DOI:
10.1039/D3TB02637J
(Paper)
J. Mater. Chem. B, 2024,
12, 3079-3091
Strong, tough, and elastic poly(vinyl alcohol)/polyacrylamide DN hydrogels based on the Hofmeister effect for articular cartilage replacement†
Received
7th November 2023
, Accepted 20th February 2024
First published on 20th February 2024
Abstract
Traditional hydrogels are usually weak and brittle, which limit their application in articular cartilage replacement because cartilage is generally strong, tough, and elastic in nature. Therefore, it is highly desirable to construct hydrogels to mimic the mechanical properties of the native articular cartilage. Herein, in this work, poly(vinyl alcohol)/polyacrylamide (PVA/PAM) DN hydrogels were prepared by in situ polymerization, which were then treated with Hofmeister series ions (Cit3−, SO42−, and Cl−) to achieve H-PVA/PAM DN hydrogels. Among the three Hofmeister ions, the DN hydrogel treated with Cit3− (named PVA/PAM–Cit) showed the densest microstructure and the highest crystallinity degree. In this context, PVA/PAM–Cit exhibited a tensile strength of 18.9 ± 1.6 MPa, a compressive strength of 102.3 ± 7.9 MPa, a tensile modulus of 10.6 ± 2.1 MPa, a compressive modulus of 8.9 ± 0.8 MPa, and a roughness of 66.2 ± 4.2 MJ m−3, respectively, which were the highest strength and modulus, and the second highest toughness when compared with those of the reported PVA and PVA based DN hydrogels so far. It also showed an extreme high elasticity, which could maintain a stress of 99.2% after 500 cycles of fatigue testing. Additionally, PVA/PAM–Cit can promote the adhesion, spreading and proliferation of chondrocytes. These results verify that such a strong, tough, and elastic hydrogel could be a novel candidate material for articular cartilage replacement.
1. Introduction
Articular cartilage, which is a load-bearing tissue in the diarthrodial joint, and a highly hydrated connective tissue covered on the natural joint surface, plays a vital role in maintaining the stability and lubrication in the knees or joints.1,2 However, owing to its confined intrinsic avascular and cell-free natural state, articular cartilage has a very limited self-repair potential, which usually results in osteoarthritis.3,4 Current strategies for cartilage repair include micro-fracture, autologous cartilage cell implantation, and osteochondral transplantation.5–7 However, they have disadvantages such as the limited origin of the donor, unmatched mechanical properties, risks of disease transmission, and immunogenicity.8 Therefore, it is essential to develop a cost-effective and high-performance material for articular cartilage replacement.
Hydrogels have been extensively explored as a cartilage substitute as they are characterized by their three-dimensional porous network that can perfectly simulate the extracellular matrix (ECM) of human tissues and a high-water content (70–90 wt%) that is similar to natural articular cartilage (70–80 wt%) as well as a low permeability.9–11 Among the hydrogels, a growing emphasis has been placed on polyvinyl alcohol (PVA) hydrogels due to their high hydrophilicity, complexing ability, biocompatibility, and so on.12,13 However, the poor mechanical properties limit their application as a cartilage substitute.14,15 For example, the toughness, tensile strength and compressive strength of pure PVA hydrogels are generally 0.2–0.8 MJ m−3, 0.2–0.5 MPa and 0.2–2.1 MPa,16–19 respectively, which are all much lower than those of native cartilage (toughness: 1.0 MJ m−3, tensile strength: 0.8–25.0 MPa, and compressive strength of 8.4–59.0 MPa).20 The weak mechanical properties of PVA hydrogels are largely due to the single network structure, which results in the intrinsic structure heterogeneity and the lack of effective energy dissipation mechanisms. Forming double-network (DN) hydrogels that are composed of two polymers with different properties can achieve high strength, toughness and elasticity.21–23 For instance, Li et al.24 produced PVA/PAM (polyacrylamide) DN hydrogels with a toughness of 1.4 MJ m−3. Zhang et al.25 prepared PVA/PAM DN hydrogels with a compressive strength of 2.7 MPa, while Ou et al.26 prepared PVA/PAM DN hydrogels with a tensile strength of 1.5 MPa and a modulus of 0.5 MPa. The mechanical properties of PVA/PAM DN hydrogels are better than those of pure PVA hydrogels, but still less than satisfactory compared to those of cartilage. Therefore, increasing the strength and toughness of PVA based hydrogels to mimic the mechanical properties of native articular cartilage is highly desired.
A Hofmeister effect is a well-known concept based on the salt-in and salt-out properties, and is now proposed as an effective and universal strategy to toughen weak hydrogels by forming enhanced hydrogen bonds induced by ions due to the salt-out effect.27,28 A typical Hofmeister order for anions tending to cause the salt-out effect to enhance the mechanical properties is usually ordered: Cit3− > SO42− > S2O32− > H2PO4− > F− > CH3COO− > Cl−.29 To date, the Hofmeister effect has been observed for many polymers, such as starch polymers,30 polyvinylpyrrolidone,31 methylcellulose,32 gelatin,33 PVA,34 and so on. For example, Wu et al.35 fabricated PVA hydrogels with tunable mechanical properties via the Hofmeister effect and they showed a maximum tensile strength of 15.5 MPa and an extreme high toughness of 153.4 MJ m−3 by freezing PVA hydrogels in the saturated Na2SO4 aqueous solution, in which the enhanced hydrogen bonds between the hydroxyl groups of PVA played key roles in enhancing the strength and toughness. The hydrogels prepared utilizing the Hofmeister effect have weak elasticity. A natural cartilage tissue has high strength, toughness and high elasticity. In this context, the combination of DN hydrogels and the Hofmeister effect is highly expected to achieve strong, tough, and elastic PVA based hydrogels, which are not yet reported to our best knowledge.
Herein, in this work, PVA/PAM DN hydrogels were prepared via in situ polymerization first. And then, Cit3−, SO42−, and Cl− were selected as the model Hofmeister series to treat the DN hydrogels to achieve H-PVA/PAM DN hydrogels (Scheme 1). Afterwards, the morphologies, chemical properties, crystallization behavior, mechanical properties (modulus, strength, toughness, and elasticity), and cytocompatibility of H-PVA/PAM DN hydrogels were investigated.
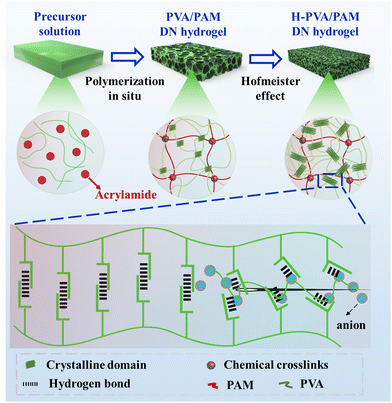 |
| Scheme 1 The preparation procedure of H-PVA/PAM DN hydrogels based on the Hofmeister effect. | |
2. Experimental methods
2.1 Materials
PVA (1799, Mw: 74
800 g mol−1, >99% hydrolysis), anhydrous sodium chloride, anhydrous sodium sulfate, and anhydrous sodium citrate (analytical grade) were all provided by Shanghai Macklin Biochemical Co, Ltd, Shanghai, China. Acrylamide (AM, analytical grade), ammonium persulphate (APS, analytical grade), and N,N′-methylenebisacrylamide (MBA, analytical grade) were purchased from Shanghai Aladdin Reagent Co, Ltd, Shanghai, China. All materials were used as received without further purification.
