Optimization of Fenton-like reaction pathways using Ov-containing ZnO@nitrogen-rich porous carbon: the electron transfer and 1O2 triggered non-radical process†
Received
17th August 2024
, Accepted 10th November 2024
First published on 22nd November 2024
Abstract
With the development of persulfate-based Fenton-like catalysis, how to control the PDS reaction pathway is a great challenge. Herein, we prepared catalysts with nitrogen-rich porous carbon (NPC) layers and oxygen vacancy (Ov) sites for PDS activation to degrade sulfamethazine (SMZ). Results revealed that the ZnO@NPC/PDS system exhibited only non-radical pathways, which comprised the singlet oxygen (1O2) and electron transfer process. The intrinsic mechanism underlying the production of active species was further verified by comparing the results of the ZnO@NPC/PDS and ZnO@NPC-Etch/PDS systems, Raman analysis and DFT calculations. Adsorption of PDS by carbon layers resulted in the formation of a catalyst–PDS complex, which not only elongated the S–O bond and accelerated the decomposition of PDS to generate 1O2 but also provided access for electron transfer. Meanwhile, Ov sites increased electron density and electron migration strength, which promoted more electron transfer from Ovs to PDS molecules through nitrogen-rich porous carbon layers. Moreover, the ZnO@NPC/PDS system could maintain a degradation rate of >90% for SMZ in real water matrixes. T. E. S. T software prediction and toxicity tests were used to investigate environmental implications of degradation intermediates, which showed reduced ecological toxicity compared with SMZ. This work fabricated the ZnO@NPC/PDS system and explored the interaction between nitrogen-rich porous carbon layers and Ov to regulate the occurrence of non-radical pathways, which could provide a strategy to control the PDS reaction pathway.
Environmental significance
The treatment of antibiotic wastewater using Fenton reactions has been extensively studied. In this work, we use ZnO as a precursor to synthesize ZnO@NPC, which has a nitrogen-rich porous carbon layer and oxygen vacancies (Ov). ZnO@NPC can optimize Fenton-like reaction pathways, degrading SMZ only through non-radical pathways. Mechanism study further revealed that Ovs played a key role in electron transfer, and nitrogen-rich porous carbon layers served as key sites for the rapid generation of 1O2. This study achieved the targeted design of non-radical pathways, which are expected to be the key pathway for wastewater treatment in the future.
|
1. Introduction
Since the 20th century, there has been a significant increase in emerging contaminants, such as hormones and antibiotics.1,2 Antibiotics, widely used to inhibit the proliferation of bacterial cells, pose considerable risks to ecosystems when released into water and soil.3 Countries worldwide have implemented regulations to address the contamination and hazards posed by antibiotics.4,5 Many techniques, such as adsorption and Fenton-like technology, have been used to remove antibiotics from the environment.6,7 Fenton-like processes could effectively degrade antibiotics present in the environment. The persulfate-based Fenton-like process has been intensively investigated in recent years compared with the traditional Fenton reaction, which demonstrates higher selectivity and pH adaptability.8,9
With a supply of energy, such as thermal or photochemical, persulfate (PS) can remove a wide range of organics by generating reactive oxygen species (ROS) (e.g., SO4˙−, O2˙− and ˙OH) (eqn (1)–(4)).10,11 Recently, non-radical pathways have become new members of Fenton-like processes based on PS, such as singlet oxygen (1O2) and electron transfer, and could achieve higher selectivity and immunity for pollutant removal.12 Non-radical pathways have faster degradation rates and selectivity for pollutants. Additionally, they are resistant to disturbance from intricate water bodies. Non-radical pathways also enhance the mineralization of pollutants, resulting in a more complete degradation of hazardous substances.12,13 The different activation pathways of PS are related to the ways that bonds are broken. Most studies found that PS was prone to produce SO4˙− owing to the instability of the O–O bond.14,15 The S–O bond in peroxydisulfate (PDS) was also stretched and broken to produce peroxymonosulfate (PMS).16 The performance of the catalyst also affects the activation pathway of PDS. Peng et al. found that hollow ZIF-8-derived carbon and solid ZIF-8-derived carbon exhibited different pathways dominating the reaction.17 Consequently, the differences in activation pathways depend on the different ways of bond breaking in PDS and the type of catalyst.
| S2O82− + R˙ = SO42− + SO4˙− + R+ | (1) |
| RH + SO4˙− = R˙ + SO42− | (2) |
| 2S2O82− + 2H2O = 4SO42− + 1O2 + 4H+ | (3) |
|  | (4) |
In the PDS-based heterogenous Fenton-like process, catalysts have been classified as metal-based catalysts (such as metal oxides) and carbon-based catalysts.
18 Carbon catalysts (
e.g., biochar, rGO, and carbon nanotubes) were unique in that they were resistant to acid and alkali, did not have metal leaching, and environmentally friendly, which have received much attention.
19 Yang
et al. developed a metal-free biochar catalyst for PS activation that can degrade AAF by triggering electron transfer and O
2˙
−.
20 Heteroatom doping has also been used to enhance enrichment and electron transfer to design more efficient carbon-based catalysts.
21,22 For example, metal doping can provide more active sites, improve utilization efficiency, and reduce metal agglomeration and leaching.
18 Liu
et al. doped metal into the carbon structure and found a large contribution from non-radical pathways to remove contaminants.
4 Most studies concluded that carbon catalysts favor the emergence of non-radical pathways.
19,23 For example, carbon catalysts can be coupled with PS to enhance the electric field and electron transfer,
24 which was mainly attributed to the fact that PS adsorbed on the material can capture electrons on the contaminants in the reaction system.
12,25 The catalyst–PS complex as reactive species can degrade pollutants by electron transfer.
20 Consequently, carbon catalysts are more favorable to increase the contribution of non-radical pathways.
MOFs are a new type of material that can be classified into various types depending on the organic ligands and metal ions, such as MIL, UIO, and ZIFs.26,27 ZIFs are materials with zeolite-like framework structures and high stability.28 The modifiability of their framework facilitates the targeted design and integration of specific functional groups, making them an excellent carbon precursor.29 Wang et al. carbonized three MOFs to prepare N-doped porous carbons (NPC), finding that the ZIF-8 derived carbons displayed the best performance.30 The ZIF-8 derived nitrogen-doped porous carbon with high nitrogen content had abundant pores and a stable carbon–nitrogen structure, which could be used as an excellent metal carrier in the Fenton-like system.11 Yang et al. prepared a bimetallic catalyst for efficient activation of PMS by introducing Fe and Co into ZIF-8 followed by carbonization. The unique N-coordinated diatomic Fe–Co sites greatly facilitated electron transport and were highly catalytically active in the reaction.31 Therefore, it is a good choice to use ZIF-8 as a precursor of nitrogen-rich porous carbon to construct the required carbon-based catalysts.
