Nano zinc oxide activates MdCDF2 to promote DNA replication and cell proliferation in apple calli†
Received
25th October 2024
, Accepted 18th December 2024
First published on 27th December 2024
Abstract
Apple (Malus domestica) is grown worldwide. The yield and quality of apple depend on cultivation techniques and nutrition. Nano zinc oxide (ZnO NPs) has been widely applied in agricultural production, commonly used in fertilizers to help crops increase yield and enhance abiotic stress tolerance. However, there are few studies on the effects of ZnO NPs on apple growth. This study found that treatment with 500 ppm ZnO NPs can promote the proliferation of apple callus cells. Using Inductively Coupled Plasma Optical Emission Spectrometry (ICP-OES) technology, it was confirmed that ZnO NPs can enter apple callus cells more efficiently than ZnO bulk particles (ZnO BPs) and ZnSO4. Through scanning electron microscopy (SEM) and transmission electron microscopy (TEM), it was observed that the ZnO NPs were mainly aggregated in the cytoplasm and nucleus. Transcriptome analysis showed that the expression levels of some zinc finger-containing transcription factors (TFs) were up-regulated after treatment with 500 ppm ZnO NPs. Kyoto Encyclopedia of Genes and Genomes (KEGG) enrichment analysis and Gene Ontology (GO) analysis found that the expression levels of some DNA replication-related genes were significantly changed. DNA pull-down experiments proved that MdCDF2 (a zinc finger-containing TF) can bind to the promoter regions of three DNA replication-related genes (MdClpB1, MD01G1182200, MD12G1082300). These data indicated that ZnO NPs may promote DNA replication and cell proliferation in apple callus cells by increasing the expression level of MdCDF2. This study provides new insights into the molecular mechanisms by which ZnO NPs enhance plant growth efficiency and crop yield.
Environmental significance
The production and application of nano zinc oxide (ZnO NPs) in agriculture are on the rise, with potential environmental implications. This study reveals that ZnO NPs can enhance apple growth by promoting cell proliferation, a finding that could have significant agricultural benefits. However, the environmental deposition of ZnO NPs raises concerns about their accumulation in soil and water, and subsequent bioaccumulation in the food chain. This could lead to increased human exposure to these nanoparticles, highlighting the need for further research on their environmental and health impacts. Our work provides insights into the molecular mechanisms of ZnO NPs in plants, which is crucial for developing safe and effective nanotechnological applications in agriculture.
|
1. Introduction
As a global fruit, apple is cultivated in over 80 countries worldwide.1 Nowadays, China is the world's largest producer and exporter of apples.2 Apples possess high nutritional value, being rich in a variety of antioxidant compounds and serving as an important dietary source of the trace element zinc.3–6 Apple is a plant that is highly sensitive to zinc, and a deficiency of this element in the soil can lead to the development of zinc deficiency chlorosis, commonly known as little leaf disease, in apple trees.7 Consequently, studying the molecular mechanisms of zinc absorption and utilization in apple and their effects on apple growth is important for improving apple yield and quality.
Zinc plays a pivotal role in various aspects of plant growth and development.8 For instance, the application of zinc fertilizers can significantly enhance root development and leaf growth, in Zea mays L. and Glycine max L.9 Zinc fertilizers can increase the chlorophyll content in leaves of Oryza sativa L.,10 strengthen photosynthesis, and thereby improve yield and quality. In plants, zinc fertilizer not only promotes root development, leaf formation, and chlorophyll synthesis but also enhances stress resistance. In various crops, such as Triticum aestivum, Helianthus annuus L., Solanum lycopersicum, and Brassica oleracea L. var. capitata f. rubra, adequate zinc demonstrates the function of enhancing plant drought resistance.11–13 However, both zinc deficiency and excessive intake can result in harm.14–16 In Phaseolus vulgaris L., treatment with 50 ppm ZnSO4·7H2O results in a relative zinc deficiency, leading to a reduction in biomass, a decrease in chlorophyll content, and an increase in the levels of malondialdehyde (MDA) and hydrogen peroxide (H2O2).17 Similarly, the application of an excessive amount of zinc fertilizer to plants can also lead to some adverse reactions. In Brassica chinensis L., exposure to 1000–2000 ppm of ZnO NPs results in a decrease in chlorophyll content and an increase in membrane permeability, leading to chlorosis and leaf senescence.18 Treatment of Cajanus cajan (L) Millsp. with 500–1000 ppm ZnO NPs causes a reduction in nodulation, nitrogen fixation, chlorophyll content, and yield, along with an increase in oxidative stress, disturbance in mineral nutrition, and an enhancement of both enzymatic and non-enzymatic antioxidant defense systems.19–21 Thus, zinc must be carefully regulated within appropriate concentrations and timeframes.