2.2 Fabrication of H-PVA/PAM DN hydrogels
First, 10 wt% PVA aqueous solution, 9 wt% MBA aqueous solution, and 9 wt% APS aqueous solution were prepared for the following sample fabrication. Then, by using the optimal parameters, PAV/PAM DN hydrogels were prepared. Briefly, 8 g of an AM monomer, 40 μL of APS aqueous solution (initiator) and 100 μL of MBA aqueous solution were added into 40 g of PVA aqueous solution, followed by magnetic stirring to achieve a mixture solution. After heating at 65 °C for 30 min, the initial DN hydrogel was prepared, which was then subjected to freeze–thawing for 3 times to achieve the final PVA/PAM hydrogel. Afterwards, the PVA/PAM hydrogel was immersed in 1.5 M salt solutions with various Hofmeister ions (Cl−, SO42− and Cit3−) for 24 h to produce H-PVA/PAM DN hydrogels, which were named PVA/PAM–Cl, PVA/PAM–SO4, and PVA/PAM–Cit, respectively.
2.3 Characterization
To determine the morphologies of the hydrogels using a scanning electron microscope (SEM, HITACHI SU-8010, Hitachi, Japan), all hydrogel samples were immersed in distilled water for 24 h before freeze drying. The pore size was measured from the SEM images using Nano measure. The chemical and crystal structures were analyzed using a Fourier transform infrared spectrometer (FTIR, PerkinElmer Spectrum one, USA) and an X-Ray diffractometer (XRD) (Panaco Shadow, Xpert Pro MPD), respectively. The water content was calculated using the ratio of the wet weight and the dry weight after freeze drying. The porosity was determined by the liquid displacement method (liquid
:
ethanol)36 and was calculated using the equation of P = (M2 − M1)/ρV, where M1 is the dry weight of the sample, M2 is the wet weight of the sample, V is the increased volume after the immersion of samples in ethanol, and ρ is the density of ethanol (0.8 g cm−3). The amount of Hofmeister series ions inside the hydrogels was calculated using the equation of ΔM = (M2 − M1), where M2 is the original solute quality of Hofmeister series ions, and M1 is the solute quality of Hofmeister series ions after immersing hydrogels and unfixed ion washing from hydrogels. The swelling degree was determined using the equation of P = (M2 − M1)/M1, where M1 is the dry weight of the sample and M2 is the wet weight of the sample.37
2.4 Mechanical properties tests
For the tensile test, the hydrogels were cut into dog-bone shaped specimens (length: 15 mm, thicknesses: 2 mm, and neck width: 4 mm) using an electronic universal testing machine (CMT4104, MTS Systems Co., Ltd, Shanghai, China) at a tensile rate of 200 mm min−1. The toughness was calculated using the integrated area of the tensile stress–strain curve. For the compression mechanics test, all the hydrogels were cut into cylinders (height: 12 mm and diameter: 10 mm) for testing by using the above machine at a compression rate of 10 mm min−1. At the same time, the specimens (height: 6 mm and diameter: 10 mm) were tested via a precise miniature electromagnetic fatigue testing machine (M100, Tianjin Care Measure & Control Co., Ltd, Tianjin, China) with a compressive strain of 50% (the maximum force of this machine) for 500 cycles to determine their elasticity.
2.5 Assessment of cytocompatibility
The samples of PVA, PAM, PVA/PAM, and PVA/PAM–Cit were first freeze dried and then sterilized with UV irradiation for 12 h. Afterwards, all the samples were washed with sterile phosphate buffer solution (PBS). The chondrocytes were seeded on the samples at a density of 2 × 104 cells per well. The incubation was carried out in Dulbecco's modified Eagle's medium (DMEM) supplemented with 10% fetal bovine serum (FBS) and 1% penicillin–streptomycin solution for 1, 3, and 5 days at 37 °C in a 5% CO2 environment. After the completion of incubation, a routine cell counting Kit-8 (CCK-8, Solarbio, China) assay was performed in the dark and the cells were cultured for 2 h at 37 °C and 5% CO2. Then, the cells were transferred to 96-well plates and the assay was conducted to assess the proliferation by measuring the absorbance at 450 nm using a microplate reader (iMark, Bio Rad, California, USA).
Fluorescence cell images were obtained using the live/dead assay. After incubation for 1, 3, and 5 days under the aforementioned conditions and washing twice with PBS, cells on the samples were stained for 30 s using a live/dead staining reagent (fluorescein diacetate/propidium iodide, Shanghai Aladdin Reagent Co., Ltd, Beijing, China) and then observed using a fluorescence inverted microscope (TS2, Nikon, Tokyo, Japan) at 490 nm and 550 nm to determine the morphology of living and dead cells on the samples, respectively.
The cell morphology was observed with a SEM. After incubation for 3 days under the aforementioned conditions, the culture medium was removed, while the samples washed with PBS three times were fixed with 2.5% glutaraldehyde solution at 4 °C for 12 h and dehydrated in a graded series of ethanol (30, 40, 50, 60, 70, 80, 90, and 100%) for 10 min. Finally, after freeze-drying, the samples were coated with gold spraying and the cell morphology was observed using the aforementioned SEM.
To observe the cytoskeleton and nuclei, the materials co-cultured with cells were taken out from 24-well plates after incubation for 3 days under the aforementioned conditions and washed three times with PBS. Subsequently, the cells were fixed with 500 μL of 4% paraformaldehyde tissue fixative for 10 min in each well, and the above solution was sucked out. The materials were washed with PBS three times. The materials were treated in the same way with 0.3% Triton. Each well was added with 500 μL of phalloidin solution (100 nM) to stain the cytoskeleton for 30 min, and the materials were washed with PBS three times. The cell nuclei were counter-stained with 5 μL mL−1 4,6-diamidino-2-phenylindole (DAPI, Solarbio, Beijing, China) for 10 min. Afterwards, the samples were observed using a confocal laser scanning microscope (CLSM, TCS SP8, Leica Microsystems SMS GmbH, Wetzlar, Germany).
2.6 Assessment of differentiation
Immunofluorescence staining for COL II was performed after culturing the BMSCs for 7 days. Briefly, after fixing in 4% paraformaldehyde for 15 minutes, the samples were permeated with a solution of 0.1% Triton X-100 for 10 min and then blocked with a solution of 1% BSA (bovine serum albumin) for 30 min. Next, the samples were incubated with anti-COL II antibodies (dilution 1
:
200) (Beyotime Co., Ltd, Guangzhou, China) overnight at 4 °C, followed by incubation with an FITC-conjugated secondary antibody solution (dilution 1
:
200) (Beyotime Co., Ltd, Guangzhou, China) for 2 h at room temperature. Washing with 10% PBST (VTween-20
:
VPBS = 1
:
9) was carried out between each step in the process. The nucleus of BMSCs was visualized by DAPI (Solarbio, Beijing, China).
2.7 Statistical analysis
All experiments were conducted in triplicate unless otherwise stated. The Origin and statistical product service solutions (SPSS) software packages were used for statistical data processing and analysis. All data were presented as mean ± standard deviation (SD). Statistical significance was defined as p-values < 0.05.