In recent studies, Ov present in metal oxides have been the focus of research. According to several researchers, it has been found that Ov possessed a remarkable abundance of localized electrons. These electrons played a crucial role in accelerating the adsorption and activation process of PDS by prolonging the O–O bond.14 The presence of Ov also exerted an influence on the electronic structure of reaction active sites.32 Khiem et al. fabricated yolk–shell Co3O4 nanospheres with various Ov, and the appearance of Ov changed the surrounding local electron structure, which enhanced the electron transfer and free radical production.33 Ov generation can be engineered by improving oxygen-rich materials (e.g., transition metal oxides). Liang et al. prepared a surface Ov-rich catalyst by adjusting the amount of Co doping into zinc ferrite spinel to improve pollutant removal efficiency.34 Hu et al. developed carbon spheres embedded with Ov-rich cobalt oxide nanoparticles; the presence of Ov sites and carbon cooperatively accelerated the electron transfer and exhibited a superior degradation performance.35 Generally, the presence of Ov is important for the increase of catalysis properties, especially the improvement of the electron transfer process. The introduction of Ov into carbon or MOF-derived carbon structure can exploit the electron transfer properties and reactant enrichment capacity. Therefore, the design of metal oxide@NPC catalysts with Ov is beneficial for non-radical pathways in PS-based Fenton-like reactions.
Herein, taking advantage of the constructability of MOF materials, ZIF-8 and ZnO@ZIF-8 were synthesized with Zn2+ and ZnO as precursors, respectively. Nitrogen-rich porous catalysts NPC and ZnO@NPC with different active sites were prepared under high-temperature carbonization. The catalytic performance and active sites of catalysts were investigated through quantitative experiments, characterization, and density-functional theory (DFT) calculations. Notably, the ZnO@NPC/PDS system achieved the efficient degradation of SMZ of up to 0.252 min−1 which was nearly 12 times higher than that of the NPC/PDS system. Based on the analyses, the adsorption of PDS on the carbon layer could form catalyst–PDS complexes, which promoted the breaking of the S–O bond to generate 1O2. More importantly, the presence of Ov could act as an electron source to provide electrons and promote electron transport by increasing the electron density. The interaction between nitrogen-rich porous carbon layers and Ov provides a directional design guide for non-radical pathways of PDS-based Fenton-like processes for degradation pollutants.
2. Experiments and methods
Experimental details, methods, and theoretical basis are all shown in ESI.†
3. Results and discussion
3.1 Material characterizations
The synthesis procedures of ZnO@NPC and NPC are illustrated in Fig. 1a and S1.† Firstly, the XRD patterns (Fig. 1d) indicated the successful synthesis of ZnO@ZIF-8, which had the diffraction peaks of both ZnO and ZIF-8. As shown in Fig. 1b and S2a,† the topography of ZnO@ZIF-8 can be clearly seen and was accumulated into flakes made of particles of ZIF-8. Then, ZnO@NPC was synthesized by calcination of the ZnO@ZIF-8 at 900 °C. SEM and TEM were used to characterize the topography of ZnO@NPC and NPC. As shown in Fig. 1e and f, the shape of NPC (ZIF-8 after carbonization at 900 °C) was demonstrated to be rhombic dodecahedra and the topography of ZnO@NPC (ZnO@ZIF-8 after carbonization at 900 °C) retained a lamellar structure similar to the morphology of ZnO (Fig. S2b†).29 The TEM images verified that ZnO@NPC consisted of nitrogen-rich porous carbon layers and ZnO (Fig. 1g and h). Additionally, the energy dispersive spectroscopy (EDS) presented the distribution and nominal ratio of Zn, N, and O elements (Fig. 1j). The composite structure of ZnO@NPC had nitrogen-rich porous carbon layers and ZnO, which is also demonstrated by TG under air atmosphere, as seen in Fig. S3.† Two of these decompositions were the evaporation of water and decomposition of the carbon layer, respectively. Nitrogen adsorption–desorption isotherms (Fig. 2a) attest that ZnO@NPC had diverse pores compared to NPC and ZnO. The nitrogen adsorption–desorption isotherm of ZnO@NPC was type IV with an H3-type hysteresis loop, indicating that the pore structure was dominated by mesopores. The hierarchical porous structure and capillary cohesion maximized the capability for pollutant adsorption and PDS activation. NPC and ZnO-900 were type I adsorption isotherms, containing narrow microporous structures and smooth surfaces, respectively.17 As shown in Fig. S4,† the pore sizes of ZnO@NPC were uniformly distributed in all particle sizes. XRD was used to illustrate the crystal structure of ZnO@NPC. As shown in Fig. S5,† two distinct peaks that might correspond to graphitic carbon were present in the nitrogen-rich porous carbon.22 As shown in Fig. 1i, the image of electron diffraction also showed the peaks of C (002) and C (100). The broad band of (002) suggested that ZnO@NPC possesses an amorphous-like structure of carbon. Furthermore, the carbon skeletons of ZnO@NPC and NPC were revealed by the Raman spectra (Fig. 2b). The typical peak D band is associated with in-plane defects in honeycomb-like sp2-hybridized carbon. The peak at 1580 cm−1 was the G band, which was attributed to the highly ordered pyrolytic graphitic carbon. The peak-to-height (ID/IG) values of ZnO@NPC and NPC were 1.60 and 1.38, respectively. The 2D band (2680 cm−1) represented the presence of graphitic carbon. ZnO@NPC was found to have more defects and a relatively lower graphitization degree.20