In recent years, with the extensive research and application of nanomaterials, ZnO NPs have gradually been widely applied in agricultural production.22 ZnO NPs refer to ZnO particles with dimensions in the range of 1 to 100 nm.23 Compared to ZnO BPs, ZnO NPs exhibit unique properties such as a higher surface area-to-volume ratio, quantum-size effect, and enhanced chemical reactivity. Recent studies indicate that an appropriate concentration of ZnO NPs can promote the growth of Zea mays L.,24Solanum lycopersicum,25Cucurbita pepo L.,26Arabidopsis,27etc. In Zea mays L., 50 ppm ZnO NPs treatment could reduce the time required for seed germination and increase the average germination rate. Additionally, ZnO NPs at 50 ppm and 100 ppm significantly increased the growth and elongation of the root system and shoot, which is beneficial for the accumulation of biomass.28 Similar conclusions were reached in studies involving cabbage29 (<80 ppm) and Spinacia oleracea30 (10 ppm). However, the impact of ZnO NPs on plant physiology is dose-dependent, and higher concentrations may lead to toxicity, affecting plant development and overall health. High concentrations of ZnO NPs can inhibit the growth of Lolium perenne L., Zea mays L.31 and lettuce.32 The varying sensitivities of different plants to ZnO NPs necessitate precise analysis of each plant's response to ZnO NPs, enabling the development of more targeted nano fertilizers.
The molecular mechanism by which ZnO NPs affect plant growth has been studied in some plants, such as Arabidopsis thaliana, Solanum lycopersicum, and Phaseolus vulgaris. In Arabidopsis, ZnO NPs promote plant growth by affecting the synthesis of endogenous hormones and signal transduction.33 In Solanum lycopersicum, ZnO NPs affect plant growth by influencing the expression of genes related to cell division, cell wall synthesis, and other growth-related genes.34 In Phaseolus vulgaris, ZnO NPs influence plant development by modulating the metabolic response, inducing changes in the synthesis of stress-related proteins and secondary metabolites with antioxidant capacity.35 Nevertheless, the investigations into the molecular mechanisms by which ZnO NPs influence apple growth are notably scarce.
Apple “orin” calli grow rapidly and are easy to handle, making them widely used in experimental research, especially the study of gene expression changes in apples in response to environmental stress.36,37 This study demonstrates that ZnO NPs can efficiently promote the growth of apple calli. Transcriptome analysis revealed that treatment with ZnO NPs activated TFs containing zinc finger domains. These TFs regulated the expression of genes related to DNA replication, thereby promoting the proliferation of apple calli. This research offered empirical evidence for elucidating the impact of ZnO NPs on the proliferation of apple calli and established a theoretical basis for the utilization of nano-fertilizers in apple cultivation.
2. Materials and methods
2.1. Plant materials and treatment
In this study, calli from ‘orin’ apple (Malus domestica) were used as the material for the cell experiments. Calli were cultured in an MS medium (6-BA 200 mg L−1, 2,4-D 1500 mg L−1, pH 5.9–6.0) in an incubator under dark conditions (25 °C) for 11 to 14 days.
In this study, initially, young calli were transferred to an MS liquid medium (30 g L−1, 6-BA 200 mg L−1, 2,4-D 1500 mg L−1, pH 5.9–6.0) and cultured in a light-protected constant temperature shaker (110 rpm, 25 °C) for later use. All callus suspensions were thoroughly mixed and randomly divided into four equal parts, namely Control Check (CK) and ZnO NP treatment groups. The initial absorbance value of the callus suspension was maintained at no less than 0.4 at a wavelength of 600 nm (OD 600 nm). The ZnO NP treatment group was divided into three concentration gradients; the three concentrations are 200 ppm, 500 ppm, and 1000 ppm. After 0 h, 1 h, 3 h, 6 h, 9 h, 12 h, 24 h, 36 h, and 48 h of treatment, samples were collected from both the CK and ZnO NP treatment groups for various biochemical index measurements. ZnO NPs with a particle size of 20 nm were selected in this study (Shanghai Pantian Power Meterial Co., Ltd.).
