DOI:
10.1039/D4QO01793E
(Research Article)
Org. Chem. Front., 2024,
11, 7011-7026
Pyrrolylsulfonium salts: stable, accessible and versatile pseudohalides for Stille couplings†
Received
25th September 2024
, Accepted 16th October 2024
First published on 24th October 2024
Abstract
Pyrrolyl halides can be difficult to synthesise in a regioselective manner and are often unstable, which has hampered their application in cross-coupling. Here we introduce pyrrolylsulfonium salts as advantageous pseudohalide coupling partners and showcase their applicability in Stille couplings. Benefits of these salts include their straightforward synthesis via an “interrupted Pummerer” process, their high stability, and the ability to selectively introduce the sulfonium group at either the pyrrole α- or β- position as required. The Stille coupling has been demonstrated for aryl, heteroaryl and alkynylstannanes, and the effect of the pyrrole substituents on the regioselectivity of S–C bond activation has been investigated. Conditions to effect N-desulfonylation of N-trisyl coupling products have been identified.
Introduction
The pyrrole ring system is commonly found in organic materials,1 as well as in natural products2 and drug substances3 (Fig. 1). For example, GS70 is a pyrrole-based electron acceptor which has been used to fabricate organic solar cells that can achieve high power conversion efficiencies,4 P(DKPP-TPTH) is a pyrrole-containing polymer which has been used to construct organic field-effect transistors,5 and OCF3-BnPyV is a viologen-substituted pyrrole which has been used in electrochromic devices.6 The natural products cycloprodigiosin7 and heronapyrrole A8 have immunosuppressant and antibiotic properties, respectively. The licensed drug vonoprazan9 is a potassium-competitive acid blocker indicated for gastroduodenal ulcers and the investigational drug resminostat is in trials for oncology indications.10
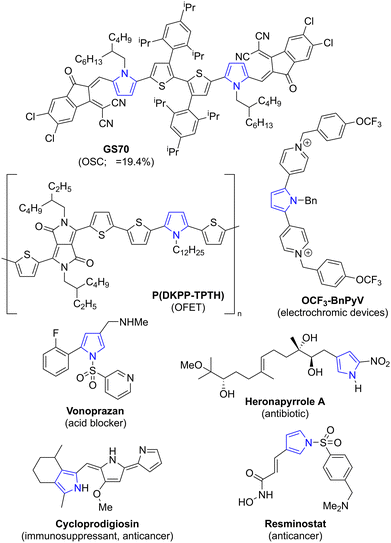 |
| Fig. 1 A selection of pyrrole-containing molecules. | |
Many synthetic strategies to access pyrrole-containing targets have been reported. These may be divided into de novo pyrrole syntheses11 (where the pyrrole ring is formed with substituents already in place) and pyrrole functionalization approaches (where substituents are introduced onto a pre-existing pyrrole ring). In this latter category, SEAr and pyrrole metalation protocols are well developed.12 Transition metal-catalysed cross-couplings have also been studied for pyrrole functionalization,13 although some shortcomings remain to be overcome. For example, the use of pyrrolyl halides as classical electrophilic cross-coupling partners may be hindered by difficulties of synthetic access and/or instability. Thus, halogenation of N-H pyrrole or simple N-alkyl/N-aryl derivatives with a variety of electrophilic halogen sources reportedly often leads to complex mixtures of mono- and polyhalopyrroles. Moreover, the parent N-H-2-halopyrroles are markedly unstable, decomposing upon attempted isolation (Scheme 1a).14,15 Selectivity for halogenation at C2 vs. C3 can also vary depending on the substrate and synthetic method, and separation is usually challenging. Whilst N-halosuccinmides can exhibit good selectivity for halogenation at C2 over C3, overreaction to the 2,4-dihalopyrrole can occur.14 To access 3-halopyrroles, an acid-mediated isomerization (“halogen dance”) of the 2-halo isomer can sometimes be employed, but this can also induce a degree of disproportionation (Scheme 1b).14,16 Halopyrroles substituted with electron-withdrawing groups can be more stable in some cases. For example, pyrroles bearing a carbonyl at C2 generally undergo electrophilic halogenation to give stable products, but regioselectivity between C4 and C5 depends on the halogenating agent and on the nature of the carbonyl (Scheme 1c).15,16a,17 Alternatively, use of an electron-withdrawing protecting group on nitrogen can sometimes increase stability. For example, considering bromopyrroles specifically, N-Boc-2-bromopyrrole18 can reportedly be stored as a solution in hexane at −10 °C,19 and N-tosyl-2-bromopyrrole20 is stable in pure form. N-Tosyl-3-bromopyrrole may be synthesized by treating N-tosyl-pyrrole with Br2 under acidic conditions,21 and N-Boc-3-bromopyrrole is synthesized from N-TIPS-3-bromopyrrole by protecting group exchange22 (Scheme 1d). However, all of these examples have in common that the yields reported for their preparation (by the same procedure) vary appreciably.
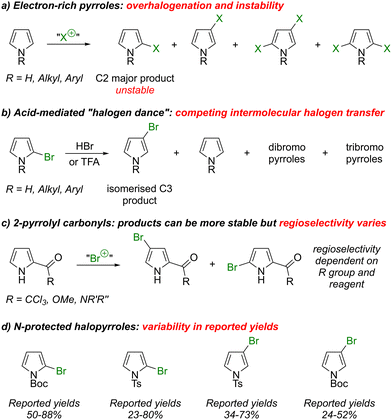 |
| Scheme 1 Stability and selectivity problems in the formation of halopyrroles. | |
Whilst some cross-couplings of halopyrroles such as those in Scheme 1 have been reported,23 their more widespread utilisation has been hampered by the issues described above. An alternative approach is the use of a pyrrole pseudohalide for cross-coupling. Thus far, there are some examples of pyrrole triflates being used as pseudohalides in cross-couplings,24 primarily in natural product total synthesis, but the preparation of the substrates has not been generalised. Pyrrole C–H functionalisation approaches have also been developed, each with varying scope in substrate, coupling partner, catalyst, etc.25
Sulfonium salts have become established as pseudohalides that can have advantages over classical halide coupling partners. Cross-coupling of sulfonium salts was first reported by Liebeskind et al.,26 who demonstrated Stille, Suzuki–Miyaura and Negishi couplings with aryl, heteroaryl, alkenyl and benzyl sulfonium salts. Since then the scope of sulfonium salt cross-coupling has been expanded to include various other nucleophilic coupling partners, carbonylative couplings, reductive cross-electrophile couplings, etc., employing both Pd and Ni (Scheme 2a).27,28 Sulfonium salts are also synthetically useful in other contexts, and their chemistry has been reviewed.29 We previously reported the synthesis of azulenesulfonium salts and their use in cross-coupling.30 These salts proved superior to azulene halides in terms of their stability and ease of synthesis. Synthesis of azulene halides by SEAr reaction on the electron-rich five-membered ring typically leads to overhalogenation, and the products are unstable (unless electron-withdrawing groups are also present). In contrast azulenesulfonium salts may be synthesised in good yield, as the sole products, and are stable without any special handling/storage precautions (Scheme 2b). The cationic sulfonium substituent serves to reduce the electron density of the aromatic system, thereby imparting stability. We recognised that many of the drawbacks of azulene halides (instability, difficulty of synthesis/purification) were shared with pyrrolyl halides, which also comprise an electron-rich five-membered aromatic ring. Therefore we sought to develop pyrrolylsulfonium salts for use in cross-coupling, anticipating that they would share the advantages of azulenesulfonium salts. The results of these studies are reported here (Scheme 2c). Pyrrolylsulfonium salts are known,31 but to date they have been primarily used as radical precursors, in sigmatropic rearrangements or as substrates for dealkylation to give pyrrolyl thioethers.
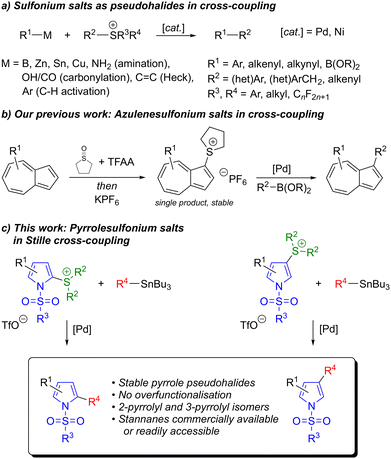 |
| Scheme 2 Sulfonium salts are competent pseudohalides in cross-coupling. | |
Results and discussion
Aryl sulfonium salts can be synthesised by various approaches, including reaction of aryl thiols/thioethers with electrophiles, or activation of sulfoxides and attack by nucleophiles.29j For the synthesis of pyrrolylsulfonium salts we opted to employ an “interrupted Pummerer” reaction,32 wherein a sulfoxide is activated by an acid anhydride, followed by attack at sulfur by a nucleophilic (i.e. electron-rich) arene, loss of an oxygen leaving group and rearomatisation (Scheme 3a). In Pummerer reactions the sulfoxide is most commonly activated with a carboxylic acid anhydride, but in this case we employed a sulfonic acid anhydride (specifically triflic anhydride). This was due to our previous findings that use of a carboxylic acid anhydride can promote an alternative reaction pathway affording Δ3-pyrrol-2-one products.33
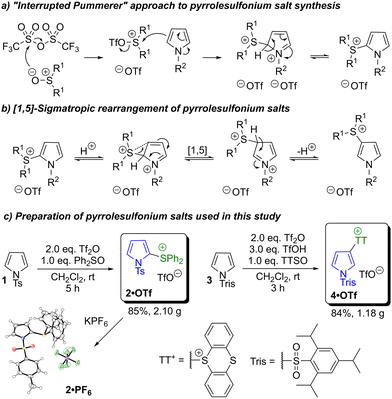 |
| Scheme 3 Synthesis of sulfonium salts by “interrupted Pummerer” reaction and their isomerisation by [1,5]-sigmatropic rearrangement. TTSO = thianthrene-S-oxide. | |
A key advantage of pyrrolylsulfonium salts as reagents for cross-coupling is easy access to both the 2-pyrrolyl and 3-pyrrolyl regioisomers. The interrupted Pummerer process typically installs the sulfonium salt at the 2-position, in keeping with the established SEAr reactivity of pyrrole. This product may then be isomerised to the 3-pyrrolylsulfonium salt via a Brønsted acid-catalysed [1,5]-sigmatropic rearrangement (Scheme 3b).31b–d Analogous migrations of various other functional groups on the pyrrole ring have been reported,34 although stability of the substrates to the highly acidic reaction conditions varies. In contrast, pyrrolylsulfonium salts are robust under these conditions and may be isomerised in high yield. Furthermore the interrupted Pummerer/rearrangement reaction cascade may be performed as a one-pot process. We prepared and screened multiple pyrrolylsulfonium salts to identify optimal reagents for cross-coupling that (a) are easily prepared in good yield on gram scale; (b) exhibit good stability; (c) have good solubility in the solvents to be used for cross-coupling; and (d) undergo oxidative addition into the correct C–S bond. From this screening we identified the novel salt diphenyl (N-(p-toluenesulfonyl)-1H-pyrrol-2-yl)sulfonium triflate 2·OTf as an ideal reagent for the preparation of 2-substituted pyrroles, which was prepared on a gram scale (Scheme 3c). Reaction workup involved straightforward partitioning between MeCN and hexane,35 followed by recrystallisation from methanol. The reaction generates an equivalent of triflic acid as a byproduct, which might be expected to catalyse the [1,5]-sigmatropic rearrangement shown in Scheme 3b. However, we found the specific combination of N-tosyl and diphenyl sulfonium substituents disfavoured the rearrangement under these reaction conditions, and hence 2-pyrrolyl (non-rearranged) salt 2·OTf could be isolated in 85% yield. In contrast, we found that thianthrenium salts underwent the rearrangement more readily and that additional triflic acid facilitated the process. Formation of the 3-pyrrolylthianthrenium salt with an N-tosyl protecting group occurred in variable yield and the product was only sparingly soluble. Switching to a more sterically demanding N-trisyl group improved product solubility and rendered the synthesis reproducible and also scalable to gram scale. We thus identified the novel 5-(N-(2,4,6-triisopropylphenylsulfonyl)-1H-pyrrol-3-yl)-5H-thianthrenium triflate 4·OTf as an ideal reagent for the preparation of 3-substituted pyrroles (Scheme 3c). Amongst the various sulfonium groups used as synthetic handles in the literature, the thianthrenium group has been extensively exploited due to its particular properties.36–38 For example, its introduction onto an aromatic ring typically proceeds in an exceptionally regioselective manner.28,39 In the case of N-trisyl pyrrole, NMR studies indicate that the initial thianthrenation affords a mixture of 4·OTf and its 2-pyrrolyl regioisomer. This then undergoes rearrangement to 4·OTf, such that after 3 hours 4·OTf is the only product present.31m,o Introduction of a bulky group at nitrogen is known to favour 3-substituted products in pyrrole SEAr reactions.22
To establish the applicability of pyrrolylsulfonium salts 2·OTf and 4·OTf in cross-coupling, we employed them as pseudohalides in the Migita–Kosugi–Stille coupling.40,41 Examples of pyrrolyl halide Stille couplings are known,42 although competing dehalogenation can reportedly be significant in some cases.23p Organostannanes are advantageous coupling partners due to their stability to air and moisture, as well as their availability from commercial sources or through straightforward syntheses.43 The robust nature of the Stille coupling and its efficiency under mild reaction conditions mean it has proven successful in many instances where other cross-coupling methodologies have failed.44 Whilst the (variable) toxicity of organostannanes45 necessitates the thorough removal of tin residues from active pharmaceutical ingredients prepared through Stille coupling, methods to achieve this are well developed.46
We selected phenyltributylstannane as an archetypal aryl stannane reagent for reaction optimisation. Reaction parameters were varied as shown in Scheme 4 and Table 1. Initial attempts using Pd(PPh3)4 and 1.3 equivalents of stannane showed the coupling was viable in DMF, toluene and t-butanol (entries 1–3). Desired product 5a formed in each case, with the highest conversion in DMF. Formation of byproducts was also observed, either as a result of N-sulfonyl group cleavage (giving 6) or reductive cleavage of the thianthrenium group (giving 3). This latter process is analogous to the dehalogenation sometimes observed with classical halide substrates. The beneficial effects of copper salt additives on Stille couplings are well documented,47 so we evaluated the effect of CuI as an additive in DMF and toluene (entries 4 and 5), but no improvement in conversion was observed. Fluoride salts are also known to promote Stille couplings,48 and synergistic effects arising from the presence of both copper and fluoride salts have been reported.49 Multiple conditions including caesium fluoride as an additive were evaluated (entry 6), but formation of desired product 5a was negligible in each case, with N-sulfonyl cleavage dominating instead to give 6. Use of an alternative Cu(I) source did not increase conversion to 5a (entries 7–10). Use of triphenylarsine as ligand50 in conjunction with Pd2(dba)3 as palladium source in DMF gave greater conversion to 5a (entries 11–15). Reaction temperature could be lowered to 50 °C without a reduction in conversion, and 2 mol% loading of Pd2(dba)3 (i.e. 4 mol% of Pd) was identified as optimal. Finally, a change in reaction stoichiometry to 2.2 equivalents of stannane increased conversion to 84% of 5a and suppressed formation of byproducts 3 and 6 (entry 15).