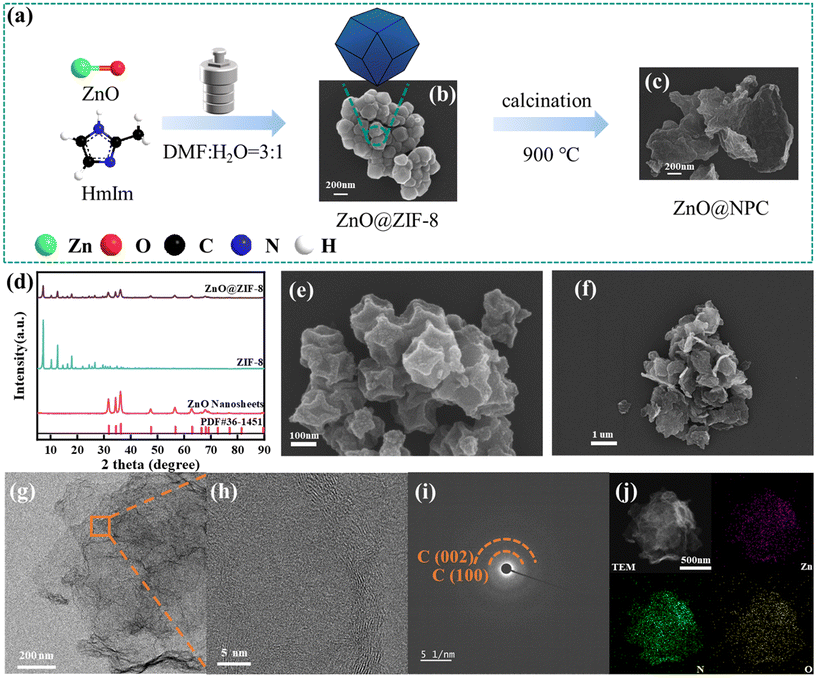 |
| Fig. 1 Preparation process of ZnO@NPC (a); SEM of ZnO@ZIF-8 (b), ZnO@NPC (c) and (f), and NPC (e); XRD (d); TEM of ZnO@NPC (g and h); electron diffraction (i) and EDS (j) of ZnO@NPC. | |
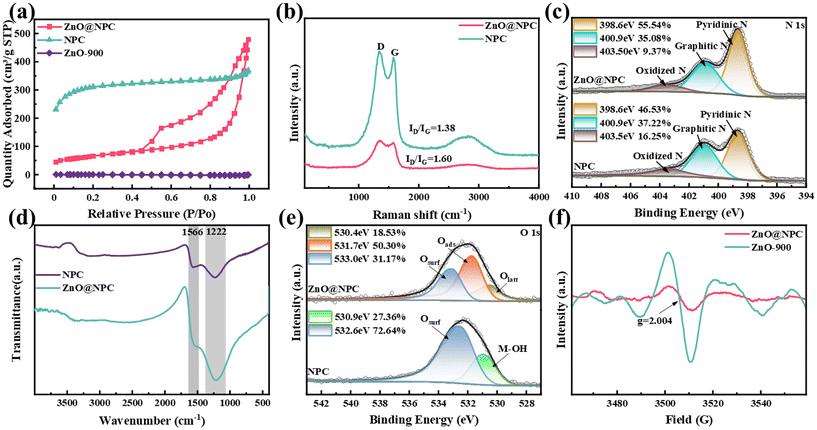 |
| Fig. 2 Nitrogen adsorption–desorption isotherms (a); Raman of ZnO@NPC and NPC (b); N 1s (c) and O 1s (e) of ZnO@NPC and NPC; FT-IR of ZnO@NPC and NPC (d); EPR of different catalysts (f). | |
X-ray photoelectron spectroscopy (XPS) was carried out to further reveal the elemental and chemical structures of the catalysts. Fig. S6a and b† indicated that the main elements of ZnO@NPC and NPC were C, N, Zn, and non-negligible O. The high-resolution C 1s spectrum displayed three main peaks at 284.8, 286.0, and 288.0 eV (Fig. S6c†), which were ascribed to C
C/C–C, C–N, and C–O in ZnO@NPC, respectively. Meanwhile, the fitted peaks of N signals, shown in Fig. 2c, exhibited three binding energy peaks at 398.6, 400.9, and 403.5 eV and were attributed to pyridinic N, graphitic N and oxidized N, respectively. Notably, the characteristic peaks in ZnO@NPC and NPC coincided, suggesting that the nitrogen-rich porous carbon layers of ZnO@NPC were consistent with NPC. Graphitic N has been demonstrated to be the active site for triggering AOPs,11 while oxidized N is generally considered to be less active.19 The Fourier transform infrared spectra (FT-IR) (Fig. 2d) of the catalysts also showed similar results to XPS. Specifically, there were two distinct peaks at 1566 and 1222 cm−1 attributed to the C
C and C–N vibrations, respectively.36,37 The fitted peaks in O 1s appeared at 530.4, 531.7, and 533.0 eV for ZnO@NPC (Fig. 2e) and were attributed to lattice oxygen with metal (Olatt), oxygen species adsorbed on surficial Ov (Oads) and surface adsorbed water molecules (Osurf), respectively.33,34 Oads are usually detected when molecular oxygen (O2) is adsorbed on active sites near Ov and activated to ROS such as superoxide (O2−), monoatomic oxygen (O−), and so on.38 The presence of Oads indicated that Ov were formed and related to the lattice defects in ZnO@NPC. However, NPC did not have a peak of Oads. Asymmetric signals were clearly observed at g = 2.004 in the electron paramagnetic resonance (EPR) spectra (Fig. 2f), which indicated the existence of Ov in ZnO@NPC and ZnO-900.32 The relatively weak signal intensity of ZnO@NPC implied that nitrogen-rich porous carbon layers wrapped the ZnO.
3.2 Evaluation of PDS activation performance
SMZ was selected as a contaminant to evaluate the PDS activation performance of the derived carbon synthesized from different zinc feedstocks (ZnO@NPC and NPC). Firstly, the results of the adsorption experiment of ZnO@NPC and the pristine PDS activation experiment indicated that the chemisorption of ZnO@NPC and PDS-oxidation of SMZ were negligible (30% and 3.5%, respectively) (Fig. S7 and S8a†).39 Meanwhile, the effect of PDS and catalyst dosages on pollutant degradation was explored and results are shown in Fig. S7.† The most cost-effective reaction conditions were chosen, which included catalysts = 0.1 g L−1, PDS = 0.8 mM, and SMZ = 20 mg L−1. As expected, ZnO@NPC exhibited a much higher catalytic degradation rate, with a SMZ degradation efficiency of up to 90% within 15 min (Fig. 3a) under optimal conditions (Fig. 3a). As shown in Fig. S8b,† the Kobs of the ZnO@NPC/PDS/SMZ system was 0.252 min−1, almost 12 times higher than that of the NPC/PDS/SMZ system (0.020 min−1). Notably, the Kobs in the ZnO@NPC/PDS/SMZ system outperformed most catalysts in reported works, as presented in Fig. S9 and Table S1.†
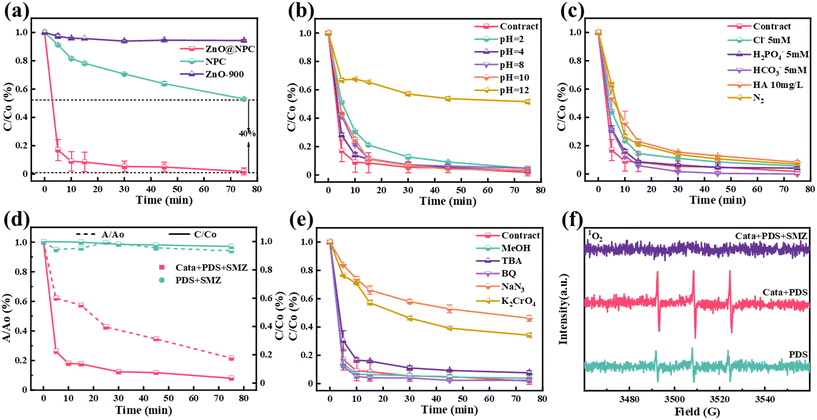 |
| Fig. 3 Degradation effect of different samples (a); influence of the initial pH value on SMZ removal (b); influence of co-existing ions on SMZ removal (c); PDS residues in different systems (d); influence of different quenching agents (e); the EPR detection results of 1O2 (f). Degradation conditions: catalytic = 0.1 g L−1, PDS = 0.8 mM, SMZ = 20 mg L−1, MeOH/TBA = 0.1 M, BQ/NaN3 = 10 mM, K2CrO4 = 5 mM, temp = 25 °C, shake 150 rpm. | |
Next, we further evaluated the possible impact of the ZnO@NPC/PDS system in water treatment. Firstly, the influence of initial pH (Fig. 3b) was assessed, and the ZnO@NPC/PDS system achieved excellent interference resistance between pH 2–10 (Fig. S10a†). Zetasizer measurements (Fig. S10b†) showed that ZnO@NPC had zero charge at pH = 10.4; ZnO@NPC exhibited opposite electric properties compared with those of SMZ and PDS at pH < 10.4, which resulted in electrostatic attraction in the reaction system.40,41 The quick migration of SMZ and S2O52− to ZnO@NPC was beneficial to the reaction, which was consistent with pH experiment results. As shown in Fig. 3c and S11a,† the ZnO@NPC/PDS system could prevent the disturbance of SMZ removal performance from humic acid (HA) or co-existing anions, indicating its strong resistance to the water matrixes. The presence of N2 atmosphere had a slight effect on the degradation effect which might be attributed to the O2 in the solution contributing to the production of reactive oxygen species but not significantly. This suggested that the ZnO@NPC/PDS system is promising for the treatment of complex water.