3. Results and discussion
3.1 Morphologies
In this work, a two-step procedure was applied to prepare strong, tough, and elastic PVA based DN hydrogels. Briefly, using the optimal parameters, PVA/PAM DN hydrogels were prepared, which were then immersed in the Hofmeister series solution to achieve H-PVA/PAM DN hydrogels. Fig. 1(A) shows the macro-scale morphologies of PVA, PAM, PVA/PAM, PVA/PAM–Cl, PVA/PAM–SO4, and PVA/PAM–Cit, which were 14.0 mm, 14.0 mm, 14.0 mm, 16.4 mm, 12.2 mm, and 10.4 mm in diameter, respectively. PVA and PVA/PAM hydrogels were semitransparent, while the PAM hydrogel was transparent. Compared with PVA/PAM, an obvious (negative Hofmeister effect) volume expansion could be found for PVA/PAM–Cl hydrogels, which could be due to Cl− solution increasing the solubility of PVA in a lower concentration (<2 M).32,35 PVA/PAM–SO4 and PVA/PAM–Cit hydrogels displayed a volume shrinkage and became non-transparent when compared to PVA/PAM because of the salt-out effect for SO42−and Cit3−. The volume shrinkage degree was met well with the salt-out effect order of the Hofmeister series.32 As seen in Fig. 1(B), the PVA hydrogel displayed an inhomogeneous porous structure, while the pores of PAM, PVA/PAM, PVA/PAM–Cl, PVA/PAM–SO4, and PVA/PAM–Cit hydrogels were more homogeneous. Compared with the PVA/PAM hydrogel, the PVA/PAM–Cl hydrogel became looser while PVA/PAM–SO4 and PVA/PAM–Cit were much denser. The pore sizes of PVA, PAM, PVA/PAM, PVA/PAM–Cl, PVA/PAM–SO4, and PVA/PAM–Cit were 2.0 ± 0.1 μm, 58.0 ± 0.7 μm, 11.0 ± 0.1 μm, 15.8 ± 0.4 μm, 7.2 ± 0.1 μm, and 4.5 ± 0.5 μm, respectively (Fig. 1(C)). It can be clearly seen that when compared with PAM, the PVA/PAM DN hydrogel showed a much smaller pore size, which was due to the increased crosslinking density after compositing, resulting in reduced pore size and porosity.58 The positive Hofmeister effect (SO42− and Cit3−) significantly reduced the pore size of the PVA/PAM DN hydrogel, and the reduction coefficient depended on the types of Hofmeister series ions. However, they were still bigger than those of PVA hydrogels. It can be explained that the Hofmeister effect triggered the salting-out of the polymers, thereby resulting in the spontaneous collapse of polymer chains and formation of the small pores.35 Similarly, the negative Hofmeister effect (Cl−) increased the pore size of the PVA/PAM DN hydrogel, which agree well with the volume change result. As expected, the porosities of PVA, PAM, PVA/PAM, PVA/PAM–Cl, PVA/PAM–SO4, and PVA/PAM–Cit were 53.1 ± 6.3%, 86.6 ± 2.6%, 83.9 ± 1.7%, 84.0 ± 0.8%, 76.8 ± 1.6%, and 72.3 ± 0.8%, respectively (Fig. 1(D)). It should be noted that the amounts of Hofmeister series ions of the NaCl, Na2SO4 and Na3Cit within the hydrogels were 5.3 ± 1.9 mg, 12.4 ± 3.2 mg, and 26.9 ± 7.6 mg, respectively (Fig. S1, ESI†).
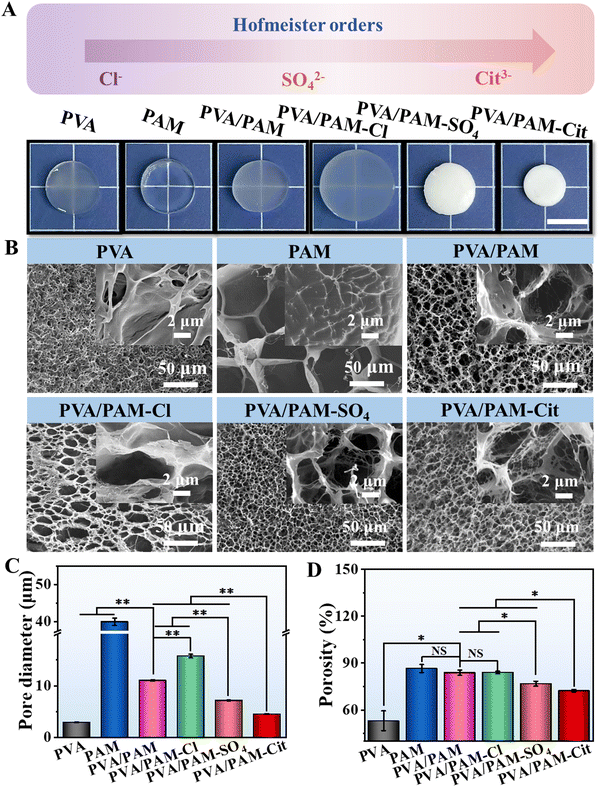 |
| Fig. 1 Digital photographs (A), SEM images (B), pore diameters (C), and porosities (D) of PVA, PAM, PVA/PAM, PVA/PAM–Cl, PVA/PAM–SO4, and PVA/PAM–Cit. The significance was defined as *p < 0.05 or **p < 0.01, n = 5. Scale bar: 10 mm. | |
3.2 Chemical and crystal structures
FTIR spectroscopy was performed to determine the chemistry changes in the formation of the PVA/PAM DN hydrogel and the influence of the Hofmeister effect on the H-PVA/PAM DN hydrogels. As seen in Fig. 2(A), PVA showed a broad band at 3299 cm−1 revealing the –OH stretching and a sharp band at 1094 cm−1 corresponding to the symmetrical stretching of the C–O–C group.38 From the FTIR spectrum of PAM, bands at 3338 cm−1 and 3192 cm−1 which are attributed to the –NH stretching, and absorption bands at 1658 cm−1 and 1608 cm−1 attributed to the –C
O stretching (amide I) and –NH2 bending vibration (amide II) could be clearly observed, respectively.39 According to the FTIR spectra of PVA/PAM, a shift (from 3299 cm−1 to 3395 cm−1) of the absorption band of –OH stretching was observed when compared to PVA, indicating the formation of hydrogen bonds between the –OH of PVA and –NH of PAM.40 One peak at about 3338 cm−1 of –NH stretching overlapped with –OH, and thus only a band at 3200 cm−1 related to –NH was observed, and the same phenomenon was also reported by Elbarbary et al.40 and Panpinit et al.41 Besides, the absorption bands of amide I and amide II were observed at 1663 cm−1 and 1605 cm−1, respectively. For H-PVA/PAM DN hydrogels, it could be observed that their absorption bands were almost the same as for the PVA/PAM hydrogel. However, a slight shift of –OH of PVA/PAM–SO4 (3395 cm−1 to 3413 cm−1) and PVA/PAM–Cit (3395 cm−1 to 3433 cm−1) was found when compared to PVA/PAM, suggesting that more abundant hydrogen bonds were formed after the treatment of Hofmeister series ions.35
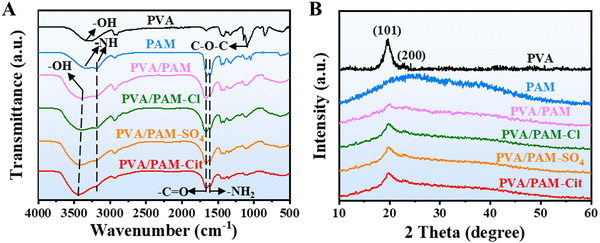 |
| Fig. 2 FTIR spectra (A) and XRD patterns (B) of PVA, PAM, PVA/PAM, PVA/PAM–Cl, PVA/PAM–SO4, and PVA/PAM–Cit. | |
The XRD patterns of PVA, PAM, PVA/PAM, PVA/PAM–Cl, PVA/PAM–SO4, and PVA/PAM–Cit are presented in Fig. 2(B). No crystalline peak (typical “steamed bread” peak) for PAM could be found, suggesting that PAM was amorphous, which agrees with the reported result.26 For PVA, two characteristic peaks at angles 2θ of 19.6° and 23.7° could be found, which correspond to the (101) and (200) crystallographic planes of PVA.42,43 The same peaks (19.6° and 23.7°) could be found in the XRD patterns of PVA/PAM, PVA/PAM–Cl, PVA/PAM–SO4, and PVA/PAM–Cit hydrogels, indicating that the formation of PVA/PAM and the Hofmeister effect did not change the crystal structure of PVA. The crystallization degree (χc) of PVA was 36.4%, which was similar to the reported value (39.0%).44 The incorporation of PAM can destruct the ordered packing of PVA45 and thus reduced the χc of PVA. The χc value of PVA/PAM decreased to 11.8%, which agreed with the reported value (10.8%).26 The Hofmeister effect can enhance the crystallization capability. The χc values of PVA/PAM–Cl, PVA/PAM–SO4, and PVA/PAM–Cit were 22.1%, 37.8% and 46.7%, respectively, whereby the χc followed the order of Hofmeister effect ions: Cit3− > SO2-4 > Cl−.46 The differences in crystallinity can be explained by the increased hydrophobic interaction between ions and PVA chains caused by the increased salt-out effect in the order of Cl−, SO2-4, and Cit3−.27,35
