li, X. Wei, M. Shao and M. Gu, Cu-Doped Iron Oxide for the Efficient Electrocatalytic Nitrate Reduction Reaction, Nano Lett., 2023, 23(5), 1897–1903 CrossRef.
- T. Zhao, S. Wang, Y. Li, C. Jia, Z. Su, D. Hao, B. Ni, Q. Zhang and C. Zhao, Heterostructured V-Doped Ni2P/Ni12P5 Electrocatalysts for Hydrogen Evolution in Anion Exchange Membrane Water Electrolyzers, Small, 2022, 18(40), 2204758 CrossRef.
- Z. Chen, L. Wang, H. Li, S. Zeng, R. Li, H. Chen, Y. Zheng, Q. Yao and K. Qu, Highly Enhanced Bifunctionality by Trace Co Doping into Ru Matrix towards Hydrazine Oxidation-Assisted Energy-Saving Hydrogen Production, Fuel, 2024, 360, 130602 CrossRef.
- T. Cui, J. Chi, J. Zhu, X. Sun, J. Lai, Z. Li and L. Wang, Tuning the Size and Chemisorption of FeP4 by Trace Ru Doping for Hydrazine-Assisted Hydrogen Evolution in Seawater at Large-current-density, Appl. Catal., B, 2022, 319, 121950 CrossRef.
- L. Hui, Y. Xue, C. Xing, Y. Liu, Y. Du, Y. Fang, H. Yu, C. Zhang, F. He and Y. Li, Atomic Alloys of Nickel-Platinum on Carbon Network for Methanol Oxidation, Nano Energy, 2022, 95, 106984 CrossRef CAS.
- J. Wei, K. Xiao, Y. Chen, X.-P. Guo, B. Huang and Z.-Q. Liu, In Situ Precise Anchoring of Pt Single Atoms in Spinel Mn3O4 for a Highly Efficient Hydrogen Evolution Reaction, Energy Environ. Sci., 2022, 15(11), 4592–4600 RSC.
- H. Yu, W. Wang, Q. Mao, K. Deng, Z. Wang, Y. Xu, X. Li, H. Wang and L. Wang, Pt Single Atom Captured by Oxygen Vacancy-Rich NiCo Layered Double Hydroxides for Coupling Hydrogen Evolution with Selective Oxidation of Glycerol to Formate, Appl. Catal., B, 2023, 330, 122617 CrossRef CAS.
- Z. Yu, C. Si, F. Sabaté, A. P. LaGrow, Z. Tai, V. M. Diaconescu, L. Simonelli, L. Meng, M. J. Sabater, B. Li and L. Liu, Defective Ru-Doped α-MnO2 Nanorods Enabling Efficient Hydrazine Oxidation for Energy-Saving Hydrogen Production via Proton Exchange Membranes at near-Neutral pH, Chem. Eng. J., 2023, 470, 144050 CrossRef CAS.
- X. Xu, L. Su, X. Yu, J. Sun and X. Miao, Spin Configuration Modulation of Co3O4 by Ru Doping for Boosting the Overall Water Splitting and Hydrazine Oxidation Reactions, Inorg. Chem. Front., 2024, 11(5), 1381–1393 RSC.
- Z. Liu, Y. Li, H. Guo, J. Zhao, H. Zhang and R. Song, Interface Engineering of Amorphous/Crystalline Heterojunctions with Synergistic Ru Doping for Efficient Hydrazine Oxidation Assisted Overall Water Splitting, J. Mater. Chem. A, 2023, 11(45), 24667–24677 RSC.
- X. Zhai, Q. Yu, G. Liu, J. Bi, Y. Zhang, J. Chi, J. Lai, B. Yang and L. Wang, Hierarchical Microsphere MOF Arrays with Ultralow Ir Doping for Efficient Hydrogen Evolution Coupled with Hydrazine Oxidation in Seawater, J. Mater. Chem. A, 2021, 9(48), 27424–27433 RSC.
- Q. Zhang, K. Lian, Q. Liu, G. Qi, S. Zhang, J. Luo and X. Liu, High Entropy Alloy Nanoparticles as Efficient Catalysts for Alkaline Overall Seawater Splitting and Zn-Air Batteries, J. Colloid Interface Sci., 2023, 646, 844–854 CrossRef.
- M. Wang, H. Yang, J. Shi, Y. Chen, Y. Zhou, L. Wang, S. Di, X. Zhao, J. Zhong, T. Cheng, W. Zhou and Y. Li, Alloying Nickel with Molybdenum Significantly Accelerates Alkaline Hydrogen Electrocatalysis, Angew. Chem., Int. Ed., 2021, 60(11), 5771–5777 CrossRef.
- Y. Nakaya and S. Furukawa, Catalysis of Alloys: Classification, Principles, and Design for a Variety of Materials and Reactions, Chem. Rev., 2023, 123(9), 5859–5947 CrossRef CAS PubMed.
- Z.-P. Wu, D. T. Caracciolo, Y. Maswadeh, J. Wen, Z. Kong, S. Shan, J. A. Vargas, S. Yan, E. Hopkins, K. Park, A. Sharma, Y. Ren, V. Petkov, L. Wang and C.-J. Zhong, Alloying–Realloying Enabled High Durability for Pt–Pd-3d-Transition Metal Nanoparticle Fuel Cell Catalysts, Nat. Commun., 2021, 12(1), 859 CrossRef CAS.
- Q. Mao, W. Wang, K. Deng, H. Yu, Z. Wang, Y. Xu, X. Li, L. Wang and H. Wang, Low-Content Pt-Triggered the Optimized d-Band Center of Rh Metallene for Energy-Saving Hydrogen Production Coupled with Hydrazine Degradation, J. Energy Chem., 2023, 85, 58–66 CrossRef CAS.
- Y. Yu, S. J. Lee, J. Theerthagiri, Y. Lee and M. Y. Choi, Architecting the AuPt Alloys for Hydrazine Oxidation as an Anolyte in Fuel Cell: Comparative Analysis of Hydrazine Splitting and Water Splitting for Energy-Saving H2 Generation, Appl. Catal., B, 2022, 316, 121603 CrossRef CAS.
- S. Zhao, Y. Zhang, H. Li, S. Zeng, R. Li, Q. Yao, H. Chen, Y. Zheng and K. Qu, Regulating Ru Active Sites by Pd Alloying to Significantly Enhance Hydrazine Oxidation for Energy-Saving Hydrogen Production, J. Mater. Chem. A, 2023, 11(25), 13783–13792 RSC.
- M. Zhang, Z. Wang, Z. Duan, S. Wang, Y. Xu, X. Li, L. Wang and H. Wang, Anodic Hydrazine Oxidation Assisted Hydrogen Evolution over Bimetallic RhIr Mesoporous Nanospheres, J. Mater. Chem. A, 2021, 9(34), 18323–18328 RSC.