3.3 Identification of reactive species
Based on the PDS depletion experiments, PDS depletion reached 80% with the presence of ZnO@NPC (Fig. 3d), suggesting that PDS can be activated rapidly by ZnO@NPC to produce reactive species. To identify the dominant active species generated in these systems, methanol (MeOH) and tert-butanol (TBA) were used to quench sulfate radical (SO4˙−) and hydroxyl radical (˙OH). As shown in Fig. 3e, a high proportion of MeOH and TPA (quenching: PDS = 1000
:
1) did not affect the ZnO@NPC/PDS system for SMZ degradation, which suggested that SO4˙− and ˙OH have no contribution in the ZnO@NPC/PDS/SMZ system. 1,4-Benzoquinone (BQ) was used to quench superoxide radicals (O2˙−), and the results suggested that O2˙− played a minimal role. Then, NaN3 was used to quench 1O2, and a significant inhibitory effect on SMZ degradation was exhibited which indicated that 1O2 played an important role in the ZnO@NPC/PDS system (Fig. S11b†). Meanwhile, the decomposition efficiency of SMZ was reduced by 30% in the presence of K2CrO4, as shown in Fig. 3e, suggesting that the electron transfer pathway existed in the ZnO@NPC/PDS system. Therefore, 1O2 and electron transfer both played important roles in the ZnO@NPC/PDS system. In contrast, there was nearly no SO4˙− in the NPC/PDS system, but it had ˙OH and 1O2 (Fig. S12†). To further verify the production of 1O2, DPBF was used as a trapping agent and detected by UV spectrophotometer at a wavelength of 411 nm (ESI† Note S10). The DPBF was fully oxidized within 5 min (Fig. S13†), further suggesting that 1O2 was present in the ZnO@NPC/PDS system, and the rate of 1O2 production was faster than that in the NPC/PDS system. To prove whether the 1O2 originated from dissolved oxygen (DO), N2 was passed over the reaction solution to create a N2 atmosphere. The results are shown in Fig. 3c: the production of 1O2 was independent of DO. Subsequently, the presence of ROS was further explored by EPR characterization. The results showed that the signals of SO4˙−, ˙OH, and O2˙− were not present in the ZnO@NPC/PDS system, and only the signal of 1O2 was detected (Fig. 3f and S14†). Many researchers have reported that 1O2 can degrade pollutants more stably in Fenton-like systems.12,14,42 After the addition of SMZ, the signal peaks of 1O2 disappeared in the ZnO@NPC/PDS/SMZ system, suggesting that 1O2 was in reaction with SMZ. Exploratory experiments showed that 1O2 was the most important reactive species in the ZnO@NPC/PDS/SMZ system, and electron transfer also played an important role.
3.4 Exploration of catalytic mechanisms
Previous studies have reported that 1O2 can be generated from the Haber–Weiss reaction (e.g., O2˙− converts to 1O2), oxygen-containing functional groups (e.g., ketone groups (–C
O)), oxygen vacancies in metal oxides, valence changes of metals, nano-confined catalysts and so on.4,12,42,43 The only metal in ZnO@NPC is Zn2+, which is known to have difficulty changing valence state and low activity because zinc has a filled 3d10 configuration.9,44 PMSO oxidation experiments were used to detect a high-valent metal-oxo that could oxidize PMSO to PMSO2. As shown in Fig. S15,† PMSO was not degraded and oxidized to PMSO2 in the ZnO@NPC/PDS system, further suggesting that no high-valent metal-oxo is present. The nitrogen-rich porous carbon generated after self-growth and pyrolysis on the ZnO surface also indicated there was no nano-confined structure for PDS activation to generate 1O2 in the ZnO@NPC/PDS system. The results from SEM and TEM (Fig. 1c and g) did not exhibit obvious nano-confined structures in the flanks of ZnO@NPC. In order to validate the Haber–Weiss reaction process, we used NBT as a trap for O2˙− in the ZnO@NPC/PDS system (ESI† Note S9). There was no peak detected by the UV spectrophotometer scanning at 530 nm (Fig. 4a), indicating that O2˙− did not exist in this reaction system. O2˙− also did not exist in the NPC/PDS system (Fig. S16†). Therefore, there was only 1O2 in the ZnO@NPC/PDS/SMZ system without free radicals, and the Haber–Weiss reaction process was absent. No oxygen-containing functional groups were observed in the reaction system (Fig. 2d and e), indicating that functional groups did not play a role in 1O2 production.
 |
| Fig. 4 The degradation of NBT in the ZnO@NPC/PDS system (a); O 1s of ZnO@NPC before and after reaction (b); degradation effects of different systems (c); Raman of PDS and ZnO@NPC + PDS (d); energy change (e) and bond lengths (f) before and after adsorption. Degradation conditions: catalysts = 0.1 g L−1, PDS = 0.8 mM, SMZ = 20 mg L−1, NaN3 =10 mM, temp = 25 °C, and shake 150 rpm. | |
The generated 1O2 was further explored, as ˙OH, O2˙− and high-value metals were not present in the ZnO@NPC/PDS system. Comparing the XPS images of O 1s before and after the reaction (Fig. 4b), it was found that the percentage of Oads decreased after the reaction, while the water molecules adsorbed on the surface (Osurf) and lattice oxygen with metal (Olatt) of the material increased. This may be attributed to the Ov in the reaction capturing the oxygen atoms in the PDS and adsorbing more contaminants or oxygen-containing species. To further confirm the role of ZnO in the reaction, ZnO@NPC was acid-immersed to remove ZnO and obtain ZnO@NPC-Etch. It was found that only 60% of SMZ was removed in 15 min by ZnO@NPC-Etch in the SMZ degradation experiments (Fig. 4c), and the rate of degradation was reduced from 0.252 (ZnO@NPC) to 0.007 min−1 (ZnO@NPC-Etch) (Fig. S17a†). This result indicated that the Ov on ZnO were the key active sites of the reaction system. The quenching of the key ROS (1O2) in the ZnO@NPC/PDS system was also carried out. As shown in Fig. 4c, 1O2 played an absolute role in SMZ degradation in the ZnO@NPC-Etch/PDS and NPC/PDS systems, which was different from the dual pathway of 1O2 and electron transfer in the ZnO@NPC/PDS system. This further suggested that Ov may have a direct contributing role to electron transfer, which fitted with other literature that Ov can act as an electron source to provide electrons.33 Ov also accelerated the adsorption and reaction processes and increased the reaction rate. This also indicated that the nitrogen-rich porous carbon layer played a key role in the production of 1O2. Comparing the N 1s before and after the reaction (Fig. S17b and Table S2†), the peak of graphitic N shifted to a lower binding energy and the contents of graphitic N were reduced.25 This suggested that graphitic N may be the adsorption site of PDS and the shift of electron pairs toward PDS through graphitic N drives the production of 1O2, which was similar to a previous study.11,45
Previous studies have reported that PDS can decompose in several ways to generate ROS in the reaction system.10,16 To further reveal the formation process of 1O2 in the ZnO@NPC/PDS system, Raman spectra were taken and are shown in Fig. 4d. After the addition of ZnO@NPC, a sharp decrease in the peak of S2O82− indicated the rapid decomposition of PDS. The appearance of the peak of
showed that PDS formed catalyst–PDS* complexes, mediating the electron transfer occurring for the oxidation of contaminants. The emergence of SO3− (HSO5−) at 1046 cm−1 (ref. 32) suggested that ZnO@NPC may promote the decomposition of PDS to form SO3− and SO42− (eqn (5)). DFT was simulated by the Vienna Ab initio Simulation Package (VASP) software package, where the corresponding energy was first obtained, and the adsorption energy of the material was calculated as −1.86 eV (Fig. 4e). This indicated that ZnO@NPC had better adsorption properties for PDS, which was conducive to the formation of PDS*-mediated structures. By comparing the changes of the O–O (1.321 Å) and S–O (1.961 Å) bonds before and after adsorption (Fig. 4f), it was found that the O–O (1.322 Å) bond lengths had no change and the S–O (2.034 Å) bond lengths increased significantly in the reaction system. This also indicated the high reactivity of ZnO@NPC and can accelerate the PDS decomposition (Fig. S18†). Based on the above results, PDS was more likely to break the S–O bond to produce SO3− after being adsorbed by ZnO@NPC,16 which was consistent with the Raman results. Therefore, the formulas for generating 1O2 were inferred to be eqn (5)–(7).46 This work has constructed a catalyst with Ov and a nitrogen-rich porous carbon layer to activate PDS and accelerate the generation of 1O2 to degrade contaminants more stably.