3.3 Water content and swelling properties
The water content of the PVA, PAM, PVA/PAM, PVA/PAM–Cl, PVA/PAM–SO4, and PVA/PAM–Cit was expected to show the same trends as pore sizes and porosities. It was 88.4 ± 0.4%, 81.8 ± 0.4%, 84.1 ± 0.3%, 85.0 ± 0.2%, 79.5 ± 0.7%, and 78.7 ± 0.7%, respectively (Fig. 3(A)), which were all close to that of natural cartilage (70–80 wt%).11 Normally, the sample with a higher water content would have a larger macro size and pores in the absence of violent phase separation. However, PVA has the highest water content, but PAM exhibited the largest pores (Fig. 1(C)). PVA and PAM are semi-crystalline and amorphous polymers, respectively.47,48 Therefore, the reason for such a mismatch between the pore and the water content could be due to the formation of the crystallization for PVA during freeze-drying. The swelling curves of PVA, PAM, PVA/PAM, PVA/PAM–Cl, PVA/PAM–SO4, and PVA/PAM–Cit are presented in Fig. 3(B). It can be clearly seen that PAM showed the highest equilibrium swelling of 10.3 ± 0.4 g g−1, which may be due to the larger pore size and porosity that can store more water. In contrast, the PVA exhibited the smallest porosity and pore size and thus showed the lowest equilibrium swelling of 3.2 ± 0.2 g g−1. In this case, a medium equilibrium swelling of 9.9 ± 0.5 g g−1 for PVA/PAM was determined. The equilibrium swelling of PVA/PAM–Cl, PVA/PAM–SO4 and PVA/PAM–Cit was 7.2 ± 0.5 g g−1, 6.2 ± 0.2 g g−1, and 6.1 ± 0.3 g g−1, respectively. After Hofmeister series treatment, all the hydrogels showed a smaller equilibrium swelling and the reduction degrees also followed the order of Cl−, SO2–4, and Cit3−. The reason could be that besides the impact of the pore diameter and porosity of the hydrogels, the Hofmeister effect increased the crystal domain of the PVA hydrogel to form the dipole–ion interaction of the stable swelling network, which can thus enhance the anti-swelling performance.
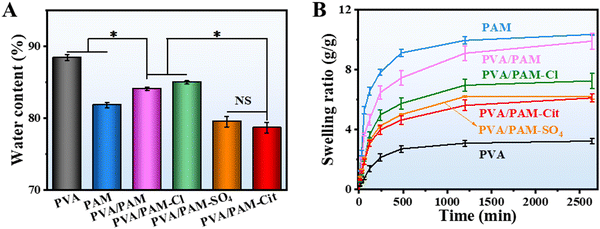 |
| Fig. 3 The water content (A) and swelling ratio (B) of PVA, PAM, PVA/PAM, PVA/PAM–Cl, PVA/PAM–SO4, and PVA/PAM–Cit. The significance was defined as *p < 0.05, n = 5. | |
3.4 Mechanical properties
The typical tensile stress–strain curves are displayed in Fig. 4(A). The tensile strengths of PVA, PAM, PVA/PAM, PVA/PAM–Cl, PVA/PAM–SO4, and PVA/PAM–Cit were 414.8 ± 105.0 kPa, 110.2 ± 45.4 kPa, 819.0 ± 83.3 kPa, 1.8 ± 0.1 MPa, 5.5 ± 0.7 MPa, and 9.1 ± 0.1 MPa, respectively (Fig. 4(B)), while their Young's moduli were 120.0 ± 1.0 kPa, 82.7 ± 5.2 kPa, 401.0 ± 54.6 kPa, 613.3 ± 40.4 kPa, 2.2 ± 0.1 MPa, and 4.8 ± 0.2 MPa, respectively (Fig. 4(C)). Compared with PVA and PAM, PVA/PAM exhibited a much higher mechanical strength, likely due to the formation of the DN structure and the intermolecular hydrogen bonds.49 What is more, the Hofmeister effect can further enhance the mechanical properties of PVA/PAM DN hydrogels, while the PVA/PAM–Cit showed the highest tensile strength and Young's modulus, which were 11.1 and 12.0 times stronger than those of PVA/PAM, respectively. On the one hand, it is due to the reduction of water content resulting in a denser structure (Fig. 1). On the other hand, it can be explained that the hydrogen bond is more abundant after the Hofmeister effect treatment, resulting in high polymer chain aggregation and higher crystallization that can act as rigid high functionality cross-linkers.50 For the elongation at break (Fig. 4(D)), PAM showed the highest value of 1063.8 ± 82.3%, while the PVA showed the lowest value of 458.8 ± 40.5%. The PVA/PAM hydrogel showed an elongation at break of 643.4 ± 22.5%, which decreased with the Hofmeister effect order. PVA/PAM–Cl, PVA/PAM–SO4, and PVA/PAM–Cit showed the elongations at break of 388.8 ± 13.1%, 537.2 ± 27.6%, and 602.9 ± 9.1%, respectively. Additionally, the toughness values of PVA, PAM, PVA/PAM, PVA/PAM–Cl, PVA/PAM–SO4, and PVA/PAM–Cit were 976.7 ± 280.6 kJ m−3, 532.2 ± 136.4 kJ m−3, 2.4 ± 0.3 MJ m−3, 3.1 ± 0.1 MJ m−3, 15.0 ± 1.9 MJ m−3, and 27.7 ± 0.9 MJ m−3, respectively (Fig. 4(E)). It can be explained that the crystalline domains delayed the fracture of individual nanofibrils by crack pinning leading to the toughness enhancement.51 Overall, the formation of the DN hydrogel and the Hofmeister effect can significantly improve the mechanical properties of PVA, in which, PVA/PAM–Cit showed the best tensile strength, tensile modulus and roughness. In this work, the influence of the Cit3− concentration on the mechanical properties of the DN hydrogel was also investigated. As seen in Table S1 (ESI†), a lower concentration of Cit3− (1 M) showed a lower strength (3.5 ± 0.3 MPa), modulus (1.1 ± 0.1 MPa), and toughness (8.6 ± 1.3 MJ m−3), respectively, while the saturated solution (2.6 M) exhibited a higher strength (18.9 ± 1.6 MPa), modulus (10.6 ± 2.1 MPa), and toughness (66.2 ± 4.2 MJ m−3), respectively. To our best knowledge, the PVA/PAM–Cit (saturated) showed the highest tensile modulus and tensile strength among all the report values for the PVA and PVA based DN hydrogels (Fig. 4(F) and Table S2, ESI†). It is worth mentioning that the toughness of PVA/PAM–Cit (saturated) was the second highest value among all the reported values for the PVA and PVA based DN hydrogels (Table S3, ESI†).
