DOI:
10.1039/D4TB01847H
(Paper)
J. Mater. Chem. B, 2024,
12, 11359-11367
The cyano positional isomerism strategy for constructing mitochondria-targeted AIEgens with type I reactive oxygen species generation capability†
Received
15th August 2024
, Accepted 4th October 2024
First published on 7th October 2024
Abstract
In this work, a series of cationic luminogens (designated as PSMP isomers) were developed based on the cyano positional isomerism strategy. The isomerism of the cyano substituent on the molecular skeleton can finely regulate the optical behaviour, the type of photoinduced reactive oxygen species (ROS), and mitochondria-targeted capability of isomers. Interestingly, PSMP-4, with the cyano group installed at an appropriate location, exhibits a special aggregation-induced emission effect and potent O2˙− generation efficacy through the type I photochemistry pathway. Notably, PSMP-4 can accumulate in mitochondria with high specificity. Taking advantage of its excellent photostability, PSMP-4 realizes in situ mitochondria imaging in a washing-free manner and sensitive response to the change of mitochondrial membrane potential. The integration of comprehensive photophysical properties and mitochondrial specificity enable PSMP-4 to successfully trigger the death of cancer cells through an efficient type I photodynamic therapy process both in vitro and in multicellular tumor spheroid models.
Introduction
Mitochondria, double-layered membranous organelles that generate energy in the form of adenosine triphosphate in most eukaryotic cells, orchestrate pivotal biological functions far beyond bioenergetics including signal transduction, lipids metabolism, oxidative stress, and cellular proliferation and differentiation.1–6 Mitochondrial dysfunctions have been proven to involve in diverse pathologies, such as metabolic syndrome, cardio-cerebrovascular disease, and neurodegeneration.7–9 Especially, mitochondrial homeostasis disequilibrium has been shown to be closely associated with tumorigenesis, malignant invasion and multiple drug resistance.10,11 The indispensable roles of mitochondria make them attractive therapeutic targets in intervening disease progression.12,13 Unfortunately, the phenotypic threshold of mitochondrial malfunctions is elusive, compromising our understanding about the contributions of mitochondria to disease regulation.14 Therefore, there is an incentive to develop multifaceted molecular tools with precise mitochondria-targeted capacity, aiming to achieve real-time tracking of mitochondria-participating intricate biological networks as well as in situ regulation of mitochondria-mediated cell death.
The emergence of mitochondria-targeted luminogens affords a distinctive bioimaging regimen in monitoring morphological integrities and functional variations in mitochondria.15,16 In addition, the mitochondria-targeted luminogens with photosensitization activities can evoke apoptotic cell death under the stimulation of reactive oxygen species (ROS) produced by light, providing noninvasive and spatiotemporal controllable tactics in managing disease progression through photodynamic therapy (PDT). Flourishing mitochondria-targeted phototheranostic luminogens have been designed and applied for cancer cell eradication.17,18 Nevertheless, while tremendous progress has been made in cancer-specific phototheranostics, the scope of biological applications of conventional luminogens poses challenges due to their inherent defects. For example, the “always on” fluorescence of luminogens is susceptible to interference from nonspecific fluorescence signals of other organelles, reducing the signal-to-noise ratio (SNR) during mitochondria-targeted imaging.19 Meanwhile, the undifferentiated fluorescence behaviours before and after mitochondrial staining and the aberrant quenching of photosensitization when luminogens aggregate at lesion sites inevitably lead to off-target toxicity and attenuated ROS generation capacity.20,21 Besides, the reliance on O2 participation contradicted with the hypoxic microenvironment of hostile tumors (pO2 < 5 mmHg), which is apt to impede the exertion of PDT efficacy.22,23 Constructing potent luminogens with excellent mitochondria-targeted capability to overcome these shortcomings has remained a formidable conundrum to date.
Aggregation-induced emission luminogens (AIEgens) provide an ideal tool to overcome the aforementioned drawbacks.24,25 On the one hand, the “light-up” of mitochondria by AIEgen accumulation perfectly circumvents the non-specific fluorescence interference from other organelles.26 On the other hand, the photosensitization tends to be activated when AIEgens aggregate in targeted organelles, avoiding negative cytotoxicity impacts caused by off target effects.27–29 Additionally, by adjusting the electronic properties, some AIEgens have been proved to possess potent type I ROS (such as O2˙− and ˙OH) generation capabilities through the electron transfer pathway, exhibiting significant advantages in conquering hypoxic tumors.30–35 The most popular strategy for fabricating mitochondria-targeted AIEgens is the introduction of positively charged groups into AIE-active molecular backbones.16,36 The delocalized lipocationic luminogens selectively accumulate in mitochondria through electrostatic interactions owing to the lower potential of the inner mitochondrial membrane.37 Additionally, mitochondrial selectivity can be regulated by fine tuning the hydrophobicity of AIEgens using side chain engineering.38,39 Recent study has revealed that mitochondria specificity can be facilely achieved through the employment of appealing isomerism approaches.40–42 Hitherto, plenty of mitochondria-targeted AIEgens based on privileged molecular skeletons, such as tetraphenylethylene,43 triphenylamine,44 coumarin,45 and cyanine,46 have been developed. Nevertheless, the complexity of synthesis and the elusive nature of the structure–activity relationships still make it a daunting challenge to construct mitochondria specific AIEgens and endow them with controllable ROS generation capabilities.