- W. Zhu, A. Gandi Naidu, Q. Wu, H. Yan, M. Zhao, Z. Wang and H. Liang, Simultaneous Electrocatalytic Hydrogen Production and Hydrazine Removal from Acidic Waste Water, Chem. Eng. Sci., 2022, 258, 117769 CrossRef.
- Y.-C. Shi, T. Yuan, J.-J. Feng, J. Yuan and A.-J. Wang, Rapid Fabrication of Support-Free Trimetallic Pt53Ru39Ni8 Nanosponges with Enhanced Electrocatalytic Activity for Hydrogen Evolution and Hydrazine Oxidation Reactions, J. Colloid Interface Sci., 2017, 505, 14–22 CrossRef.
- T. Yuan, A. Wang, K. Fang, Z. Wang and J. Feng, Hydrogen Evolution-Assisted One-Pot Aqueous Synthesis of Hierarchical Trimetallic PdNiRu Nanochains for Hydrazine Oxidation Reaction, J. Energy Chem., 2017, 26(6), 1231–1237 CrossRef.
- S. Ge, L. Zhang, J. Hou, S. Liu, Y. Qin, Q. Liu, X. Cai, Z. Sun, M. Yang, J. Luo and X. Liu, Cu2O-Derived PtCu Nanoalloy toward Energy-Efficient Hydrogen Production via Hydrazine Electrolysis under Large Current Density, ACS Appl. Energy Mater., 2022, 5(8), 9487–9494 CrossRef.
- X. Liu, T. Wang, Y. Chen, J. Wang, W. Xie, R. Wu, X. Xu, L. Pang, X. Zhang, Y. Lv, G. Wang, Y. Yamauchi and T. Jin, Distinctive P-d Orbital Hybridization in RuSb Nanobranches for Simultaneously Enhanced Hydrogen Evolution and Hydrazine Oxidation in Alkaline Seawater, Appl. Catal., B, 2023, 333, 122771 CrossRef CAS.
- W. Tian, X. Zhang, Z. Wang, L. Cui, M. Li, Y. Xu, X. Li, L. Wang and H. Wang, Amorphization Activated RhPb Nanflowers for Energy-Saving Hydrogen Production by Hydrazine-Assisted Water Electrolysis, Chem. Eng. J., 2022, 440, 135848 CrossRef CAS.
- Y. Ao, S. Chen, C. Wang and X. Lu, Palladium Cobalt Alloy Encapsulated in Carbon Nanofibers as Bifunctional Electrocatalyst for High-Efficiency Overall Hydrazine Splitting, J. Colloid Interface Sci., 2021, 601, 495–504 CrossRef CAS.
- Y. Jeong, S. Shankar Naik, Y. Yu, J. Theerthagiri, S. J. Lee, P. L. Show, H. C. Choi and M. Y. Choi, Ligand-Free Monophasic CuPd Alloys Endow Boosted Reaction Kinetics toward Energy-Efficient Hydrogen Fuel Production Paired with Hydrazine Oxidation, J. Mater.
Sci. Technol., 2023, 143, 20–29 CrossRef CAS.
- G. Feng, Y. Pan, D. Su and D. Xia, Constructing Fully-Active and Ultra-Active Sites in High-Entropy Alloy Nanoclusters for Hydrazine Oxidation-Assisted Electrolytic Hydrogen Production, Adv. Mater., 2024, 36(13), 2309715 CrossRef CAS.
- H. Liu, H. Qin, J. Kang, L. Ma, G. Chen, Q. Huang, Z. Zhang, E. Liu, H. Lu, J. Li and N. Zhao, A Freestanding Nanoporous NiCoFeMoMn High-Entropy Alloy as an Efficient Electrocatalyst for Rapid Water Splitting, Chem. Eng. J., 2022, 435, 134898 CrossRef.
- H. Kamaruddin, Z. Jianghong, L. Yu, W. Yuefan and H. Yizhong, A Review of Noble Metal-Free High Entropy Alloys for Water Splitting Applications, J. Mater. Chem. A, 2024, 12(17), 9933–9961 RSC.
- D. Zhang, Y. Shi, X. Chen, J. Lai, B. Huang and L. Wang, High-Entropy Alloy Metallene for Highly Efficient Overall Water Splitting in Acidic Media, Chin. J. Catal., 2023, 45, 174–183 CrossRef.
- S.-Q. Chang, C.-C. Cheng, P.-Y. Cheng, C.-L. Huang and S.-Y. Lu, Pulse Electrodeposited FeCoNiMnW High Entropy Alloys as Efficient and Stable Bifunctional Electrocatalysts for Acidic Water Splitting, Chem. Eng. J., 2022, 446, 137452 CrossRef.
- B. Wang, W. Liu, Y. Leng, X. Yu, C. Wang, L. Hu, X. Zhu, C. Wu, Y. Yao and Z. Zou, Strain Engineering of High-Entropy Alloy Catalysts for Electrocatalytic Water Splitting, iScience, 2023, 26(4), 106326 CrossRef CAS.
- J. Yang, L. Xu, W. Zhu, M. Xie, F. Liao, T. Cheng, Z. Kang and M. Shao, Rh/RhOx Nanosheets as pH-Universal Bifunctional Catalysts for Hydrazine Oxidation and Hydrogen Evolution Reactions, J. Mater. Chem. A, 2022, 10(4), 1891–1898 RSC.
- X. Fu, D. Cheng, A. Zhang, J. Zhou, S. Wang, X. Zhao, J. Chen, P. Sautet, Y. Huang and X. Duan, Ag–Ru Interface for Highly Efficient Hydrazine Assisted Water Electrolysis, Energy Environ. Sci., 2024, 17(6), 2279–2286 RSC.
- L. Cheng, Y. Li, J. Fan, M. Xie, X. Liu, P. Sun and X. Dong, High Efficiency Photothermal Synergistic Degradation of Toluene Achieved through the Utilization of a Nickel Foam Loaded Pt-CeO2 Monolithic Catalyst, Sep. Purif. Technol., 2024, 333, 125742 CrossRef CAS.
- J. Huang, Z. Zhuang, Y. Zhao, J. Chen, Z. Zhuo, Y. Liu, N. Lu, H. Li and T. Zhai, Back-Gated van Der Waals Heterojunction Manipulates Local Charges toward Fine-Tuning Hydrogen Evolution, Angew. Chem., Int. Ed., 2022, 61(32), e202203522 CrossRef CAS.
- D. Yang, T. Lv, J. Song, J. Chen, L. Hao, Q. Tian and L. Cui, Enabling Stable High Lithium Storage of Si Anode via Synergistic Effects of Nanosized Fe3C and Partially Graphitized Porous Carbon, Chem. Eng. J., 2024, 496, 153844 CrossRef.
