DOI:
10.1039/D4TB01703J
(Paper)
J. Mater. Chem. B, 2024,
12, 11381-11388
A DNA nanowire based-DNAzyme walker for amplified imaging of microRNA in tumor cells†
Received
1st August 2024
, Accepted 7th October 2024
First published on 15th October 2024
Abstract
Sensitive imaging of microRNAs (miRNAs) in tumor cells holds great significance in the domains of pathology, drug development, and personalized diagnosis and treatment. DNA nanostructures possess excellent biostability and programmability and are suitable as carriers for intracellular imaging probes. With its highly controllable motion mechanism and remarkable target recognition specificity, the DNA walker is an ideal tool for living cell imaging. Here, we report a DNA nanowire based-DNAzyme Walker (D-Walker), which loads the DNAzyme based-molecular beacon (D-MB) onto DNA nanowires (NWs) functionalized with aptamers. The experimental results demonstrated that the intracellular target miRNA can specifically activate the pre-locked DNAzyme through a strand displacement reaction, thereby triggering the cleavage of its substrate molecular beacon (MB) and subsequent fluorescence emission. NWs decorated with aptamers can effectively prevent the degradation of the D-Walker by nuclease, and can enter target cells without any transfection reagents, which enhances the stability and reliability of cell imaging. Furthermore, the D-Walker exhibited a remarkable sensitivity with a limit of detection (LOD) of 61 pM and was capable of distinguishing miRNA-21 from other closely related family members. This study provides a novel strategy for intracellular miRNA imaging, offering a promising tool for cancer diagnosis and treatment.
Introduction
MicroRNAs (miRNAs) are a class of endogenous non-coding RNA molecules (approximately 22 nucleotides)1 which are involved in various biological processes such as cell proliferation, differentiation and apoptosis.2,3 Increasing evidence suggests that aberrant expression of miRNA-21 is closely associated with the occurrence and progression of cancer,4,5 indicating the potential value of miRNA-21 as an important biomarker for cancer. However, miRNA sensing is challenging due to short sequences, low abundance and high sequence homology.6,7 Imaging of intracellular miRNAs can distinguish cancer cells at the local or even single-cell level, revealing pathophysiological information before abnormal cell morphology, and providing valuable information for early diagnosis and prognosis of cancer.5,8,9 At present, many methods for imaging miRNA have been reported, for example, strand replacement reaction-based and DNAzyme-mediated DNA walkers,10–12 palindrome-catalyzed DNA assembly strategies,13,14 frame nucleic acid binding catalytic hairpin assembly amplifiers15 and various other sensing platforms.16 Almost all of these methods have one or more inherent disadvantages, such as complex and time-consuming preparation, low cell permeation efficiency, susceptibility to nuclease degradation and slow reaction kinetics. Hence, there is a need to develop new, straightforward, and efficient platforms for miRNA imaging.
In recent years, DNA nanotechnology has emerged as a burgeoning research focus, widely used in biomedical fields such as drug delivery,17,18 detection,10,19–21 bioimaging22–24 and bio-interference.25–27 DNA nanostructures have many advantages, such as programmability, biocompatibility, and cell permeability.28 DNA nanowires, which can be constructed using various methods such as continuous hybridization with unit motifs29–31 or rolling circle amplification (RCA),32 have been employed for biosensing and cargo delivery. Due to simple and diverse construction methods, as well as excellent anti-degradation ability, DNA nanowires are ideally suited as probe carriers for intracellular biomolecular imaging.
To achieve precise localization of cancer cells, various strategies including the hybrid chain reaction (HCR),33 catalytic hairpin assembly (CHA),34 and DNAzyme35 have been employed. DNAzymes, as externally selected single-stranded DNA molecules, are frequently employed as components for catalyzing DNA walker motion or achieving specific functionalities due to their unique intrinsic catalytic activity and high controllability.36–39 However, the application of DNAzyme-based probes inside cells still encounters significant obstacles. First, DNAzymes and their substrates typically face the challenge of restricted entry into cells, which hinders in situ amplification reactions. Second, the stability of these probes can be compromised within the complex biological environment,40–42 potentially leading to false positives. While nanoparticles and metal organic frameworks (MOFs) have been utilized as delivery agents to facilitate DNAzyme uptake,39,43–45 the complex preparation process and potential biotoxicity have indeed become major challenges.
Inspired by motor proteins,46 researchers have constructed numerous DNA walkers with the ability to autonomously move precisely along predefined paths. These DNA walkers have been designed to enable various functions, including signal amplification,24,47 cargo delivery,48 and molecular computation.49–51 One drawback that cannot be ignored is the complexity involved in the preparation process and the potential toxicity associated with certain materials. As a result, constructing a walker solely composed of DNA offers a viable solution for imaging intracellular biomolecules.
Motivated by the aforementioned challenges, a biocompatibility DNA nanowire based-DNAzyme walker (D-Walker) was constructed for intracellular miRNA imaging. We used DNAzyme to design a molecular beacon (D-MB) that can rapidly respond to miRNA-21. In order to address the challenge of poor cellular uptake and limited stability exhibited by simple probes, a previously reported DNA nanostructure31 was slightly modified to construct the probe vector. The D-Walker was obtained by assembling the D-MB and the hairpin-shaped substrate part without DNAzyme (MB) on the NW decorated with aptamer sgc8 (Apt) in a certain proportion. Due to the excellent stability of the NW and the precise recognition ability of the aptamer it carries, the D-Walker can be specifically and efficiently taken up by tumor cells, while effectively resisting the degradation of nucleases. When the target miRNA-21 is absent, the orbital MB of the D-Walker cannot be burned, so the FAM group on MB is quenched due to its proximity to Dabcyl. When the target miRNA is present, the cleaving activity of DNAzyme is activated through a toehold-initiated strand displacement reaction, completing the transduction process from the binding event of the target and the Lock-d to the fluorescence emission, and the “walking” behavior of continuous cleaving of different MB by the D-Walker realizes efficient signal amplification. Utilizing the D-Walker, sensitive imaging of miRNA-21 within tumor cells can be achieved, and even cells containing different levels of miRNA-21 can be distinguished by fluorescence intensity, representing a promising tool for early cancer diagnosis, progression monitoring, and therapeutic efficacy evaluation.