Aiming at these critical issues, in this report for the first time, we developed a series of cationic luminogens (designated as PSMP isomers) based on the positional isomerism strategy, which exhibit regulated photophysical properties and mitochondrial selectivity. The translocation of the cyano substituent endows the PSMP-4 isomer with typical AIE characteristics. Meanwhile, reversible mechanochromic luminescence properties are observed for indicated PSMP isomers. The cyano positional isomerism influences the type of ROS generated by four isomers, enabling PSMP-4 to produce O2˙− through the low oxygen-dependent type I photochemistry pathway. Meanwhile, PSMP-4 also exhibits the best mitochondrial staining performance. Further experiments have shown that PSMP-4 permits in situ mitochondrial imaging without washing and sensitive response to mitochondrial membrane potential (MMP) change due to its excellent photostability. The mitochondrial specificity and efficient type I ROS generation capability allow PSMP-4 to successfully trigger cancer cell death both in vitro and in multicellular tumor spheroid models (MCTSs) (Scheme 1).
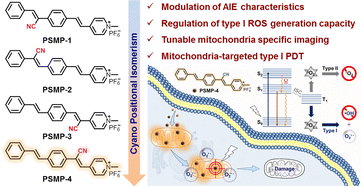 |
| Scheme 1 Molecular structures of PSMP isomers and schematic diagram of PSMP-4 for mitochondria-targeted imaging and type I PDT. | |
Results and discussion
Molecular design and synthesis
The PSMP isomers were designed by fixing the core molecular skeleton of pyridinium while varying the position of the cyano substituent. The cationic pyridinium part serves as both the electron-withdrawing group and mitochondria-targeting moiety. The cyano group acts as an electronic effect regulator and a molecular rotor, modulating photophysical properties as well as the type of ROS. The PSMP isomers were synthesized as displayed in Schemes S1–S4 (ESI†). Taking PSMP-4 as an example, the Knoevenagel condensation between 4-bromophenylacetonitrile and 4-pyridinecarboxaldehyde yielded an intermediate of 4-1, which reacted with 4-vinylpyridine through palladium-catalyzed Heck coupling to give product 4-2. The methyl group was further installed, followed by anion exchange by KPF6, giving the desired isomer PSMP-4. Similar synthetic procedures were adopted to obtain other three isomers. The structures of all PSMP isomers were verified by 1H and 13C NMR, and high resolution mass spectrometry (Fig. S26–S53, ESI†).
Photophysical properties
The photophysical properties of developed luminogens were evaluated initially. As displayed in Fig. 1a, the maximum absorption peaks of PSMP isomers in DMSO were located at 381 (PSMP-1), 388 (PSMP-2), 409 (PSMP-3), and 418 nm (PSMP-4), respectively. The fluorescence properties of PSMP isomers in mixed solvents (DMSO/water) with indicated water (poor solvent) volumetric fractions (fw) were next measured. As shown in Fig. 1b and c, weak fluorescence of PSMP-4 centred at 603 nm was observed when it was dissolved in DMSO with a fluorescence quantum yield (Φf) of 2.8% and an average fluorescence lifetime (τavg) of 3.01 ns. Negligible fluorescence change was detected when fw increased from 0 to 80%. Continuous increase in fw resulted in fluorescence boost with the hypochromatic shift of emission from 603 to 547 nm. The emission intensity reached the maximum at fw of 99%, giving 65-fold of emission enhancement with a Φf of 9.9% and τavg of 13.47 ns. The PSMP-4 formed nano-aggregates at fw of 99%, giving a particle size of 589 nm as revealed by dynamic light scattering analysis (Fig. 1d). The level-off tail appeared in the absorption spectra of PSMP-4 at fw of 99% (Fig. S1, ESI†), suggesting the formation of nanoaggregates. Clearly, PSMP-4 is a typical AIEgen. As shown in Fig. S2–S4 (ESI†), moderate emission in DMSO (Φf 3.1–26%) was obtained for other three isomers, whereas it barely enhanced when the fw was increased to 99% (Φf 1.2–21%), implying the lack of AIE feature. In addition, the emission of PSMP isomers in the solution state red shifted from 510 to 603 nm in the order from PSMP-1 to PSMP-4 (Table S1, ESI†), which is consistent with their red shifted absorption peak. The solid emission of PSMP isomers varied from 528 to 634 nm (Fig. S5, ESI†). It should be noted that AIE-active PSMP-4 possesses large Stokes shifts (>180 nm), which is beneficial to achieve higher SNR during biological imaging.47 Meanwhile, the emission intensity of PSMP-4 in PBS with 10% FBS can remain for 48 h (Fig. S6, ESI†), suggesting its good stability.