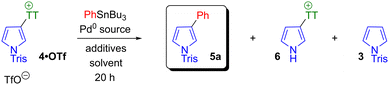 |
| Scheme 4 Stille coupling of 4·OTf to form desired 3-phenylpyrrole 5a and byproducts 6 and 3. | |
Table 1 Optimisation of Stille coupling of 3-pyrrolyl thianthrenium salt 4·OTf
Entry |
Pd(0) source |
PhSnBu3 (equiv.) |
Additive |
Ligand |
Solvent |
Conc. (M) |
Temp. (°C) |
Conv.% 5a |
Conv.% 6 |
Conv.% 3 |
Conversions obtained from crude 1H-NMR spectra by comparison to an internal standard (mesitylene). Cu(OTf)·Tol = copper(I) trifluoromethanesulfonate–toluene complex. 13% of starting material 4 remained. |
1 |
Pd(PPh3)4 (4 mol%) |
1.3 |
— |
— |
DMF |
0.12 |
110 |
64 |
<5 |
7 |
2 |
Pd(PPh3)4 (4 mol%) |
1.3 |
— |
— |
Toluene |
0.12 |
110 |
58 |
23 |
10 |
3a |
Pd(PPh3)4 (4 mol%) |
1.3 |
— |
— |
t
BuOH |
0.12 |
80 |
38 |
— |
6 |
4 |
Pd(PPh3)4 (4 mol%) |
1.3 |
CuI (16 mol%) |
— |
DMF |
0.12 |
110 |
38 |
<5 |
8 |
5 |
Pd(PPh3)4 (4 mol%) |
1.3 |
CuI (16 mol%) |
— |
Toluene |
0.12 |
110 |
50 |
— |
6 |
6 |
Pd(PPh3)4 (4 mol%) |
1.3 |
CsF (2.2 eq.) with/without CuI |
— |
DMF/toluene |
0.12 |
80–110 |
<5 |
10–50 |
<5 |
7 |
Pd(PPh3)4 (4 mol%) |
1.3 |
Cu(OTf)·Tol (16 mol%) |
— |
Toluene |
0.12 |
110 |
46 |
<5 |
5 |
8 |
Pd(PPh3)4 (8 mol%) |
1.3 |
Cu(OTf)·Tol (16 mol%) |
— |
Toluene |
0.12 |
85 |
24 |
8 |
5 |
9 |
Pd(PPh3)4 (4 mol%) |
1.3 |
Cu(OTf)·Tol (16 mol%) |
— |
Toluene |
0.12 |
80 |
44 |
16 |
6 |
10 |
Pd(PPh3)4 (4 mol%) |
1.3 |
Cu(OTf)·Tol (16 mol%) |
— |
Toluene |
0.07 |
110 |
47 |
16 |
10 |
11 |
Pd2(dba)3 (2 mol%) |
1.3 |
— |
AsPh3 (16 mol%) |
DMF |
0.12 |
80 |
71 |
6 |
5 |
12 |
Pd2(dba)3 (2 mol%) |
1.3 |
— |
AsPh3 (16 mol%) |
DMF |
0.12 |
50 |
70 |
3 |
6 |
13 |
Pd2(dba)3 (1 mol%) |
1.3 |
— |
AsPh3 (16 mol%) |
DMF |
0.12 |
50 |
57 |
— |
21 |
14 |
Pd2(dba)3 (4 mol%) |
1.3 |
— |
AsPh3 (16 mol%) |
DMF |
0.12 |
50 |
69 |
5 |
10 |
15
|
Pd
2
(dba)
3
(2 mol%)
|
2.2
|
— |
AsPh
3
(16 mol%)
|
DMF
|
0.12
|
50
|
84
|
— |
— |
An analogous reaction optimisation was carried out for the coupling of phenyltributylstannane with 2-pyrrolyl sulfonium salt 2·OTf. Reaction parameters were varied as shown in Scheme 5 and Table 2. An initial attempt using Pd(PPh3)4 in DMF showed the coupling to be viable, with desired 2-phenylpyrrole 7a forming in moderate yield (entry 1). Three byproducts were also identified, namely the N-sulfonyl cleavage product 8, the sulfonium reductive cleavage product 1 and the parent pyrrole 9. This latter byproduct (which was not observed in the coupling of 4·OTf) presumably arises from N-sulfonyl cleavage from 1, highlighting the greater stability of the N-trisyl group compared to N-tosyl.‡ Use of a fluoride additive with a copper(I) source was once again unproductive (entry 2). Use of AsPh3/Pd2(dba)3 in DMF proved superior to the Pd(PPh3)4 system, and no increase in conversion was observed when the loading of Pd2(dba)3 was increased beyond 2 mol% or the temperature was increased beyond 50 °C (entries 3–5). With this palladium/ligand combination, copper(I) additives again proved deleterious (entries 6 and 7). However, a change in reaction stoichiometry to 2.2 equivalents of stannane was beneficial (as was the case for the coupling of 4·OTf), increasing conversion to 74% of 7a and minimising byproduct formation.
 |
| Scheme 5 Stille coupling of 2·OTf to form desired 2-phenylpyrrole 7a and byproducts 8, 1 and 9. | |
Table 2 Optimisation of Stille coupling of 2-pyrrolyl sulfonium salt 2·OTf
Entry |
Pd(0) source |
PhSnBu3 (equiv.) |
Additive |
Ligand |
Solvent |
Temp. (°C) |
Conv% 7a |
Conv% 8 |
Conv% 1 |
Conv% 9 |
Conversions obtained from crude 1H-NMR spectra by comparison to an internal standard (mesitylene). Cu(OTf)·Tol = copper(I) trifluoromethanesulfonate–toluene complex. |
1 |
Pd(PPh3)4 (4 mol%) |
1.3 |
— |
— |
DMF |
80 |
31 |
6 |
23 |
7 |
2 |
Pd(PPh3)4 (4 mol%) |
1.3 |
CsF (2.2 eq.) Cu(OTf)·Tol (16 mol%) |
— |
DMF/toluene |
50–110 |
Trace |
20–60 |
Trace |
— |
3 |
Pd2(dba)3 (2 mol%) |
1.3 |
— |
AsPh3 (16 mol%) |
DMF |
50 |
64 |
14 |
15 |
— |
4 |
Pd2(dba)3 (4 mol%) |
1.3 |
— |
AsPh3 (16 mol%) |
DMF |
50 |
58 |
6 |
16 |
— |
5 |
Pd2(dba)3 (2 mol%) |
1.3 |
— |
AsPh3 (16 mol%) |
DMF |
80 |
53 |
10 |
18 |
— |
6 |
Pd2(dba)3 (2 mol%) |
1.3 |
CuI (16 mol%) |
AsPh3 (16 mol%) |
DMF |
50 |
24 |
16 |
25 |
6 |
7 |
Pd2(dba)3 (2 mol%) |
1.3 |
CuI (16 mol%) |
AsPh3 (16 mol%) |
DMF |
25 |
7 |
— |
12 |
3 |
8
|
Pd
2
(dba)
3
(2 mol%)
|
2.2
|
— |
AsPh
3
(16 mol%)
|
DMF
|
50
|
74
|
4 |
3 |
— |
With optimised conditions in hand for the coupling of both salts, substrate scope for the couplings was examined using a range of commercially available stannanes. For the coupling of 4·OTf (Scheme 6), both (substituted) phenyl (5a–5e) and heteroaryl (5f–5g) products were isolated in good yield. In the formation of chlorophenyl pyrrole 5d, no evidence was seen of unwanted C–Cl bond activation in the product.51 Alkynyl stannanes were also competent coupling partners (5h–5i). Use of a bis(stannyl)thiophene effected a double coupling to give 5j. To the best of our knowledge, the heteroaryl triad in 5j with this particular connectivity (2,5-bis(3-pyrrolyl)thiophene) is a previously unknown structural motif outside the field of porphyrin chemistry.52
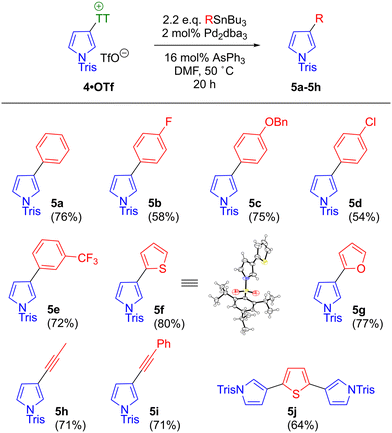 |
| Scheme 6 Coupling of 4·OTf with a range of stannanes. Yields in parentheses are isolated yields. | |
For the coupling of 2·OTf (Scheme 7), here also (substituted) phenyl (7a–7c), heteroaryl (7d–7e) and alkynyl (7f) products were accessible in good to moderate yield.
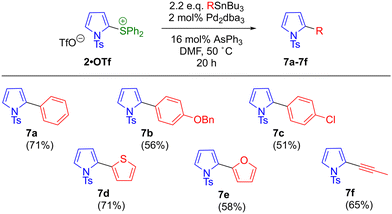 |
| Scheme 7 Coupling of 2·OTf with a range of stannanes. Yields in parentheses are isolated yields. | |
Preparation of sulfonium salts from C-substituted pyrroles was next attempted (Scheme 8). An N-tosyl pyrrole bearing a methyl ketone formed diphenylsulfonium salt 10·OTf in low yield, along with byproducts potentially arising from enol triflate formation, although 10·OTf was nevertheless isolable in pure form after chromatography. In contrast, a similar ketone-bearing substrate formed thianthrenium salt 11·OTf in a much better yield. Here, the presence of electron-withdrawing groups at both N1 and C3 seemingly disfavours the migration of the sulfonium group. Pyrrole substrates lacking N-sulfonyl protection were also examined, with salts 12·OTf and 13·OTf forming from the corresponding N-methyl pyrroles. Salt 13·OTf is the only regioisomer that can form from the corresponding substrate (1,2,5-trimethylpyrrole). However, 12·OTf forms from 2-formyl-N-methylpyrrole, which has 3 potential sites of attachment. As such, the exclusive isolation of the product with the thianthrenium group at C4 is noteworthy, given that the regioselectivity of SEAr reactions on 2-pyrrolyl carbonyls can vary (see Scheme 1c).
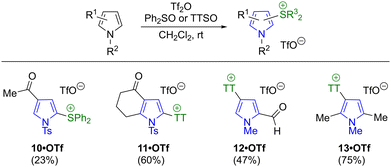 |
| Scheme 8 Synthesis of sulfonium salts from substituted pyrroles. TTSO = thianthrene-S-oxide. Yields in parentheses are isolated yields. | |
The successful cross-coupling of salts 2·OTf and 4·OTf requires that the initial oxidative addition step of the catalytic cycle is selective for the correct S–C bond. That is to say, palladium must insert into the bond between sulfur and the pyrrole ring, and not into a bond between sulfur and a phenyl ring (of the diphenyl sulfonium or thianthrenium group). During optimisation of the coupling conditions and during preparation of the products in Schemes 6 and 7, only products arising from activation of the correct S–C bond were isolated, and no products from coupling at the “wrong” S–C bond were ever detected. To determine the structural motif(s) responsible for regioselectivity in S–C bond activation, sulfonium salts from Scheme 8 were cross-coupled under the established conditions. Results with 2·OTf and 4·OTf indicate that with a sulfonium group at either C2 or C3, when the only other substituent is an electron-withdrawing (sulfonyl) group at N1, the desired regioselectivity is observed. Salts 10·OTf and 11·OTf are both substrates with a sulfonium handle at C2 as well as electron-withdrawing groups at N1 and C4. Coupling of 10·OTf with PhSnBu3 led to isolation of 14 and coupling of 11·OTf with 2-furyl-SnBu3 led to isolation of 15 as the sole product in each case (Scheme 9), illustrating that the desired regioselectivity is observed in this scenario also. In contrast, salt 12·OTf possesses a sulfonium handle at C4 and an electron-withdrawing group at C2, but lacks an electron-withdrawing group at N1. This salt underwent the coupling to give two products in approximately equal conversion, namely the desired product 16 and the product arising from ring cleavage of the thianthrenium motif, 17. Under the same reaction conditions, salt 13·OTf (lacking any electron-withdrawing groups) formed only the thianthrene ring cleavage product 18, in high conversion.