|  | (5) |
|  | (6) |
The results of the quenching and trapping experiments revealed the existence of an electron transfer pathway in the ZnO@NPC/PDS system in addition to the important role of 1O2. To appraise the electron transfer pathway in the ZnO@NPC/PDS system, K2CrO4 was used as an electron quencher.20,47 As shown in Fig. 3e, the electron also played an important role in the ZnO/PDS/SMZ system. To gain further insights into the activation process between PDS, SMZ, and ZnO@NPC, the Raman spectra were further analyzed. As shown in Fig. 4d, a strong
peak appeared in ZnO@NPC/PDS, suggesting that PDS and ZnO@NPC can form PDS* mediated electron transfer for the oxidation of contaminants. Specifically, the PDS electrophilic molecules formed catalyst–PDS complexes with ZnO@NPC by preabsorbing on the nitrogen-rich porous carbon layers, then causing electron transfer and rearrangement.20 In addition, the characteristic peaks of PDS in the ZnO@NPC/PDS system were slightly shifted towards higher wavenumbers (from 1077 to 1082 cm−1 and from 835 to 836 cm−1) compared to PDS alone, which was derived from the increase in electron density due to electron transfer.14,32 The transfer capacity of ZnO@NPC was further determined and measured by a variety of electrochemical experiments. Firstly, electrochemical impedance spectra (EIS) of ZnO@NPC and NPC accurately modeled the equivalent electrical circuit, which had interfacial charge-transfer resistance (Rt) and electrolyte solution resistance (Rs). As shown in Fig. 5d, a smaller arc radius of ZnO@NPC revealed the lower charge transfer resistance and enhanced electronic conductivity. Fitting calculations showed that the Rt of ZnO@NPC (20.16 Ω) was lower than that of NPC (25.42 Ω). Furthermore, the more distinct current jumping brought on by PDS addition in the reaction system indicated that ZnO@NPC can transport more electrons to PDS than NPC, which was exposed in the i–t curves (Fig. 5a and S19†). Meanwhile, a weak change in current when SMZ was added to the system may be attributed to the interactions of ZnO@NPC, PDS, and SMZ to form an equilibrium system around the working electrode.20 Linear sweep voltammetry (LSV) (Fig. S20†) also showed that the current increases gradually with the addition of PDS and SMZ, which was in agreement with the results of the i–t curves, and the current was maximum when both PDS and SMZ were present. Overall, there were more unstable electron sources to transport and accelerate electrons in the reaction process of the ZnO@NPC/PDS/SMZ system, indicating that ZnO@NPC can activate PDS more rapidly.
 |
| Fig. 5
I–t curves (a); current change graph of GOP (b); pollutant removal rate (c); electrochemical impedance spectroscopy (d); CDD before (e) and after (f) adsorption; chromatogram of different times (g); TOC removal effect (h); acute toxicity of T. pyriformis LC50 (48 h) (i). | |
The galvanic oxidation process (GOP) device could directly elucidate the movement of electrons during the reaction and consisted of two beakers with salt solution connected by an agarose salt bridge, and a galvanometer connecting two catalyst-loaded graphite electrodes formed the current pathway (Fig. S21†). Fig. 5b reveals that there was no distinct current in the systems without PDS. However, a distinct change of current appeared in the ZnO@NPC, PDS, and SMZ coexisting system. In the first 5 min of the ZnO@NPC/PDS/SMZ reaction, the electron transfer rate was fast and gradually stabilized, which was consistent with the rapid decrease of SMZ concentration. The change in SMZ concentration was measured in the GOP process, and it was found that pure electron transfer could remove about 15% of SMZ (Fig. 5c), which further indicated that electron transfer had an important role in the ZnO@NPC/PDS system. This was consistent with the assumed goal that nitrogen-rich porous carbon layers can act in conjunction with Ov to improve the electron transfer pathway and degrade pollutants more stably. To further verify the electron distribution and the occurrence of electron transfer, the charge density difference (CDD) of ZnO@NPC before and after PDS adsorption was calculated. As shown in Fig. 5e, before PDS adsorption, there were more yellow areas near the Ov, suggesting that the Ov could increase the unstable charge density. Following the adsorption of PDS (Fig. 5f), charge density was significantly changed. Specifically, no significant charge accumulation near the Ov in the ZnO@NPC was seen, but a considerable electron accumulation at PDS was clearly observed. This was direct evidence of the greater number of electrons transferred from Ov to the PDS molecule through the nitrogen-rich porous carbon layers. These results further illustrated the importance of Ov for the ZnO@NPC/PDS system and indicated that the presence of Ov can provide a large source of electrons and accelerate electron transfer to PDS.
3.5 Identification and toxicity analysis of degradation products
To further investigate the intermediates and degradation pathways of SMZ in the ZnO@NPC/PDS/SMZ system, chromatograms of reaction solutions with different degradation times were determined and analyzed using liquid chromatography–mass spectrometry (LC–MS). As shown in Fig. 5g, the chromatographs of the reaction solutions at different times clearly show that the prominent peak of SMZ corresponding to a retention time of 5.69 min and an M/Z value of 279 decreased rapidly within 5 min. The other intermediate degradation products demonstrated a propensity to rise and then fall, suggesting that SMZ was progressively decomposed into some smaller molecules and further oxidized to inorganic substances. The possible degradation pathways and mass spectra are shown in Fig. S22,† and detailed intermediates information is given in Table S3.† The total organic carbon (TOC) of SMZ and mixture pollutants (SMZ, NOF, and TC) (Fig. 5h) decreased by 92.4% and 84.8%, respectively, indicating that the ZnO@NPC/PDS/SMZ system exhibited a strong oxidation towards pollutants. As shown in Fig. S23,† the LC–MS analysis of the reaction intermediates revealed two possible degradation pathways. For path one, SMZ was converted to P1 through hydroxylation. For path two, SMZ was converted to P2 by taking off SO2. Then, P1 and P2 are be transformed to P3 and P5 by the attack of 1O2 and converted to small molecules with deprotonation. In addition, toxicological analyses were carried out in two ways. First, the toxicity prediction results of degradation intermediates, as shown in Table S4,† included the acute toxicity for T. pyriformis, fathead minnow, and Daphnia magna, and the bioconcentration factor, mutagenicity value, and developmental toxicity value, which were predicted via T. E. S. T. As shown in Fig. 5i and S24,† the acute toxicity to T. pyriformis (or other bios) decreased progressively with the reaction proceeding, and the small molecules (such as P4) were relatively not harmful. Then, seed germination and radicle elongation tests (Fig. S25†) also showed that the toxicity of the degradation intermediates was low, as shown in Table S5.† SMZ had the most toxic and greatest effect on seeds, while the toxicity of the degradation intermediates was greatly reduced.