Experimental section
Reagents and materials
All DNA oligonucleotides used in the experiment were synthesized by Shanghai Sangon Biological Engineering Technology Service Co., Ltd (Shanghai, China), and these base sequences are shown in Table S1 (ESI†). These oligonucleotides were dissolved in ultrapure water (
18 MΩ cm resistivity, Milli-Q, Millipore) and adjusted to 10 μM as a stock solution at 4 °C for future use.
Loading buffer (6× and 2×), Dulbecco's Modified Eagle's Medium (DMEM) and PBS (pH = 7.2–7.4) were purchased from Beijing Solarbio Science & Technology Co., Ltd (Beijing, China). DNase I and 17β-Estradiol (E2) were bought from Beyotime Biotechnology Co., Ltd (Shanghai, China). Lip2000Transfection Reagent, Calcium Phosphate Cell Transfection Kit, Enhanced Cell Counting Kit-8 (CCK-8), Opti-MEM I, Hoechst 33342, DNA Marker and SYBR Green I were obtained from BioSharp (Beijing, China). Penicillin–streptomycin (PS) and fetal bovine serum (FBS) purchased from Gibco (USA). HeLa cells (human cervical carcinoma cell line) and MCF-7 cells (human breast cancer cell line) were provided by Pricella (Wuhan, China). All other chemicals were of analytical grade without further purification.
Atomic force microscopy (AFM) characterization
D-Walker solution (0.5 μM) was diluted 5 times with 1 × TAMg buffer, and 8 μL of the resulting solution was dropped onto a mica surface for 20 min. The mica surface was then carefully washed with ultra-pure water several times. Subsequently, the sample was dried in an oven at 37 °C for 30 min before being scanned by an atomic force microscope. AFM scanning was performed on a MultiMode 8 atomic force microscope (Bruker, Germany) in ScanAsyst Mode (Cantilever Type: ScanAsyst-Air, Bruker, Germany).
Cell culture
HeLa and MCF-7 cells were cultured in Dulbecco's Modified Eagle Medium (DMEM) supplemented with 10% Fetal Bovine Serum (FBS) and 1% penicillin–streptomycin solution (PS). Prior to confocal imaging analysis, all cells were maintained at 37 °C in a humidified incubator with 5% CO2.
Confocal fluorescence imaging
To evaluate the intracellular imaging capabilities of the D-MB, HeLa cells were cultured in 35 mm confocal laser culture dishes for 24 h until reaching 80% confluency. Following PBS (137 mM NaCl, 2.7 mM KCl, 10 mM Na2HPO4, 2 mM KH2PO4, pH 7.4) washing, HeLa cells were separately incubated with pre-prepared D-MB, the D-Walker with the Random replaced the an aptamer (R-Walker) and D-Walker (0.5 μM each) for 4 h. After removal of the incubation materials by PBS washing, cellular fixation was performed using 4% paraformaldehyde solution for 15 min, succeeded by another round of PBS washing. Subsequently, cellular staining was conducted using 10 μg mL−1 Hoechst 33342 staining solution for 15 min. Prior to fluorescence imaging, cells underwent a final wash with PBS.
For the assessment of miRNA-21 expression levels at various stages of cell growth, MCF-7 cells and E2-treated (17β-Estradiol) MCF-7 cells were utilized. These cells were individually incubated with the D-Walker prepared using Cy5-modified S1 (S1-Cy5) instead of S1 for 4 h. Subsequently, the same procedural steps described above were followed prior to fluorescence imaging.
Results and discussion
Design and working principle of the D-Walker
The oligonucleotide sequences used for the construction of the D-Walker are described in Table S1 (ESI†), while the stepwise assembly process is described in Scheme 1. As shown in Scheme 1, Na+-dependent DNAzyme hybridizes with Lock-d to form Locked-DNAzyme, which is in an inactivated state. The molecular beacon (MB) is formed by partial complementarity between a 5'-fluorophore (FAM)-labeled strand (F-S) and a 3'-quencher (Dabcyl)-labeled strand (D-S), where F-S contains an adenosine ribonucleotide (rA) in the loop segment as a cleavable site. MB was partially hybridized with Locked-DNAzyme to form D-MB. NW, which served as the carriers for the D-Walker, was synthesized by annealing four single-stranded DNAs (S1, S2, S3 and S4). S1 and S2 are partially hybridized to form unit 1 (U-1), and S3 and S4 are partially hybridized to form unit 2 (U-2). Continuous hybridization between U-1 and U-2 can be carried out through the arms at both ends to form the nanowire structure. With the linker's assistance, D-MB and MB can be assembled on one side of the NW by hybridizing with S2 or S4, while aptamer sgc8 is assembled directly on the other side of NW by hybridizing with S1 or S3, respectively, therefore the D-Walker is built in this way. Scheme S1 (ESI†) illustrates hybridization between nucleic acid sequences that make up the parts of the D-Walker.