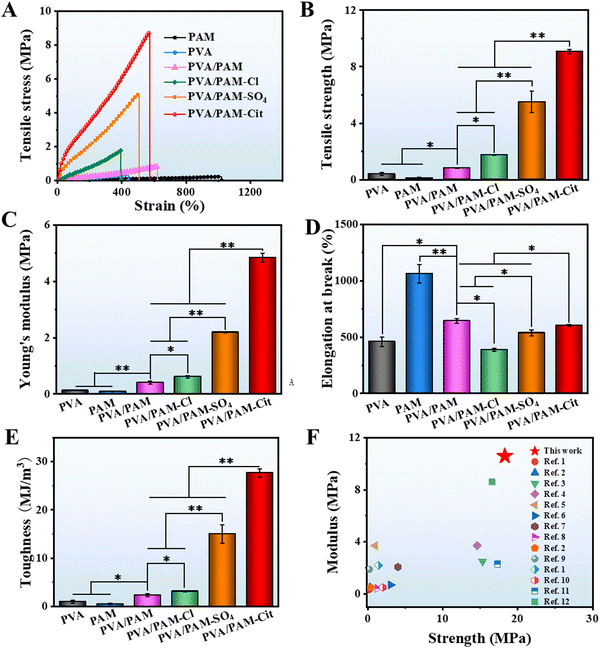 |
| Fig. 4 (A–E) The mechanical properties of PVA, PAM, PVA/PAM, PVA/PAM–Cl, PVA/PAM–SO4, and PVA/PAM–Cit. (A) Tensile stress–strain curve. (B) Tensile strength. (C) Young's modulus. (D) Elongation at break. (E) Toughness. The significance was defined as *p < 0.05 or **p < 0.01, n = 5. (F) Comparisons of the tensile modulus and strength between PVA/PAM–Cit and other previously reported PVA and PVA based DN hydrogels. | |
Fig. 5(A) shows the typical compressive stress–strain curves for PVA, PAM, PVA/PAM, PVA/PAM–Cl, PVA/PAM–SO4, and PVA/PAM–Cit. Their compressive strengths at a strain of 90% are shown in Fig. 5(B). PVA and PAM showed poor compressive properties with compressive strengths of 4.1 ± 0.2 MPa and 2.8 ± 0.2 MPa, respectively, while it was 7.7 ± 0.2 MPa for the PVA/PAM hydrogel. Compared with PVA, the PVA/PAM hydrogel showed a much higher compressive strength by forming the DN structure, which agreed well with the reported result.52 After the Hofmeister series treatment, higher compressive strengths of 8.4 ± 0.3 MPa, 11.5 ± 1.3 MPa, and 50.1 ± 3.0 MPa for PVA/PAM–Cl, PVA/PAM–SO4, and PVA/PAM–Cit could be determined, respectively. The compressive moduli of PVA, PAM, PVA/PAM, PVA/PAM–Cl, PVA/PAM–SO4, and PVA/PAM–Cit were 46.3 ± 1.5 kPa, 80.7 ± 3.1 kPa, 952.9 ± 16.9 kPa, 1.3 ± 0.1 MPa, 1.6 ± 0.1 MPa, and 4.3 ± 0.1 MPa, respectively (Fig. 5(C)), which showed the same trend as that of the compressive strength. All the results showed that both the formation of the DN hydrogel and the Hofmeister effect could enhance the mechanical properties of PVA. As previously depicted, the enhancement may be that the crystalline domains acted as cross-linkers. The crystalline domains may further aggregate and became denser during the compression process resulting in an enhancement in the compressive strength and modulus. PVA/PAM–Cit had the densest structure and χc and thus it showed the best compressive mechanical properties among all the hydrogels. What is more, as seen in Table S1 (ESI†), the compressive mechanical properties were positively correlated with the concentration of Cit3−. After the treatment with 1 M Cit3− solution, PVA/PAM–Cit showed a compressive strength of 11.6 ± 1.3 MPa and a compressive modulus of 1.4 ± 0.5 MPa, respectively, while they (saturated) were 102.3 ± 7.9 MPa and 8.9 ± 0.8 MPa, respectively. To our best knowledge, both the compressive strength and modulus of PVA/PAM–Cit (saturated) surpassed the values of all PVA and PVA based DN hydrogels reported so far (Fig. 5(D) and Table S4, ESI†), suggesting its great potential in articular cartilage replacement. Overall, the Hofmeister ions such as Cl−, SO42− and Cit3− can polarize water molecules in the polymer chains, leading to the dehydration of polymers. As the polymer chains are closer to each other, interactions including hydrogen bonds and hydrophobic interactions prevail and result in the formation of more abundant crystalline regions. That is why the Hofmeister series (Cl−, SO42− and Cit3−) can significantly enhance the mechanical properties of the PVA/PAM hydrogel. Furthermore, the hydration reaction is correlated with the charge density of different ions, which follows the order of Cl− < SO42− < Cit3− (Fig. 5(E)). In this case, after the treatment of Cl−, SO42− and Cit3−, PVA/PAM–Cit showed the best mechanical properties, while the weakest mechanical properties were found for PVA/PAM–Cl. Therefore, PVA/PAM–Cit was selected as the model hydrogel for the following investigation.
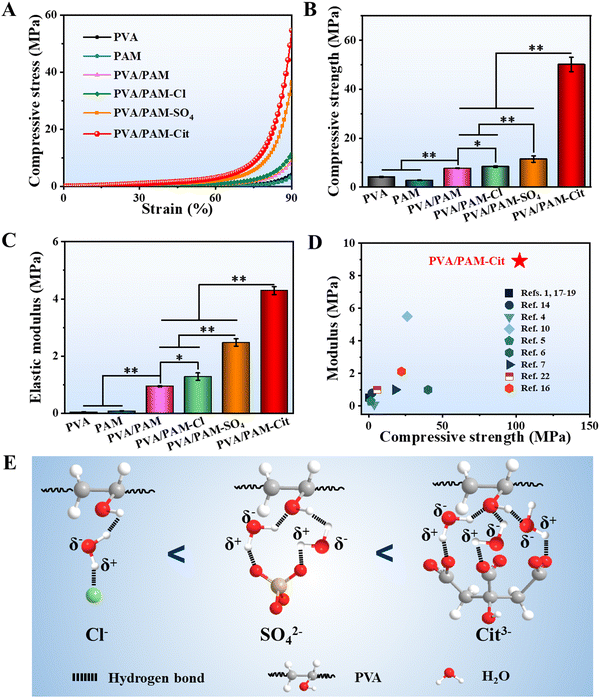 |
| Fig. 5 (A–C) The mechanical properties of PVA, PAM, PVA/PAM, PVA/PAM–Cl, PVA/PAM–SO4, and PVA/PAM–Cit. (A) Compressive stress–strain curve. (B) Compressive strength. (C) Compressive modulus. The significance was defined as *p < 0.05 or **p < 0.01, n = 5. (D) Comparisons of the compressive strength and modulus between PVA/PAM–Cit and other previously reported PVA and PVA based hydrogels. (E) Scheme showing the interaction order of Cl−, SO42− and Cit3− with PVA chains and water. | |
As a bearing articular cartilage, it is necessary to have an outstanding elasticity except for the high strength and toughness. As seen in Fig. 6(A), at a lower compression strain (15%), all the samples exhibit a strain retention of 99.9%. PVA/PAM–Cit showed the best strain retention among all the samples. The strain retention of 99.0% could still be achieved at a strain of 60%, while it was 96.8%, 95.0%, and 97.5% for PVA, PAM, and PVA/PAM at the same strain, respectively. Even at a strain of 90%, PVA/PAM–Cit showed a strain retention of 88.8%. A cycling test for 500 times with a strain of 50% (the maximum force for the machine) for all the samples was further conducted. As shown in Fig. 6(B) and Fig. S2 (ESI†), compared with PAM and PVA, both PVA/PAM–Cit and PVA/PAM were insensitive to repeated