- K. Zhang, Y. Han, J. Qiu, X. Ding, Y. Deng, Y. Wu, G. Zhang and L. Yan, Interface Engineering of Ni/NiO Heterostructures with Abundant Catalytic Active Sites for Enhanced Methanol Oxidation Electrocatalysis, J. Colloid Interface Sci., 2023, 630, 570–579 CrossRef PubMed.
- Y. Chen, J. Song, Y. Li, Q. Tian, J. Chen and L. Yang, High Lithium Storage Performance of CoO with a Distinctive Dual-Carbon-Confined Nanoarchitecture, Nanoscale, 2021, 13(30), 12938–12950 RSC.
- J. Qin, N. Zhao, C. Shi, E. Liu, F. He, L. Ma, Q. Li, J. Li and C. He, Sandwiched C@SnO2@C Hollow Nanostructures as an Ultralong-Lifespan High-Rate Anode Material for Lithium-Ion and Sodium-Ion Batteries, J. Mater. Chem. A, 2017, 5(22), 10946–10956 RSC.
- D. Qi, S. Liu, H. Chen, S. Lai, Y. Qin, Y. Qiu, S. Dai, S. Zhang, J. Luo and X. Liu, Rh Nanoparticle Functionalized Heteroatom-Doped Hollow Carbon Spheres for Efficient Electrocatalytic Hydrogen Evolution, Mater. Chem. Front., 2021, 5(7), 3125–3131 RSC.
- X. Guan, Y. Sun, S. Zhao, H. Li, S. Zeng, Q. Yao, R. Li, H. Chen and K. Qu, Selectively Nucleotide-Derived RuP on N, P-Codoped Carbon with Engineered Mesopores for Energy-Efficient Hydrogen Production Assisted by Hydrazine Oxidation, SusMat, 2024, 4(1), 166–177 CrossRef CAS.
- X. Liu, W. Sun, X. Hu, J. Chen and Z. Wen, Self-Powered H2 Generation Implemented by Hydrazine Oxidation Assisting Hybrid Electrochemical Cell, Chem. Eng. J., 2023, 474, 145355 CrossRef CAS.
- Y. Li, W. Wang, M. Cheng, Q. Qian, Y. Zhu and G. Zhang, Environmentally Benign General Synthesis of Nonconsecutive Carbon-Coated RuP2 Porous Microsheets as Efficient Bifunctional Electrocatalysts under Neutral Conditions for Energy-Saving H2 Production in Hybrid Water Electrolysis, Catal. Sci. Technol., 2022, 12(13), 4339–4349 RSC.
- H.-L. Huang, X. Guan, H. Li, R. Li, R. Li, S. Zeng, S. Tao, Q. Yao, H. Chen and K. Qu, Ir Nanoclusters/Porous N-Doped Carbon as a Bifunctional Electrocatalyst for Hydrogen Evolution and Hydrazine Oxidation Reactions, Chem. Commun., 2022, 58(14), 2347–2350 RSC.
- C. Zhang, H. Liu, Y. Liu, X. Liu, Y. Mi, R. Guo, J. Sun, H. Bao, J. He, Y. Qiu, J. Ren, X. Yang, J. Luo and G. Hu, Rh2S3/N-Doped Carbon Hybrids as pH-Universal Bifunctional Electrocatalysts for Energy-Saving Hydrogen Evolution, Small Methods, 2020, 4(9), 2000208 CrossRef.
- W. Wang, Q. Qian, Y. Li, Y. Zhu, Y. Feng, M. Cheng, H. Zhang, Y. Zhang and G. Zhang, Robust and Highly Efficient Electrochemical Hydrogen Production from Hydrazine-Assisted Water Electrolysis Enabled by the Metal–Support Interaction of Ru/C Composites, ACS Appl. Mater. Interfaces, 2023, 15(22), 26852–26862 CrossRef PubMed.
- S. S. Narwade, B. B. Mulik, S. M. Mali and B. R. Sathe, Silver Nanoparticles Sensitized C60(Ag@C60) as Efficient Electrocatalysts for Hydrazine Oxidation: Implication for Hydrogen Generation Reaction, Appl. Surf. Sci., 2017, 396, 939–944 CrossRef.
- Y. Ji, J. Song, Y. Li, X. Lu, Q. Tian, L. Yang and J. Chen, High Lithium Storage of Co3O4 Enabled by Integrating Hollow and Porous Carbon Scaffolds, Ceram. Int., 2022, 48(11), 15252–15260 CrossRef CAS.
- Y. Li, J. Song, X. Hong, Q. Tian, Z. Sui and L. Yang, Boosting the Lithium Storage Performance of Tin Dioxide by Carbon Nanotubes Supporting and Surface Engineering, J. Colloid Interface Sci., 2021, 602, 789–798 CrossRef CAS PubMed.
- J. Song, S. Ke, P. Sun, D. Yang, C. Luo, Q. Tian, C. Liang and J. Chen, High-Performance Si@C Anode for Lithium-Ion Batteries Enabled by a Novel Structuring Strategy, Nanoscale, 2023, 15(33), 13790–13808 RSC.
- Y. Li, Y. Zhang, K. Qian and W. Huang, Metal–Support Interactions in Metal/Oxide Catalysts and Oxide–Metal Interactions in Oxide/Metal Inverse Catalysts, ACS Catal., 2022, 12(2), 1268–1287 CrossRef.
- T. Kamada, T. Ueda, S. Fukuura, T. Yumura, S. Hosokawa, T. Tanaka, D. Kan and Y. Shimakawa, Ultralong Distance Hydrogen Spillover Enabled by Valence Changes in a Metal Oxide Surface, J. Am. Chem. Soc., 2023, 145(3), 1631–1637 CrossRef PubMed.
- X. Liu, G. Chen, Y. Guo, T. Li, J. Huang, W. Chen and K. Ostrikov, Fabric-like Rhodium-Nickel-Tungsten Oxide Nanosheets for Highly-Efficient Electrocatalytic H2 Generation in an Alkaline Electrolyte, J. Colloid Interface Sci., 2024, 659, 895–904 CrossRef PubMed.
- Z. Wang, Y. Wang, H. Zhang, H. Yu, K. Deng, Y. Xu, H. Wang and L. Wang, Au–Rh2P Mesoporous Nanotubes for the Hydrogen Evolution Reaction and Hydrazine Oxidation Electrooxidation, ACS Appl. Nano Mater., 2023, 6(21), 20183–20189 CrossRef.
- Z. Wang, G. Yang, P. Tian, K. Deng, H. Yu, Y. Xu, X. Li, H. Wang and L. Wang, Heterointerface Engineering of Rh/Pd Metallene for Hydrazine Oxidation-Assisted Energy-Saving Hydrogen Production, J. Mater. Chem. A, 2023, 11(19), 10222–10227 RSC.