 |
| Scheme 1 Schematic illustration of the stepwise assembly of a DNA nanowire (NW) based-DNAzyme Walker (D-Walker) and its mechanism for amplified imaging of miRNA-21 in tumor cells. | |
The NW offer significant advantages for achieving miRNA imaging in live cells due to its excellent biocompatibility, ability to penetrate the cell cytoplasm without the need for transfection agents and high resistance to intracellular degradation.32,52,53 The introduction of aptamer can enable the D-Walker to have tumor cell targeting and thus achieve accurate imaging of intracellular targets. In the absence of target miRNA-21, the hybridization of F-S and D-S made FAM and dabcyl close to each other, leading to efficient fluorescence quenching. Even in complex intracellular environments, the D-Walker does not exhibit significant fluorescence changes due to the excellent biostability conferred by the NW. When target miRNA-21 is present, the target-lock hybridization activates the DNAzyme and the active secondary structure forms in the catalytic core. Subsequently, the activated DNAzyme cleaves the substrate at the ribonucleotide site with the assistance of Na+. Due to the lower affinity, the FAM-labeled short single-stranded DNA is cleaved off, resulting in a pronounced increase in fluorescence intensity. Meanwhile, the active DNAzyme is released and binds to adjacent MB or D-MB to drive another cycle of cleaving and walking. This leads to signal amplification, enabling accurate and sensitive detection of target miRNA in live cells.
Construction of the D-Walker and feasibility for target miRNA-21 detection
The assembly of nanowires was first validated by gel electrophoresis analysis, and the experimental results are shown in Fig. 1A. Lanes 1–4 correspond to the four single-stranded DNAs, while lanes 5 and 6 indicate that S1 and S2 can assemble to form Unit-1, and S3 and S4 can assemble to form Unit-2 similarly. Lane 7 shows the combination of Unit-1 and Unit-2, indicating that S1, S2, S3, and S4 successfully self-assembled into the nanowire structure. The length of the nanowires was controlled by adjusting the U-2/U-1 ratio, and the experimental details are shown in Fig. S1 (ESI†). Further characterization of the DNA nanowires was performed using atomic force microscopy (AFM). As shown in Fig. 1B, in stark contrast to the U-1, the NWs have a linear structure. The average length of U-1 and NW is 23 nm and 167 nm, respectively. The length of U-2 and U-1 is theoretically the same, therefore, it can be inferred that the number of U-1 and U-2 monomers in NW is about 3.6, which was calculated from the equation: 167/46. The AFM images provide strong evidence for the successful synthesis of linear DNA nanostructures of NW. As shown in Fig. S2 (ESI†), both S1 and S3 can hybridize with the Apt. Subsequently, the assembly of the D-Walker was validated, and the results are shown in Fig. 1C. Lane 3, lane 4 and lane 6 demonstrate that the Linker can hybridize with S2, S4 and MB, respectively. Lane 8 and lane 10 indicate that the Linker can connect S2 and S4 with D-MB, respectively. The electrophoretic mobility of the band in lane 13 was significantly reduced compared with lane 12, indicating the successful assembly of the D-Walker.
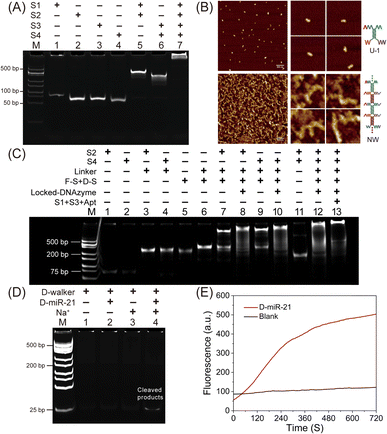 |
| Fig. 1 Assembly of the D-walker and feasibility verification of its response to miRNA-21. (A) Native PAGE analysis of the assembly of DNA nanowires (NWs). (B) AFM images of NWs and one of its units (U-1). The furthest right panel shows the diagrams of the corresponding DNA nanostructures. Scale bar: 500 nm. (C) Native PAGE analysis to verify the stepwise assembly of the D-Walker. (D) Native PAGE analysis to verify the response of the D-Walker to target D-miR-21. (E) Real-time monitoring of the fluorescence intensity of the D-Walker in the presence of D-miR-21. | |
Due to its upregulation in various cancer cells and its recognized oncogenic role, miRNA-21 is frequently selected as a target.54–56 First, PAGE analysis was used to verify the Locked-DNAzyme can be unlocked via toehold-initiated strand displacement reaction based on the target. Here, D-miR-21, a DNA analog of miR-21, was used for verification in vitro. The results are shown in Fig. S3 (ESI†), indicating the Lock-d strand readily hybridized with the target D-miR-21, thereby activating the previously inactive DNAzyme. Further evaluation of the response of the D-Walker to miR-21 in vitro is shown in Fig. 1D. To facilitate observation of the experimental results, no DNA fluorescent dye was added to the sample. Consequently, the simple D-Walker did not form distinct bands in the PAGE gel, as the FAM group modified on the MB was in close proximity to the quencher Dabcyl. Lane 4 exhibited a clear band, indicating that the simultaneous presence of D-miR-21 and Na+ activated the DNAzyme to cleave the substrate, allowing the FAM-labeled short DNA strand to detach from the MB and move away from Dabcyl, resulting in bright bands. In contrast, no visible bands were formed when only the target (lane 2) or Na+ (lane 3) were present. These results suggest that the D-Walker can effectively respond to D-miR-21 and cleave the substrate in the presence of the cofactor Na+.
Fig. 1E illustrates the dynamic alterations in fluorescence emitted by the D-Walker in response to the D-miR-21 during real-time fluorescence monitoring, showing a trend of rapid initial increase followed by a gradual plateauing in fluorescence intensity compared to the blank control group. Meanwhile, as shown in Fig. S4 (ESI†), similar results were achieved with D-MB, suggesting that having NW as a carrier of D-MB does not affect its ability to detect D-miR-21. This confirms the feasibility of the constructed D-Walker for detecting miR-21.