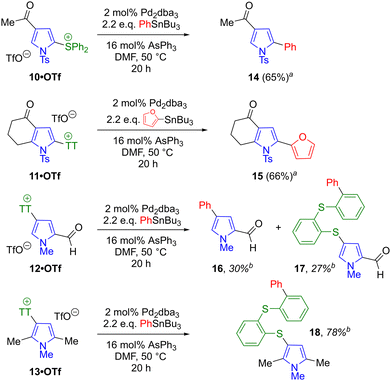 |
| Scheme 9 Coupling of C-substituted pyrrolylsulfonium salts. aIsolated yield. bConversion by 1H-NMR. | |
Formation of the products depicted in Scheme 9 highlights the role of the N-sulfonyl group in ensuring that the desired regioselectivity in the S–C bond activation step is achieved. Therefore, since pyrrolylsulfonium couplings of the type reported here are likely to be carried out on N-sulfonyl substrates specifically, we investigated removal of the N-sulfonyl group from selected coupling products. For N-tosyl pyrroles of the type shown in Scheme 7, conditions for N-deprotection are widely reported in the literature using, for example, sodium hydroxide in ethanol.20a–c However for N-trisyl pyrroles of the type shown in Scheme 6, the additional steric bulk renders the N-sulfonyl group much more resistant to removal. Attempted deprotection of thienylpyrrole 5f under basic hydrolysis conditions (NaOH/MeOH/Δ) led to recovery of starting material. A reported procedure for N-sulfonyl cleavage using triflic acid led to decomposition.53 Attempts at reductive S–N bond cleavage using SmI2 or complexes thereof also returned starting material.54 However, use of Mg in MeOH at 50 °C under sonication55 was successful at removing the N-trisyl group to give 3-(2-thienyl)pyrrole 19. Instability of this substance in air or upon attempted purification hampered quantitation of its formation. Therefore a less electron-rich N-trisylpyrrole was selected for deprotection (p-fluorophenyl product 5b). The resultant deprotection product 20 exhibited greater stability than 19, and it was determined to have formed in 73% conversion (Scheme 10).
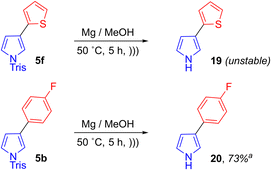 |
| Scheme 10
N-Deprotection of N-trisylpyrroles. aConversion by 1H-NMR. | |
A possible mechanism for a representative pyrrolylsulfonium Stille coupling is depicted in Scheme 11. The Pd0 active catalyst 21 will engage in oxidative addition into the C–S bond of a pyrrolylsulfonium salt such as 22 to give PdII complex 23. Whereas oxidative addition with a classical halide coupling partner would form a neutral PdII complex with a Pd–X bond, we propose that use of pyrrolylsulfonium pseudohalide 22 may instead lead to a cationic tricoordinate complex of type 23. In the specific case of thianthrenium salts, Ritter et al. have presented evidence that thianthrene (“TT”) is notably non-coordinating towards PdII complexes (less coordinating than triflate),56 although this may not necessarily apply for other sulfonium salts such as the diphenylsulfonium salts 2·OTf and 10·OTf. Transmetallation with the stannane reagent would afford tetracoordinate 24, which after isomerisation could undergo reductive elimination to give product 26. DMF is reportedly a non-innocent solvent in Stille couplings, and transmetallation can occur from a PdII complex in which DMF occupies a coordination site (i.e. L = DMF).50b Accordingly we do not speculate as to the specific identity of the “L” substituents in each of the complexes in Scheme 11. The mechanism shown is most likely a simplification of the true process, which may vary depending on the nature of the pyrrolylsulfonium salt and stannane used.40b,57 Accordingly the mechanism of this process merits further study.
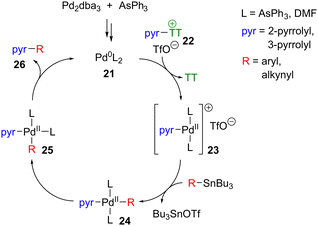 |
| Scheme 11 Proposed mechanistic cycle for pyrrolylsulfonium Stille couplings. | |
Conclusions
We have described the straightforward synthesis of multiple pyrrolylsulfonium salts and demonstrated their applicability as pseudohalides in Stille couplings. The approach used for the synthesis of these salts allows for their regioselective installation at either the pyrrole α- or β-position, through the choice of appropriate sulfonium substituents and reaction conditions for the pyrrole in question. The salts are formed in good yield and exhibit good stability, and the synthesis is not prone to overfunctionalisation; these are all significant advantages over the classical pyrrolyl halide coupling partners. Additionally, we have identified reaction conditions that are able to effect the Stille coupling of the pyrrolylsulfonium salts with a range of (hetero)aryl- and alkynyl-stannanes. When the pyrrolylsulfonium salt possesses an N-sulfonyl substituent, cross-coupling occurs at the desired S–C bond only. The sulfide byproduct is therefore recoverable and could be recycled for the synthesis of additional pyrrolylsulfonium salt, if required. Both the N-tosyl and N-trisyl groups have been shown to be removable subsequent to the coupling step. Furthermore, variants of this methodology may be applicable to other heterocycles. For example, while Stille couplings of other arylthianthrenium salts are unknown so far, we note that indolyl thianthrenium salts have been reported.§ For the reasons listed above we anticipate that the methodology described here may find applications in various synthetic contexts.
Author contributions
S.E.L. and J.L.H. conceived the project. J.L.H. carried out the synthesis. C.L.L. and J.L.H acquired and interpreted NMR data. G.K.-K. carried out X-ray crystallography. S.E.L. wrote the manuscript with input from all authors.
Data availability
The data supporting this article have been included as part of the ESI.† Crystallographic data for 2·PF6 and 5f have been deposited at the CCDC under 2382855 and 2382856.†
Conflicts of interest
There are no conflicts to declare.
Acknowledgements
We thank EPSRC for a DTP PhD studentship to J. L. H. (EP/T518013/1). We acknowledge the research facilities at the University of Bath (https://doi.org/10.15125/mx6j-3r54). We thank Dr Alex Cresswell for helpful discussions.
References
- C. Bulumulla, R. Gunawardhana, P. L. Gamage, J. T. Miller, R. N. Kularatne, M. C. Biewer and M. C. Stefan, Pyrrole-Containing Semiconducting Materials: Synthesis and Applications in Organic Photovoltaics and Organic Field-Effect Transistors, ACS Appl. Mater. Interfaces, 2020, 12, 32209–32232 CrossRef CAS PubMed.
-
(a) N. Singh, S. Singh, S. Kohli, A. Singh, H. Asiki, G. Rathee, R. Chandra and E. A. Anderson, Recent progress in the total synthesis of pyrrole-containing natural products (2011–2020), Org. Chem. Front., 2021, 8, 5550–5573 RSC;
(b) I. S. Young, P. D. Thornton and A. Thompson, Synthesis of natural products containing the pyrrolic ring, Nat. Prod. Rep., 2010, 27, 1801–1839 RSC.
- G. L. Petri, V. Spanò, R. Spatola, R. Holl, M. V. Raimondi, P. Barraja and A. Montalbano, Bioactive pyrrole-based compounds with target selectivity, Eur. J. Med. Chem., 2020, 208, 112783 CrossRef PubMed.
- J. Wang, C. Wang, Y. Wang, J. Qiao, J. Ren, J. Li, W. Wang, Z. Chen, Y. Yu, X. Hao, S. Zhang and J. Hou, Pyrrole-Based Fully Non-fused Acceptor for Efficient and Stable Organic Solar Cells, Angew. Chem., Int. Ed., 2024, 63, e202400565 CrossRef CAS PubMed.
- R. Agneeswari, I. Shin, V. Tamilavan, D. Y. Lee, S. Cho, Y. Jin, S. H. Park and M. H. Hyun, Modulation of the properties of pyrrolo[3,4-c]pyrrole-1,4-dione based polymers containing 2,5-di(2-thienyl)pyrrole derivatives with different substitutions on the pyrrole unit, New J. Chem., 2015, 39, 4658–4669 RSC.
- B. Deng, Y. Zhu, M. U. Ali, K. Li, X. Liu, X. Zhang, J. Ning, Z. Hu, H. Chen, J. He, Y. He and H. Meng, Pyrrole-based viologen derivatives with high contrast and magenta color for electrochromic-fluorescent devices, Sol. Energy Mater. Sol. Cells, 2023, 251, 112149 CrossRef CAS.
-
(a) R. Pandey, R. Chander and K. B. Sainis, Prodigiosins as anti cancer agents: living up to their name, Curr. Pharm. Des., 2009, 15, 732–741 CrossRef CAS PubMed;
(b) H. H. Wasserman and J. M. Fukuyama, The synthesis of (±)-cycloprodigiosin, Tetrahedron Lett., 1984, 25, 1387–1388 CrossRef CAS;
(c) E. E. Schultz and R. Sarpong, Application of In Situ-Generated Rh-Bound Trimethylenemethane Variants to the Synthesis of 3,4-Fused Pyrroles, J. Am. Chem. Soc., 2013, 135, 4696–4699 CrossRef CAS PubMed;
(d) R. E. Johnson, T. de Rond, V. N. G. Lindsay, J. D. Keasling and R. Sarpong, Synthesis of Cycloprodigiosin Identifies the Natural Isolate as a Scalemic Mixture, Org. Lett., 2015, 17, 3474–3477 CrossRef CAS PubMed.
-
(a) R. Raju, A. M. Piggott, L. X. Barrientos Diaz, Z. Khalil and R. J. Capon, Heronapyrroles A−C: Farnesylated 2-Nitropyrroles from an Australian Marine-Derived Streptomyces sp., Org. Lett., 2010, 12, 5158–5161 CrossRef CAS PubMed;
(b) T. Matsuo, S. Hashimoto, K. Nishikawa, T. Kodama, S. Kikuchi, Y. Tachi and Y. Morimoto, Total synthesis and complete stereochemical assignment of heronapyrroles A and B, Tetrahedron Lett., 2015, 56, 5345–5348 CrossRef CAS.
-
(a) K. P. Garnock-Jones, Vonoprazan: First Global Approval, Drugs, 2015, 75, 439–443 CrossRef CAS PubMed;
(b) Y. Arikawa, H. Nishida, O. Kurasawa, A. Hasuoka, K. Hirase, N. Inatomi, Y. Hori, J. Matsukawa, A. Imanishi, M. Kondo, N. Tarui, T. Hamada, T. Takagi, T. Takeuchi and M. Kajino, Discovery of a Novel Pyrrole Derivative 1-[5-(2-Fluorophenyl)-1-(pyridin-3-ylsulfonyl)-1H-pyrrol-3-yl]-N-methylmethanamine Fumarate (TAK-438) as a Potassium-Competitive Acid Blocker (P-CAB), J. Med. Chem., 2012, 55, 4446–4456 CrossRef CAS PubMed.
- S. Mandl-Weber, F. G. Meinel, R. Jankowsky, F. Oduncu, R. Schmidmaier and P. Baumann, The novel inhibitor of histone deacetylase resminostat (RAS2410) inhibits proliferation and induces apoptosis in multiple myeloma (MM) cells, Br. J. Haematol., 2010, 149, 518–528 CrossRef CAS PubMed.
-
(a) I. V. Efimov, L. N. Kulikova, A. R. Miftyakhova, M. D. Matveeva and L. G. Voskressensky, Recent Advances for the Synthesis of N-Unsubstituted Pyrroles, ChemistrySelect, 2021, 6, 13740–13772 CrossRef CAS;
(b) S. C. Philkhana, F. O. Badmus, I. C. Dos Reis and R. Kartika, Recent Advancements in Pyrrole Synthesis, Synthesis, 2021, 1531–1555 CrossRef CAS PubMed;
(c) V. Estévez, M. Villacampa and J. C. Menéndez, Recent advances in the synthesis of pyrroles by multicomponent reactions, Chem. Soc. Rev., 2014, 43, 4633–4657 RSC;
(d) M. A. Yurovskaya and R. S. Alekseyev, New Perspectives on Classical Heterocyclic Reactions Involving Pyrrole Derivatives, Chem. Heterocycl. Compd., 2014, 49, 1400–1425 CrossRef CAS.
- M. K. Hunjan, S. Panday, A. Gupta, J. Bhaumik, P. Das and J. K. Laha, Recent Advances in Functionalization of Pyrroles and their Translational Potential, Chem. Rec., 2021, 21, 715–780 CrossRef CAS PubMed.
-
(a) E. M. Beck and M. J. Gaunt, Pd-catalyzed C-H bond functionalization on the indole and pyrrole nucleus, Top. Curr. Chem., 2010, 292, 85–121 CrossRef CAS PubMed;
(b) M. G. Banwell, T. E. Goodwin, S. Ng, J. A. Smith and D. J. Wong, Palladium-Catalysed Cross-Coupling and Related Reactions Involving Pyrroles, Eur. J. Org. Chem., 2006, 3043–3060 CrossRef CAS.
-
(a) H. M. Gilow and D. E. Burton, Bromination and chlorination of pyrrole and some reactive 1-substituted pyrroles, J. Org. Chem., 1981, 46, 2221–2225 CrossRef CAS;
(b) M. De Rosa, Acid catalyzed chlorination of pyrrole with N-chloroacetanilide, J. Heterocycl. Chem., 1982, 19, 1585 CrossRef CAS.
-
(a) G. A. Cordell, 2-Halopyrroles. Synthesis and chemistry, J. Org. Chem., 1975, 40, 3161–3169 CrossRef CAS;
(b) V. Leen, E. Braeken, K. Luckermans, C. Jackers, M. van der Auweraer, N. Boens and W. Dehaen, A versatile, modular synthesis of monofunctionalized BODIPY dyes, Chem. Commun., 2009, 4515–4517 RSC.
-
(a) F. Tutino, G. Papeo and F. Quartieri, Acid catalyzed halogen dance on deactivated pyrroles, J. Heterocycl. Chem., 2010, 47, 112–117 CrossRef CAS;
(b) E. Dvornikova and K. Kamieńska-Trela, Synthesis of 2- and 3-Substituted N-Methylpyrroles, Synlett, 2002, 1152–1154 CrossRef CAS.
-
(a) P. Belanger, Electrophilic substitutions on 2-trichloroacetylpyrrole, Tetrahedron Lett., 1979, 20, 2505–2508 CrossRef;
(b) P. E. Sonnet, Preparation and properties of ternary iminium salts of pyrrole aldehydes and ketones. Synthesis of 4-substituted pyrrole-2-carboxaldehydes, J. Org. Chem., 1972, 37, 925–929 CrossRef CAS;
(c) O. Castillo-Aguilera, P. Depreux, L. Halby, N. Azaroual, P. B. Arimondo and L. Goossens, Regioselective and efficient halogenation of 4,5-unsubstituted alkyl 3-hydroxypyrrole/3-hydroxythiophene-2-yl-carboxylates, Tetrahedron Lett., 2017, 58, 2537–2541 CrossRef CAS;
(d) S. Gao, T. K. Bethel, T. Kakeshpour, G. E. Hubbell, J. E. Jackson and J. J. Tepe, Substrate Controlled Regioselective Bromination of Acylated Pyrroles Using Tetrabutylammonium Tribromide (TBABr3), J. Org. Chem., 2018, 83, 9250–9255 CrossRef CAS PubMed.