- Y. Wang, H. Su, Y. He, L. Li, S. Zhu, H. Shen, P. Xie, X. Fu, G. Zhou, C. Feng, D. Zhao, F. Xiao, X. Zhu, Y. Zeng, M. Shao, S. Chen, G. Wu, J. Zeng and C. Wang, Advanced Electrocatalysts with Single-Metal-Atom Active Sites, Chem. Rev., 2020, 120(21), 12217–12314 CrossRef CAS PubMed.
- J. Su, L. Zhuang, S. Zhang, Q. Liu, L. Zhang and G. Hu, Single Atom Catalyst for Electrocatalysis, Chin. Chem. Lett., 2021, 32(10), 2947–2962 CrossRef CAS.
- Q. Zhang and J. Guan, Single-Atom Catalysts for Electrocatalytic Applications, Adv. Funct. Mater., 2020, 30(31), 2000768 CrossRef CAS.
- F. Luo, S. Pan, Y. Xie, C. Li, Y. Yu, H. Bao and Z. Yang, Hydrazine-Assisted Acidic Water Splitting Driven by Iridium Single Atoms, Adv. Sci., 2023, 10(32), 2305058 CrossRef CAS.
- X. Guan, Q. Wu, H. Li, S. Zeng, Q. Yao, R. Li, H. Chen, Y. Zheng and K. Qu, Identifying the Roles of Ru Single Atoms and Nanoclusters for Energy-Efficient Hydrogen Production Assisted by Electrocatalytic Hydrazine Oxidation, Appl. Catal., B, 2023, 323, 122145 CrossRef.
- Y. Zhai, C. Jin, Q. Xia, W. Han, J. Wu, X. Zhao and X. Zhang, Atomically Confined Ru Sites in Octahedral Co3O4 for High-Efficiency Hydrazine Oxidation, Adv. Funct. Mater., 2024, 34(13), 2311063 CrossRef.
- J. Li, C. Zhang, C. Zhang, H. Ma, Y. Yang, Z. Guo, Y. Wang and H. Ma, Electronic Configuration of Single Ruthenium Atom Immobilized in Urchin-like Tungsten Trioxide towards Hydrazine Oxidation-Assisted Hydrogen Evolution under Wide pH Media, Chem. Eng. J., 2022, 430, 132953 CrossRef.
- X. Peng, Y. Mi, X. Liu, J. Sun, Y. Qiu, S. Zhang, X. Ke, X. Wang and J. Luo, Self-Driven Dual Hydrogen Production System Based on a Bifunctional Single-Atomic Rh Catalyst, J. Mater. Chem. A, 2022, 10(11), 6134–6145 RSC.
- S. Zhou, Y. Zhao, R. Shi, Y. Wang, A. Ashok, F. Héraly, T. Zhang and J. Yuan, Vacancy-Rich MXene-Immobilized Ni Single Atoms as a High-Performance Electrocatalyst for the Hydrazine Oxidation Reaction, Adv. Mater., 2022, 34(36), 2204388 CrossRef CAS PubMed.
- H. Wang, Y. Tong and P. Chen, Microenvironment Regulation Strategies of Single-Atom Catalysts for Advanced Electrocatalytic CO2 Reduction to CO, Nano Energy, 2023, 118, 108967 CrossRef CAS.
- H. Wang, J. Zhu, X. Ren, Y. Tong and P. Chen, Heterogeneous Cobalt Phthalocyanine/Sulfur-Modified Hollow Carbon Sphere for Boosting CO2 Electroreduction and Zn–CO2 Batteries, Adv. Funct. Mater., 2024, 34(14), 2312552 CrossRef CAS.
- X. Peng, J. Hou, Y. Mi, J. Sun, G. Qi, Y. Qin, S. Zhang, Y. Qiu, J. Luo and X. Liu, Bifunctional Single-Atomic Mn Sites for Energy-Efficient Hydrogen Production, Nanoscale, 2021, 13(9), 4767–4773 RSC.
- Q. Sun, L. Wang, Y. Shen, M. Zhou, Y. Ma, Z. Wang and C. Zhao, Bifunctional Copper-Doped Nickel Catalysts Enable Energy-Efficient Hydrogen Production via Hydrazine Oxidation and Hydrogen Evolution Reduction, ACS Sustainable Chem. Eng., 2018, 6(10), 12746–12754 CrossRef.
- M. Abdolmaleki, A. Bodaghi, J. Hosseini and S. Jamehbozorgi, Preparation of Nanostructured Co–Mo Alloy Electrodes and Investigation of Their Electrocatalytic Activity for Hydrazine Oxidation in Alkaline Medium, J. Chin. Chem. Soc., 2018, 65(8), 970–976 CrossRef.
- Q. Sun, Y. Li, J. Wang, B. Cao, Y. Yu, C. Zhou, G. Zhang, Z. Wang and C. Zhao, Pulsed Electrodeposition of Well-Ordered Nanoporous Cu-Doped Ni Arrays Promotes High-Efficiency Overall Hydrazine Splitting, J. Mater. Chem. A, 2020, 8(40), 21084–21093 RSC.
- Y. Wang, T. Xie, Q. Zhu, J. Fu, Y. Peng, J. Wang and S. Liu, Three-Dimensional Nanoporous Cu-Doped Ni Coating as Bifunctional Electrocatalyst for Hydrazine Sensing and Hydrogen Evolution Reaction, Nanotechnology, 2021, 32(30), 305502 CrossRef.
- P. Sun, L. Cheng, S. Chen, M. Xie, F. Dong and X. Dong, Nickel Foam Based Monolithic Catalyst Supporting Transition Metal Oxides for Toluene Combustion: Experimental and Theoretical Study of Interfacial Synergistic Oxidation and Water Resistance, Chem. Eng. J., 2024, 483, 149176 CrossRef CAS.
- Y. Sun, X. Li, T. Zhang, K. Xu, Y. Yang, G. Chen, C. Li and Y. Xie, Nitrogen-Doped Cobalt Diselenide with Cubic Phase Maintained for Enhanced Alkaline Hydrogen Evolution, Angew. Chem., Int. Ed., 2021, 60(39), 21575–21582 CrossRef CAS.
- W. Wu, Y. Tong, Y. Ye, G. Zhou, J. He, J. Zhu, X. Ren, N. Zhang, H. Wang and P. Chen, In-Situ Electrochemical Transformation of F-Modified Metallic Bismuth for Highly-Efficient CO2 Electroreduction and Zn-CO2 Battery, Chem. Eng. J., 2024, 494, 153105 CrossRef CAS.