Evaluation of the D-Walker for in vitro miRNA detection
Following the completion of the feasibility analysis for target miRNA detection, we systematically optimized the detection conditions. As a walking subject, DNAzyme is very important for the generation of detection signals. At the same time, the proportion of DNAzyme and its substrate assembly on the NW needs to be explored in detail. Therefore, the ratio of DNAzyme-containing D-MB to hairpin substrate MB assembled on NW was investigated, using the relative fluorescence intensity (F/F0) induced by the target at a specific concentration (50 nM) as a measure. As shown in Fig. S5A (ESI†), the optimal molar ratio of D-MB to MB was determined to be 1
:
4. On this basis, we optimized the number of bases in the locking strand. Six kinds of locking strands with a different number of bases were used in the optimization experiment, among which Lock-d contained 22 bases that could complement DNAzyme and showed the largest relative fluorescence intensity (F/F0) (Fig. S5B, ESI†), so it was identified as the most suitable locking strand to be used in the subsequent experiment. Subsequently, the fluorescence variations of the D-Walker were meticulously analyzed under different concentrations of cofactor Na+, as a result, an optimal concentration of 250 mM Na+ was chosen for in vitro analysis (Fig. S5C, ESI†). The concentration of intracellular Na+ in almost all animal cells is about 10–40 mM.57 It has been verified that Na+ specific DNAzyme can work in HeLa cells under normal culture conditions.58 Thus, it is anticipated that the abundant and stable concentration of intracellular Na+ could specifically fuel the D-Walker. Finally, the reaction time between the D-walker and the target was optimized. Through continuous monitoring of the fluorescence intensity generated by the D-Walker at different reaction times, the optimal reaction time was determined to be 4 h (Fig. S5D, ESI†). Therefore, the D-Walker probe exhibited a high signal-to-noise ratio for miR-21 detection, which minimized the occurrence of false positive signals resulting from non-specific assembly.
On the basis of the established optimum conditions, the sensitivity of the D-Walker for D-miR-21 detection was validated by measuring the fluorescence intensity across a range of D-miR-21 concentrations from 0 to 20 nM (Fig. 2A). The relationship between fluorescence intensity and the concentration of D-miR-21 is illustrated in Fig. 2B. It is evident that the fluorescence intensity increases with the target concentration, exhibiting an excellent linear correlation between fluorescence values and D-miR-21 concentration. The LOD was determined to be 61 pM using 3σ method, which significantly outperforms other sensing methods that rely on DNAzyme cleavage.38,59–61
 |
| Fig. 2 The assay performance of the D-Walker in vitro. (A) Fluorescence intensity of the D-Walker upon the addition of DNA targets at different concentrations. (B) Dynamic relationship between fluorescence intensity at 520 nm and D-miR-21 concentration, ranging from 0 to 20 nM, accompanied by a linear regression equation, where F represents fluorescence intensity, and C denotes D-miR-21 concentration. (C) Relative fluorescence intensity of mutant targets with different number of mismatched bases incubated with the D-Walker. (D) Relative fluorescence intensity of DNA mimics of non-target miRNAs incubated with the D-Walker. For (C) and (D), relative fluorescence intensity was calculated by (F − F0)/(Ft − F0) × 100%, and the relative fluorescence intensity of D-miR-21 was defined as 100%. F represents the fluorescence intensity induced by mutant targets or DNA mimics of non-target miRNAs, and Ft and F0 represent the fluorescence intensity induced by the target D-miR-21 and blank, respectively. Error bars represent standard deviations from three parallel assays. | |
One of the crucial prerequisites for the applicability of newly developed detection methods or probes in biomedical diagnostics is the ability to accurately identify target molecules and distinguish the complementary target from mismatched nontargets, ensuring their reliability and accuracy in practical applications. To confirm the specificity of the D-Walker, we designed several non-target sequences containing mismatched bases and collected corresponding fluorescence signals individually (Fig. 2C). In the reaction with the D-Walker, the target D-miR-21 induced remarkably strong fluorescence intensity. Meanwhile, the fluorescence intensity induced by the mismatched sequences include mis-2, mis-3, mis-4 and mis-5 was weak or even negligible, and the fluorescence intensity induced by mis-1 is about 50% of that by the fully matched target, indicating a single-base discrimination capability of the D-Walker. Usually, multiple miRNAs coexist within cells, therefore, DNA analogs of various miRNAs such as let-7d, miR-429, miR-200 and miR-141 were used to further investigate the detection specificity of the D-Walker (Fig. 2D). It can be observed that the fluorescence signals induced by all nontarget molecules were significantly fainter compared to those of D-miR-21, indicating that the D-Walker has excellent specificity in detecting the target.
D-Walker's molecular mechanism of signal amplification
As a detector based on the DNAzyme walker, its walking ability not only determines its sensitivity and accuracy in detecting target molecules but also directly affects the adaptability and reliability in complex biological environments. In theory, the D-Walker achieves “walking” by continuously cleaving the substrate MB assembled on NW, and realizes signal transduction and amplification through “walking”. To ensure that the D-Walker can function effectively in practical applications, it is imperative to confirm that this detector can move according to the anticipated pathways and mechanisms.