3.6 Evaluation of practical applications
To evaluate the degradation capacity of the reaction systems in real water, SMZ removal experiments were carried out in Xiangjiang River water (Changsha, China), municipal wastewater (Changsha, China), and tap water. It was found that there was no significant change in the catalytic performance of ZnO@NPC (Fig. S26a†), which was consistent with the co-existing ions experiment. This indirectly proved that the ZnO@NPC/PDS system is not dominated by free radicals, because free radicals are susceptible to interference by other substances.48,49 The NPC/PDS system had low degradation performance in real water (Fig. S26b†). In addition, the oxidation performance of the ZnO@NPC/PDS system was further estimated for the degradation of phenol, 4-chlorophenol (4-CP), tetracycline (TC), norfloxacin (NOF), sulfamethizole (SMT), benzoic acid (BA), and carbamazepine (CBZ). As shown in Fig. S27,† phenol, 4-CP, and TC were almost completely decomposed within 10 min. The results showed that the ZnO@NPC/PDS system with non-radical pathways could degrade electron-rich pollutants effectively and has immunity from interference.12 Compounds with electron-withdrawing groups were resistant to degradation in the ZnO@NPC/PDS system (e.g., BA and CBZ).4 This was consistent with the findings of previous studies, and may be attributed to the blocking of electron transfer in solution. The catalytic performance of the ZnO@NPC was below expectation with the number of times of use (Fig. S28†), which may be attributed to the change of the nitrogen-rich porous carbon layer on the surface and Ov in the catalyst. Comparing the O 1s and N 1s before and after the reaction (Fig. 4b and S17b†), the poor cycling performance was attributed to the reduced electron structure in the carbon layers and the depletion of Ov, which made it difficult for PDS to adsorb and transfer electrons.
4. Conclusion
In summary, this work developed metal oxides@carbon catalysts with Ov and nitrogen-rich porous carbon layers which achieved targeted modulation of non-radical pathways. The ZnO@NPC/PDS system could purify real water matrixes and showed good stability and selectivity. Experimental analyses and theoretical calculations revealed the mechanism of reactive species generation and pollutant removal. It was found that Ov played a key role in electron transfer and could provide a number of electrons for electron transfer as the source. Therefore, ZnO@NPC with Ov had better degradation properties than NPC. The nitrogen-rich porous carbon layers of ZnO@NPC served as key adsorption sites for PDS, as they can promote the breaking of the S–O bond on PDS and the rapid generation of 1O2. Meanwhile, the nitrogen-rich porous carbon layers also modulated the electron distribution of Ov and enhanced electron transfer, which could further control the occurrence of non-radical pathways. The intermediates of SMZ showed reduced ecological toxicity compared with SMZ according to the results of T. E. S. T prediction and germination experiments. Overall, this study achieved the targeted design of non-radical pathways, which are expected to be key for wastewater treatment in the future.
Data availability
The data supporting this article have been included as part of the ESI.†
Author contributions
Zhenfeng Zhang: writing – original draft; conceptualization; data curation; formal analysis; Tianli Xiong: writing – review & editing; validation; Haihao Peng: writing – review & editing; conceptualization; validation; Honglin Zhang: writing – review & editing; Siying He: writing – review & editing; Xuran Liu: writing – review & editing; Yanan Liu: writing – review & editing; Wenyi Feng: writing – review & editing; Zhaohui Yang: funding acquisition; Weiping Xiong: funding acquisition.
Conflicts of interest
The authors declare that they have no known competing financial interests or personal relationships that could have appeared to influence the work reported in this paper.
Acknowledgements
The work was financially supported by the National Natural Science Foundation of China (52379064), Hunan Provincial Natural Science Foundation of China (2023JJ10013), Special Funding for the Construction of Hunan's Innovative Province (2021SK2040), the Science and Technology Innovation Program of Hunan Province (2021RC3133), the National Youth Foundation of China (52300227), the Hunan Municipal Natural Science Foundation (2023JJ41048), and the Changsha Municipal Natural Science Foundation (kq2208423).
References
- T. Rasheed, M. Bilal, F. Nabeel, M. Adeel and H. M. N. Iqbal, Environmentally-related contaminants of high concern: Potential sources and analytical modalities for detection, quantification, and treatment, Environ. Int., 2019, 122, 52–66 CrossRef CAS PubMed.
- Y.-J. Zhang, G.-X. Huang, L. R. Winter, J.-J. Chen, L. Tian, S.-C. Mei, Z. Zhang, F. Chen, Z.-Y. Guo, R. Ji, Y.-Z. You, W.-W. Li, X.-W. Liu, H.-Q. Yu and M. Elimelech, Simultaneous nanocatalytic surface activation of pollutants and oxidants for highly efficient water decontamination, Nat. Commun., 2022, 13, 3005 CrossRef CAS PubMed.
- F. C. Cabello, Heavy use of prophylactic antibiotics in aquaculture: a growing problem for human and animal health and for the environment, Environ. Microbiol., 2006, 8, 1137–1144 CrossRef CAS PubMed.
- T. Liu, S. Xiao, N. Li, J. Chen, X. Zhou, Y. Qian, C.-H. Huang and Y. Zhang, Water decontamination via nonradical process by nanoconfined Fenton-like catalysts, Nat. Commun., 2023, 14, 2881 CrossRef CAS PubMed.
- F. Ahmadijokani, H. Molavi, S. Tajahmadi, M. Rezakazemi, M. Amini, M. Kamkar, O. J. Rojas and M. Arjmand, Coordination chemistry of metal–organic frameworks: Detection, adsorption, and photodegradation of tetracycline antibiotics and beyond, Coord. Chem. Rev., 2022, 464, 214562 CrossRef CAS.
- S. Li, Y. Wu, H. Zheng, H. Li, Y. Zheng, J. Nan, J. Ma, D. Nagarajan and J.-S. Chang, Antibiotics degradation by advanced oxidation process (AOPs): Recent advances in ecotoxicity and antibiotic-resistance genes induction of degradation products, Chemosphere, 2023, 311, 136977 CrossRef CAS.
- W. Xue, X. Shi, J. Guo, S. Wen, W. Lin, Q. He, Y. Gao, R. Wang and Y. Xu, Affecting factors and mechanism of removing antibiotics and antibiotic resistance genes by nano zero-valent iron (nZVI) and modified nZVI: A critical review, Water Res., 2024, 253, 121309 CrossRef CAS.