-
(a) P. Hernández-Lladó, K. Garrec, D. C. Schmitt and J. W. Burton, Transition Metal-Free, Visible Light-Mediated Radical Cyclisation of Malonyl Radicals onto 5-Ring Heteroaromatics, Adv. Synth. Catal., 2022, 364, 1724–1731 CrossRef;
(b) R. F. Yilmaz, Y. Derin, B. A. Misir, V. E. Atalay, Ö.F Tutar, S. Ökten and A. Tutar, Synthesis and spectral properties of symmetrically arylated BODIPY dyes: Experimental and computational approach, J. Mol. Struct., 2023, 1291, 135962 CrossRef CAS;
(c) W. Chen and M. P. Cava, Convenient synthetic equivalents of 2-lithiopyrrole and 2,5-dilithiopyrrole, Tetrahedron Lett., 1987, 28, 6025–6026 CrossRef CAS.
- W. Chen, E. K. Stephenson, M. P. Cava and Y. A. Jackson, 2-Substituted Pyrroles From N-tert-Butoxycarbonyl-2-bromopyrrole: N-tert-Butoxycarbonyl-2-trimethylsilylpyrrole, Org. Synth., 1992, 70, 151 CrossRef CAS.
-
(a) D. W. Cho, M. Fujitsuka, J. H. Ryu, M. H. Lee, H. K. Kim, T. Majima and C. Im, S2 emission from chemically modified BODIPYs, Chem. Commun., 2012, 48, 3424–3426 RSC;
(b) J. H. Ryu, Y. K. Eom, J.-C. G. Bünzli and H. K. Kim, Ln(III)-cored complexes based on boron dipyrromethene (Bodipy) ligands for NIR emission, New J. Chem., 2012, 36, 723–731 RSC;
(c) L. Knight, J. Huffman and M. Isherwood, 2-Bromo-N-(p-toluenesulfonyl)pyrrole: A Robust Derivative of 2-Bromopyrrole, Synlett, 2003, 1993–1996 CAS;
(d) T. Koike, Y. Shinohara, T. Nishimura, M. Hagiwara, S. Tobinaga and N. Takeuchi, Condensation Reactions of a Nitrodienamine with Organocopper and Alkyllithium Reagents Prepared from Pyrrole Derivatives, Heterocycles, 2000, 53, 1351–1359 CrossRef CAS.
-
(a) T. Okumi, A. Mori and K. Okano, Regiocontrolled halogen dance of 2,5-dibromopyrroles using equilibrium between dibromopyrrolyllithiums, Chem. Commun., 2023, 59, 1046–1049 RSC;
(b)
L. Chumakova, A. Patron, C. Priest, D. S. Karanewsky, R. Kimmich, B. C. Boren, J. R. Hammaker, V. Chumakov, W. Zhao, A. Noncovich and J. Ung, Compounds useful as modulators of TRPM8, World Pat, WO2014130582, 2014 Search PubMed;
(c) C. Zonta, F. Fabris and O. de Lucchi, The Pyrrole Approach toward the Synthesis of Fully Functionalized Cup-Shaped Molecules, Org. Lett., 2005, 7, 1003–1006 CrossRef CAS PubMed.
-
(a) B. L. Bray, P. H. Mathies, R. Naef, D. R. Solas, T. T. Tidwell, D. R. Artis and J. M. Muchowski,
N-(Triisopropylsilyl)pyrrole. A progenitor “par excellence” of 3-substituted pyrroles, J. Org. Chem., 1990, 55, 6317–6328 CrossRef CAS;
(b) J. Leroy, E. Porhiel and A. Bondon, Synthesis and characterization of partially β-fluorinated 5,10,15,20-tetraphenylporphyrins and some derivatives, Tetrahedron, 2002, 58, 6713–6722 CrossRef CAS;
(c) K. Billingsley and S. L. Buchwald, Highly Efficient Monophosphine-Based Catalyst for the Palladium-Catalyzed Suzuki−Miyaura Reaction of Heteroaryl Halides and Heteroaryl Boronic Acids and Esters, J. Am. Chem. Soc., 2007, 129, 3358–3366 CrossRef CAS PubMed;
(d)
F. J. Lopez-Tapia, L. E. Lowrie Jr and D. Nitzan, Arylsulfonyl pyrrolidines as 5-HT6 inhibitors, World Pat, WO2008055847, 2008 Search PubMed.
- For selected examples, see:
(a) Y. Okui, Y. Yasuda, A. Mori and K. Okano, Total Synthesis of Lamellarins U and A3 by Interrupting Halogen Dance, Synthesis, 2022, 2647–2660 CAS;
(b) K. Cui, M. Gao, H. Zhao, D. Zhang, H. Yan and H. Huang, An Efficient Synthesis of Aryl-Substituted Pyrroles by the Suzuki–Miyaura Coupling Reaction of SEM-Protected Pyrroles, Molecules, 2019, 24, 1594 CrossRef CAS PubMed;
(c) M. A. Schafroth, S. M. Rummelt, D. Sarlah and E. M. Carreira, Enantioselective Iridium-Catalyzed Allylic Cyclizations, Org. Lett., 2017, 19, 3235–3238 CrossRef CAS PubMed;
(d) S. J. Mishra, S. Ghosh, A. R. Stothert, C. A. Dickey and B. S. J. Blagg, Transformation of the Non-Selective Aminocyclohexanol-Based Hsp90 Inhibitor into a Grp94-Seletive Scaffold, ACS Chem. Biol., 2017, 12, 244–253 CrossRef CAS PubMed;
(e) R. B. Alnoman, S. Rihn, D. C. O'Connor, F. A. Black, B. Costello, P. G. Waddell, W. Clegg, R. D. Peacock, W. Herrebout, J. G. Knight and M. J. Hall, Circularly Polarized Luminescence from Helically Chiral N,N,O,O-Boron-Chelated Dipyrromethenes, Chem. – Eur. J., 2016, 22, 93–96 CrossRef CAS PubMed;
(f) J. Thireau, J. Marteaux, P. Delagrange, F. Lefoulon, L. Dufourny, G. Guillaumet and F. Suzenet, Original Design of Fluorescent Ligands by Fusing BODIPY and Melatonin Neurohormone, ACS Med. Chem. Lett., 2014, 5, 158–161 CrossRef CAS PubMed;
(g) R. Y. Huang, P. T. Franke, N. Nicolaus and M. Lautens, Domino C–H functionalization reactions of gem-dibromoolefins: synthesis of N-fused benzo[c]carbazoles, Tetrahedron, 2013, 69, 4395–4402 CrossRef CAS;
(h) P. Kancharla and K. A. Reynolds, Synthesis of 2,2′-bipyrrole-5-carboxaldehydes and their application in the synthesis of B-ring functionalized prodiginines and tambjamines, Tetrahedron, 2013, 69, 8375–8385 CrossRef CAS;
(i) N. Sakamoto, C. Ikeda, M. Yamamura and T. Nabeshima, α-Bridged BODIPY oligomers with switchable near-IR photoproperties by external-stimuli-induced foldamer formation and disruption, Chem. Commun., 2012, 48, 4818–4820 RSC;
(j) H. K. Tanui, E. Hao, M. I. Ihachi, F. R. Fronczek, K. M. Smith and M. G. H. Vicente, Efficient synthesis and reactions of 1,2-dipyrrolylethynes, J. Porphyrins Phthalocyanines, 2011, 15, 412–420 CrossRef CAS PubMed;
(k) G. Barker, J. L. McGrath, A. Klapars, D. Stead, G. Zhou, K. R. Campos and P. O'Brien, Enantioselective, Palladium-Catalyzed α-Arylation of N-Boc Pyrrolidine: In Situ React IR Spectroscopic Monitoring, Scope, and Synthetic Applications, J. Org. Chem., 2011, 76, 5936–5953 CrossRef CAS PubMed;
(l) Y. Matano, M. Fujita, A. Saito and H. Imahori, Synthesis, structures, optical and electrochemical properties, and complexation of 2,5-bis(pyrrol-2-yl)phospholes, C. R. Chim., 2010, 13, 1035–1047 CrossRef CAS;
(m) A. A. Kanakis and V. Sarli, Total Synthesis of (±)-Marinopyrrole A via Copper-Mediated N-Arylation, Org. Lett., 2010, 12, 4872–4875 CrossRef CAS PubMed;
(n) A. Gagnon, M. Duplessis, P. Alsabeh and F. Barabé, Palladium-Catalyzed Cross-Coupling Reaction of Tricyclopropylbismuth with Aryl Halides and Triflates, J. Org. Chem., 2008, 73, 3604–3607 CrossRef CAS PubMed;
(o) A. Dinsmore, D. G. Billing, K. Mandy, J. P. Michael, D. Mogano and S. Patil, Magnesiation Employing Grignard Reagents and Catalytic Amine. Application to the Functionalization of N-Phenylsulfonylpyrrole, Org. Lett., 2004, 6, 293–296 CrossRef CAS PubMed;
(p) S. T. Handy, H. Bregman, J. Lewis, X. Zhang and Y. Zhang, An unusual dehalogenation in the Suzuki coupling of 4-bromopyrrole-2-carboxylates, Tetrahedron Lett., 2003, 44, 427–430 CrossRef CAS;
(q) H. Miyaji, W. Sato, J. L. Sessler and V. M. Lynch, A ‘building block’ approach to functionalized calix[4]pyrroles, Tetrahedron Lett., 2000, 41, 1369–1373 CrossRef CAS;
(r) L. H. Thoresen, H. Kim, M. B. Welch, A. Burghart and K. Burgess, Synthesis of 3,5-Diaryl-4,4-difluoro-4-bora-3a,4a-diaza-s-indacene (BODIPY®) Dyes, Synlett, 1998, 1276–1278 CrossRef CAS;
(s) P. A. Jacobi and S. Rajeswari, Tetrapyrroles. IV. A highly efficient synthesis of homochiral dihydropyrromethenones via Pd0 mediated coupling of iodopyrroles and acetylenic amides, Tetrahedron Lett., 1992, 33, 6235–6238 CrossRef CAS;
(t) S. Martina and A. D. Schlüter, Soluble polyarylenes with alternating sequences of alkyl-substituted phenylene and pyrrolic or terpyrrolic units, Macromolecules, 1992, 25, 3607–3608 CrossRef CAS.
- For selected examples, see:
(a) S. Doniz Kettenmann, M. White, J. Colard-Thomas, M. Kraft, A. T. Feßler, K. Danz, G. Wieland, S. Wagner, S. Schwarz, A. Wiehe and N. Kulak, Investigating Alkylated Prodigiosenes and Their Cu(II)-Dependent Biological Activity: Interactions with DNA, Antimicrobial and Photoinduced Anticancer Activity, ChemMedChem, 2022, 17, e202100702 CrossRef CAS PubMed;
(b) E. Marchal, D. A. Smithen, M. I. Uddin, A. W. Robertson, D. L. Jakeman, V. Mollard, C. D. Goodman, K. S. MacDougall, S. A. McFarland, G. I. McFadden and A. Thompson, Synthesis and antimalarial activity of prodigiosenes, Org. Biomol. Chem., 2014, 12, 4132–4142 RSC;
(c) I. Kholod, O. Vallat, A.-M. Buciumas, A. Neels and R. Neier, Eur. J. Org. Chem., 2014, 7865–7877 CrossRef CAS;
(d) C. Minard, C. Palacio, K. Cariou and R. H. Dodd, Selective Suzuki–Miyaura Monocouplings with Symmetrical Dibromoarenes and Aryl Ditriflates for the One-Pot Synthesis of Unsymmetrical Triaryls, Eur. J. Org. Chem., 2014, 2942–2955 CrossRef CAS;
(e) H. Kamiyama, Y. Kubo, H. Sato, N. Yamamoto, T. Fukuda, F. Ishibashi and M. Iwao, Synthesis, structure–activity relationships, and mechanism of action of anti-HIV-1 lamellarin α 20-sulfate analogues, Bioorg. Med. Chem., 2011, 19, 7541–7550 CrossRef CAS PubMed;
(f) K. Yoshida, K. Hayashi and A. Yanagisawa, Construction of Carbocyclic Ring of Indoles Using Ruthenium-Catalyzed Ring-Closing Olefin Metathesis, Org. Lett., 2011, 13, 4762–4765 CrossRef CAS PubMed;
(g) S. Hirao, Y. Yoshinaga, M. Iwao and F. Ishibashi, A formal total synthesis of the telomerase inhibitor dictyodendrin B, A General Method for the Synthesis of N-Unsubstituted 3,4-Diarylpyrrole-2,5-dicarboxylates, Tetrahedron Lett., 2010, 51, 533–536 CrossRef CAS;
(h) T. Fukuda, Y. Hayashida and M. Iwao, A General Method for the Synthesis of N-Unsubstituted 3,4-Diarylpyrrole-2,5-dicarboxylates, Heterocycles, 2009, 77, 1105–1122 CrossRef CAS PubMed;
(i) J. T. Reeves, A Concise Synthesis of Butylcycloheptylprodigiosin, Org. Lett., 2007, 9, 1879–1881 CrossRef CAS PubMed;
(j) J. T. Tomlinson, G. Park, J. A. Misenheimer, G. L. Kucera, K. Hesp and R. A. Manderville, Photoinduced Cytotoxicity and Thioadduct Formation by a Prodigiosin Analogue, Org. Lett., 2006, 8, 4951–4954 CrossRef CAS PubMed;
(k) A. Fürstner, K. Radkowski and H. Peters, Chasing a Phantom by Total Synthesis: The Butylcycloheptylprodigiosin Case, Angew. Chem., Int. Ed., 2005, 44, 2777–2781 CrossRef PubMed;
(l) A. Fürstner, J. Grabowski and C. W. Lehmann, Total Synthesis and Structural Refinement of the Cyclic Tripyrrole Pigment Nonylprodigiosin, J. Org. Chem., 1999, 64, 8275–8280 CrossRef PubMed;
(m) R. D'Alessio and A. Rossi, Short synthesis of undecylprodigiosine. A new route to 2,2′-bipyrrolyl-pyrromethene systems, Synlett, 1996, 513–514 CrossRef.