- S. Zhu, R. Yang, H. J. W. Li, S. Huang, H. Wang, Y. Liu, H. Li and T. Zhai, Reconstructing Hydrogen-Bond Network for Efficient Acidic Oxygen Evolution, Angew. Chem., Int. Ed., 2024, 63(17), e202319462 CrossRef CAS PubMed.
- W. Jia, X. Wang, Z. Lu, Y. Wu, R. Sheng and J. Lv, Hydrazine Hydrate-Assisted Adjustment of Sulfur-Rich MoS2 as Hydrogen Evolution Electrocatalyst, J. Alloys Compd., 2021, 885, 160990 CrossRef CAS.
- T. Wang, Y. Cao, H. Wu, C. Feng, Y. Ding and H. Mei, N-Doped M/CoO (M = Ni, Co, and Mn) Hybrid Grown on Nickel Foam as Efficient Electrocatalyst for the Chemical-Assisted Water Electrolysis, Int. J. Hydrogen Energy, 2022, 47(9), 5766–5778 CrossRef.
- F. Liu, H. Li, Y. Li, Q. Cao, X. Geng, C. Gu, W. Sun and J. Wang, P-Doped CoCO3 Nanosheets: An Ultra-Active Versatile Electrocatalyst for Hydrogen Evolution, Oxygen Evolution and Hydrazine Oxidation Reactions, Sustainable Energy Fuels, 2021, 5(8), 2257–2265 RSC.
- J. Wang, Y. Zhang, W. Zhang, Q. Zhao, J. Li and G. Liu, Constructing Sulfide/Phosphide Heterostructure Boosts the Activity of Iron-Manganese Bimetallic Electrocatalysts for Oxygen Evolution Reaction at Large Current Densities, Electrochim. Acta, 2023, 438, 141563 CrossRef.
- L. Lin, S. Piao, Y. Choi, L. Lyu, H. Hong, D. Kim, J. Lee, W. Zhang and Y. Piao, Nanostructured Transition Metal Nitrides as Emerging Electrocatalysts for Water Electrolysis: Status and Challenges, EnergyChem, 2022, 4(2), 100072 CrossRef.
- X. Peng, Y. Yan, X. Jin, C. Huang, W. Jin, B. Gao and P. K. Chu, Recent Advance and Prospectives of Electrocatalysts Based on Transition Metal Selenides for Efficient Water Splitting, Nano Energy, 2020, 78, 105234 CrossRef CAS.
- M. Wang, L. Zhang, Y. He and H. Zhu, Recent Advances in Transition-Metal-Sulfide-Based Bifunctional Electrocatalysts for Overall Water Splitting, J. Mater. Chem. A, 2021, 9(9), 5320–5363 RSC.
- S. Anantharaj, S. R. Ede, K. Sakthikumar, K. Karthick, S. Mishra and S. Kundu, Recent Trends and Perspectives in Electrochemical Water Splitting with an Emphasis on Sulfide, Selenide, and Phosphide Catalysts of Fe, Co, and Ni: A Review, ACS Catal., 2016, 6(12), 8069–8097 CrossRef CAS.
- Z. Feng, E. Wang, S. Huang and J. Liu, A Bifunctional Nanoporous Ni-Co-Se Electrocatalyst with a Superaerophobic Surface for Water and Hydrazine Oxidation, Nanoscale, 2020, 12(7), 4426–4434 RSC.
- J. Hou, X. Peng, J. Sun, S. Zhang, Q. Liu, X. Wang, J. Luo and X. Liu, Accelerating Hydrazine-Assisted Hydrogen Production Kinetics with Mn Dopant Modulated CoS2 Nanowire Arrays, Inorg. Chem. Front., 2022, 9(12), 3047–3058 RSC.
- X. Wang, W. Zhang, Q. Yu, X. Liu, Q. Liang, X. Meng, X. Wang and L. Wang, Fe-Doped CoNiP@N-Doped Carbon Nanosheet Arrays for Hydrazine Oxidation Assisting Energy-Saving Seawater Splitting, Chem. Eng. J., 2022, 446, 136987 CrossRef CAS.
- T. Shi, B. Gao, H. Meng, Y. Fu, D. Kong, P. Ren, H. Fu and Z. Feng, In Situ Electronic Redistribution of NiCoZnP/NF Heterostructure via Fe-Doping for Boosting Hydrazine Oxidation and Hydrogen Evolution, Green Chem., 2024, 26(7), 4209–4220 RSC.
- N. Sinha, C. Das and P. Roy, Iron-Doped Cobalt Nitride as an Efficient Electrocatalyst towards Energy Saving Hydrazine Assisted Seawater Splitting in near Neutral to Highly Alkaline pH Achieving Industry Level Current Density, Int. J. Hydrogen Energy, 2024, 51, 1011–1021 CrossRef.
- R.-Q. Li, S. Zeng, B. Sang, C. Xue, K. Qu, Y. Zhang, W. Zhang, G. Zhang, X. Liu, J. Deng, O. Fontaine and Y. Zhu, Regulating Electronic Structure of Porous Nickel Nitride Nanosheet Arrays by Cerium Doping for Energy-Saving Hydrogen Production Coupling Hydrazine Oxidation, Nano Res., 2023, 16(2), 2543–2550 CrossRef.
- X. Liu, J. He, S. Zhao, Y. Liu, Z. Zhao, J. Luo, G. Hu, X. Sun and Y. Ding, Self-Powered H2 Production with Bifunctional Hydrazine as Sole Consumable, Nat. Commun., 2018, 9(1), 4365 CrossRef.
- J. Zhang, Y. Liu, J. Li, X. Jin, Y. Li, Q. Qian, Y. Wang, A. El-Harairy, Z. Li, Y. Zhu, H. Zhang, M. Cheng, S. Zeng and G. Zhang, Vanadium Substitution Steering Reaction Kinetics Acceleration for Ni3N Nanosheets Endows Exceptionally Energy-Saving Hydrogen Evolution Coupled with Hydrazine Oxidation, ACS Appl. Mater. Interfaces, 2021, 13(3), 3881–3890 CrossRef CAS.
- Y. Liu, J. Zhang, Y. Li, Q. Qian, Z. Li, Y. Zhu and G. Zhang, Manipulating Dehydrogenation Kinetics through Dual-Doping Co3N Electrode Enables Highly Efficient Hydrazine Oxidation Assisting Self-Powered H2 Production, Nat. Commun., 2020, 11(1), 1853 CrossRef CAS.
- C. Feng, M. Lv, J. Shao, H. Wu, W. Zhou, S. Qi, C. Deng, X. Chai, H. Yang, Q. Hu and C. He, Lattice Strain Engineering of Ni2P Enables Efficient Catalytic Hydrazine Oxidation-Assisted Hydrogen Production, Adv. Mater., 2023, 35(42), 2305598 CrossRef CAS.