- N. Jiang, H. Xu, L. Wang, J. Jiang and T. Zhang, Nonradical Oxidation of Pollutants with Single-Atom-Fe(III)-Activated Persulfate: Fe(V) Being the Possible Intermediate Oxidant, Environ. Sci. Technol., 2020, 54, 14057–14065 CrossRef CAS PubMed.
- Z. Zhao, H. Tan, P. Zhang, X. Liang, T. Li, Y. Gao and C. Hu, Turning the Inert Element Zinc into an Active Single-Atom Catalyst for Efficient Fenton-Like Chemistry, Angew. Chem., Int. Ed. Engl., 2023, 62, e202219178 CrossRef CAS PubMed.
- T. Yuan, S. Ding, F. Xue, Z. Du, X. Yang, Q. Han, M. Ma and X. Chen, Reactivity and reaction pathways of peroxymonosulfate and peroxydisulfate with neonicotinoid insecticides, Water Res., 2024, 248, 120852 CrossRef CAS.
- Y. Shi, D. Feng, S. Ahmad, L. Liu and J. Tang, Recent advances in metal–organic frameworks–derived carbon-based materials in sulfate radical-based advanced oxidation processes for organic pollutant removal, Chem. Eng. J., 2023, 454, 140244 CrossRef CAS.
- J. Yu, L. Tang, Y. Pang, X. Liang, Y. Lu, H. Feng, J. Wang, L. Deng, J. Zou, X. Zhu and J. Tang, Non-radical oxidation in environmental catalysis: Recognition, identification, and perspectives, Chem. Eng. J., 2022, 433, 134385 CrossRef CAS.
- L. Bu, S. Zhou, S. Zhu, Y. Wu, X. Duan, Z. Shi and D. D. Dionysiou, Insight into carbamazepine degradation by UV/monochloramine: Reaction mechanism, oxidation products, and DBPs formation, Water Res., 2018, 146, 288–297 CrossRef CAS.
- Z. Zhao, P. Wang, C. Song, T. Zhang, S. Zhan and Y. Li, Enhanced Interfacial Electron Transfer by Asymmetric Cu-Ov-In Sites on In2O3 for Efficient Peroxymonosulfate Activation, Angew. Chem., Int. Ed., 2023, 62, e202216403 CrossRef CAS.
- Z. Wang, J. Bao, H. He, S. Mukherji, L. Luo and J. Du, Single-Atom iron catalyst activating peroxydisulfate for efficient organic contaminant degradation relying on electron transfer, Chem. Eng. J., 2023, 458, 141513 CrossRef CAS.
- Y. Shang, X. Liu, Y. Li, Y. Gao, B. Gao, X. Xu and Q. Yue, Boosting fenton-like reaction by reconstructed single Fe atom catalyst for oxidizing organics: Synergistic effect of conjugated π-π sp2 structured carbon and isolated Fe-N4 sites, Chem. Eng. J., 2022, 446, 137120 CrossRef.
- H. Peng, W. Xiong, Z. Yang, J. Tong, Y. Xiang, Z. Zhang and Z. Xu, Insights into the mechanism of persulfate activation by hollow MOF-derived carbon: electron transfer-triggered non-radical oxidization for antibiotic removal, Environ. Sci.: Nano, 2024, 11, 216–228 RSC.
- H. C. Yan, C. Lai, S. Y. Liu, D. B. Wang, X. R. Zhou, M. M. Zhang, L. Li, X. P. Li, F. H. Xu and J. X. Nie, Metal-carbon hybrid materials induced persulfate activation: Application, mechanism, and tunable reaction pathways, Water Res., 2023, 234, 119808 CrossRef CAS.
- H. Luo, H. Fu, H. Yin and Q. Lin, Carbon materials in persulfate-based advanced oxidation processes: The roles and construction of active sites, J. Hazard. Mater., 2022, 426, 128044 CrossRef CAS PubMed.
- H. Yang, R. Qiu, Y. Tang, S. Ye, S. Wu, F. Qin, L. Xiang, X. Tan, G. Zeng and M. Yan, Carbonyl and defect of metal-free char trigger electron transfer and O2·− in persulfate activation for Aniline aerofloat degradation, Water Res., 2023, 231, 119659 CrossRef CAS.
- G. Qu, P. Jia, S. Tang, M. N. Pervez, Y. Pang, B. Li, C. Cao and Y. Zhao, Enhanced peroxymonosulfate activation via heteroatomic doping defects of pyridinic and pyrrolic N in 2D N-doped carbon nanosheets for BPA degradation, J. Hazard. Mater., 2024, 461, 132626 CrossRef CAS PubMed.
- M. Li, Z. He, H. Zhong, L. Hu and W. Sun, Multi-walled carbon nanotubes facilitated Roxarsone elimination in SR-AOPs by accelerating electron transfer in modified electrolytic manganese residue and forming surface activated-complexes, Water Res., 2021, 200, 117266 CrossRef CAS PubMed.
- C. Cheng, W. Ren, F. Miao, X. Chen, X. Chen and H. Zhang, Generation of FeIV=O and its Contribution to Fenton-Like Reactions on a Single-Atom Iron−N−C Catalyst, Angew. Chem., Int. Ed., 2023, 62, e202218510 CrossRef CAS PubMed.
- C. Yu, Z. Zhao, Y. Zong, L. Xu, B. Zhang and D. Wu, Electric field-enhanced coupled with metal-free peroxymonosulfate activactor: The selective oxidation of nonradical species-dominated system, Water Res., 2022, 227, 119323 CrossRef CAS PubMed.
- H. Ming, X. Bian, J. Cheng, C. Yang, Y. Hou, K. Ding, J. Zhang, M. Anpo and X. Wang, Carbon nitride with a tailored electronic structure toward peroxymonosulfate activation: A direct electron transfer mechanism for organic pollutant degradation, Appl. Catal., B, 2024, 341, 123314 CrossRef CAS.
- M. Jia, W. Xiong, Z. Yang, J. Cao, Y. Zhang, Y. Xiang, H. Xu, P. Song and Z. Xu, Metal-organic frameworks and their derivatives-modified photoelectrodes for photoelectrochemical applications, Coord. Chem. Rev., 2021, 434, 213780 CrossRef CAS.
- H. Zhang, M. Jia, J. Tong, H. Peng, Y. Xiang, Z. Chen, Z. Xu, Z. Yang and W. Xiong, Coupling effects between metal–organic framework derivatives and oxygen-deficient TiO2 nanotubes: identified charge-transfer processes and photoelectric synergistic effect, Environ. Sci.: Nano, 2023, 10, 1993–2009 RSC.
- M. Jia, Q. Liu, W. Xiong, Z. Yang, C. Zhang, D. Wang, Y. Xiang, H. Peng, J. Tong, J. Cao and H. Xu, Ti3+ self-doped TiO2 nanotubes photoelectrode decorated with Ar-Fe2O3 derived from MIL-100(Fe): Enhanced photo-electrocatalytic performance for antibiotic degradation, Appl. Catal., B, 2022, 310, 100318 CrossRef.
- Z. Mo, D. Tai, H. Zhang and A. Shahab, A comprehensive review on the adsorption of heavy metals by zeolite imidazole framework (ZIF-8) based nanocomposite in water, Chem. Eng. J., 2022, 443, 136320 CrossRef CAS.