- For selected examples, see:
(a) L. Filippini, M. Gusmeroli and R. Riva, Palladium-catalyzed cross-coupling of pyrrolyl anions with organic halides, Tetrahedron Lett., 1992, 33, 1755–1758 CrossRef CAS;
(b) R. D. Rieth, N. P. Mankad, E. Calimano and J. P. Sadighi, Palladium-Catalyzed Cross-Coupling of Pyrrole Anions with Aryl Chlorides, Bromides, and Iodides, Org. Lett., 2004, 6, 3981–3983 CrossRef CAS PubMed;
(c) X. Wang, B. S. Lane and D. Sames, Direct C-Arylation of Free (NH)-Indoles and Pyrroles Catalyzed by Ar−Rh(III) Complexes Assembled In Situ, J. Am. Chem. Soc., 2005, 127, 4996–4997 CrossRef CAS PubMed;
(d) N. R. Deprez, D. Kalyani, A. Krause and M. S. Sanford, Room Temperature Palladium-Catalyzed 2-Arylation of Indoles, J. Am. Chem. Soc., 2006, 128, 4972–4973 CrossRef CAS PubMed;
(e) P. Forgione, M.-C. Brochu, M. St-Onge, K. H. Thesen, M. D. Bailey and F. Bilodeau, Unexpected Intermolecular Pd-Catalyzed Cross-Coupling Reaction Employing Heteroaromatic Carboxylic Acids as Coupling Partners, J. Am. Chem. Soc., 2006, 128, 11350–11351 CrossRef CAS PubMed;
(f) B. Liégault, D. Lapointe, L. Caron, A. Vlassova and K. Fagnou, Establishment of Broadly Applicable Reaction Conditions for the Palladium-Catalyzed Direct Arylation of Heteroatom-Containing Aromatic Compounds, J. Org. Chem., 2009, 74, 1826–1834 CrossRef PubMed;
(g) D. T. Gryko, O. Vakuliuk, D. Gryko and B. Koszarna, Palladium-Catalyzed 2-Arylation of Pyrroles, J. Org. Chem., 2009, 74, 9517–9520 CrossRef CAS PubMed;
(h) F. Jafarpour, S. Rahiminejadan and H. Hazrati, Triethanolamine-Mediated Palladium-Catalyzed Regioselective C-2 Direct Arylation of Free NH-Pyrroles, J. Org. Chem., 2010, 75, 3109–3112 CrossRef CAS PubMed;
(i) A. García-Rubia, B. Urones, R. Gómez Arrayás and J. C. Carretero, PdII-Catalysed C–H Functionalisation of Indoles and Pyrroles Assisted by the Removable N,-(2-Pyridyl)sulfonyl Group: C2-Alkenylation and Dehydrogenative Homocoupling, Chem. – Eur. J., 2010, 16, 9676–9685 CrossRef PubMed;
(j) E. T. Nadres, A. Lazareva and O. Daugulis, Palladium-Catalyzed Indole, Pyrrole, and Furan Arylation by Aryl Chlorides, J. Org. Chem., 2011, 76, 471–483 CrossRef CAS PubMed;
(k) N. Thies, C. G. Hrib and E. Haak, Ruthenium-Catalyzed Functionalization of Pyrroles and Indoles with Propargyl Alcohols, Chem. – Eur. J., 2012, 18, 6302–6308 CrossRef CAS PubMed;
(l) Y. Xu, L. Zhao, Y. Li and H. Doucet, Palladium-Catalysed Regioselective Sequential C-5 and C-2 Direct Arylations of 3-Acetylpyrroles with Aryl Bromides, Adv. Synth. Catal., 2013, 355, 1423–1432 CrossRef CAS;
(m) R. Jin, K. Yuan, E. Chatelain, J.-F. Soulé and H. Doucet, Palladium-Catalysed Direct Desulfitative Arylation of Pyrroles using Benzenesulfonyl Chlorides as Alternative Coupling Partners, Adv. Synth. Catal., 2014, 356, 3831–3841 CrossRef CAS;
(n) W. Chen, H.-J. Li, Y.-F. Cheng and Y.-C. Wu, Direct C2-arylation of N-acyl pyrroles with aryl halides under palladium catalysis, Org. Biomol. Chem., 2021, 19, 1555–1564 RSC;
(o) M. Petkovic, M. Jovanovic, P. Jovanovic, M. Simic, G. Tasic and V. Savic, Dual Role of the Arylating Agent in a Highly C(II)-Selective Pd-Catalysed Functionalisation of Pyrrole Derivatives, Synthesis, 2022, 2839–2848 CrossRef CAS;
(p) W. Chen and M. Liu, A Highly Active Palladium System Catalyzed C2-Arylation of N-Acyl Pyrroles with Aryl Halides and Their Biological Activity, Asian J. Org. Chem., 2023, 12, e202200648 CrossRef;
(q) N. Munir, N. Gürbüz, M. N. Zafar, E. Evren, B. Şen, M. Aygün and İ. Özdemir, Plausible PEPPSI catalysts for direct C-H functionalization of furans and pyrroles, J. Mol. Struct., 2024, 1295, 136679 CrossRef CAS;
(r) K. Jiang, X. Li, L. Wang and J. Han, Basicity-Controlled Reactivity of meta-OTf-Substituted Diaryliodonium Salts for Direct Arylation or Diels-Alder Cycloaddition of Pyrrole Derivatives, Asian J. Org. Chem., 2024, 13, e202400139 CrossRef CAS;
(s) J. Märsch, S. Reiter, T. Rittner, R. E. Rodriguez-Lugo, M. Whitfield, D. J. Scott, R. J. Kutta, P. Nuernberger, R. de Vivie-Riedle and R. Wolf, Cobalt-Mediated Photochemical C−H Arylation of Pyrroles, Angew. Chem., Int. Ed., 2024, 63, e202405780 CrossRef PubMed.
-
(a) C. Savarin, J. Srogl and L. S. Liebeskind, Thiol Ester−Boronic Acid Cross-Coupling. Catalysis Using Alkylative Activation of the Palladium Thiolate Intermediate, Org. Lett., 2000, 2, 3229–3231 CrossRef CAS PubMed;
(b) S. Zhang, D. Marshall and L. S. Liebeskind, Efficient Pd-Catalyzed Heterobenzylic Cross-Coupling Using Sulfonium Salts as Substrates and (PhO)3P as a Supporting Ligand, J. Org. Chem., 1999, 64, 2796–2804 CrossRef CAS PubMed;
(c) J. Srogl, G. D. Allred and L. S. Liebeskind, Sulfonium Salts. Participants par Excellence in Metal-Catalyzed Carbon−Carbon Bond-Forming Reactions, J. Am. Chem. Soc., 1997, 119, 12376–12377 CrossRef CAS.
- For selected examples, see:
(a) J.-W. Song, F. Xia, X.-L. Zhang and C.-P. Zhang, Pd-catalyzed relay Heck arylation of alkenyl alcohols with arylsulfonium salts, Org. Chem. Front., 2024, 11, 4219–4230 RSC;
(b) S. Ni, J. Yan, S. Tewari, E. J. Reijerse, T. Ritter and J. Cornella, Nickel Meets Aryl Thianthrenium Salts: Ni(I)-Catalyzed Halogenation of Arenes, J. Am. Chem. Soc., 2023, 145, 9988–9993 CrossRef CAS PubMed;
(c) M. Gao and C. Gosmini, Co-Catalyzed Reductive Cross-Couplings of Csp3−S with Callyl−O Electrophiles to form Csp3−Csp3 Bonds, Adv. Synth. Catal., 2023, 365, 3597–3602 CrossRef CAS;
(d) Y. Ye, J. Zhu, H. Xie and Y. Huang, Rhodium-Catalyzed Divergent Arylation of Alkenylsulfonium Salts with Arylboroxines, Angew. Chem., Int. Ed., 2022, 61, e202212522 CrossRef CAS PubMed;
(e) W. Wang, K. Yao and F. Wu, Nickel-Catalyzed Reductive Cross-Coupling of Benzylic Sulfonium Salts with Aryl Iodides, Synlett, 2022, 361–366 Search PubMed;
(f) N.-N. Ma, J.-A. Ren, X. Liu, X.-Q. Chu, W. Rao and Z.-L. Shen, Nickel-Catalyzed Direct Cross-Coupling of Aryl Sulfonium Salt with Aryl Bromide, Org. Lett., 2022, 24, 1953–1957 CrossRef CAS PubMed;
(g) C. Chen, M. Wang, H. Lu, B. Zhao and Z. Shi, Enabling the Use of Alkyl Thianthrenium Salts in Cross-Coupling Reactions by Copper Catalysis, Angew. Chem., Int. Ed., 2021, 60, 21756–21760 CrossRef CAS PubMed;
(h) A. Selmani, A. G. Gevondian and F. Schoenebeck, Transition-Metal-Free, Formal C–H Germylation of Arenes and Styrenes via Dibenzothiophenium Salts, Org. Lett., 2021, 23, 4779–4784 CrossRef CAS PubMed;
(i) Y. Ye, J. Zhu and Y. Huang, Diverse C–P Cross-Couplings of Arylsulfonium Salts with Diarylphosphines via Selective C–S Bond Cleavage, Org. Lett., 2021, 23, 2386–2391 CrossRef CAS PubMed;
(j) Y. Wu, Y.-H. Huang, X.-Y. Chen and P. Wang, Site-Selective Silylation of Arenes Mediated by Thianthrene S-Oxide, Org. Lett., 2020, 22, 6657–6661 CrossRef CAS PubMed;
(k) A. Selmani, A. G. Gevondian and F. Schoenebeck, Germylation of Arenes via Pd(I) Dimer Enabled Sulfonium Salt Functionalization, Org. Lett., 2020, 22, 4802–4805 CrossRef CAS PubMed;
(l) J. Wu, Z. Wang, X.-Y. Chen, Y. Wu, D. Wang, Q. Peng and P. Wang,
Para-selective borylation of monosubstituted benzenes using a transient mediator, Sci. China: Chem., 2020, 63, 336–340 CrossRef CAS;
(m) K. Kafuta, A. Korzun, M. Böhm, C. Golz and M. Alcarazo, Synthesis, Structure, and Reactivity of 5-(Aryl)dibenzothiophenium Triflates, Angew. Chem., Int. Ed., 2020, 59, 1950–1955 CrossRef CAS PubMed;
(n) T. Yanagi, R. J. Somerville, K. Nogi, R. Martin and H. Yorimitsu, Ni-Catalyzed Carboxylation of C(sp2)—S Bonds with CO2: Evidence for the Multifaceted Role of Zn, ACS Catal., 2020, 10, 2117–2123 CrossRef CAS;
(o) Z.-Y. Tian and C.-P. Zhang, Ullmann-type N-arylation of anilines with alkyl(aryl)sulfonium salts, Chem. Commun., 2019, 55, 11936–11939 RSC;
(p) H. Minami, K. Nogi and H. Yorimitsu, Palladium-Catalyzed Alkoxycarbonylation of Arylsulfoniums, Org. Lett., 2019, 21, 2518–2522 CrossRef CAS PubMed;
(q) H. Minami, K. Nogi and H. Yorimitsu, Palladium-Catalyzed Homo-Coupling of Heteroarylsulfoniums via Borylation/Suzuki-Miyaura Coupling Sequence, Heterocycles, 2018, 97, 998–1007 CrossRef CAS PubMed;
(r) M. H. Aukland, F. J. T. Talbot, J. A. Fernandez-Salas, M. Ball, A. P. Pulis and D. J. Procter, An Interrupted Pummerer/Nickel-Catalysed Cross-Coupling Sequence, Angew. Chem., Int. Ed., 2018, 57, 9785–9789 CrossRef CAS PubMed;
(s) Z.-Y. Tian, S.-M. Wang, S.-J. Jia, H.-X. Song and C.-P. Zhang, Sonogashira Reaction Using Arylsulfonium Salts as Cross-Coupling Partners, Org. Lett., 2017, 19, 5454–5457 CrossRef CAS PubMed;
(t) H. Kawashima, T. Yanagi, C.-C. Wu, K. Nogi and H. Yorimitsu, Regioselective C–H Sulfanylation of Aryl Sulfoxides by Means of Pummerer-Type Activation, Org. Lett., 2017, 19, 4552–4555 CrossRef CAS PubMed;
(u) S.-M. Wang, H.-X. Song, X.-Y. Wang, N. Liu, H.-L. Qin and C.-P. Zhang, Palladium-catalyzed Mizoroki–Heck-type reactions of [Ph2SRfn][OTf] with alkenes at room temperature, Chem. Commun., 2016, 52, 11893–11896 RSC;
(v) D. Vasu, H. Yorimitsu and A. Osuka, Base-Free Palladium-Catalyzed Cross-Coupling of Arylsulfonium Salts with Sodium Tetraarylborates, Synthesis, 2015, 3286–3291 CAS;
(w) D. Vasu, H. Yorimitsu and A. Osuka, Palladium-Assisted “Aromatic Metamorphosis” of Dibenzothiophenes into Triphenylenes, Angew. Chem., Int. Ed., 2015, 54, 7162–7166 CrossRef CAS PubMed;
(x) M.-L. Xu and W. Huang, Metal-free carbon–carbon cross-couplings between the ion pairs in sulfonium tetraphenylborates, Tetrahedron Lett., 2014, 55, 4230–4232 CrossRef CAS;
(y) H. Lin, X. Dong, Y. Li, Q. Shen and L. Lu, Highly Selective Activation of Vinyl C–S Bonds Over Aryl C–S Bonds in the Pd-Catalyzed Coupling of (E)-(β-Trifluoromethyl)vinyldiphenylsulfonium Salts: Preparation of Trifluoromethylated Alkenes and Dienes, Eur. J. Org. Chem., 2012, 4675–4679 CrossRef CAS;
(z) C. Vanier, F. Lorge, A. Wagner and C. Mioskowski, Traceless, Solid-Phase Synthesis of Biarylmethane Structures through Pd-Catalyzed Release of Supported Benzylsulfonium Salts, Angew. Chem., Int. Ed., 2000, 39, 1679–1683 CrossRef CAS.