For verification, a new D-Walker analogue, VD-Walker, was constructed, as shown in Fig. 3A. Locked-DNAzyme assembled on a non-FAM modified substrate (ND-MB) made of DNA sequence substrate and D-S, while other MBs contained FAM groups. In the absence of D-miR-21, DNAzyme does not form an active conformation due to a Lock-d blockade, so the VD-MB cannot be cleaved, resulting in no fluorescence signal. In the presence of D-miR-21, if DNAzyme could not walk according to the preset mechanism, it would stop its activity after cleaving ND-MB, and therefore could not generate fluorescence signals. In contrast, only DNAzyme with a continuous walking ability can cleave MB after cleaving D-MB and then produce a large amount of fluorescence. The experimental results in Fig. 3B and C corroborate this hypothesis, affirming the walking capability of the D-Walker.
 |
| Fig. 3 Verification of the molecular mechanism for signal amplification and stability evaluation of the D-Walker. (A) Schematic illustration of verifying D-Walker walking in the presence of miR-21. The D-MB without a FAM group named ND-MB was assembled by using the sequence substrate instead of F-S to construct a D-Walker for verification (V-D-Walker) according to the established method. The structure diagram of ND-MB is shown in the solid red box; the blue dashed box is a partial structural diagram used to demonstrate the reaction process on the right side. (B) Fluorescence intensity of the V-D-Walker in the presence and absence of D-miR-21. (C) Quantitative evaluation of the fluorescence intensity of the samples in (B). (D) The dPAGE analysis verified the stability of the D-Walker incubated with DNase I (1 U mL−1) at 37 °C. (E) Quantitative evaluation of the fluorescence intensity of the bands in (D) by ImageJ software. The error bars represent the standard deviations from three parallel assays. | |
Anti-degradation assay of the D-Walker
To achieve high-quality imaging of intracellular biomolecules, an important premise is that the probe has good biological stability. Since nucleic acid probes are prone to degradation by nucleases in complex biological environments, resulting in false positive detection results, we investigated the stability of the D-Walker in a liquid environment containing DNase I by 10% denatured polyacrylamide gel electrophoresis (dPAGE) analysis. No additional fluorescent dyes were used for imaging the dPAGE, and the fluorescence of the bands came from the FAM groups labeled on the F-S. As shown in Fig. 3D, it is evident that D-MB gradually degrades over time, with its band almost completely degraded at 30 min and disappearing entirely by 120 min, indicating complete degradation. In contrast, the fluorescence intensity of D-Walker's bands showed little visible change even after a reaction time of up to 120 minutes. At the same time, this result was clearly quantified in Fig. 3E. This suggests that DNA NWs decorated with aptamers improve the stability of F-S in the face of nuclease degradation. This may be due to the steric hindrance generated by the unidimensional rigid structure that prevents nucleases from approaching the surface of the DNA NWs. Specifically, the arrangement of hairpin aptamers and Locked-DNAzyme on the DNA NWs forms an effective protective shell for F-S, preventing the approach of various nucleases. To further evaluate this performance, we assessed the stability of the D-Walker in cell lysate (Fig. S6, ESI†). It demonstrates that using nanowires as a carrier endows the D-Walker with significant biological stability, ensuring its reliability for imaging in complex cellular environments.
Fluorescence imaging of intracellular miRNA-21
Efficient uptake of sensor probes by target cells is essential for intracellular biomolecular imaging. Simple DNA probes with negative charges are easily affected by electrostatic repulsion from cells, thus exhibiting low cellular uptake efficiency.62 Therefore cationic polymers and liposomes are often used to transfect nucleic acid probes to assist in intracellular imaging,13,63,64 however, potential hazards such as toxicity, immunogenicity, and inability to be completely degraded are unavoidable.65 To solve this problem, the aptamer sgc8 targeting PTK-7 was introduced into the DNA NW carrier in this study, hoping to improve the cell uptake efficiency of nucleic acid probes by tumor cell associated receptor mediated-cell internalization.
To evaluate the cellular uptake of DNA probes, the D-Walker was compared with Lipo2000, and calcium phosphate transfection groups. As shown in Fig. S7 (ESI†), the fluorescence intensity of the D-MB group was extremely weak, while the D-Walker group showed fluorescence levels comparable to Lipo2000 and calcium phosphate transfection groups, all exhibiting strong FAM signals. This suggests that aptamer-decorated DNA NWs introduced in the D-Walker can effectively improve cell uptake efficiency. The results of quantitative analysis (right panel) further confirm this conclusion. In order to evaluate the imaging effect of the D-Walker on miRNA-21 in target cells, HeLa cells were used in confocal fluorescence imaging experiments. At the same time, to validate the role of aptamer in enhancing the uptake of the D-Walker by target tumor cells, a counterpart named R-Walker was constructed by substituting Apt with Random based on the D-Walker. The confocal fluorescence images (Fig. 4A) of HeLa cells incubated with the D-Walker and other counterparts revealed that the fluorescence intensity of D-MB and R-Walker treatment groups were extremely weak, almost negligible, while a strong FAM fluorescence signal could be distinctly observed with the D-Walker treatment group, as evidenced by quantitative analysis of fluorescence intensity (right panel). The above phenomenon confirmed that the introduction of Apt significantly promoted the cell uptake efficiency of the D-Walker, and thereby achieved sensitive imaging in tumor cells. The further analysis of cellular internalization efficiency of it was conducted by flow cytometry, and the fluorescence signal of the D-Walker was higher than that of the other control groups (Fig. 4B and C), which was consistent with the confocal fluorescence images.
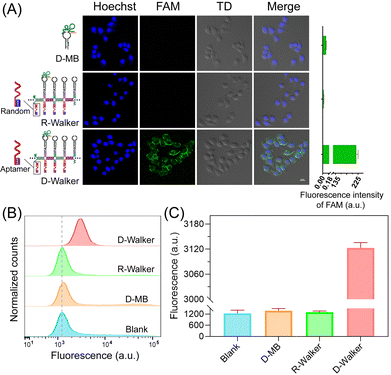 |
| Fig. 4 Efficacy evaluation of D-Walker targeting tumor cells in response to miRNA-21. (A) Confocal imaging of HeLa cells incubated with D-MB, R-Walker, and D-Walker, respectively. Scale bar: 20 μm. The right panel shows the quantitative evaluation of FAM fluorescence intensity by ImageJ software. (B) Flow cytometry analysis of the samples in panel A. (C) Quantitative evaluation of the fluorescence intensity of the samples in (B) by ImageJ software. Error bars represent standard deviations from three parallel assays. | |
To investigate the performance of the D-Walker in distinguishing between different levels of miRNA in cells, MCF-7 cells and E2-treated MCF-7 cells were used for exploration to eliminate the interference caused by the different uptake efficiency of the D-Walker by different cells. On the basis of the D-Walker, S1 was replaced with S1-Cy5 to prepare a Cy5-labeled D-Walker, which was incubated with E2-treated and untreated MCF-7 cells respectively for 4 h under the same conditions, and then confocal fluorescence imaging was performed.