 |
| Fig. 1 (a) Absorption spectra of PSMP isomers (50 μM) in DMSO solution. (b) Fluorescence spectra of PSMP-4 (50 μM) in DMSO/water mixtures with different fw. (c) Plots of I/I0versus different fw for PSMP-4. I0 and I represent the emission intensity before and after addition of water. (d) The particle size distribution of PSMP-4 (50 μM) in DMSO/water mixtures with fw of 99%. (e) The fluorescence lifetime of PSMP-4 (50 μM) in DMSO/water mixtures with fw of 0% and 99%. Plots of I/I0versus irradiation time in the presence of PSMP isomers (50 μM) and (f) DCFH-DA, (g) DHR123, and (h) HPF. I0 and I represent the emission intensities of DCF, DHR123, and HPF before and after white light irradiation. (i) Plots of A/A0versus irradiation time in the presence of PSMP isomers (50 μM) and ABDA. A0 and A represent the absorbance of ABDA before and after white light irradiation. | |
The potent ROS production capability is an indispensable requirement in the process of PDT. Therefore, we assessed the ROS generation efficacy of PSMP isomers under light illumination. A white LED light (400–700 nm, 25 mW cm−2) was set as the irradiation source as the absorption spectra of PSMP isomers were located within the visible light region. The broad-spectrum ROS probe termed 2′,7′-dichlorodihydrofluorescein diacetate (DCFH-DA) was employed and the fluorescence intensity of DCF (oxidized form of DCFH-DA) at 530 nm was collected under indicated white light irradiation to evaluate the ROS generation efficacy of PSMP isomers at specific time intervals. As shown in Fig. 1f and Fig. S7 (ESI†), distinct fluorescence behaviours were obtained when irradiating DCFH-DA and PSMP isomers with white light. The fluorescence intensities increased rapidly with the elapse of irradiation time and eventually gave 233-(PSMP-2), 182-(PSMP-3), and 161-fold (PSMP-4) emission enhancement within 120 s compared with the group with DCFH-DA only, demonstrating the successful generation of ROS. However, only 22-fold fluorescence enhancement was obtained after irradiating the mixture containing PSMP-1 and DCFH-DA, indicating the lack of ROS generation of PSMP-1. The intercepted intersystem crossing (ISC) from S1 to T1 could be the main reason that forbids PSMP-1 from generating ROS.48 Afterwards, the commercial probes including dihydrorhodamine 123 (DHR123), hydroxyphenyl fluorescein (HPF), and 9,10-anthracenediyl-bis(methylene) dimalonic acid (ABDA) were introduced to explore the specific type of ROS, where ABDA was used as the 1O2 scavenger and DHR123 and HPF served as O2˙− and ˙OH indicators, respectively.49 As described in Fig. 1g and Fig. S8 (ESI†), the fluorescence intensity of DHR123 was boosted after irradiating with continuous white light for 300 s in the presence of PSMP isomers, giving 35-(PSMP-1), 205-(PSMP-2), 130-(PSMP-3), and 105-fold (PSMP-4) emission enhancement. Replacing DHR123 by HPF, 33-fold fluorescence enhancement was observed for PSMP-3 after irradiating with white light for 600 s. However, negligible fluorescence response was detected for other three isomers (Fig. 1h and Fig. S9, ESI†). Notably, after changing the ROS indicator to ABDA and exposing to white light illumination, the absorption peak of ABDA at 378 nm decreased in the presence of PSMP-2 or PSMP-3. Contrarily, ignorable absorption changes were observed for PSMP-1 and PSMP-4 under identical conditions (Fig. 1i and Fig. S10, ESI†). Clearly, subtle migration of the cyano substituent finely tunes the electronic push–pull strength and the ISC efficacy of PSMP isomers. It is interesting to note that PSMP-4 generates O2˙− through the type I photochemistry pathway, which is beneficial for hypoxic tumour PDT.
Theoretical calculations
The influence of cyano positional isomerism on the electronic properties of PSMP isomers was further validated by theoretical calculations based on the DFT method. As shown in Fig. 2, the highest occupied molecular orbital (HOMO) of PSMP isomers was mainly delocalized over the central phenyl ring and adjacent styryl group, whereas the lowest unoccupied molecular orbital (LUMO) was primarily distributed on the central phenyl ring and adjacent vinylpyridinium part. The partially separated HOMO and LUMO indicated the existence of intramolecular charge transfer transitions. The energy gaps between the HOMO and LUMO were calculated to be 4.308 (PSMP-1), 4.303 (PSMP-2), 4.019 (PSMP-3), and 3.678 eV (PSMP-4), respectively. The gradually decreased energy levels is well consistent with the red shifted absorption profiles.
 |
| Fig. 2 Frontier molecular orbital distributions of the HOMO and LUMO of PSMP isomers and their energy gaps between the HOMO and LUMO calculated using the DFT method at the CAM-B3LYP/6-31G(d,p) basis set. | |
Mechanochromic luminescence properties
Mechanochromic luminescence (ML) properties are prominent features of smart fluorescence materials, which changes the fluorescence behaviours in response to external stimulation including grinding and stretching processes. The reversible fluorescence characteristics of ML materials make them applicable to the aspects of information encryption, stress sensors, information storage and so on.50 The distinctive emission profiles caused by subtle cyano group translocation inspired us to investigate the ML properties of the developed isomers. As shown in Fig. 3, the as-prepared sample of PSMP-2 and PSMP-4 displayed emission peaks at 526 and 573 nm. After gentle grinding, however, the emission peaks of the above isomers red shifted to 551 and 582 nm, respectively. The collapse of the crystalline state after grinding probably enables the above isomers to adopt a more planar conformation, resulting in a red-shifted emission.51 A salient feature of ML materials is that when the ground sample is fumed with an organic solvent, the fluorescence signal can be restored to its initial state. To our delight, this characteristic was applicable to the ground isomers of PSMP-2 and PSMP-4 when fumed with acetone vapour, while the emission peak nearly restored to its initial position. Notably, the reversible emission changes could be repeated at least five times without sacrificing the fluorescence intensity. As shown in the XRD pattern (Fig. S11, ESI†), sharp diffraction peaks were observed for the as-prepared sample of PSMP-4, which almost disappeared after grinding. The above results revealed that the ML properties of PSMP-4 originated from the morphological change. However, the isomers PSMP-1 and PSMP-3 possessed ignorable ML properties (Fig. S12, ESI†), which could be attributed to the less changes of molecular conformation under external stimulation.