- X. Wei, S. Zhang, X. Lv, S. Dai, H. Wang and M. Huang, Local-Reconstruction Enables Cobalt Phosphide Array with Bifunctional Hydrogen Evolution and Hydrazine Oxidation, Appl. Catal., B: Environ. Energy, 2024, 345, 123661 CrossRef CAS.
- D. Xiong, X. He, X. Liu, S. Gong, C. Xu, Z. Tu, D. Wu, J. Wang and Z. Chen, 1D/3D Heterogeneous Assembling Body of Cobalt Nitrides for Highly Efficient Overall Hydrazine Splitting and Supercapacitors, Small, 2024, 20(8), 2306100 CrossRef CAS.
- Q. Qian, Y. Li, Y. Liu, Y. Guo, Z. Li, Y. Zhu and G. Zhang, Hierarchical Multi-Component Nanosheet Array Electrode with Abundant NiCo/MoNi4 Heterostructure Interfaces Enables Superior Bifunctionality towards Hydrazine Oxidation Assisted Energy-Saving Hydrogen Generation, Chem. Eng. J., 2021, 414, 128818 CrossRef CAS.
- S. Zhuang, Y. Tang, X. Tai, Q. Huang, P. Wan, Y. Chen, Y. Sun, J. Pan and X. J. Yang, Hydrogen and Electricity Co-Generation from Hydrazine-Assisted Water Electrolysis on Hierarchical Porous Heteroatoms-Doped CoCu Catalysts, Appl. Catal., B, 2022, 306, 121132 CrossRef.
- Y. Yang, X. Li, G. Liu, H. Liu, Y. Shi, C. Ye, Z. Fang, M. Ye and J. Shen, Hierarchical Ohmic Contact Interface Engineering for Efficient Hydrazine-Assisted Hydrogen Evolution Reaction, Adv. Mater., 2024, 36(7), 2307979 CrossRef.
- Q. Qian, J. Zhang, J. Li, Y. Li, X. Jin, Y. Zhu, Y. Liu, Z. Li, A. El-Harairy, C. Xiao, G. Zhang and Y. Xie, Artificial Heterointerfaces Achieve Delicate Reaction Kinetics towards Hydrogen Evolution and Hydrazine Oxidation Catalysis, Angew. Chem., Int. Ed., 2021, 60(11), 5984–5993 CrossRef PubMed.
- H.-M. Yang, H.-Y. Wang, M.-L. Sun and Z.-Y. Yuan, Interface Engineering of Bifunctional Nickel Hydroxide/Nickel Phosphide Heterostructure for Efficient Intermittent Hydrazine-Assisted Water Splitting, Chem. Eng. J., 2023, 475, 146134 CrossRef.
- Y. Liu, J. Zhang, Y. Li, Q. Qian, Z. Li and G. Zhang, Realizing the Synergy of Interface Engineering and Chemical Substitution for Ni3N Enables Its Bifunctionality Toward Hydrazine Oxidation Assisted Energy-Saving Hydrogen Production, Adv. Funct. Mater., 2021, 31(35), 2103673 CrossRef CAS.
- X. Wang, H. Hu, X. Yan, Z. Zhang and M. Yang, Activating Interfacial Electron Redistribution in Lattice-Matched Biphasic Ni3N-Co3N for Energy-Efficient Electrocatalytic Hydrogen Production via Coupled Hydrazine Degradation, Angew. Chem., Int. Ed., 2024, 63(19), e202401364 CrossRef CAS PubMed.
- S. Ma, B. Yu, B. Y. Xia and B. You, A Pyridinic Nitrogen-Rich Carbon Paper for Hydrazine Oxidation-Hybrid Seawater Electrolysis toward Efficient H2 Generation, Sci. China Mater., 2024, 67(3), 752–761 CrossRef CAS.
- Y. Zhu, J. Zhang, Q. Qian, Y. Li, Z. Li, Y. Liu, C. Xiao, G. Zhang and Y. Xie, Dual Nanoislands on Ni/C Hybrid Nanosheet Activate Superior Hydrazine Oxidation-Assisted High-Efficiency H2 Production, Angew. Chem., Int. Ed., 2022, 61(2), e202113082 CrossRef CAS.
- W. Liu, T. Shi and Z. Feng, Bifunctional Zeolitic Imidazolate Framework-67 Coupling with CoNiSe Electrocatalyst for Efficient Hydrazine-Assisted Water Splitting, J. Colloid Interface Sci., 2023, 630, 888–899 CrossRef CAS PubMed.
- H. Wang, S. Wang, S. Liu, Y. Dai, Z. Jia, X. Li, S. Liu, F. Dang, K. J. Smith, X. Nie, S. Hou and X. Guo, Redox-Induced Controllable Engineering of MnO2-MnxCo3−xO4 Interface to Boost Catalytic Oxidation of Ethane, Nat. Commun., 2024, 15(1), 4118 CrossRef CAS PubMed.
- X. Cheng and Y. Tong, Interface Coupling of Cobalt Hydroxide/Molybdenum Disulfide Heterostructured Nanosheet Arrays for Highly Efficient Hydrazine-Assisted Hydrogen Generation, ACS Sustainable Chem. Eng., 2023, 11(8), 3219–3227 CrossRef CAS.
- Y. Guo, X. Liu, Y. Zang, Y. Wu, Q. Zhang, Z. Wang, Y. Liu, Z. Zheng, H. Cheng, B. Huang, Y. Dai and P. Wang, Constructing a Bifunctional MoO2/Co Heterojunction for Efficient Electrocatalytic Hydrogen Evolution and Hydrazine Oxidation, J. Mater. Chem. A, 2022, 10(33), 17297–17306 RSC.
- L. Gao, J. Xie, S. Liu, S. Lou, Z. Wei, X. Zhu and B. Tang, Crystalline Cobalt/Amorphous LaCoOx Hybrid Nanoparticles Embedded in Porous Nitrogen-Doped Carbon as Efficient Electrocatalysts for Hydrazine-Assisted Hydrogen Production, ACS Appl. Mater. Interfaces, 2020, 12(22), 24701–24709 CrossRef.
- D. R. Cummins, U. Martinez, A. Sherehiy, R. Kappera, A. Martinez-Garcia, R. K. Schulze, J. Jasinski, J. Zhang, R. K. Gupta, J. Lou, M. Chhowalla, G. Sumanasekera, A. D. Mohite, M. K. Sunkara and G. Gupta, Efficient Hydrogen Evolution in Transition Metal Dichalcogenides via a Simple One-Step Hydrazine Reaction, Nat. Commun., 2016, 7(1), 11857 CrossRef PubMed.
- X. Xu, T. Wang, L. Dong, W. Lu and X. Miao, Energy-Efficient Hydrogen Evolution Reactions via Hydrazine Oxidation over Facile Synthesis of Cobalt Tetraoxide Electrodes, ACS Sustainable Chem. Eng., 2020, 8(21), 7973–7980 CrossRef.