- F. Berger, M. B. Plutschack, J. Riegger, W. Yu, S. Speicher, M. Ho, N. Frank and T. Ritter, Site-selective and versatile aromatic C−H functionalization by thianthrenation, Nature, 2019, 567, 223–228 CrossRef CAS PubMed.
- For reviews, see:
(a) S. Timmann, Z. Feng and M. Alcarazo, Recent Applications of Sulfonium Salts in Synthesis and Catalysis, Chem. – Eur. J., 2024, 30, e202402768 CrossRef PubMed;
(b) X. Wu, P. Gao and F. Chen, Synthetic Applications of Sulfonium Salts as Aryl Radical Precursors, Eur. J. Org. Chem., 2023, e202300864 CrossRef CAS;
(c) L. van Dalsen, R. E. Brown, J. A. Rossi-Ashton and D. J. Procter, Sulfonium Salts as Acceptors in Electron Donor-Acceptor Complexes, Angew. Chem., Int. Ed., 2023, 62, e202303104 CrossRef CAS PubMed;
(d) Z.-Y. Tian, Y. Ma and C.-P. Zhang, Alkylation Reactions with Alkylsulfonium Salts, Synthesis, 2022, 1478–1502 CAS;
(e) G. J. P. Perry and H. Yorimitsu, Sulfur(IV) in Transition-Metal-Free Cross-Couplings for Biaryl Synthesis, ACS Sustainable Chem. Eng., 2022, 10, 2569–2586 CrossRef CAS;
(f) Q.-Z. Li, W.-L. Zou, Z.-Q. Jia and J.-L. Li, Recent Advances on Annulation Reactions with Allyl and Propargyl Sulfonium Salts, Synthesis, 2022, 67–78 CrossRef;
(g) H. Xu, J. Zhang, J. Zuo, F. Wang, J. Lü, X. Hun and D. Yang, Recent Advances in Visible-Light-Catalyzed C—C Bonds and C—Heteroatom Bonds Formation Using Sulfonium Salts, Chin. J. Org. Chem., 2022, 42, 4037–4059 CrossRef CAS;
(h) R. Melngaile and J. Veliks, Synthetic Applications of Monofluoromethylsulfonium Salts, Synthesis, 2021, 4549–4558 CAS;
(i) R. Fan, C. Tan, Y. Liu, Y. Wei, X. Zhao, X. Liu, J. Tan and H. Yoshida, A leap forward in sulfonium salt and sulfur ylide chemistry, Chin. Chem. Lett., 2021, 32, 299–312 CrossRef CAS;
(j) H. Yorimitsu, Catalytic Transformations of Sulfonium Salts via C—S Bond Activation, Chem. Rec., 2021, 21, 3356–3369 CrossRef CAS PubMed;
(k) S. I. Kozhushkov and M. Alcarazo, Synthetic Applications of Sulfonium Salts, Eur. J. Inorg. Chem., 2020, 2486–2500 CrossRef CAS PubMed;
(l) Á. Péter, G. J. P. Perry and D. J. Procter, Radical C−C Bond Formation using Sulfonium Salts and Light, Adv. Synth. Catal., 2020, 362, 2135–2142 CrossRef;
(m) D. Kaiser, I. Klose, R. Oost, J. Neuhaus and N. Maulide, Bond-Forming and -Breaking Reactions at Sulfur(IV): Sulfoxides, Sulfonium Salts, Sulfur Ylides, and Sulfinate Salts, Chem. Rev., 2019, 119, 8701–8780 CrossRef CAS PubMed;
(n) Z.-Y. Tian, Y.-T. Hu, H.-B. Teng and C.-P. Zhang, Application of arylsulfonium salts as arylation reagents, Tetrahedron Lett., 2018, 59, 299–309 CrossRef CAS.
-
(a) P. Cowper, Y. Jin, M. D. Turton, G. Kociok-Köhn and S. E. Lewis, Azulenesulfonium Salts: Accessible, Stable, and Versatile Reagents for Cross-Coupling, Angew. Chem., Int. Ed., 2016, 55, 2564–2568 CrossRef CAS PubMed;
(b) C. M. López-Alled, F. J. O. Martin, K.-Y. Chen, G. Kociok-Köhn, T. D. James, J. Wenk and S. E. Lewis, Azulenesulfonium and azulenebis(sulfonium) salts: Formation by interrupted Pummerer reaction and subsequent derivatisation by nucleophiles, Tetrahedron, 2020, 76, 131700 CrossRef.
-
(a) F. Franco, R. Greenhouse and J. M. Muchowski, Novel syntheses of 5-aroyl-1,2-dihydro-3H-pyrrolo[1,2-a]pyrrole-1-carboxylic acids, J. Org. Chem., 1982, 47, 1682–1688 CrossRef CAS;
(b) K. Hartke and D. Strangemann, Sulfonioindolides and Sulfoniopyrrolides, Heterocycles, 1986, 24, 2399–2402 CrossRef CAS;
(c) K. Hartke, D. Teuber and H.-D. Gerber, Indole- and pyrrole-sulfonium ylides, Tetrahedron, 1988, 44, 3261–3270 CrossRef CAS;
(d) H. H. Wendebourg and K. Hartke, 3-Pyrrolylsulfonium Salts and 3-Pyrrolylsulfonium Ylides, Synthesis, 1989, 329–331 CrossRef CAS;
(e) D. K. Bates, R. T. Winters and J. A. Picard, Intramolecular capture of Pummerer rearrangement intermediates. 3. Interrupted Pummerer rearrangement: capture of tricoordinate sulfur species generated under Pummerer rearrangement conditions, J. Org. Chem., 1992, 57, 3094–3097 CrossRef CAS;
(f) F. Bellesia, F. Ghelfi, R. Grandi, U. M. Pagnoni and A. Pinetti, The reaction of pyrroles with trimethylhalosilanes-dialkyl sulfoxides, J. Heterocycl. Chem., 1993, 30, 617–621 CrossRef CAS;
(g) D. K. Bates and K. A. Tafel, Sulfenylation Using Sulfoxides. Intramolecular Cyclization of 2- and 3-Acylpyrroles, J. Org. Chem., 1994, 59, 8076–8080 CrossRef CAS;
(h) J. A. Picard, S. Chen and D. K. Bates, Intramolecular Sulfenylation Using Sulfoxides. Preparation of 5H-Pyrrolo[1,2-a][3,1]benzothiazines, Heterocycles, 1994, 38, 1775–1789 CrossRef CAS;
(i) N. E. Shevchenko, A. S. Karpov, E. P. Zakurdaev, V. G. Nenajdenko and E. S. Balenkova, Synthesis of methylthiosubstituted heterocycles using the complex of trifluoromethanesulfonic anhydride with dimethyl sulfide, Chem. Heterocycl. Compd., 2000, 36, 137–143 CrossRef CAS;
(j) N. E. Shevchenko, V. G. Nenajdenko and E. S. Balenkova, Triflic Anhydride-Promoted Cyclization of Sulfides: A Convenient Synthesis of Fused Sulfur Heterocycles, Synthesis, 2003, 1191–1200 CAS;
(k) A. Thompson, R. J. Butler, M. N. Grundy, A. B. E. Laltoo, K. N. Robertson and T. S. Cameron, Sulfur-Based Protecting Groups for Pyrroles and the Facile Deprotection of 2-(2,4-Dinitrobenzene)sulfinyl and Sulfonyl
Pyrroles, J. Org. Chem., 2005, 70, 3753–3756 CrossRef CAS PubMed;
(l) L. M. Yagupolskii, A. V. Matsnev, R. K. Orlova, B. G. Deryabkin and Y. L. Yagupolskii, A new method for the synthesis of trifluoromethylating agents—Diaryltrifluoromethylsulfonium salts, J. Fluorine Chem., 2008, 129, 131–136 CrossRef CAS;
(m) J. Kobayashi, S.-Y. Nakafuji, A. Yatabe and T. Kawashima, A novel ylide-stabilized carbene; formation and electron donating ability of an amino(sulfur-ylide)carbene, Chem. Commun., 2008, 6233–6235 RSC;
(n) A. J. Eberhart, C. Cicoira and D. J. Procter, Nucleophilic ortho-Allylation of Pyrroles and Pyrazoles: An Accelerated Pummerer/Thio-Claisen Rearrangement Sequence, Org. Lett., 2013, 15, 3994–3997 CrossRef CAS PubMed;
(o) H. Steinert, C. Schwarz, A. Kroll and V. H. Gessner, Towards the Preparation of Stable Cyclic Amino(ylide)Carbenes, Molecules, 2020, 25, 796 CrossRef CAS PubMed;
(p) D. Wang, C. G. Carlton, M. Tayu, J. J. W. McDouall, G. J. P. Perry and D. J. Procter, Trifluoromethyl Sulfoxides: Reagents for Metal-Free C−H Trifluoromethylthiolation, Angew. Chem., Int. Ed., 2020, 59, 15918–15922 CrossRef CAS PubMed;
(q) E. M. Alvarez, T. Karl, F. Berger, L. Torkowski and T. Ritter, Late-Stage Heteroarylation of Hetero(aryl)sulfonium Salts Activated by α-Amino Alkyl Radicals, Angew. Chem., Int. Ed., 2021, 60, 13609–13613 CrossRef CAS PubMed;
(r) W. Zhang, T. Liu, H. T. Ang, P. Luo, Z. Lei, X. Luo, M. J. Koh and J. Wu, Modular and Practical 1,2-Aryl(Alkenyl) Heteroatom Functionalization of Alkenes through Iron/Photoredox Dual Catalysis, Angew. Chem., Int. Ed., 2023, 62, e202310978 CrossRef CAS PubMed;
(s) Y. Guo, L. Wei, H. Jiang and C. Qi, Palladium-Catalyzed Carbonylation of Aryl Sulfonium Salts with CO2 as a CO Surrogate, Asian J. Org. Chem., 2024, 13, e202400142 CrossRef CAS.
- For reviews, see:
(a) Y. Liang and B. Peng, Revisiting Aromatic Claisen Rearrangement Using Unstable Aryl Sulfonium/Iodonium Species: The Strategy of Breaking Up the Whole into Parts, Acc. Chem. Res., 2022, 55, 2103–2122 CrossRef CAS PubMed;
(b) K. Higuchi and M. Tayu, The Interrupted Pummerer Reaction: Design of Sulfoxides and Their Utility in Organic Synthesis, Heterocycles, 2021, 102, 783–824 CrossRef CAS;
(c) X. Zhao, J. Zeng, L. Meng and Q. Wan, Application of Interrupted Pummerer Reaction Mediated (IPRm) Glycosylation in Natural Product Synthesis, Chem. Rec., 2020, 20, 743–751 CrossRef CAS PubMed;
(d) Z. He, A. P. Pulis, G. J. P. Perry and D. J. Procter, Pummerer chemistry of benzothiophene S-oxides: Metal-free alkylation and arylation of benzothiophenes, Phosphorus, Sulfur Silicon Relat. Elem., 2019, 194, 669–677 CrossRef CAS;
(e) T. Yanagi, K. Nogi and H. Yorimitsu, Recent development of ortho-C—H functionalization of aryl sulfoxides through [3,3] sigmatropic rearrangement, Tetrahedron Lett., 2018, 59, 2951–2959 CrossRef CAS;
(f) H. Yorimitsu, Cascades of Interrupted Pummerer Reaction-Sigmatropic Rearrangement, Chem. Rec., 2017, 17, 1156–1167 CrossRef CAS PubMed;
(g) L. H. S. Smith, S. C. Coote, H. F. Sneddon and D. J. Procter, Beyond the Pummerer Reaction: Recent Developments in Thionium Ion Chemistry, Angew. Chem., Int. Ed., 2010, 49, 5832–5844 CrossRef CAS PubMed.
- J. L. Hann, C. L. Lyall, G. Kociok-Köhn, C. Faverio, G. D. Pantoş and S. E. Lewis, Unusual Regio- and Chemoselectivity in Oxidation of Pyrroles and Indoles Enabled by a Thianthrenium Salt Intermediate, Angew. Chem., Int. Ed., 2024, 63, e202405057 CrossRef CAS PubMed.
-
(a) O. Carmona, R. Greenhouse, R. Landeros and J. M. Muchowski, Synthesis and rearrangement of 2-(arylsulfinyl)- and 2-(alkylsulfinyl)pyrroles, J. Org. Chem., 1980, 45, 5336–5339 CrossRef CAS;
(b) J. R. Carson and N. M. Davis, Acid-mediated rearrangement of acylpyrroles, J. Org. Chem., 1981, 46, 839–843 CrossRef CAS;
(c) J. DeSales, R. Greenhouse and J. M. Muchowski, Synthesis and rearrangement of pyrrolyl sulfides and sulfones, J. Org. Chem., 1982, 47, 3668–3672 CrossRef CAS;
(d) M. Kakushima, P. Hamel, R. Frenette and J. Rokach, Regioselective synthesis of acylpyrroles, J. Org. Chem., 1983, 48, 3214–3219 CrossRef CAS;
(e) M. C. Pina, V. A. Budilin, M. Rodrigues and Y. G. Bundel, Synthesis and prototropic isomerization of 1-nitrophenyl-2-acylpyrroles, Chem. Heterocycl. Compd., 1989, 25, 268–271 CrossRef;
(f) J.-H. Mirebeau, M. Haddad, M. Henry-Ellinger, G. Jaouen, J. Louvel and F. Le Bideau, Rearrangement of 2,5-Bis(silylated)-N-Boc Pyrroles into the Corresponding 2,4-Species, J. Org. Chem., 2009, 74, 8890–8892 CrossRef CAS PubMed.
- B. Altundas, S. Kumar and F. F. Fleming, Acetonitrile–Hexane Extraction Route to Pure Sulfonium Salts, ACS Omega, 2020, 5, 13384–13388 CrossRef CAS PubMed.