 |
| Fig. 3 The photographs of ground and fumed samples of (a) PSMP-2 and (d) PSMP-4 under irradiation of a 365 nm UV lamp. Fluorescence spectra of as-prepared, ground, and fumed samples of (b) PSMP-2 and (e) PSMP-4. Reversible switching of fluorescence response of (c) PSMP-2 and (f) PSMP-4 through repeated grinding and fuming cycles. | |
Cell imaging performance
According to previous seminal reports, positively charged luminogens tend to reside in mitochondria or nucleus owing to the electrostatic interaction.52 Therefore, the subcellular location of cationic PSMP isomers in living cells was studied. The cell images after staining by PSMP isomers were collected using a confocal laser scanning microscope (CLSM). Different staining performance was obtained after incubating HeLa cells with PSMP isomers. As shown in Fig. S13 (ESI†), the HeLa cells were successfully labelled by PSMP-1, PSMP-2 and PSMP-4, giving intense fluorescence signals from the cytoplasm. However, ignorable fluorescence was obtained for cells treated with PSMP-3. Further co-staining experiments using MitoTracker Deep Red (MTDR, mitochondria probe) revealed that the fluorescence signals of PSMP-1, PSMP-2, and PSMP-4 overlapped with the red fluorescence of MTDR, giving Pearson's correlation coefficient (PCC) values of 0.84, 0.85, and 0.94, respectively (Fig. 4a and Fig. S14, ESI†). Furthermore, poor co-localization profiles were observed when cells were co-stained with PSMP-4 and other organelle probes including ER-Tracker Red (ETR, endoplasmic reticulum probe), HCS LipidTOXTM (HCSLT, lipid droplet probe), Hoechst 33342 (nucleus probe) and Lyso Tracker Deep Red (LTDR, lysosome probe), giving PCC values less than 0.43 (Fig. 4a and Fig. S15a, ESI†). The above results demonstrated that PSMP-4 exhibits the best mitochondria specificity in living cells.
 |
| Fig. 4 (a) Colocalization CLSM images of HeLa cells stained by PSMP-4 (10 μM) and organelle probes including MTDR, ETR, HCSLT, and Hoechst 33342. (b) Time-dependent CLSM images of HeLa cells after being stained by PSMP-4 (10 μM) in a washing-free manner. (c) The fluorescence signal loss versus the time of laser scans for PSMP-4 and MTDR. Inset photographs represent the CLSM images of PSMP-4 and MTDR pre-stained HeLa cells at 0 and 180 s under continuous laser scan. (d) The plot of the fluorescence intensity of PSMP-4 versus the concentrations of CCCP. Inset photographs represent the CLSM images of HeLa cells treated with PSMP-4 and different concentration of CCCP. The data are presented as the mean ± standard deviation (n = 3). Scale bar: 10 μm. | |
By virtue of the AIE feature, the washing-free staining ability of PSMP-4 was assessed. As shown in Fig. 4b, with the elapse of the incubation time to 60 min, the fluorescence signals of PSMP-4 gradually increased, giving the images with an excellent SNR. After 60 min, PSMP-4 still remained in mitochondria with the PCC value as high as 0.94 (Fig. S15b, ESI†). As shown in Fig. 4c, after scanning the stained cells with a continuous laser, a faint fluorescence signal loss of PSMP-4 was obtained, which is comparable to MTDR. For PSMP-1 and PSMP-2, however, rapid loss of fluorescence was observed (Fig. S16, ESI†). The MMP response of PSMP-4 was further evaluated. As displayed in Fig. 4d and Fig. S17 (ESI†), the fluorescence intensity gradually decreased when the cells were pre-treated with increased concentrations of carbonyl cyanide m-chlorophenylhydrazone (CCCP) which can open the mitochondrial permeability transition pore and dissipate the MMP. CHO cells were further selected as normal cells to assess the selectivity of PSMP-4 for cancer cells. Slight fluorescence signals were observed in CHO cells after staining with PSMP-4 (Fig. S18, ESI†), suggesting that PSMP-4 possesses potential cancer cell distinguishing capability.
In vitro investigation of PDT properties
On account of the potent type I ROS generation and mitochondria targeting capability, the PDT efficacy of PSMP-4 for cancer cells was thoroughly investigated. Firstly, the intracellular ROS generation of PSMP-4 was measured using DCFH-DA. As shown in Fig. 5a and Fig. S19 (ESI†), blurry green fluorescence representing DCF signals appeared after irradiating PSMP-4 pre-stained HeLa cells with white light (25 mW cm−2) for 60 s. The whole cells were filled with green fluorescence after extending the irradiation time to 180 s, indicating that the generation of ROS in living cells was successfully activated. However, ignorable fluorescence signals were observed in the absence of PSMP-4 or light irradiation (Fig. S20, ESI†), revealing excellent spatiotemporal controlled ROS generation of PSMP-4. Further quantitative flow cytometry analysis gave 99.1% DCF-positive cell populations after white light illumination for 180 s (Fig. 5b), which was well consistent with the CLSM imaging results. In addition, the red fluorescence of MitoSOX (indicator for the generation of mitochondrial ROS) appeared when the PSMP-4 stained cells were irradiated with white light (Fig. 5c and d), suggesting that the generated ROS mainly come from mitochondria. Afterwards, commercial probes including DHE, HPF, and SOSG were employed to track the generation of O2˙−, ˙OH, and 1O2 by photosensitized PSMP-4 (Fig. 5e and f). Intense red fluorescence attributed to DHE was observed after illuminating PSMP-4, indicating the existence of type I ROS of O2˙−. When cells were simultaneously treated with PSMP-4 and HPF (or SOSG) under identical light irradiation conditions, no fluorescence appeared in treated cells, suggesting the absence of intracellular ˙OH and 1O2. Similar results were obtained under hypoxic conditions (Fig. 5g and h), which was consistent with the extracellular results. However, negligible fluorescence was obtained in the shield of white light illumination (Fig. S21, ESI†).