- F. Shen, Z. Wang, Y. Wang, G. Qian, M. Pan, L. Luo, G. Chen, H. Wei and S. Yin, Highly Active Bifunctional Catalyst: Constructing FeWO4-WO3 Heterostructure for Water and Hydrazine Oxidation at Large Current Density, Nano Res., 2021, 14(11), 4356–4361 CrossRef.
- B. Li, K. Wang, J. Ren and P. Qu, NiOOH@Cobalt Copper Carbonate Hydroxide Nanorods as Bifunctional Electrocatalysts for Highly Efficient Water and Hydrazine Oxidation, New J. Chem., 2022, 46(16), 7615–7625 RSC.
- X. H. Chen, H. C. Fu, L. L. Wu, X. L. Li, B. Yang, T. Li, F. Gu, J. L. Lei, N. B. Li and H. Q. Luo, Tuning the D-Band Center of NiC2O4 with Nb2O5 to Optimize the Volmer Step for Hydrazine Oxidation-Assisted Hydrogen Production, Green Chem., 2022, 24(14), 5559–5569 RSC.
- S. Chen, C. Wang, S. Liu, M. Huang, J. Lu, P. Xu, H. Tong, L. Hu and Q. Chen, Boosting Hydrazine Oxidation Reaction on CoP/Co Mott–Schottky Electrocatalyst through Engineering Active Sites, J. Phys. Chem. Lett., 2021, 12(20), 4849–4856 CrossRef CAS PubMed.
- Q. Sun, M. Zhou, Y. Shen, L. Wang, Y. Ma, Y. Li, X. Bo, Z. Wang and C. Zhao, Hierarchical Nanoporous Ni(Cu) Alloy Anchored on Amorphous NiFeP as Efficient Bifunctional Electrocatalysts for Hydrogen Evolution and Hydrazine Oxidation, J. Catal., 2019, 373, 180–189 CrossRef CAS.
- Y. Peng, M. Huang, Q. Yang, Z. Xing and Z.-H. Lu, Replacing Oxygen Evolution with Hydrazine Borane Oxidation for Energy-Saving Electrochemical Hydrogen Production, Inorg. Chem., 2023, 62(28), 11056–11063 CrossRef CAS PubMed.
- Q. Liu, M.-Y. Li, Y.-M. Shi, C.-B. Liu, Y.-F. Yu and B. Zhang, In Situ Structural Reconstruction of NiMo Alloy as a Versatile Organic Oxidation Electrode for Boosting Hydrogen Production, Rare Met., 2022, 41(3), 836–843 CrossRef CAS.
- T. Zhang, S. Debow, F. Song, Y. Qian, W. R. Creasy, B. G. DeLacy and Y. Rao, Interface Catalysis of Nickel Molybdenum (NiMo) Alloys on Two-Dimensional (2D) MXene for Enhanced Hydrogen Electrochemistry, J. Phys. Chem. Lett., 2021, 12(46), 11361–11370 CrossRef CAS PubMed.
- A. Nairan, P. Zou, C. Liang, J. Liu, D. Wu, P. Liu and C. Yang, NiMo Solid Solution Nanowire Array Electrodes for Highly Efficient Hydrogen Evolution Reaction, Adv. Funct. Mater., 2019, 29(44), 1903747 CrossRef.
- D. Senthil Raja, C.-C. Cheng, Y.-C. Ting and S.-Y. Lu, NiMo-MOF-Derived Carbon-Armored Ni4Mo Alloy of an Interwoven Nanosheet Structure as an Outstanding pH-Universal Catalyst for Hydrogen Evolution Reaction at High Current Densities, ACS Appl. Mater. Interfaces, 2023, 15(16), 20130–20140 CrossRef.
- Z. Xiao, J. Wang, H. Lu, Y. Qian, Q. Zhang, A. Tang and H. Yang, Hierarchical Co/MoNi Heterostructure Grown on Monocrystalline CoNiMoOx Nanorods with Robust Bifunctionality for Hydrazine Oxidation-Assisted Energy-Saving Hydrogen Evolution, J. Mater. Chem. A, 2023, 11(29), 15749–15759 RSC.
- S. Zhang, C. Zhang, X. Zheng, G. Su, H. Wang and M. Huang, Integrating Electrophilic and Nucleophilic Dual Sites on Heterogeneous Bimetallic Phosphide via Enhancing Interfacial Electronic Field to Boost Hydrazine Oxidation and Hydrogen Evolution, Appl. Catal., B, 2023, 324, 122207 CrossRef.
- W. Zhang, X. Liu, Q. Yu, X. Wang, H. Mao, J. Chi, B. Li, J. Wan and L. Wang, In Situ Electronic Redistribution of Ni2P Hierarchical Structure for Energy-Saving Hydrogen Production in Seawater, Chem. Eng. J., 2023, 454, 140210 CrossRef CAS.
- Y. Liang, Y. Li, H. Wang, J. Zhou, J. Wang, T. Regier and H. Dai, Co3O4 Nanocrystals on Graphene as a Synergistic Catalyst for Oxygen Reduction Reaction, Nat. Mater., 2011, 10(10), 780–786 CrossRef CAS PubMed.
- S. Hu, Y. Tan, C. Feng, H. Wu, J. Zhang and H. Mei, Synthesis of N Doped NiZnCu-Layered Double Hydroxides with Reduced Graphene Oxide on Nickel Foam as Versatile Electrocatalysts for Hydrogen Production in Hybrid-Water Electrolysis, J. Power Sources, 2020, 453, 227872 CrossRef CAS.
- M. Zabihinezhad, T. Shahrabi, C. Zheng, T. Shao, G. B. Darband and J. Li, Pulse-Reverse Electrodeposited Reduced Graphene Oxide Electrode Decorated by Ni-P Porous Nano-Layer as a High Performance Electrocatalyst for H2 Production Assisted by Hydrazine
Electrooxidation, Appl. Catal., A, 2023, 666, 119415 CrossRef CAS.
- J. Ding, D. Guo, A. Hu, X. Yang, K. Shen, L. Chen and Y. Li, Resisting Metal Aggregation in Pyrolysis of MOFs towards High-Density Metal Nanocatalysts for Efficient Hydrazine Assisted Hydrogen Production, Nano Res., 2023, 16(5), 6067–6075 CrossRef CAS.
- F. Sun, J. Qin, Z. Wang, M. Yu, X. Wu, X. Sun and J. Qiu, Energy-Saving Hydrogen Production by Chlorine-Free Hybrid Seawater Splitting Coupling Hydrazine Degradation, Nat. Commun., 2021, 12(1), 4182 CrossRef CAS PubMed.