As illustrated in Fig. 5, there was virtually no discrepancy in the Cy5 fluorescence intensity between pre- and post-E2-treated MCF-7 cells, suggesting the uptake efficiency of the D-Walker by the two groups of cells was similar. In addition, the FAM fluorescence intensity in E2-treated MCF-7 cells was significantly weaker than that in untreated MCF-7 cells, which was due to decreased levels of miR-21 in E2-treated MCF-7 cells according to previous reports.12,14 The qRT-PCR further confirmed that miRNA expression in E2-treated MCF-7 cells decreased to 56.6% (Fig. S8 and Table S2, ESI†). It was confirmed that the D-Walker could accurately identify tumor cells with different expression levels of miR-21.
 |
| Fig. 5 Confocal fluorescence imaging of estrogen (E2)-treated or untreated MCF-7 cells incubated with the D-Walker. Scale bar: 20 μm. The right panel shows quantitative evaluation of FAM and Cy5 fluorescence intensity by ImageJ software. Error bars represent standard deviations from three parallel assays. | |
The cytotoxicity of the D-Walker was analyzed by CCK-8 assay. It showed that cell survival rates were all above 90%, indicating minimal toxic effects on cells (Fig. S9A, ESI†). In addition, as shown in Fig. S9B (ESI†), the average cell viability of the D-Walker group reached 92%, while the cell viability of the two transfection groups significantly decreased, with survival rates of 26%. This indicates that the toxicity of the D-Walker to cell is negligible. Compared with other studies, the D-Walker was found to have good sensitivity and biostability, and the most outstanding feature is that it can penetrate into cells without the help of other transfection reagents or organic/inorganic materials, which further ensures biosafety (Table S3, ESI†).
Conclusions
In conclusion, a DNA nanowire based-DNAzyme walker was successfully constructed for high-sensitivity imaging of miRNA-21 in tumor cells. The following features have been demonstrated in this detection platform. (1) The Na+-dependent DNAzyme was employed based on the abundant presence of Na+ in biological systems, eliminating the need for additional supplementation. (2) Due to the presence of DNA NW-based carrier equipped with aptamers, sensing probes could enter live cells without any transfection autonomously, which avoided potential adverse reactions. (3) The D-Walker exhibits outstanding enzymatic resistance biostability. The NW-based carrier endows the probes with good resistance to DNase I degradation, increasing stability. (4) The D-Walker has good sensitivity and specificity, and can accurately distinguish miRNA-21 with different expression levels in cells. Due to the programmability of detection probe DNA sequences, it is possible to achieve imaging of multiple target miRNAs by designing specific sequences. This provides a novel approach for monitoring low-abundance biomarkers in live cells. Therefore, this detection platform is anticipated to serve as a valuable tool for tumor prevention, early diagnosis, and prognosis.
Data availability
The data supporting this article have been included as part of the ESI.†
Conflicts of interest
The authors declare that they have no known competing financial interests or personal relationships that could have appeared to influence the work reported in this paper.
Acknowledgements
This work was supported by the National Natural Science Foundation of China (Grant No. 22274068 and 22076073), the Natural Science Foundation of Shandong Province (No. ZR2023MB139 and ZR2023QB057), the Major Basic Research Project of Natural Science Foundation of Shandong Province (No. ZR2023ZD27), the Key R&D Projects of Linyi City (No. 2022022), and the high-level talent research start-up fund of Linyi University (No. Z6122023).
Notes and references
- D. P. Bartel, Cell, 2004, 116, 281–297 CrossRef CAS PubMed.
- J. Brennecke, D. R. Hipfner, A. Stark, R. B. Russell and S. M. Cohen, Cell, 2003, 113, 25–36 CrossRef CAS PubMed.
- V. Ambros, Cell, 2001, 107, 823–826 CrossRef CAS PubMed.
- F. Xin, M. Li, C. Balch, M. Thomson, M. Fan, Y. Liu, S. M. Hammond, S. Kim and K. P. Nephew, Bioinformatics, 2009, 25, 430–434 CrossRef CAS PubMed.
- G. A. Calin and C. M. Croce, Nat. Rev. Cancer, 2006, 6, 857–866 CrossRef CAS PubMed.
- C. C. Pritchard, H. H. Cheng and M. Tewari, Nat. Rev. Genet., 2012, 13, 358–369 CrossRef CAS PubMed.
- R. Liu, Q. Wang, Q. Li, X. Yang, K. Wang and W. Nie, Biosens. Bioelectron., 2017, 87, 433–438 CrossRef CAS PubMed.
- X. He, T. Zeng, Z. Li, G. Wang and N. Ma, Angew. Chem., Int. Ed., 2016, 55, 3073–3076 CrossRef CAS PubMed.
- X. Huang, Y. Zhang, J. Chen, L. Zhang, Y. Xu, W. Yin, Y. Shi, S.-Y. Liu, X. Zou and Z. Dai, Anal. Chem., 2022, 94, 12221–12230 CrossRef CAS PubMed.
- T. Li, M. Sun, Q. Zhou, P. Liang, T. Huang, M. Guo, B. Xie, C. Li, M. Li, W.-J. Duan, J.-X. Chen, Z. Dai and J. Chen, Anal. Chem., 2023, 95, 15025–15032 CrossRef CAS PubMed.