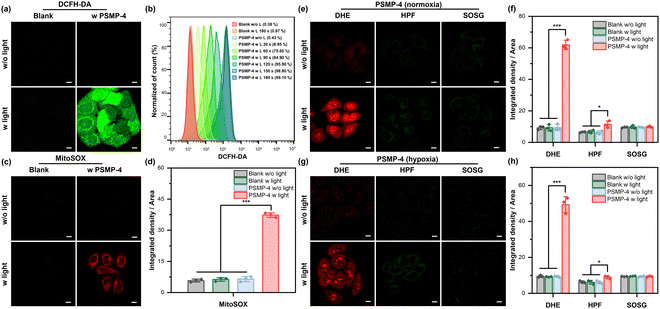 |
| Fig. 5 (a) CLSM images of DCFH-DA pre-stained HeLa cells after different treatments. (b) Flow cytometry analysis of fluorescence intensities in DCFH-DA pre-stained HeLa cells. (c) CLSM images of MitoSOX after treating PSMP-4 (10 μM) pre-stained HeLa cells under different conditions. The CLSM images of DHE, HPF, and SOSG under normoxia and hypoxia. The corresponding fluorescence intensities extracted from (d) (c), (f) (e) and (h) (g). The data are presented as the mean ± standard deviation (n = 3), *p < 0.05, ***p < 0.001. Scale bar: 10 μm. | |
As shown in Fig. 6a, b, and Fig. S22 (ESI†), when cells were treated with PSMP-4 and white light, green fluorescence of rhodamine 123 disappeared and green fluorescence of JC-1 appeared, indicating the occurrence of mitochondrial malfunction by the PDT effect of PSMP-4. The cell death triggered by the PSMP-4 mediated PDT process was also verified using calcein acetoxymethyl ester (calcein AM) and propidium iodide (PI) as live/dead cell indicators. As displayed in Fig. 6c, the calcein AM is hydrolyzed in cells treated with PSMP-4 in the dark, producing intense green fluorescence. When white light irradiation is introduced, the red fluorescence signal of PI emerged at the expense of the decrease of the green fluorescence of calcein AM, which intuitively demonstrated the potent tumoricidal effect by PSMP-4-mediated PDT. Mitochondria-targeted PDT prefers to induce the apoptosis of cells. Therefore, the annexin V-FITC/PI detection kit was selected to monitor cellular apoptosis. As shown in Fig. 6d, and Fig. S23 (ESI†), upon prolonging the cellular incubation time after treating the cells with PSMP-4 and light irradiation, the green fluorescence of annexin V-FITC gradually enhanced, accompanied by the emergence of red fluorescence of the PI in the nucleus, demonstrating the occurrence of apoptosis. The flow cytometry analysis exhibited high death cell population (99.8%) when the PSMP-4 pre-stained cells were irradiated by white light and incubated for an additional 2 h (Fig. 6e). Finally, the anticancer potency of PSMP-4 mediated PDT was quantitatively evaluated using the MTT method. As shown in Fig. 6f, negligible cytotoxicity of PSMP-4 was observed even when the concentration of PSMP-4 reached 100 μM, suggesting its good biocompatibility. By introducing white light irradiation, the photo-induced cytotoxicity increased while the cell viability dropped to 10% in the presence of 100 μM of PSMP-4. Similar photo-induced cytotoxicity of PSMP-4 was obtained under hypoxic conditions (Fig. S24a, ESI†). However, reduced photo-induced cytotoxicity of PSMP-4 was detected in CHO cells (Fig. S24b, ESI†), which was consistent with the imaging performance. The above results imply that PSMP-4 is a promising type I photosensitizer against the proliferation of hypoxic tumors.
 |
| Fig. 6 (a) Rhodamine 123 staining assay for the HeLa cells under different conditions. (b) The corresponding fluorescence intensities extracted from a. (c) The live/dead cell staining assay for PSMP-4 (10 μM) treated HeLa cells with (or without) white light (25 mW cm−2, 30 min). (d) Time-dependent CLSM images of Annexin V-FITC/PI staining of HeLa cells after treating with PSMP-4 (10 μM) and white light (25 mW cm−2, 30 min). (e) Flow cytometry profiles of cell death triggered by PSMP-4 (10 μM) with (or without) white light (25 mW cm−2, 30 min). (f) Cell viability assays of HeLa cells subjected to different concentrations of PSMP-4 with or without white light (25 mW cm−2, 30 min). (g) Z-stack images after incubation of PSMP-4 (50 μM) in HeLa cell MCTS. Z-Axis images scanning from top to bottom of an intact spheroid every 20 μm. (h) 3D Z-stack of an intact spheroid of g. (i) The live/dead cell staining assays for PSMP-4 (50 μM) treated HeLa cell MCTS with (or without) white light (25 mW cm−2, 30 min every day). The data are presented as the mean ± standard deviation (n = 3 for b, 5 for f), ***p < 0.001. Scale bar for a, c and d: 10 μm. Scale bar for g and i: 200 μm. | |
MCTSs are used to mimic the hypoxic environment of tumors.53 To evaluate the type I PDT efficacy of PSMP-4, the MCTSs of HeLa cells were cultured using the hanging drop method. As shown in Fig. 6g, h and Fig. S25 (ESI†), the whole spheroid exhibited bright yellow fluorescence after staining with PSMP-4, suggesting that PSMP-4 can infiltrate the MCTSs. Furthermore, when the PSMP-4 stained MCTS was irradiated with white light for 2 days (30 min every day), the green fluorescence of calcein AM disappeared whereas the red fluorescence of PI emerged from the entire MCTS (Fig. 6i). The above results proved the potential therapeutic applications of PSMP-4-mediated type I PDT to inhibit the proliferation of hypoxic tumors.