- For reviews, see:
(a) F. Berger and T. Ritter, Site-Selective Late-Stage C–H Functionalization via Thianthrenium Salts, Synlett, 2022, 339–345 CAS;
(b) X.-Y. Chen, Y. Wu and P. Wang, Recent Advances in Thianthrenation/Phenoxathiination Enabled Site-Selective Functionalization of Arenes, Synthesis, 2022, 3928–3940 CAS;
(c) H. Meng, M.-S. Liu and W. Shu, Organothianthrenium salts: synthesis and utilization, Chem. Sci., 2022, 13, 13690–13707 RSC;
(d) M. Kim, K. Targos, D. E. Holst, D. J. Wang and Z. K. Wickens, Alkene Thianthrenation Unlocks Diverse Cation Synthons: Recent Progress and New Opportunities, Angew. Chem., Int. Ed., 2024, 63, e202314904 CrossRef CAS PubMed.
- For selected examples, see:
(a) L. Zhang, Y. Xie, Z. Bai and T. Ritter, Suzuki–Miyaura coupling of arylthianthrenium tetrafluoroborate salts under acidic conditions, Nat. Synth., 2024 DOI:10.1038/s44160-024-00631-4;
(b) F. Jin, Q. Hu, Q. Wang, J. Sun, K. Huang, C.-G. Yan, Y. Han, H. Fei and L. Wang, Synthesis of Sulfoxides by Palladium-Catalyzed Arylation of Sulfenate Anions with Aryl Thianthrenium Salts, J. Org. Chem., 2024, 89, 13319–13328 CrossRef CAS PubMed;
(c) R. Kumar, A. Sharma and A. Sharma, Mechanochemically Induced Thianthrenium Salts-Based Arylation of Diverse Heterocyclic Scaffolds, ACS Sustainable Chem. Eng., 2024, 12, 12808–12818 CrossRef CAS;
(d) M. Jiao, J. Zhang, M. Wang, H. Lu and Z. Shi, Metallaphotoredox deuteroalkylation utilizing thianthrenium salts, Nat. Commun., 2024, 15, 5067 CrossRef CAS PubMed;
(e) G. Bao, X. Song, Y. Li, Z. He, Q. Zuo, R. E. T. Yu, K. Li, J. Xie, W. Sun and R. Wang, Orthogonal bioconjugation targeting cysteine-containing peptides and proteins using alkyl thianthrenium salts, Nat. Commun., 2024, 15, 6909 CrossRef PubMed;
(f) P. Hartmann, K. Bohdan, M. Hommrich, F. Juliá, L. Vogelsang, J. Eirich, R. Zangl, C. Farès, J. B. Jacobs, D. Mukhopadhyay, J. M. Mengeler, A. Vetere, M. S. Sterling, H. Hinrichs, S. Becker, N. Morgner, W. Schrader, I. Finkemeier, K.-J. Dietz, C. Griesinger and T. Ritter, Chemoselective umpolung of thiols to episulfoniums for cysteine bioconjugation, Nat. Chem., 2024, 16, 380–388 CrossRef CAS PubMed;
(g) S. R. Nasireddy, G. C. Upreti and A. Singh, Photochemical Trifluoromethylative Difunctionalization of Styrenes and Phenylacetylenes via a Catalytic EDA Platform, Eur. J. Org. Chem., 2024, e202400114 CrossRef CAS;
(h) Y. Fang, Q. Liang, L. Shi, J. Wen, X. Liu, X. Hong, X. Zha and F. Ji, Site-Selective S-Arylation of 1-Thiosugars with Aryl Thianthrenium Salts through Copper(I)-Mediated, Photoredox- Catalyzed Reactions, Adv. Synth. Catal., 2024, 366, 2344–2351 CrossRef CAS;
(i) B. Mondal, A. Chatterjee, N. C. Saha, M. Jana and J. Saha, Thianthrenation-promoted photoinduced alkene difunctionalization and aryl allylation with Morita–Baylis–Hillman adducts, Chem. Commun., 2024, 60, 7184–7187 RSC;
(j) G. Magagnano, V. Poirier, F. Romoli, D. Corbisiero, F. Calogero, P. G. Cozzi and A. Gualandi, Dielectrophilic Approach to Sequential Heterofunctionalization of Ethylene from Vinylthianthrenium Salt, Eur. J. Org. Chem., 2024, e202400224 CrossRef CAS;
(k) P. Sarró, A. Gallego-Gamo, R. Pleixats, A. Vallribera, C. Gimbert-Suriñach and A. Granados, Access to Benzyl Oxindoles via Electron Donor-Acceptor Complex Photoactivation Using Thianthrenium Salts and Potassium Carbonate, Adv. Synth. Catal., 2024, 366, 2587–2595 CrossRef;
(l) J. Zhang, M. Jiao, Z. Lu, H. Lu, M. Wang and Z. Shi, Hydrodeuteroalkylation of Unactivated Olefins Using Thianthrenium Salts, Angew. Chem., Int. Ed., 2024, 63, e202409862 CrossRef CAS PubMed;
(m) Z. He and P. Dydio, Photoinduced Cu(II)-Mediated Decarboxylative Thianthrenation of Aryl and Heteroaryl Carboxylic Acids, Angew. Chem., Int. Ed., 2024, 63, e202410616 CAS;
(n) G. Zhang, Z. Luo, G. Mei, H. Wang and C. Ding, EDA Complex from BCP-Thianthrenium Salt: A Catalyst-Free Strategy To Access 1-Trifluoromethyl-3-quinoxaline Derivatives Bicyclo[1.1.1]pentanes, Eur. J. Org. Chem., 2024, e202400386 CrossRef CAS;
(o) D. Dupommier, M. Vuagnat, J. Rzayev, S. Roy, P. Jubault and T. Besset, Site-Selective Ortho/Ipso C−H Difunctionalizations of Arenes using Thianthrene as a Leaving Group, Angew. Chem., Int. Ed., 2024, 63, e202403950 CrossRef CAS PubMed;
(p) H. Moon, J. Jung, J.-H. Choi and W.-J. Chung, Stereospecific syn-dihalogenations and regiodivergent syn-interhalogenation of alkenes via vicinal double electrophilic activation strategy, Nat. Commun., 2024, 15, 3710 CrossRef CAS PubMed;
(q) M.-S. Liu, H.-W. Du, H. Meng, Y. Xie and W. Shu, Unified metal-free intermolecular Heck-type sulfonylation, cyanation, amination, amidation of alkenes by thianthrenation, Nat. Commun., 2024, 15, 529 CrossRef CAS PubMed;
(r) Z. Zhang, X. Chen, Z.-J. Niu, Z.-J. Niu, Z.-M. Li, Q. Li, W.-Y. Shi, T. Ding, X.-Y. Liu and Y.-M. Liang, A Practical and Regioselective Strategy for Aromatic C–H Difunctionalization via Site-Selective C–H Thianthrenation, Org. Lett., 2024, 26, 1813–1818 CrossRef CAS PubMed;
(s) W. Qi, S. Gu and L.-G. Xie, Reductive Radical-Polar Crossover Enabled Carboxylative Alkylation of Aryl Thianthrenium Salts with CO2 and Styrenes, Org. Lett., 2024, 26, 728–733 CrossRef CAS PubMed;
(t) F. Xiang, D. Wang, K. Xu and C.-C. Zeng, Paired Electrolysis Enabled Trifluoromethylheteroaromatization of Alkenes and Alkyne with Trifluoromethyl Thianthrenium Triflate (TT-CF3+OTf–) as a Bifunctional Reagent, Org. Lett., 2024, 26, 411–415 CrossRef CAS PubMed;
(u) J. Zhang, L.-C. Wang, Y. Wang, B.-H. Teng and X.-F. Wu, Site-selective carbonylation of arenes via C(sp2)-H thianthrenation: Palladium-catalyzed direct access to α,β-unsaturated ketones, J. Catal., 2024, 432, 115454 CrossRef CAS;
(v) L.-Z. Qin, M.-Y. Wu, X. Yuan, H. Sun, X. Duan, J.-K. Qiu and K. Guo, The development and application of a novel trideuterium methylation reagent, Cell Rep. Phys. Sci., 2024, 5, 101843 CrossRef CAS;
(w) B. Kerwin, S. E. Liu, T. Sadhukhan, A. Dasgupta, L. O. Jones, R. López-Arteaga, T. T. Zeng, A. Facchetti, G. C. Schatz, M. C. Hersam and T. J. Marks, Trifluoromethylation of 2D Transition Metal Dichalcogenides: A Mild Functionalization and Tunable p-Type Doping Method, Angew. Chem., Int. Ed., 2024, 63, e202403494 CrossRef CAS PubMed;
(x) X.-B. Hu, Q.-Q. Fu, X.-Y. Huang, X.-Q. Chu, Z.-L. Shen, C. Miao and W. Chen, Hydroxylation of Aryl Sulfonium Salts for Phenol Synthesis under Mild Reaction Conditions, Molecules, 2024, 29, 831 CrossRef CAS PubMed;
(y) T. Zhang, J. Rabeah and S. Das, Red-light-mediated copper-catalyzed photoredox catalysis promotes regioselectivity switch in the difunctionalization of alkenes, Nat. Commun., 2024, 15, 5208 CrossRef CAS PubMed;
(z) T. Liu, T. Li, Z. Y. Tea, C. Wang, T. Shen, Z. Lei, X. Chen, W. Zhang and J. Wu, Modular assembly of arenes, ethylene and heteroarenes for the synthesis of 1,2-arylheteroaryl ethanes, Nat. Chem., 2024, 16, 1705–1714 CrossRef CAS PubMed.
- For further selected examples, see:
(a) D. E. Holst, C. Dorval, C. K. Winter, I. A. Guzei and Z. K. Wickens, Regiospecific Alkene Aminofunctionalization via an Electrogenerated Dielectrophile, J. Am. Chem. Soc., 2023, 145, 8299–8307 CrossRef CAS PubMed;
(b) A. Dewanji, L. van Dalsen, J. A. Rossi-Ashton, E. Gasson, G. E. M. Crisenza and D. J. Procter, A general arene C–H functionalization strategy via electron donor–acceptor complex photoactivation, Nat. Chem., 2023, 15, 43–52 CrossRef CAS PubMed;
(c) W. Liu, H. Hou, H. Jing, S. Huang, W. Ou and C. Su, Photoinduced Phosphination of Arenes Enabled by an Electron Donor–Acceptor Complex Using Thianthrenium Salts, Org. Lett., 2023, 25, 8350–8355 CrossRef CAS PubMed;
(d) B. Li, D. Xing, X. Li, S. Chang, H. Jiang and L. Huang, Chemo-divergent Cyano Group Migration: Involving Elimination and Substitution of the Key α-Thianthrenium Cyano Species, Org. Lett., 2023, 25, 6633–6637 CrossRef CAS PubMed;
(e) X.-Y. Chen, Y.-N. Li, Y. Wu, J. Bai, Y. Guo and P. Wang, Cu-Mediated Thianthrenation and Phenoxathiination of Arylborons, J. Am. Chem. Soc., 2023, 145, 10431–10440 CrossRef CAS PubMed;
(f) G. Zhang, Z. Luo, C. Guan, X. Zhang and C. Ding, Nickel-Catalyzed Selective C–H Cyanation via Aromatic Thianthrenium Salts, J. Org. Chem., 2023, 88, 9249–9256 CrossRef CAS PubMed;
(g) H. Xu, X. Li, Y. Dong, S. Ji, J. Zuo, J. Lv and D. Yang, Thianthrenium-Enabled Phosphorylation of Aryl C–H Bonds via Electron Donor–Acceptor Complex Photoactivation, Org. Lett., 2023, 25, 3784–3789 CrossRef CAS PubMed;
(h) S. Ni, J. Yan, S. Tewari, E. J. Reijerse, T. Ritter and J. Cornella, Nickel Meets Aryl Thianthrenium Salts: Ni(I)-Catalyzed Halogenation of Arenes, J. Am. Chem. Soc., 2023, 145, 9988–9993 CrossRef CAS PubMed;
(i) N. Kaplaneris, A. Puet, F. Kallert, J. Pöhlmann and L. Ackermann, Late-stage C−H Functionalization of Tryptophan-Containing Peptides with Thianthrenium Salts: Conjugation and Ligation, Angew. Chem., Int. Ed., 2023, 62, e202216661 CrossRef CAS PubMed;
(j) R. A. Roberts, B. E. Metze, A. Nilova and D. R. Stuart, Synthesis of Arynes via Formal Dehydrogenation of Arenes, J. Am. Chem. Soc., 2023, 145, 3306–3311 CrossRef CAS PubMed;
(k) P. Angyal, A. M. Kotschy, Á. Dudás, S. Varga and T. Soós, Intertwining Olefin Thianthrenation with Kornblum/Ganem Oxidations: Ene-type Oxidation to Furnish α,β-Unsaturated Carbonyls, Angew. Chem., Int. Ed., 2023, 62, e202214096 CrossRef CAS PubMed;
(l) M. J. Cabrera-Afonso, A. Granados and G. A. Molander, Sustainable Thioetherification via Electron Donor–Acceptor Photoactivation Using Thianthrenium Salts, Angew. Chem., Int. Ed., 2022, 61, e202202706 CrossRef CAS PubMed;
(m) M.-S. Liu, H.-W. Du, J.-F. Cui and W. Shu, Intermolecular Metal-Free Cyclopropanation and Aziridination of Alkenes with XH2 (X=N, C) by Thianthrenation, Angew. Chem., Int. Ed., 2022, 61, e202209929 CrossRef CAS PubMed;
(n) S. Liu, H.-W. Du and W. Shu, Metal-free allylic C–H nitrogenation, oxygenation, and carbonation of alkenes by thianthrenation, Chem. Sci., 2022, 13, 1003–1008 RSC;
(o) Y. Zhao, C. Yu, W. Liang, I. L. Atodiresei and F. W. Patureau, TEMPO-mediated late stage photochemical hydroxylation of biaryl sulfonium salts, Chem. Commun., 2022, 58, 2846–2849 RSC;
(p) C. Chen, Z.-J. Wang, H. Lu, Y. Zhao and Z. Shi, Generation of non-stabilized alkyl radicals from thianthrenium salts for C–B and C–C bond formation, Nat. Commun., 2021, 12, 4526 CrossRef CAS PubMed.