- J. Wang, Y. Liu, T. Zhao, J. Shi, J. Chen, D. Li, Y. Cui, S. Xu and X. Luo, Anal. Chem., 2023, 95, 9388–9395 CrossRef CAS PubMed.
- Y. Gao, S. Zhang, C. Wu, Q. Li, Z. Shen, Y. Lu and Z.-S. Wu, ACS Nano, 2021, 15, 19211–19224 CrossRef CAS PubMed.
- S. Sun, H. Xu, Y. Yang, L. Wang, L. Ye, H. Jiang, C. Xue, Z. Shen and Z.-S. Wu, Chem. Eng. J., 2022, 428, 131150 CrossRef CAS.
- R. Liu, S. Zhang, T.-T. Zheng, Y.-R. Chen, J.-T. Wu and Z.-S. Wu, ACS Nano, 2020, 14, 9572–9584 CrossRef CAS PubMed.
- Y. Feng, Q. Liu, X. Zhao, M. Chen, X. Sun, H. Li and X. Chen, Anal. Chem., 2022, 94, 2934–2941 CrossRef CAS PubMed.
- R. Wei, K. Wang, X. Liu, M. Shi, W. Pan, N. Li and B. Tang, Biosens. Bioelectron., 2023, 115584 CrossRef CAS PubMed.
- Q. Hu, H. Li, L. Wang, H. Gu and C. Fan, Chem. Rev., 2018, 119, 6459–6506 CrossRef PubMed.
- C. Ouyang, S. Zhang, C. Xue, X. Yu, H. Xu, Z. Wang, Y. Lu and Z.-S. Wu, J. Am. Chem. Soc., 2020, 142, 1265–1277 CrossRef CAS PubMed.
- S. Sun, H. Yang, Z. Wu, S. Zhang, J. Xu and P. Shi, Chem. Commun., 2024, 60, 3098–3117 RSC.
- Y. Shao, J. Zhao, J. Yuan, Y. Zhao and L. Li, Angew. Chem., Int. Ed., 2021, 133, 9005–9013 CrossRef.
- Z. Zhou, D. Fan, J. Wang, Y. S. Sohn, R. Nechushtai and I. Willner, Small, 2021, 17, 2007355 CrossRef CAS PubMed.
- C. Li, Y. Wang, P.-F. Li and Q. Fu, Acta Biomater., 2023, 160, 1–13 CrossRef CAS PubMed.
- F. Li, J. Li, B. Dong, F. Wang, C. Fan and X. Zuo, Chem. Soc. Rev., 2021, 50, 5650–5667 RSC.
- Y. Zhang, D. Luo, Y. Zhang, Q.-H. Zhang, Q.-Y. Ji, S.-K. Zhou, S. Huang, L.-L. Li, F. Lu and W.-F. Yao, Chem. Eng. J., 2023, 454, 140489 CrossRef CAS.
- F. Li, Y. Liu, Y. Dong, Y. Chu, N. Song and D. Yang, J. Am. Chem. Soc., 2022, 144, 4667–4677 CrossRef CAS PubMed.
- Y. Dong, F. Li, Z. Lv, S. Li, M. Yuan, N. Song, J. Liu and D. Yang, Angew. Chem., Int. Ed., 2022, 134, e202207770 CrossRef.
- Y. Guo, S. Li, Z. Tong, J. Tang, R. Zhang, Z. Lv, N. Song, D. Yang and C. Yao, J. Am. Chem. Soc., 2023, 145, 23859–23873 CrossRef CAS PubMed.
- C. Li, S. Luo, J. Wang, Z. Shen and Z.-S. Wu, Nanoscale, 2021, 13, 7034–7051 RSC.
- Y. Gao, Q. Li, J. Zhang, C. Wu, Z. Shen, C. Xue, H.-T. Chang and Z.-S. Wu, ACS Appl. Mater. Interfaces, 2019, 12, 3341–3353 CrossRef PubMed.
- Q. Wei, J. Huang, J. Li, J. Wang, X. Yang, J. Liu and K. Wang, Chem. Sci., 2018, 9, 7802–7808 RSC.
- C. Xue, S. Zhang, X. Yu, S. Hu, Y. Lu and Z. S. Wu, Angew. Chem., Int. Ed., 2020, 132, 17693–17700 CrossRef.
- K. Ren, Y. Xu, Y. Liu, M. Yang and H. Ju, ACS Nano, 2018, 12, 263–271 CrossRef CAS PubMed.
- Z. Wu, G.-Q. Liu, X.-L. Yang and J.-H. Jiang, J. Am. Chem. Soc., 2015, 137, 6829–6836 CrossRef CAS PubMed.
- A. P. Karunanayake Mudiyanselage, Q. Yu, M. A. Leon-Duque, B. Zhao, R. Wu and M. You, J. Am. Chem. Soc., 2018, 140, 8739–8745 CrossRef CAS PubMed.
- S. Khan, B. Burciu, C. D. Filipe, Y. Li, K. Dellinger and T. F. Didar, ACS Nano, 2021, 15, 13943–13969 CrossRef CAS PubMed.
- Y. Wan, G. Li, L. Zou, H. Wang, Q. Wang, K. Tan, X. Liu and F. Wang, Anal. Chem., 2021, 93, 11052–11059 CrossRef CAS PubMed.
- R. R. Breaker and G. F. Joyce, Chem. Biol., 1994, 1, 223–229 CrossRef CAS PubMed.
- L. Li, Y. Ren, X. Wen, Q. Guo, J. Wang, S. Li, M. Yang and K. Wang, Anal. Chem., 2021, 93, 13919–13927 CrossRef CAS PubMed.