Conclusions
In conclusion, a series of isomers with regulated photophysical properties as well as controllable mitochondrial selectivity was designed and synthesized by adoption of the cyano positional isomerism strategy. Among the four isomers, the isomer PSMP-4 exhibited a typical AIE effect. The migration of the cyano substituent affected the ML properties of PSMP isomers. The cyano translocation also influences the photoinduced ROS generation capacity of isomers, enabling PSMP-4 to generate O2˙− through the type I photochemistry pathway. PSMP-4 exhibited the best mitochondrial staining performance and excellent photostability during the imaging process, allowing it to achieve in situ mitochondria-targeted imaging without washing as well as to respond to the changes of MMP. By virtue of the excellent photophysical properties and mitochondria specificity, PSMP-4 successfully ablated cancer cells both in vitro and in MCTSs through the potent type I PDT process. This contribution provides a distinctive concept in regulating photophysical features and mitochondria-targeted PDT properties of isomeric luminogens through subtle substituent translocation.
Author contributions
J. Z. and Q. P. performed optical measurements and analysis. J. Z. performed the imaging experiments and analysis. Z. C. and C. Z. performed the synthesis experiments. N. L. and N. Z. wrote the paper. N. L. and N. Z. conceived the idea and supervised the research.
Data availability
The detailed experimental data including synthesis, characterization, optical spectra, and cell imaging associated with this work are available in the ESI.†
Conflicts of interest
There are no conflicts to declare.
Acknowledgements
We are grateful for the financial support from the National Natural Science Foundation of China (22275119, 22077077, and 21975149) and the Fundamental Research Funds for the Central Universities (18QNGG007 and GK202301010).
Notes and references
- J. R. Friedman and J. Nunnari, Mitochondrial Form and Function, Nature, 2014, 505, 335–343 CrossRef CAS PubMed.
- H. M. McBride, M. Neuspiel and S. Wasiak, Mitochondria: More than Just a Powerhouse, Curr. Biol., 2006, 16, R551–R560 CrossRef CAS PubMed.
- A. S. Monzel, J. A. Enríquez and M. Picard, Multifaceted Mitochondria: Moving Mitochondrial Science Beyond Function and Dysfunction, Nat. Metab., 2023, 5, 546–562 CrossRef PubMed.
- L. Galluzzi, O. Kepp and G. Kroemer, Mitochondria: Master Regulators of Danger Signalling, Nat. Rev. Mol. Cell Biol., 2012, 13, 780–788 CrossRef CAS PubMed.
- J. B. Spinelli and M. C. Haigis, The Multifaceted Contributions of Mitochondria to Cellular Metabolism, Nat. Chem. Biol., 2018, 20, 745–754 CrossRef CAS PubMed.
- M. Ott, V. Gogvadze, S. Orrenius and B. Zhivotovsky, Mitochondria, Oxidative Stress and Cell Death, Apoptosis, 2007, 12, 913–922 CrossRef CAS PubMed.
- M. J. Devine and J. T. Kittler, Mitochondria at the Neuronal Presynapse in Health and Disease, Nat. Rev. Neurosci., 2018, 19, 63–80 CrossRef CAS PubMed.
- W. Chen, H. Zhao and Y. Li, Mitochondrial Dynamics in Health and Disease: Mechanisms and Potential Targets, Signal Transduction Targeted Ther., 2023, 8, 333 CrossRef PubMed.
- A. Suomalainen and J. Nunnari, Mitochondria at the Crossroads of Health and Disease, Cell, 2024, 187, 2601–2627 CrossRef CAS PubMed.
- D. C. Wallace, Mitochondria and Cancer, Nat. Rev. Cancer, 2012, 12, 685–698 CrossRef CAS PubMed.
- S. Vyas, E. Zaganjor and M. C. Haigis, Mitochondria and Cancer, Cell, 2016, 166, 555–566 CrossRef CAS PubMed.
- S. Fulda, L. Galluzzi and G. Kroemer, Targeting Mitochondria for Cancer Therapy, Nat. Rev. Drug Discovery, 2010, 9, 447–464 CrossRef CAS PubMed.
- M. P. Murphy and R. C. Hartley, Mitochondria as a Therapeutic Target for Common Pathologies, Nat. Rev. Drug Discovery, 2018, 17, 865–886 CrossRef CAS PubMed.
- Y. Zong, H. Li, P. Liao, L. Chen, Y. Pan, Y. Zheng, C. Zhang, D. Liu, M. Zheng and J. Gao, Mitochondrial Dysfunction: Mechanisms and Advances in Therapy, Signal Transduction Targeted Ther., 2024, 9, 124 CrossRef PubMed.
- S. Samanta, Y. He, A. Sharma, J. Kim, W. Pan, Z. Yang, J. Li, W. Yan, L. Liu, J. Qu and J. S. Kim, Fluorescent Probes for Nanoscopic Imaging of Mitochondria, Chem, 2019, 5, 1697–1726 CAS.
- Q. Ding, X. Wang, Y. Luo, X. Leng, X. Li, M. Gu and J. S. Kim, Mitochondria-Targeted Fluorophore: State of the Art and Future Trends, Coord. Chem. Rev., 2024, 508, 215772 CrossRef CAS.
- X. Guo, N. Yang, W. Ji, H. Zhang, X. Dong, Z. Zhou, L. Li, H. Shen, S. Q. Yao and W. Huang, Mito-Bomb: Targeting Mitochondria for Cancer Therapy, Adv. Mater., 2021, 33, 2007778 CrossRef CAS PubMed.
- J. Morgan and A. R. Oseroff, Mitochondria-Based Photodynamic Anti-Cancer Therapy, Adv. Drug Delivery Rev., 2001, 49, 71–86 CrossRef CAS PubMed.