2.2. Cell microstructure analysis and zinc content determination
The apple calli were treated with zinc particles with different concentrations (50 ppm ZnSO4/5 h, 500 ppm ZnO NPs/16 h, 500 ppm ZnO BPs/16 h), the particle size of ZnO NPs is 20 nm, and the particle size of ZnO BPs is 1 μm. The treatment groups and CK were separately centrifuged at 1968 × g for 10 minutes. After centrifugation, the supernatant was discarded, and pellets were resuspended in sterile water. This washing procedure was repeated three times to remove the unabsorded ZnO NPs. Following washing, 2.5% glutaraldehyde was added along the tube wall and fixed at 4 °C. The samples fixed with glutaraldehyde were embedded in epoxy resin, and ultrathin sections were cut using an ultramicrotome. After staining, TEM observation and photography could be conducted.38 Additionally, the samples fixed with glutaraldehyde underwent gradient dehydration and were fixed onto silicon wafers to dry, and after surface gold plating treatment, SEM observation and photography could be performed.
Measurement of zinc content in apple callus cells was performed. First, the experimental materials were subjected to vacuum freeze-drying treatment. The lyophilized samples were then weighed and recorded to control their weight at around 20–30 mg. Each sample was assigned a number and treated with 1 mL HNO3 and 200 μL H2O2. They were heated at 160 °C for 2 h, followed by cooling for 1.5 h. The processed solutions were collected and made up to a final volume of 10 mL with ultrapure water. These solutions were stored at 4 °C and analyzed using IPC-OES.
2.3. Growth situation measurement
The growth curves of apple callus cells were plotted by spectrophotometry. The absorbance values were measured at 0 h, 12 h, 24 h, 36 h, 48 h, and 60 h after the addition of ZnO NPs, respectively. The apple callus cell suspensions were harvested, and the OD600 was measured. Each experiment was repeated 3 times, and the data are presented as means with error bars indicating the standard deviation.
All statistical analyses were performed using SPSS 16.0 (IBM, SPSS, Chicago, IL). Figures were made using GraphPad Prism 8.0.2 (GraphPad Software Inc., San Diego, CA, USA). Nonlinear regression analysis was fitted using a logistic model (y = A/(1 + B × EXP(−K × time)), where A, B, and K are undetermined parameters, time is the independent variable, and EXP represents the exponential function with the natural logarithm base. The growth curve equation, R2, and the inflection point (ln
B/K) for each concentration was calculated.
2.4. MDA content, superoxide dismutase (SOD) and peroxidase (POD) activity measurement
To determine the MDA content in apple callus cells using the TBA method, the Grace Biotechnology MDA assay kit (G0110W) was employed, with the procedure from the provided manual followed and adapted as necessary. Briefly, approximately 0.1 g of calli were homogenized in 1 mL of extraction solution on ice. After centrifugation at 13
777 × g for 10 minutes at 4 °C, the supernatant was collected and kept on ice for subsequent analysis. For the assay, the spectrophotometer was preheated for 30 minutes, and a water bath was heated to 90–95 °C. In an Eppendorf tube, 300 μL of working solution and 200 μL of the prepared sample were mixed, then incubated in the water bath at 90–95 °C for 30 minutes. The mixture was cooled on ice and centrifuged at 13
777 × g for 10 minutes at room temperature. A 200 μL aliquot of the supernatant was transferred to a 96-well plate, and the absorbance (A) was measured at 532 nm and 600 nm. The difference in absorbance (ΔA = A532 − A600) was used to calculate the MDA content according to the formula provided in the manual.
The SOD activity was measured with the Grace Biotechnology assay kit (catalog no. G0104W). Callus samples were homogenized in the provided extraction solution, followed by centrifugation to isolate the supernatant. The assay procedure involved incubating the supernatant with kit reagents at 25 °C and measuring the absorbance at 560 nm. The inhibition rate was used to calculate the SOD activity in the samples.
The peroxidase (POD) activity was determined using the Grace Biotechnology assay kit (catalog no. G0107W). Callus samples were processed to extract the supernatant, which was then mixed with the reagents provided in the kit. The initial absorbance (A1) was measured immediately after mixing at 470 nm, followed by a subsequent absorbance (A2) measured one minute later. The difference in absorbance (ΔA = A2 − A1) over this one-minute interval was utilized to calculate the POD activity.