compression-relaxation cycles. After 500 cycles, the maximum stress of PVA, PAM, PVA/PAM, and PVA/PAM–Cit remained at around 93.1%, 91.0%, 94.9%, and 99.2%, respectively (Fig. 6(C)), while their remained maximum moduli were 90.3%, 89.3%, 90.6%, and 93.1%, respectively (Fig. 6(D)). The extremely high elasticity for PVA/PAM–Cit was likely due to the formation of the DN structure and the Hofmeister effect. On the one hand, it could be attributed to the energy dissipation of the double-network structures of PVA and PAM, where the first PVA networks effectively dissipated energy as sacrificial bonds, while flexible and stretchable PAM served as a second network to provide elasticity to the hydrogel.21 On the other hand, abundant hydrogen bonds were formed with the help of Cit3−, which not only resulted in strong aggregation and partial crystallization of the polymer chains to further enhance the mechanical properties of PVA but also could serve as a revisable sacrificial physical crosslinking point to achieve the high elasticity.21,50
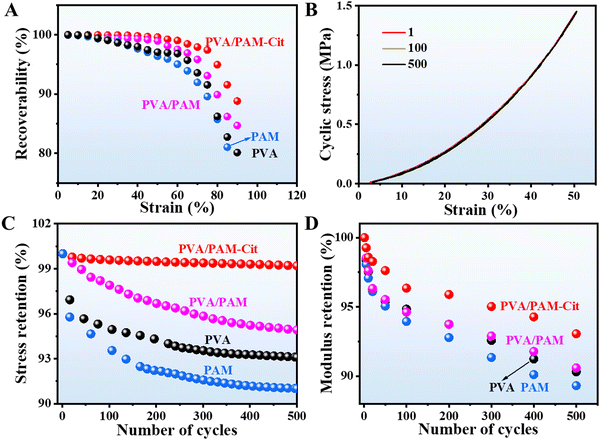 |
| Fig. 6 (A) The strain retention of PVA, PAM, PVA/PAM, and PVA/PAM–Cit at different compressive strains. (B) The compressive cycle curves of PVA/PAM–Cit with the cycles of 1, 100, and 500. (C) and (D) The stress retention (C) and retention (D) of PVA, PAM, PVA/PAM, and PVA/PAM–Cit under 500 cycles. | |
3.5 Biological assessment
The in vitro experiments were performed to evaluate the potential application of the H-PVA/PAM hydrogel as the articular cartilage. Fig. 7(A) shows the fluorescence stained photographs of the chondrocytes in PVA, PAM, PVA/PAM and PVA/PAM–Cit after coculture 1, 3, and 5 days. It could be found that with the increase of culture time, the cell number increased for all the hydrogels, indicating no cytotoxicity for all the materials. Among all the hydrogels, PVA/PAM–Cit showed the best cell proliferation capability. As seen in Fig. 7(B), the CCk-8 results were consistent with the trend shown in live/dead staining photographs. PVA/PAM–Cit did not show significant difference in the proliferation with PVA and PVA/PAM at day 1, except for PAM. On the 3rd day and 5th day, the proliferation of cells on PVA/PAM–Cit was the best among all the hydrogels.
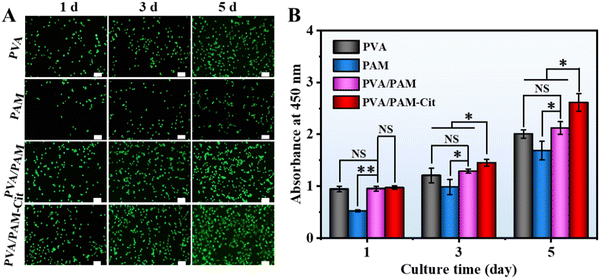 |
| Fig. 7 The FDA/PI staining images (A) and proliferation (B) of chondrocytes in the PVA, PAM, PVA/PAM, and PVA/PAM–Cit. The significance was defined as *p < 0.05 or **p < 0.01, n = 5. Scale bar: 100 μm. | |
SEM characterization was employed to determine the morphologies of chondrocytes (3rd day of coculture) on the PVA, PAM, PVA/PAM, and PVA/PAM–Cit (Fig. 8(A)). It could be found that the chondrocytes adhered to the surfaces of PVA, PAM, PVA/PAM and PVA/PAM–Cit. The cells on all the samples were observed to have an irregular shape with a flattened and extended pseudo-foot. Compared to cell morphologies on PVA, PAM, and PVA/PAM, cells on PVA/PAM–Cit showed more pseudopodia, which enabled cells to sense the morphology on the material's surface, which is beneficial for cell adhesion and spreading.53 The cell morphology and spreading were further investigated by visualizing the actin cytoskeleton. The cytoskeletal–nucleus staining of chondrocytes after being cocultured for 3 days on PVA, PAM, PVA/PAM, and PVA/PAM–Cit is shown in Fig. 8(B). The cells on all samples were spread into irregular shapes. Moreover, significantly coarser and larger amounts of actin stress fibers were observed on PVA/PAM–Cit, while only smaller and reduced bundled stress fibers were observed on PVA, PAM and PVA/PAM. As an essential cytoskeletal protein, actin can transmit the mechanical signals from sites of matrix adhesion to the nucleus and promote the reorganization of cellular architectures, thereby playing an important role in cell morphogenesis, migration, and differentiation.54 Thus, the cell adhered state is related to actin fibers,53 which suggested that PVA/PAM–Cit exhibited superior cell adhesion and spreading behavior. To quantitatively characterize the spreading status of cells on the material, the cell spread area was statistically analyzed with Image J. As shown in Fig. 8(C), the cell spread areas on PVA, PAM, PVA/PAM, and PVA/PAM–Cit were 843.2 ± 52.3 μm2, 444.4 ± 38.8 μm2, 848.8 ± 26.2 μm2, and 1343.3 ± 34.7 μm2, respectively. When compared with PVA, PAM, and PVA/PAM, the cell area of PVA/PAM–Cit increased by 59.3%, 202.3%, and 58.3%, respectively. In addition, PVA/PAM–Cit also showed the maximum nucleus volume (2233.0 ± 170.1 μm3) when compared with PVA (1724.4 ± 136.3 μm3), PAM (1257.4 ± 147.7 μm3), and PVA/PAM (1883.2 ± 110.5 μm3) (Fig. 8(D)). This also proved that PVA/PAM–Cit exhibited the best ability to promote cell adhesion and spreading, which may suggest that the matching mechanical properties (PVA/PAM–Cit) can promote adhesion and spread of cells.