- J. Mei, N. L. C. Leung, R. T. K. Kowk, J. W. Y. Lam and B. Z. Tang, Aggregation-Induced Emission: Together We Shine, United We Soar!, Chem. Rev., 2015, 115, 11718–11940 CrossRef CAS PubMed.
- H. Zhao, J. Xu, C. Feng, J. Ren, L. Bao, Y. Zhao, W. Tao, Y. Zhao and X. Yang, Tailoring Aggregation Extent of Photosensitizers to Boost Phototherapy Potency for Eliciting Systemic Antitumor Immunity, Adv. Mater., 2022, 34, 2106390 CrossRef CAS PubMed.
- H. Sun, L. Li, R. Guo, Z. Wang, Y. Guo, Z. Li and F. Song, Suppressing ACQ of Molecular Photosensitizers by Distorting the Conjugated-Plane for Enhanced Tumor Photodynamic Therapy, Chem. Sci., 2024, 15, 940–952 RSC.
- X. Li, N. Kwon, T. Guo, Z. Liu and J. Yoon, Innovative Strategies for Hypoxic-Tumor Photodynamic Therapy, Angew. Chem., Int. Ed., 2018, 57, 11522–11531 CrossRef CAS PubMed.
- Y. Wan, L. H. Fu, C. Li, J. Lin and P. Huang, Conquering the Hypoxia Limitation for Photodynamic Therapy, Adv. Mater., 2021, 33, 2103978 CrossRef CAS PubMed.
- D. Ding, K. Li, B. Liu and B. Z. Tang, Bioprobes Based on AIE Fluorogens, Acc. Chem. Res., 2013, 46, 2441–2453 CrossRef CAS PubMed.
- S. Liu, G. Feng, B. Z. Tang and B. Liu, Recent Advances of AIE Light-Up Probes for Photodynamic Therapy, Chem. Sci., 2021, 12, 6488–6506 RSC.
- J. Zhang, Q. Wang, Z. Guo, S. Zhang, C. Yan, H. Tian and W. H. Zhu, High-Fidelity Trapping of Spatial–Temporal Mitochondria with Rational Design of Aggregation-Induced Emission Probes, Adv. Funct. Mater., 2019, 29, 1808153 CrossRef.
- M. Gao and B. Z. Tang, AIE-Based Cancer Theranostics, Coord. Chem. Rev., 2020, 402, 213076 CrossRef CAS.
- M. Kang, Z. Zhang, N. Song, M. Li, P. Sun, X. Chen, D. Wang and B. Z. Tang, Aggregation-Enhanced Theranostics: AIE Sparkles in Biomedical Field, Aggregate, 2020, 1, 80–106 CrossRef.
- Z. Zhang, M. Kang, H. Tan, N. Song, M. Li, P. Xiao, D. Yan, L. Zhang, D. Wang and B. Z. Tang, The Fast-Growing Field of Photo-Driven Theranostics Based on Aggregation-Induced Emission, Chem. Soc. Rev., 2022, 51, 1983–2030 RSC.
- J. Zhuang, B. Wang, H. Chen, K. Zhang, N. Li, N. Zhao and B. Z. Tang, Efficient NIR-II Type-I AIE Photosensitizer for Mitochondria-Targeted Photodynamic Therapy through Synergistic Apoptosis–Ferroptosis, ACS Nano, 2023, 17, 9110–9125 CrossRef CAS PubMed.
- S. Liu, Y. Pei, Y. Sun, Z. Wang, H. Chen, D. Zhu, M. R. Bryce, B. Z. Tang and Y. Chang, Three Birds with One Stone Nanoplatform: Efficient Near-Infrared-Triggered Type-I AIE Photosensitizer for Mitochondria-Targeted Photodynamic Therapy against Hypoxic Tumors, Aggregate, 2024, 5, e547 CrossRef CAS.
- J. Xu, X. Jin, X. Wu, X. Li, C. Li, S. Li, Z. Zhang and J. Hua, Regulating Donor Configuration to Develop AIE-active Type I Photosensitizers for Lipid Droplet Imaging and High-Performance Photodynamic Therapy under Hypoxia, J. Mater. Chem. B, 2024, 12, 6384–6393 RSC.
- Z. Zhuang, J. Dai, M. Yu, J. Li, P. Shen, R. Hu, X. Lou, Z. Zhao and B. Z. Tang, Type I Photosensitizers Based on Phosphindole Oxide for Photodynamic Therapy: Apoptosis and Autophagy Induced by Endoplasmic Reticulum Stress, Chem. Sci., 2020, 11, 3405–3417 RSC.
- J. Li, Z. Zhuang, Z. Zhao and B. Z. Tang, AIE Photosensitizers: Mechanism and Application, View, 2022, 3, 20200121 CrossRef CAS.
- Z. Zhuang, J. Li, P. Shen, Z. Zhao and B. Z. Tang, Exploration and Leverage of Aggregation Effects on Reactive Oxygen
Species Generation in Photodynamic Therapy, Aggregate, 2024, e540 CrossRef CAS.
- H. Li, H. Kim, C. Zhang, S. Zeng, Q. Chen, L. Jia, J. Wang, X. Peng and J. Yoon, Mitochondria-Targeted Smart AIEgens: Imaging and Therapeutics, Coord. Chem. Rev., 2022, 473, 214818 CrossRef CAS.
- A. T. Hoye, J. E. Davoren, P. Wipf, M. P. Fink and V. E. Kagan, Targeting Mitochondria, Acc. Chem. Res., 2008, 41, 87–97 CrossRef CAS PubMed.
- N. Li, Y. Y. Liu, Y. Li, J. B. Zhuang, R. R. Cui, Q. Gong, N. Zhao and B. Z. Tang, Fine Tuning of Emission Behavior, Self-Assembly, Anion Sensing, and Mitochondria Targeting of Pyridinium-Functionalized Tetraphenylethene by Alkyl Chain Engineering, ACS Appl. Mater. Interfaces, 2018, 10, 24249–24257 CrossRef CAS PubMed.