- F. Juliá, Q. Shao, M. Duan, M. B. Plutschack, F. Berger, J. Mateos, C. Lu, X.-S. Xue, K. N. Houk and T. Ritter, High Site Selectivity in Electrophilic Aromatic Substitutions: Mechanism of C–H Thianthrenation, J. Am. Chem. Soc., 2021, 143, 16041–16054 CrossRef PubMed.
- For reviews, see:
(a) C. Cordovilla, C. Bartolomé, J. M. Martínez-Ilarduya and P. Espinet, The Stille Reaction, 38 Years Later, ACS Catal., 2015, 5, 3040–3053 CrossRef CAS;
(b) P. Espinet and A. M. Echavarren, The Mechanisms of the Stille Reaction, Angew. Chem., Int. Ed., 2004, 43, 4704–4734 CrossRef CAS PubMed;
(c) V. Farina, V. Krishnamurthy and W. J. Scott, The Stille Reaction, Org. React., 1997, 50, 1–652 CAS;
(d) J. K. Stille, The Palladium-Catalyzed Cross-Coupling Reactions of Organotin Reagents with Organic Electrophiles, Angew. Chem., Int. Ed. Engl., 1986, 25, 508–524 CrossRef.
- For initial reports, see:
(a) M. Kosugi, K. Sasazawa, Y. Shimizu and T. Migita, Reactions of allyltin compounds III. Allylation of aromatic halides with allyltributyltin in the presence of tetrakis(triphenylphosphine)palladium(0), Chem. Lett., 1977, 301–302 CrossRef CAS;
(b) D. Milstein and J. K. Stille, A general, selective, and facile method for ketone synthesis from acid chlorides and organotin compounds catalyzed by palladium, J. Am. Chem. Soc., 1978, 100, 3636–3638 CrossRef CAS;
(c) D. Milstein and J. K. Stille, Palladium-catalyzed coupling of tetraorganotin compounds with aryl and benzyl halides. Synthetic utility and mechanism, J. Am. Chem. Soc., 1979, 101, 4992–4998 CrossRef CAS.
- For selected examples, see:
(a) H. Muraoka, S. Kubota and S. Ogawa, Synthesis and Emission Properties of a Series of 2,3,4,5-Tetrakis(5-aryl-2-thienyl)-1-phenylpyrroles as a Sterically-crowded Star-shaped D-(π-A)4 Molecule, Chem. Lett., 2020, 49, 10–13 CrossRef CAS;
(b) A. Rana, Y. Hong, T. Y. Gopalakrishna, H. Phan, T. S. Herng, P. Yadav, J. Ding, D. Kim and J. Wu, Stable Expanded Porphycene-Based Diradicaloid and Tetraradicaloid, Angew. Chem., Int. Ed., 2018, 57, 12534–12537 CrossRef CAS PubMed;
(c) A. H. Antropow, K. Xu, R. J. Buchsbaum and M. Movassaghi, Synthesis and Evaluation of Agelastatin Derivatives as Potent Modulators for Cancer Invasion and Metastasis, J. Org. Chem., 2017, 82, 7720–7731 CrossRef CAS PubMed;
(d) J. Shi, I. Murtaza, S. Shao, X. Zhu, Y. Zhao, M. Zhu, O. Goto and H. Meng, Tetra-EDOT substituted 3D electrochromic polymers with lower band gaps, Sci. China: Chem., 2017, 60, 90–98 CrossRef CAS;
(e) B. J. Hale, M. Elshobaki, R. Gebhardt, D. Wheeler, J. Stoffer, A. Tomlinson, S. Chaudhary and M. Jeffries-EL, Evaluating the influence of heteroatoms on the electronic properties of aryl[3,4-c]pyrroledione based copolymers, Polymer, 2017, 109, 85–92 CrossRef CAS;
(f) G. Anguera, B. Kauffmann, J. I. Borrell, S. Borrós and D. Sánchez-García, Stable 5,5′-Substituted 2,2′-Bipyrroles: Building Blocks for Macrocyclic and Materials Chemistry, J. Org. Chem., 2017, 82, 6904–6912 CrossRef CAS PubMed;
(g) M. Jouanneau, B. McClary, J. C. P. Reyes, R. Chen, Y. Chen, W. Plunkett, X. Cheng, A. Z. Milinichik, E. F. Albone, J. O. Liu and D. Romo, Derivatization of agelastatin A leading to bioactive analogs and a trifunctional probe, Bioorg. Med. Chem. Lett., 2016, 26, 2092–2097 CrossRef CAS PubMed;
(h) X.-B. Ding, D. P. Furkert, R. J. Capon and M. A. Brimble, Total Synthesis of Heronapyrrole C, Org. Lett., 2014, 16, 378–381 CrossRef CAS PubMed;
(i) M. G. Banwell, B. L. Flynn, E. Hamel and D. C. R. Hockless, Convergent syntheses of the pyrrolic marine natural products lamellarin-O, lamellarin-Q, lukianol-A and some more highly oxygenated congeners, Chem. Commun., 1997, 207–208 RSC;
(j) J. Wang and A. I. Scott, An efficient synthesis of 3-vinylpyrroles by Stille coupling reaction of 3-iodopyrroles with vinyltributyltin, Tetrahedron Lett., 1995, 36, 7043–7046 CrossRef CAS.
-
(a) A. S.-Y. Lee and W.-C. Dai, A facile and highly efficient sonochemical synthesis of organostannane via Barbier reaction, Tetrahedron, 1997, 53, 859–868 CrossRef CAS;
(b) R. A. Rossi, Recent advances in the synthesis of stannanes and the scope of their posterior chemical transformations, J. Organomet. Chem., 2014, 751, 201–212 CrossRef CAS.
- J. A. Ragan, J. W. Raggon, P. D. Hill, B. P. Jones, R. E. McDermott, M. J. Munchhof, M. A. Marx, J. M. Casavant, B. A. Cooper, J. L. Doty and Y. Lu, Cross-Coupling Methods for the Large-Scale Preparation of an Imidazole−Thienopyridine: Synthesis of [2-(3-Methyl-3H-imidazol-4-yl)-thieno[3,2-b]pyridin-7-yl]-(2-methyl-1H-indol-5-yl)-amine, Org. Process Res. Dev., 2003, 7, 676–683 CrossRef CAS.
- I. J. Boyer, Toxicity of dibutyltin, tributyltin and other organotin compounds to humans and to experimental animals, Toxicology, 1989, 55, 253–298 CrossRef CAS PubMed.
- E. Le Grognec, J.-M. Chrétien, F. Zammattio and J.-P. Quintard, Methodologies Limiting or Avoiding Contamination by Organotin Residues in Organic Synthesis, Chem. Rev., 2015, 115, 10207–10260 CrossRef CAS PubMed.
-
(a) L. S. Liebeskind and R. W. Fengl, 3-Stannylcyclobutenediones as nucleophilic cyclobutenedione equivalents. Synthesis of substituted cyclobutenediones and cyclobutenedione monoacetals and the beneficial effect of catalytic copper iodide on the Stille reaction, J. Org. Chem., 1990, 55, 5359–5364 CrossRef CAS;
(b) S. Gronowitz, P. Björk, J. Malm and A.-B. Hörnfeldt, The effect of some additives on the Stille Pd0-catalyzed cross-coupling reaction, J. Organomet. Chem., 1993, 460, 127–129 CrossRef CAS;
(c) V. Farina, S. Kapadia, B. Krishnan, C. Wang and L. S. Liebeskind, On the Nature of the “Copper Effect” in the Stille Cross-Coupling, J. Org. Chem., 1994, 59, 5905–5911 CrossRef CAS;
(d) X. Han, B. M. Stoltz and E. J. Corey, Cuprous Chloride Accelerated Stille Reactions. A General and Effective Coupling System for Sterically Congested Substrates and for Enantioselective Synthesis, J. Am. Chem. Soc., 1999, 121, 7600–7605 CrossRef CAS.
- M. Hervé, G. Lefèvre, E. A. Mitchell, B. U. W. Maes and A. Jutand, On the Triple Role of Fluoride Ions in Palladium-Catalyzed Stille Reactions, Chem. – Eur. J., 2015, 21, 18401–18406 CrossRef PubMed.
-
(a) S. P. H. Mee, V. Lee and J. E. Baldwin, Stille Coupling Made Easier—The Synergic Effect of Copper(I) Salts and the Fluoride Ion, Angew. Chem., Int. Ed., 2004, 43, 1132–1136 CrossRef CAS PubMed;
(b) S. P. H. Mee, V. Lee and J. E. Baldwin, Significant Enhancement of the Stille Reaction
with a New Combination of Reagents—Copper(I) Iodide with Cesium Fluoride, Chem. – Eur. J., 2005, 11, 3294–3308 CrossRef CAS PubMed;
(c) V. Lee, Application of copper(I) salt and fluoride promoted Stille coupling reactions in the synthesis of bioactive molecules, Org. Biomol. Chem., 2019, 17, 9095–9123 RSC.
-
(a) V. Farina and B. Krishnan, Large rate accelerations in the stille reaction with tri-2-furylphosphine and triphenylarsine as palladium ligands: mechanistic and synthetic implications, J. Am. Chem. Soc., 1991, 113, 9585–9595 CrossRef CAS;
(b) C. Amatore, A. A. Bahsoun, A. Jutand, G. Meyer, A. N. Ntepe and L. Ricard, Mechanism of the Stille Reaction Catalyzed by Palladium Ligated to Arsine Ligand: PhPdI(AsPh3)(DMF) is the Species Reacting with Vinylstannane in DMF, J. Am. Chem. Soc., 2003, 125, 4212–4222 CrossRef CAS PubMed.
- J. Jing, Y. Hu, Z. Tian, Y. Wang, L. Yao, L. Qiu, L. Ackermann, K. Karaghiosoff and J. Li, C−S-Selective Stille-Coupling Enables Stereodefined Alkene Synthesis, Angew. Chem., Int. Ed., 2024, 63, e202408211 CAS.
-
(a) J. Song, S. Y. Jang, S. Yamaguchi, J. Sankar, S. Hiroto, N. Aratani, J.-Y. Shin, S. Easwaramoorthi, K. S. Kim, D. Kim, H. Shinokubo and A. Osuka, 2,5-Thienylene-Bridged Triangular and Linear Porphyrin Trimers, Angew. Chem., Int. Ed., 2008, 47, 6004–6007 CrossRef CAS PubMed;
(b) J. Song, N. Aratani, H. Shinokubo and A. Osuka, A β-to-β 2,5-thienylene-bridged cyclic porphyrin tetramer: its rational synthesis and 1:2 binding mode with C60, Chem. Sci., 2011, 2, 748–751 RSC;
(c) S. Tokuji, H. Yorimitsu and A. Osuka, Preferential Formation of Cyclic Trimers by Palladium-Catalyzed Oxidative Coupling Reactions of 2,18-Diethynylporphyrins, Angew. Chem., Int. Ed., 2012, 51, 12357–12361 CrossRef CAS PubMed;
(d) S. Omomo, Y. Maruyama, K. Furukawa, T. Furuyama, H. Nakano, N. Kobayashi and Y. Matano, Optical, Electrochemical, and Magnetic Properties of Pyrrole- and Thiophene-Bridged 5,15-Diazaporphyrin Dimers, Chem. – Eur. J., 2015, 21, 2003–2010 CrossRef CAS PubMed;
(e) I. Nishimura, T. Higashino and H. Imahori, Synthesis of thiophene-fused porphyrin dimers as effective π-extended helical chromophores, Chem. Commun., 2021, 57, 9606–9609 RSC.
- T. Javorskis and E. Orentas, Chemoselective Deprotection of Sulfonamides Under Acidic Conditions: Scope, Sulfonyl Group Migration, and Synthetic Applications, J. Org. Chem., 2017, 82, 13423–13439 CrossRef CAS PubMed.
-
(a) T. Yamagishi, H. Ichikawa, T. Haruki and T. Yokomatsu, Diastereoselective Synthesis of α,β′-Disubstituted Aminomethyl(2-carboxyethyl)phosphinates as Phosphinyl Dipeptide Isosteres, Org. Lett., 2008, 10, 4347–4350 CrossRef CAS PubMed;
(b) T. Ankner and G. Hilmersson, Instantaneous Deprotection of Tosylamides and Esters with SmI2/Amine/Water, Org. Lett., 2009, 11, 503–506 CrossRef CAS PubMed;
(c) M. Szostak, M. Spain and D. J. Procter, Determination of the Effective Redox Potentials of SmI2, SmBr2, SmCl2, and their Complexes with Water by Reduction of Aromatic Hydrocarbons. Reduction of Anthracene and Stilbene by Samarium(II) Iodide–Water Complex, J. Org. Chem., 2014, 79, 2522–2537 CrossRef CAS PubMed.
- B. Nyasse, L. Grehn and U. Ragnarsson, Mild, efficient cleavage of arenesulfonamides by magnesium reduction, Chem. Commun., 1997, 1017–1018 RSC.
- D. Zhao, R. Petzold, J. Yan, D. Muri and T. Ritter, Tritiation of aryl thianthrenium salts with a molecular palladium catalyst, Nature, 2021, 600, 444–449 CrossRef CAS PubMed.
- A. L. Casado, P. Espinet and A. M. Gallego, Mechanism of the Stille Reaction. 2. Couplings of Aryl Triflates with Vinyltributyltin. Observation of Intermediates. A More Comprehensive Scheme, J. Am. Chem. Soc., 2008, 130, 10518–10520 CrossRef PubMed.
Footnotes |
† Electronic supplementary information (ESI) available: Experimental procedures, NMR spectra and X-ray data. CCDC 2382855 and 2382856. For ESI and crystallographic data in CIF or other electronic format see DOI: https://doi.org/10.1039/d4qo01793e |
‡ An alternative explanation for the formation of 9 would be by reductive cleavage of the sulfonium group from 8. However, this appears less likely since if the reductive cleavage occurs by Pd-mediated S–C bond activation in the first step, then in 8 this would be expected to favour the S–Ph bond over the S–pyrrole bond, on the basis of the results shown in Scheme 9. |
§ The synthesis of indol-3-ylthianthrenium tetrafluoroborate is reported in the ESI† for ref. 39. |
|
This journal is © the Partner Organisations 2024 |
Click here to see how this site uses Cookies. View our privacy policy here.