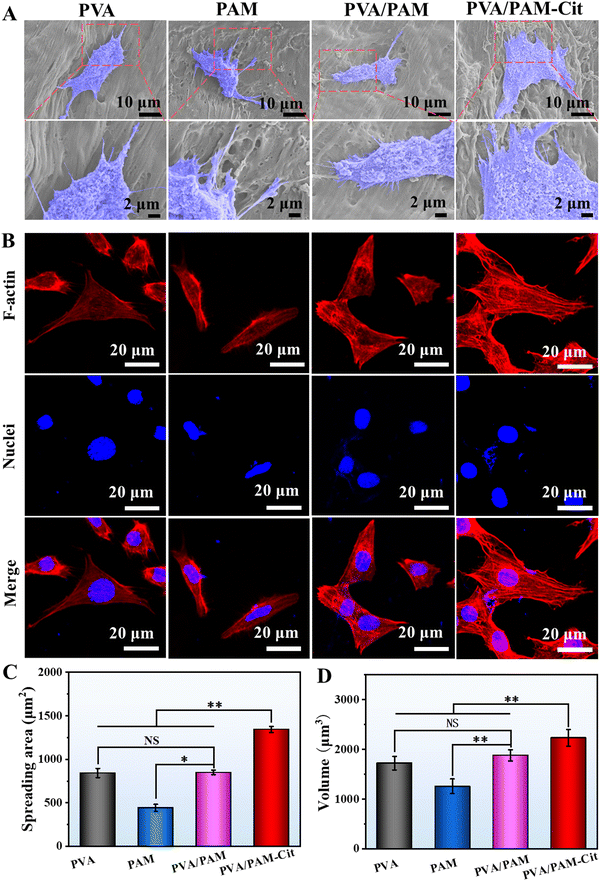 |
| Fig. 8 SEM images (A), the images of AF/DAPI staining (B), spreading area (C), and the volume (D) of chondrocyte co-cultured on PVA, PAM, PVA/PAM, and PVA/PAM–Cit for 3 days. The significance was defined as *p < 0.05 or **p < 0.01, n = 5. | |
Both the porosity and mechanical properties affect cell behavior. It is shown that porosity (>70%) can promote cell proliferation.55 Although the porosity of PAM, PVA/PAM and PVA/PAM–Cit is more than 70%, the excessive porosity of PAM leads to poor mechanical properties. In addition, the differentiation of BMSCs to chondrocytes has been conducted to evaluate the activity of the hydrogels. As seen in Fig. S3 (ESI†), the BMSCs in the PVA/PAM–Cit hydrogel achieved the highest expression of Col-II among all the samples, indicating a positive association between the mechanical properties of the hydrogel and its capacity to promote chondrocyte differentiation.56 Previous studies suggested that the tensile strength and modulus affected cell response to scaffolds57 which is not only a stimulating factor for cell differentiation, but also plays a regulatory role in many other cell behaviors.6 In this work, the mechanical properties of hydrogels might influence the signal pathway of chondrocyte differentiation, potentially promoting differentiation from BMSCs to chondrocytes and enhancing the expression of Col-II in the seeded BMSCs. Therefore, PVA/PAM–Cit shows the best ability to promote cell proliferation (Fig. 9).
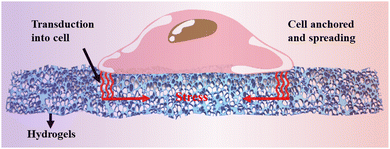 |
| Fig. 9 Diagram of the potential mechanisms of chondrocyte anchored and spread on PVA/PAM–Cit. | |
4. Conclusions
In this work, strong, tough and elastic H-PVA/PAM DN hydrogels were prepared via the combination of in situ polymerization and the Hofmeister effect. The results showed that Hofmeister series ions (Cit3−, SO42−, and Cl−) can affect the microstructure and increase the crystallinity of the PVA/PAM DN hydrogel, in which PVA/PAM–Cit showed the densest microstructure and the highest crystallinity degree. With the help of the DN structure and the Hofmeister effect, PVA/PAM DN hydrogels showed excellent mechanical properties. Especially, for PVA/PAM–Cit, it not only showed extreme high elasticity, but also exhibited the highest strength and modulus, and second highest toughness when compared with the reported PVA and PVA based DN hydrogels so far. What is more, PVA/PAM–Cit could significantly promote the adhesion, spreading and proliferation of chondrocytes. Therefore, the strong, tough, and elastic PVA/PAM hydrogel based on the Hofmeister effect is expected to be an ideal tissue engineering material for articular cartilage replacement.
Author contributions
C. Y.: investigation and writing – original draft. Z. H.: investigation. Y. Z.: methodology. K. R.: visualization. S. L.: investigation. H. L.: formal analysis. Y. W.: project administration and supervision. Q. Z.: conceptualization, writing – original draft, and writing – review and editing.
Conflicts of interest
The authors declare no competing financial interest.
Acknowledgements
This work was supported by the National Natural Science Foundation of China (Grant no. 32260238, 51973058, and 32160229), the Key Research and Development Program of Jiangxi Province (Grant no. 20192ACB80008), and the Key Project of Natural Science Foundation of Jiangxi Province (Grant no. 20202ACBL204013).
References
- J. Cao, Y. Meng, X. Zhao and L. Ye, Ind. Eeg. Chem. Res., 2020, 59, 20359–20370 CrossRef CAS.
- C. A. Tee, J. Han, J. H. P. Hui, E. H. Lee and Z. Yang, Tissue Eng., Part B, 2023, 29, 310–330 CrossRef CAS PubMed.
- J. Xie, W. Wang, R. Zhao, W. Lu, L. Chen, W. Su, M. Zeng and Y. Hu, J. Mater. Sci.: Mater. Med., 2021, 32, 2–13 CrossRef PubMed.
- J. Namkaew, P. Laowpanitchakorn, N. Sawaddee, S. Jirajessada, S. Honsawek and S. Yodmuang, Molecules, 2021, 26, 578–593 CrossRef CAS PubMed.
- K. Rajagopal, V. Dutt, B. Balakumar, S. K. Chilbule, N. Walter, P. D. Nair and V. Madhuri, Indian J. Orthop., 2021, 55, 853–860 CrossRef PubMed.
- M. K. Satapathy, Y. B. Manga, K. K. Ostrikov, W. H. Chiang, A. Pandey, L. Rethi, B. Nyambat, E. Y. Chuang and C. H. Chen, ACS Appl. Mater. Interfaces, 2020, 12, 86–95 CrossRef CAS PubMed.
- Z. Gu, J. Wang, Y. Fu, H. Pan, H. He, Q. Gan and C. Liu, Adv. Fun. Mater., 2023, 33, 2212561 CrossRef CAS.
- X. Wu, M. Zhou, F. Jiang, S. Yin, S. Lin, G. Yang, Y. Lu, W. Zhang and X. Jiang, Bioact. Mater., 2021, 6, 3976–3986 CAS.
- I. Patacho, A. S. Oliveira, P. Nolasco, R. Colaco and A. P. Serro, Ann. Med., 2021, 53, S19–S19 Search PubMed.
- A. S. Oliveira, R. Colaco and A. P. Serro, Ann. Med., 2021, 53, S20–S21 Search PubMed.
- V. M. Sardinha, L. L. Lima, W. D. Belangero, C. A. Zavaglia, V. P. Bavaresco and J. R. Gomes, Wear, 2013, 301, 218–225 CrossRef CAS.
- N. Dadgar, A. Ghiaseddin, S. Irani, S. H. A. Tafti, M. Soufi-Zomorrod and M. Soleimani, J. Biomater. Appl., 2021, 35, 1315–1326 CrossRef CAS PubMed.
- E. Muscolino, A. B. Di Stefano, M. Trapani, M. A. Sabatino, D. Giacomazza, S. Alessi, E. Cammarata, F. Moschella, A. Cordova, F. Toia and C. Dispenza, Int. J. Bio. Macromol., 2022, 222, 1861–1875 CrossRef CAS PubMed.
- W. Liang, Z. Luo and L. Zhou, Chinese J. Chem. Eng., 2020, 28, 598–602 CrossRef CAS.
- L. Cui, W. Tong, H. Zhou, C. Yan, J. Chen and D. Xiong, J. Mater. Sci., 2021, 56, 3935–3946 CrossRef CAS.
- X. Sun, C. Luo and F. Luo, Eur. Polym. J., 2020, 124, 109465 CrossRef CAS.
- X. Li, M. Shu, H. Li, X. Gao, S. Long, T. Hu and C. Wu, RSC Adv., 2018, 8, 16674–16689 RSC.