- G. Wang, S. Chen, X. Quan, H. Yu and Y. Zhang, Enhanced activation of peroxymonosulfate by nitrogen doped porous carbon for effective removal of organic pollutants, Carbon, 2017, 115, 730–739 CrossRef CAS.
- J. Yang, D. Zeng, J. Li, L. Dong, W.-J. Ong and Y. He, A highly efficient Fenton-like catalyst based on isolated diatomic Fe-Co anchored on N-doped porous carbon, Chem. Eng. J., 2021, 404, 126376 CrossRef CAS.
- L. Wu, Z. Sun, Y. Zhen, S. Zhu, C. Yang, J. Lu, Y. Tian, D. Zhong and J. Ma, Oxygen Vacancy-Induced Nonradical Degradation of Organics: Critical Trigger of Oxygen (O2) in the Fe–Co LDH/Peroxymonosulfate System, Environ. Sci. Technol., 2021, 55, 15400–15411 CrossRef CAS PubMed.
- T. C. Khiem, N. N. Huy, E. Kwon, J. Lee, W. D. Oh, X. Duan, S. Wacławek, H. Wang, G. Lisak, F. Ghanbari and K.-Y. A. Lin, Electron transfer-mediated enhancement of superoxide radical generation in fenton-like process: Key role of oxygen vacancy-regulated local electron density of cobalt sites, Appl. Catal., B, 2024, 343, 123490 CrossRef CAS.
- L. Liang, P. Cao, X. Qin, S. Wu, H. Bai, S. Chen, H. Yu, Y. Su and X. Quan, Oxygen vacancies-driven nonradical oxidation pathway of catalytic ozonation for efficient water decontamination, Appl. Catal., B, 2023, 325, 122321 CrossRef CAS.
- J. Hu, B. Qian, X. Zeng, Y. Qi, Y. Liu, L. Zhang and X. Zhang, Oxygen vacant Co3O4in situ embedded on carbon spheres: cooperatively tuning electron transfer for boosted peroxymonosulfate activation, J. Mater. Chem. A, 2021, 9, 16489–16499 RSC.
- X. Chen, W.-D. Oh, Z.-T. Hu, Y.-M. Sun, R. D. Webster, S.-Z. Li and T.-T. Lim, Enhancing sulfacetamide degradation by peroxymonosulfate activation with N-doped graphene produced through delicately-controlled nitrogen functionalization via tweaking thermal annealing processes, Appl. Catal., B, 2018, 225, 243–257 CrossRef CAS.
- B. Yang, J. Huang, J. Tong, H. Peng, Y. Xiang, M. Ruan, Z. Chen, Z. Yang and W. Xiong, Microwave synthesis of Fe–Cu diatomic active center MOF: synergistic cyclic catalysis of persulfate for degrading norfloxacin, Environ. Sci.: Nano, 2023, 10, 2778–2789 RSC.
- X. Wei, K. Li, X. Zhang, Q. Tong, J. Ji, Y. Cai, B. Gao, W. Zou and L. Dong, CeO2 nanosheets with anion-induced oxygen vacancies for promoting photocatalytic toluene mineralization: Toluene adsorption and reactive oxygen species, Appl. Catal., B, 2022, 317, 121694 CrossRef CAS.
- H. Peng, W. Xiong, Z. Yang, J. Cao, M. Jia, Y. Xiang, Q. Hu and Z. Xu, Facile fabrication of three-dimensional hierarchical porous ZIF-L/gelatin aerogel:
Highly efficient adsorbent with excellent recyclability towards antibiotics, Chem. Eng. J., 2021, 426, 130798 CrossRef CAS.
- W. Xiong, Y. Ye, D. He, S. He, Y. Xiang, J. Xiao, W. Feng, M. Wu, Z. Yang and D. Wang, Deregulation of Ribosome Biogenesis in Nitrite-Oxidizing Bacteria Leads to Nitrite Accumulation, Environ. Sci. Technol., 2023, 57, 16673–16684 CrossRef CAS PubMed.
- J. Liang, M. Yan, Z. Zhu, L. Lu, J. Ding, Q. Zhou, X. Gao, N. Tang, S. Li, X. Li and G. Zeng, The role of microorganisms in phosphorus cycling at river-lake confluences: Insights from a study on microbial community dynamics, Water Res., 2025, 268, 122556 CrossRef CAS.
- P. Shao, J. Tian, F. Yang, X. Duan, S. Gao, W. Shi, X. Luo, F. Cui, S. Luo and S. Wang, Identification and Regulation of Active Sites on Nanodiamonds: Establishing a Highly Efficient Catalytic System for Oxidation of Organic Contaminants, Adv. Funct. Mater., 2018, 28, 1705295 CrossRef.
- Q. Yi, J. Ji, B. Shen, C. Dong, J. Liu, J. Zhang and M. Xing, Singlet Oxygen Triggered by Superoxide Radicals in a Molybdenum Cocatalytic Fenton Reaction with Enhanced REDOX Activity in the Environment, Environ. Sci. Technol., 2019, 53, 9725–9733 CrossRef CAS.
- J. Wang, H. Li, S. Liu, Y. Hu, J. Zhang, M. Xia, Y. Hou, J. Tse, J. Zhang and Y. Zhao, Turning on Zn 4s Electrons in a N2-Zn-B2 Configuration to Stimulate Remarkable ORR Performance, Angew. Chem., Int. Ed., 2021, 60, 181–185 CrossRef CAS PubMed.
- X. Duan, H. Sun, Y. Wang, J. Kang and S. Wang, N-Doping-Induced Nonradical Reaction on Single-Walled Carbon Nanotubes for Catalytic Phenol Oxidation, ACS Catal., 2014, 5, 553–559 CrossRef.
- Z. Wu, B. Huang, X. Wang, C.-S. He, Y. Liu, Y. Du, W. Liu, Z. Xiong and B. Lai, Facilely Tuning the First-Shell Coordination Microenvironment in Iron Single-Atom for Fenton-like Chemistry toward Highly Efficient Wastewater Purification, Environ. Sci. Technol., 2023, 57, 14046–14057 CrossRef CAS PubMed.
- J. Yu, L. Tang, Y. Pang, G. Zeng, H. Feng, J. Zou, J. Wang, C. Feng, X. Zhu, X. Ouyang and J. Tan, Hierarchical porous biochar from shrimp shell for persulfate activation: A two-electron transfer path and key impact factors, Appl. Catal., B, 2020, 260, 118160 CrossRef CAS.
- L. Xie, P. Wang, Y. Li, D. Zhang, D. Shang, W. Zheng, Y. Xia, S. Zhan and W. Hu, Pauling-type adsorption of O2 induced electrocatalytic singlet oxygen production on N–CuO for organic pollutants degradation, Nat. Commun., 2022, 13, 5560 CrossRef CAS.
- Y. Zhao, L. Yu, C. Song, Z. Chen, F. Meng and M. Song, Selective Degradation of Electron-Rich Organic Pollutants Induced by CuO@Biochar: The Key Role of Outer-Sphere Interaction and Singlet Oxygen, Environ. Sci. Technol., 2022, 56, 10710–10720 CrossRef CAS PubMed.
|
This journal is © The Royal Society of Chemistry 2025 |
Click here to see how this site uses Cookies. View our privacy policy here.