- S. Song, Y. Zhao, M. Kang, Z. Zhang, Q. Wu, S. Fu, Y. Li, H. Wen, D. Wang and B. Z. Tang, Side-Chain Engineering of Aggregation-Induced Emission Molecules for Boosting Cancer Phototheranostics, Adv. Funct. Mater., 2021, 31, 2107545 CrossRef CAS.
- M. Chen, J. Liu, F. Liu, H. Nie, J. Zeng, G. Lin, A. Qin, M. Tu, Z. He, H. H. Y. Sung, I. D. Williams, J. W. Y. Lam and B. Z. Tang, Tailoring the Molecular Properties with Isomerism Effect of AIEgens, Adv. Funct. Mater., 2019, 29, 1903834 CrossRef.
- S. S. Chen, H. Wang, B. Wu, Q. Li, J. Gong, Y. Zhao, Y. Zhao, X. Xiao, J. W. Y. Lam, Z. Zhao, X. D. Luo and B. Z. Tang, Natural Coumarin Isomers with Dramatically Different AIE Properties: Mechanism and Application, ACS Cent. Sci., 2023, 9, 883–891 CrossRef PubMed.
- W. Zhong, J. Zhang, Y. Lin, S. Li, Y. Yang, W. Wang, C. Si, F. E. Kühn, Z. Zhao, X. M. Cai and B. Z. Tang, Multi-Site Isomerization of Synergistically Regulated Stimuli-Responsive AIE Materials Toward Multi-Level Decryption, Chem. Sci., 2024, 15, 3920–3927 RSC.
- J. Zhuang, N. Li, Y. Zhang, B. Li, H. Wen, X. Zhang, T. Zhang, N. Zhao and B. Z. Tang, Esterase-Activated Theranostic Prodrug for Dual Organelles-Targeted Imaging and Synergetic Chemo-Photodynamic Cancer Therapy, CCS Chem., 2022, 4, 1028–1043 CrossRef CAS.
- D. Wang, M. M. S. Lee, G. Shan, R. T. K. Kwok, J. W. Y. Lam, H. Su, Y. Cai and B. Z. Tang, Highly Efficient Photosensitizers with Far-Red/Near-Infrared Aggregation-Induced Emission for in Vitro and in Vivo Cancer Theranostics, Adv. Mater., 2018, 30, 1802105 CrossRef PubMed.
- Y. Li, J. Zhuang, Y. Lu, N. Li, M. Gu, J. Xia, N. Zhao and B. Z. Tang, High-Performance Near-Infrared Aggregation-Induced Emission Luminogen with Mitophagy Regulating Capability for Multimodal Cancer Theranostics, ACS Nano, 2021, 15, 20453–20465 CrossRef CAS PubMed.
- Y. Li, T. Ma, H. Jiang, W. Li, D. Tian, J. Zhu and Z. Li, Anionic Cyanine J-Type Aggregate Nanoparticles with Enhanced Photosensitization for Mitochondria-Targeting Tumor Phototherapy, Angew. Chem., Int. Ed., 2022, 61, e202203093 CrossRef CAS PubMed.
- Z. Zhao, H. Zhang, J. W. Y. Lam and B. Z. Tang, Aggregation-Induced Emission: New Vistas at the Aggregate Level, Angew. Chem., Int. Ed., 2020, 59, 9888–9907 CrossRef CAS PubMed.
- W. Yin, J. Li, Y. Ma, L. Xing, Z. Chen, B. Liu, Y. Huo, Z. Zhao and S. Ji, Molecular Engineering to Enhance the Reactive Oxygen Species Generation of AIEgens and Exploration of Their Versatile Applications, J. Mater. Chem. B, 2023, 11, 8182–8193 RSC.
- M. Li, J. Xia, R. Tian, J. Wang, J. Fan, J. Du, S. Long, X. Song, J. W. Foley and X. Peng, Near-Infrared Light-Initiated Molecular Superoxide Radical Generator: Rejuvenating Photodynamic Therapy against Hypoxic Tumors, J. Am. Chem. Soc., 2018, 140, 14851–14859 CrossRef CAS PubMed.
- F. Khan, A. Ekbote, G. Singh and R. Misra, Mechanochromic Luminogens with Hypsochromically Shifted Emission Switching Property: Recent Advances and Perspectives, J. Mater. Chem. C, 2022, 10, 5024–5064 RSC.
- Y. Q. Dong, J. W. Y. Lam and B. Z. Tang, Mechanochromic Luminescence of Aggregation-Induced Emission Luminogens, J. Phys. Chem. Lett., 2015, 6, 3429–3436 CrossRef CAS PubMed.
- T. Zhang, Y. Li, Z. Zheng, R. Ye, Y. Zhang, R. T. K. Kwok, J. W. Y. Lam and B. Z. Tang, In Situ Monitoring Apoptosis Process by a Self-Reporting Photosensitizer, J. Am. Chem. Soc., 2019, 141, 5612–5616 CrossRef CAS PubMed.
- J. An, S. Tang, G. Hong, W. Chen, M. Chen, J. Song, Z. Li, X. Peng, F. Song and W. H. Zheng, An Unexpected Strategy to Alleviate Hypoxia Limitation of Photodynamic Therapy by Biotinylation of Photosensitizers, Nat. Commun., 2022, 13, 2225 CrossRef CAS PubMed.
|
This journal is © The Royal Society of Chemistry 2024 |
Click here to see how this site uses Cookies. View our privacy policy here.