- H. Chen, X. Chen, Y. Chen, C. Zhang, Z. Sun, J. Mo, Y. Wang, J. Yang, D. Zou and Y. Luo, Analyst, 2023, 148, 1682–1693 RSC.
- Y. Yang, Y. He, Z. Deng, J. Li, X. Li, J. Huang and S. Zhong, ACS Appl. Bio Mater., 2020, 3, 6310–6318 CrossRef CAS PubMed.
- W. Zhou, Q. Chen, P.-J. J. Huang, J. Ding and J. Liu, Anal. Chem., 2015, 87, 4001–4007 CrossRef CAS PubMed.
- H. Guan, S. Yang, C. Zheng, L. Zhu, S. Sun, M. Guo, X. Hu, X. Huang, L. Wang and Z. Shen, Talanta, 2021, 233, 122543 CrossRef CAS PubMed.
- H. Wang, Y. Chen, H. Wang, X. Liu, X. Zhou and F. Wang, Angew. Chem., Int. Ed., 2019, 58, 7380–7384 CrossRef CAS PubMed.
- Y. Yang, J. Huang, X. Yang, X. He, K. Quan, N. Xie, M. Ou and K. Wang, Anal. Chem., 2017, 89, 5850–5856 CrossRef CAS PubMed.
- H. Fan, Z. Zhao, G. Yan, X. Zhang, C. Yang, H. Meng, Z. Chen, H. Liu and W. Tan, Angew. Chem., Int. Ed., 2015, 54, 4801–4805 CrossRef CAS PubMed.
- D. L. Coy, M. Wagenbach and J. Howard, J. Biol. Chem., 1999, 274, 3667–3671 CrossRef CAS PubMed.
- J. Tao, H. Zhang, M. Weinfeld and X. C. Le, Anal. Chem., 2023, 95, 14990–14997 CrossRef CAS PubMed.
- S. Sun, Y. Yang, Z. Gao, H. Jiang, L. Ye, Y. Lai, Z. Shen and Z.-S. Wu, ACS Appl. Mater. Interfaces, 2022, 14, 45201–45216 CrossRef CAS PubMed.
- L. Song, Y. Zhuge, X. Zuo, M. Li and F. Wang, Adv. Sci., 2022, 9, e2200327 CrossRef PubMed.
- J. Valero and M. Skugor, ChemPhysChem, 2020, 21, 1971–1988 CrossRef CAS PubMed.
- J. Chen, Z. Luo, C. Sun, Z. Huang, C. Zhou, S. Yin, Y. Duan and Y. Li, TrAC, Trends Anal. Chem., 2019, 120, 115626 CrossRef CAS.
- Y. Wu, H. M. Meng, J. Chen, K. Jiang, R. Yang, Y. Li, K. Zhang, L. Qu, X. B. Zhang and Z. Li, Chem. Commun., 2020, 56, 470–473 RSC.
- W. Li, X. Yang, L. He, K. Wang, Q. Wang, J. Huang, J. Liu, B. Wu and C. Xu, ACS Appl. Mater. Interfaces, 2016, 8, 25733–25740 CrossRef CAS PubMed.
- M. Liu, W. Ma, Q. Li, D. Zhao, X. Shao, Q. Huang, L. Hao and Y. Lin, Cell Prolif., 2019, 52, e12511 CrossRef PubMed.
- S. Ali, A. Ahmad, S. Banerjee, S. Padhye, K. Dominiak, J. M. Schaffert, Z. Wang, P. A. Philip and F. H. Sarkar, Cancer Res., 2010, 70, 3606–3617 CrossRef CAS PubMed.
- K. Gumireddy, D. D. Young, X. Xiong, J. B. Hogenesch, Q. Huang and A. Deiters, Angew. Chem., Int. Ed., 2008, 120, 7592–7594 CrossRef.
- A. Minta and R. Y. Tsien, J. Biol. Chem., 1989, 264, 19449–19457 CrossRef CAS PubMed.
- S. F. Torabi, P. W. Wu, C. E. McGhee, L. Chen, K. Hwang, N. Zheng, J. J. Cheng and Y. Lu, Proc. Natl. Acad. Sci. U. S. A., 2015, 112, 5903–5908 CrossRef CAS PubMed.
- C. Fan, L. Xie, F. Zhao, J. Wang, X. Lin and X. Chen, Anal. Chim. Acta, 2024, 1288, 342172 CrossRef CAS PubMed.
- K. Nie, Y. Jiang, N. Wang, Y. Wang, D. Li, L. Zhan, C. Huang and C. Li, Chemosensors, 2022, 10, 276 CrossRef CAS.
- N. Wang, Y. Jiang, K. Nie, D. Li, H. Liu, J. Wang, C. Huang and C. Li, Chin. Chem. Lett., 2023, 34, 107906 CrossRef CAS.
- C. Li, C. Xue, J. Wang, M. Luo, Z. Shen and Z.-S. Wu, Anal. Chem., 2019, 91, 11529–11536 CrossRef CAS PubMed.
- H. Xu, Y. Zheng, J. Xie, W. Duan, L. Yu, R. Lin, C.-C. Li and L. Jia, Biosens. Bioelectron., 2024, 248, 115973 CrossRef CAS PubMed.
- K. Zhang, H. Fang, Z. Wang, Z. Li, J.-S. A. Taylor and K. L. Wooley, Biomaterials, 2010, 31, 1805–1813 CrossRef CAS PubMed.
- J. I. Cutler, K. Zhang, D. Zheng, E. Auyeung, A. E. Prigodich and C. A. Mirkin, J. Am. Chem. Soc., 2011, 133, 9254–9257 CrossRef CAS PubMed.
|
This journal is © The Royal Society of Chemistry 2024 |
Click here to see how this site uses Cookies. View our privacy policy here.