- Y. Xin, K. Shen, T. Guo, L. Chen and Y. Li, Coupling Hydrazine Oxidation with Seawater Electrolysis for Energy-Saving Hydrogen Production over Bifunctional CoNC Nanoarray Electrocatalysts, Small, 2023, 2300019 CrossRef.
- J. Liang, Z. Li, X. He, Y. Luo, D. Zheng, Y. Wang, T. Li, B. Ying, S. Sun, Z. Cai, Q. Liu, B. Tang and X. Sun, Electrocatalytic Seawater Splitting: Nice Designs, Advanced Strategies, Challenges and Perspectives, Mater. Today, 2023, 69, 193–235 CrossRef.
- H. Liu, W. Shen, H. Jin, J. Xu, P. Xi, J. Dong, Y. Zheng and S.-Z. Qiao, High-Performance Alkaline Seawater Electrolysis with Anomalous Chloride Promoted Oxygen Evolution Reaction, Angew. Chem., Int. Ed., 2023, 62(46), e202311674 CrossRef PubMed.
- L. Guo, Q. Yu, X. Zhai, J. Chi, T. Cui, Y. Zhang, J. Lai and L. Wang, Reduction-Induced Interface Reconstruction to Fabricate MoNi4-Based Hollow Nanorods for Hydrazine Oxidation Assisted Energy-Saving Hydrogen Production in Seawater, Nano Res., 2022, 15, 8846–8856 CrossRef.
- Z. Li, X. He, Q. Qian, Y. Zhu, Y. Feng, W. Wan and G. Zhang, Active Site Implantation for Ni(OH)2 Nanowire Network Achieves Superior Hybrid Seawater Electrolysis at 1 A cm−2 with Record-Low Cell Voltage, Adv. Funct. Mater., 2023, 33(41), 2304079 CrossRef CAS.
- W. Liu, W. Liu, T. Hou, J. Ding, Z. Wang, R. Yin, X. San, L. Feng, J. Luo and X. Liu, Coupling Co-Ni Phosphides for Energy-Saving Alkaline Seawater Splitting, Nano Res., 2024, 17(6), 4797–4806 CrossRef CAS.
- X. Liu, H. Mao, G. Liu, Q. Yu, S. Wu, B. Li, G. Zhou, Z. Li and L. Wang, Metal Doping and Hetero-Engineering of Cu-Doped CoFe/Co Embedded in N-Doped Carbon for Improving Trifunctional Electrocatalytic Activity in Alkaline Seawater, Chem. Eng. J., 2023, 451, 138699 CrossRef CAS.
- K. Wang, C. Liang, Z. Yi, F. Xu, Y. Wang, L. Lei, L. Zhuang and Z. Xu, Bimetallic Nickel-Molybdenum/Tungsten Nanoalloys for High-Efficiency Overall Hydrazine Splitting in Seawater Electrolytes, Sci. China Mater., 2023, 66(10), 3846–3854 CrossRef CAS.
- X. Xu, H.-C. Chen, L. Li, M. Humayun, X. Zhang, H. Sun, D. P. Debecker, W. Zhang, L. Dai and C. Wang, Leveraging Metal Nodes in Metal–Organic Frameworks for Advanced Anodic Hydrazine Oxidation Assisted Seawater Splitting, ACS Nano, 2023, 17(11), 10906–10917 CrossRef CAS.
- Q. Yu, J. Chi, G. Liu, X. Wang, X. Liu, Z. Li, Y. Deng, X. Wang and L. Wang, Dual-Strategy of Hetero-Engineering and Cation Doping to Boost Energy-Saving Hydrogen Production via Hydrazine-Assisted Seawater Electrolysis, Sci. China Mater., 2022, 65(6), 1539–1549 CrossRef CAS.
- J. Zhao, H. Guo, Q. Zhang, Y. Li, L. Gu and R. Song, Trace Ru Atoms Implanted into a Ni/Fe-Based Oxalate Solid-Solution-like with High-Indexed Facets for Energy-Saving Overall Seawater Electrolysis Assisted by Hydrazine, Appl. Catal., B, 2023, 325, 122354 CrossRef CAS.
- Z. Zhao, Z. Li, Z. Zhang and X. Meng, Fe/P Dual-Doping NiMoO4 with Hollow Structure for Efficient Hydrazine Oxidation-Assisted Hydrogen Generation in Alkaline Seawater, Appl. Catal., B: Environ. Energy, 2024, 347, 123805 CrossRef.
- J. Chi, L. Guo, J. Mao, T. Cui, J. Zhu, Y. Xia, J. Lai and L. Wang, Modulation of Electron Structure and Dehydrogenation Kinetics of Nickel Phosphide for Hydrazine-Assisted Self-Powered Hydrogen Production in Seawater, Adv. Funct. Mater., 2023, 33(46), 2300625 CrossRef.
- G. Meng, Z. Chang, L. Zhu, C. Chen, Y. Chen, H. Tian, W. Luo, W. Sun, X. Cui and J. Shi, Adsorption Site Regulations of [W–O]-Doped CoP Boosting the Hydrazine Oxidation-Coupled Hydrogen Evolution at Elevated Current Density, Nano-Micro Lett., 2023, 15(1), 212 CrossRef.
- Y. Hu, T. Chao, Y. Li, P. Liu, T. Zhao, G. Yu, C. Chen, X. Liang, H. Jin, S. Niu, W. Chen, D. Wang and Y. Li, Cooperative Ni(Co)-Ru-P Sites Activate Dehydrogenation for Hydrazine Oxidation Assisting Self-Powered H2 Production, Angew. Chem., Int. Ed., 2023, 62(35), e202308800 CrossRef.
- X. Zhai, Q. Yu, J. Chi, X. Wang, B. Li, B. Yang, Z. Li, J. Lai and L. Wang, Accelerated Dehydrogenation Kinetics through Ru, Fe Dual-Doped Ni2P as Bifunctional Electrocatalyst for Hydrazine-Assisted Self-Powered Hydrogen Generation, Nano Energy, 2023, 105, 108008 CrossRef CAS.
- H. Roh, H. Choi, J.-S. Kim, H. Shin, T. Park and K. Yong, Low Power Consumed PV-Electrolysis with CoFeP Nanowires for Hydrazine-Assisted Hydrogen Production, Appl. Surf. Sci., 2022, 606, 154951 CrossRef CAS.
- F. Lv, J. Wu, X. Liu, Z. Zheng, L. Pan, X. Zheng, L. Guo and Y. Chen, Decoupled Electrolysis for Hydrogen Production and Hydrazine Oxidation via High-Capacity and Stable Pre-Protonated Vanadium Hexacyanoferrate, Nat. Commun., 2024, 15(1), 1339 CrossRef CAS.
|
This journal is © the Partner Organisations 2024 |
Click here to see how this site uses Cookies. View our privacy policy here.