- M. Sabzi, N. Samadi, F. Abbasi, G. R. Mahdavinia and M. Babaahmadi, Mater. Sci. Eng., C, 2017, 74, 374–381 CrossRef CAS PubMed.
- M. Tavakolizadeh, A. Pourjavadi, M. Ansari, H. Tebyanian, S. J. Seyyed Tabaei, M. Atarod, N. Rabiee, M. Bagherzadeh and R. S. Varma, Green Chem., 2021, 23, 1312–1329 RSC.
- C. J. Little, N. K. Bawolin and X. Chen, Tissue Eng., Part B, 2011, 17, 213–227 CrossRef CAS PubMed.
- Z. Jing, X. Xian, Q. Huang, Q. Chen, P. Hong, Y. Li and A. Shi, New J. Chem., 2020, 44, 10390–10403 RSC.
- Q. Diao, H. Liu and Y. Yang, Gels, 2022, 8, 424–436 CrossRef CAS PubMed.
- C. Jiang, X. Ding, W. Xie and D. Wu, ACS Appl. Mater. Interfaces, 2022, 14, 55143–55154 CrossRef CAS PubMed.
- S. Li, X. Bu, L. Wu, X. Ma, W. Diao, Z. Zhuang and Y. Zhou, Polym. Eng. Sci., 2019, 59, 1657–1666 CrossRef CAS.
- Y. Zhang, M. Song, Y. Diao, B. Li, L. Shi and R. Ran, RSC Adv., 2016, 6, 468–476 Search PubMed.
- K. Ou, X. Dong, C. Qin, X. Ji and J. He, Mater. Sci. Eng., C, 2017, 77, 1017–1026 CrossRef CAS PubMed.
- Y. Cai, J. Shi, F. Liu, H. Li, X. Man and S. Guan, Chinese J. Chem., 2021, 39, 3085–3092 CrossRef CAS.
- A. Yoshida and S. Tsujimura, J. Phys.: Energy, 2021, 3, 024005 CAS.
- Y. Wu, Y. Mu, Y. Luo, C. Menon, Z. Zhou, P. K. Chu and S. P. Feng, Adv. Fun. Mater., 2021, 32, 2110859 CrossRef.
- R. Li, Z. Xu, L. Li, J. Wei, W. Wang, Z. Yan and T. Chen, Chem. Eng. J., 2023, 454, 140261 CrossRef CAS.
- B. Rana, D. J. Fairhurst and K. C. Jena, J. Am. Chem. Soc., 2023, 145, 9646–9654 CrossRef CAS PubMed.
- W. Chen, D. Li, Y. Bu, G. Chen, X. Wan and N. Li, Cellulose, 2020, 27, 1113–1126 CrossRef CAS.
- H. Chen, P. Shi, F. Fan, H. Chen, C. Wu, X. Xu, Z. Wang and M. Du, LWT-Food Sci. Technol., 2020, 121, 108973 CrossRef CAS.
- J. Wei, Y. Yang, F. Pan, K. Yang, Y. Wang, Z. Zeng, Q. Wang and Z. Fu, Compsites, Part A, 2023, 172, 107626 CrossRef CAS.
- S. Wu, M. Hua, Y. Alsaid, Y. Du, Y. Ma, Y. Zhao, C.-Y. Lo, C. Wang, D. Wu, B. Yao, J. Strzalka, H. Zhou, X. Zhu and X. He, Adv. Mater., 2021, 33, 2007829 CrossRef CAS PubMed.
- M. S. Rahman, M. M. Rana, L. S. Spitzhorn, N. Akhtar, M. Z. Hasan, N. Choudhury, T. Fehm, J. T. Czernuszka, J. Adjaye and S. M. Asaduzzaman, Prog. Biomater., 2019, 8, 137–154 CrossRef CAS PubMed.
- E. Budianto and A. Amalia, J. Polym. Eng., 2020, 40, 551–560 CrossRef CAS.
- A. K. Bajpai, A. Vishwakarma and J. Bajpai, Polym. Bull., 2019, 76, 3269–3295 CrossRef CAS.
- G. Patel and M. B. Sureshkumar, Iran. Polym. J., 2014, 23, 153–162 CrossRef CAS.
- A. M. Elbarbary and Y. H. Gad, J. Inorg. Organomet. Polym., 2021, 31, 4103–4125 CrossRef CAS.
- S. Panpinit, S. Pongsomboon, T. Keawin and S. Saengsuwan, React. Funct. Polym., 2020, 156, 104739 CrossRef CAS.
- A. Swaminathan, R. Ravi, M. Sasikumar, M. Dasaiah, G. Hirankumar and S. Ayyasamy, Ionics, 2020, 26, 4113–4128 CrossRef CAS.
- P. Zhou, Y. Luo, Z. Lv, X. Sun, Y. Tian and X. Zhang, Int. J. Biol. Macromol., 2021, 183, 1903–1910 CrossRef CAS PubMed.
- H. M. Ragab and A. Rajeh, J. Mater. Sci.: Mater. Electron., 2020, 31, 16780–16792 CrossRef CAS.
- Y. Lu, R. Jing, Q. Kong and P. Zhu, J. Appl. Polym. Sci., 2014, 131, 39938–39945 CrossRef.
- S. Fanaian, N. Al-Manasir, K. Zhu, A. L. Kjoniksen and B. Nystrom, Colloid Polym. Sci., 2012, 290, 1609–1616 CrossRef CAS.
- S. Mishra, R. Bajpai, R. Katare and A. K. Bajpai, Microsyst. Technol., 2008, 14, 193–198 CrossRef CAS.
- S. El-Gamal and A. M. El Sayed, J. Compos. Mater., 2019, 53, 2831–2847 CrossRef CAS.
- Y. Zhang, D. Li, Y. Huang, S. Long, H. Li and X. Li, Macromol. Mater. Eng., 2020, 305, 1900450 CrossRef CAS.
- X. Zhao, Soft Matter, 2014, 10, 672–687 RSC.
- S. Lin, J. Liu, X. Liu and X. Zhao, Proc. Acad. Natl. Sci. U. S. A., 2019, 116, 10244–10249 CrossRef CAS PubMed.
- Y. Wu, C. Yu, M. Xing, L. Wang and G. Guan, J. Biomed. Mater. Res., Part B, 2020, 108, 117–127 CrossRef CAS PubMed.
- Y. Hou, W. Xie, L. Yu, L. C. Camacho, C. Nie, M. Zhang, H. Rainer and Q. Wei, Small, 2020, 16, 1905422 CrossRef CAS PubMed.
- J. Park, P. Kravchuk, A. Krishnaprasad, T. Roy and E. H. Kang, Int. J. Mol. Sci., 2022, 23, 509–522 CrossRef CAS PubMed.
- P. Danilevicius, L. Georgiadi, C. J. Pateman, F. Claeyssens, M. Chatzinikolaidou and M. Farsari, Appl. Surf. Sci., 2015, 336, 2–10 CrossRef CAS.
- N. Zhang, Y. Wang, J. Zhang, J. Guo and J. He, Acta Biomater., 2021, 135, 304–317 CrossRef CAS PubMed.
- M. A. Johns, Y. Bae, F. E. G. Guimaraes, E. M. Lanzoni, C. A. R. Costa, P. M. Murray, C. Deneke, F. Galembeck, J. L. Scott and R. I. Sharma, ACS Omega, 2018, 3, 937–945 CrossRef CAS PubMed.
- J. Yu, K. Xu, X. Chen, X. Zhao, Y. Yang, D. Chu, Y. Xu, Q. Zhang, Y. Zhang and Y. Cheng, Biomacromolecules, 2021, 3, 1297–1304 CrossRef PubMed.
|
This journal is © The Royal Society of Chemistry 2024 |
Click here to see how this site uses Cookies. View our privacy policy here.