2.5. RNA-seq
Total RNA was isolated utilizing a Trizol reagent kit from Invitrogen (Carlsbad, CA, USA), following the protocol provided by the manufacturer. mRNA sequencing libraries for six distinct calli were assembled on an Illumina HiSeq platform by Gene Denov O Biotechnology Co. (Guangzhou, China). The sequencing reads were aligned to the apple reference genome (GDDH13_1-1; accessible at https://Rosaceae.org) using the HISAT2 aligner39 (version 2.1.0). The reads from each sample were then assembled in a reference-guided manner with StringTie software v1.3.1. Expression levels and variations for each transcriptional unit were quantified through the calculation of FPKM values using RSEM software.40 Each group was replicated three times.
2.6. Principal component analysis (PCA)
In this study, PCA was conducted using the R package gmodels, available at https://R-project.org. PCA is a statistical method that transforms a large set of interrelated variables, such as gene expressions, into a new set of orthogonal variables known as principal components. This technique is widely applied to uncover the underlying structure and relationships among samples or datasets.
2.7. Differential gene expression and enrichment analysis
The analysis of differential gene expression was performed using DESeq2 software41 to compare two distinct groups, with edgeR also being utilized for comparisons between individual samples. Genes or transcripts with a false discovery rate (FDR) below 0.05 and an absolute fold change of at least 2 were identified as differentially expressed. The biological functions of these differentially expressed genes (DEGs) were further explored through KEGG enrichment analysis, conducted via a pipeline on the Omicshare Bioinformatics Cloud Platform provided by GENEDENOVO (Guangzhou, China).
2.8. Quantitative real-time polymerase chain reaction
The extraction of total RNA from plant calli was conducted using a specialized RNA extraction kit provided by Coolaber (originating from Beijing, China). The process of reverse transcription was facilitated by employing the TransScript RT kit, which is also a product of TransGen, based in Beijing, China. The quantitative real-time polymerase chain reaction (qRT-PCR) was performed following the previously outlined protocol. In this analysis, the gene MdUBQ served as a consistent internal control, and each gene was examined in triplicate to ensure reliability. A comprehensive list of the primers utilized in the qRT-PCR analysis can be found in the ESI, specifically in Table S1.†
2.9. Analysis of the downstream regulator of the gene
Using the PlantTFDB website42 (https://planttfdb.gao-lab.org/), an analysis of MdCDF2 was performed to identify its binding sites (AAAAAG) with downstream target genes.43 Subsequently, an analysis of the promoter regions of the downstream genes was carried out using the MEME website (https://meme-suite.org).44
2.10. DNA pull-down assay
The pull-down assay was conducted using Streptavidin (SAV) Magnetic Beads (MagBeads) procured from Yeasen Biotechnology (Shanghai) Co., Ltd. These beads are specifically designed for the capture of biotinylated targets due to their high affinity for biotin, a property that was exploited in our experiments. The biotin-labeled DNA probes were synthesized by incorporating biotin at a specific site on the DNA sequence, allowing for selective binding to the SAV MagBeads.
2.11. Protein digestion and LC-MS/MS analysis
Clearing gel pieces is initiated by using a solution of ammonium bicarbonate and acetonitrile, which is then followed by dehydration using pure acetonitrile. The gel is then treated with dithiothreitol to break disulfide bonds and iodoacetamide to prevent them from reforming. After washing and dehydrating, the gel is rehydrated with trypsin for overnight digestion at 37 °C. The peptides produced are extracted and prepared for LC-MS/MS analysis.
For LC-MS/MS analysis, peptides are loaded onto a reversed-phase column and separated using a gradient of solvents. The process involves a gradual increase in the percentage of solvent B, which is acetonitrile with formic acid, over a set time period. This is done at a constant flow rate on an EASY-nLC 1000 UPLC system to ensure accurate peptide separation and analysis.
2.12. Statistical analysis
For these assays, the mean is the mean of three replicates, and error bars indicate the standard error of the mean. All data were analyzed using one-way ANOVA in GraphPad Prism (GraphPad Prism 8.0.2) to determine the statistical significance of each parameter between groups. Values following different letters were significantly different at p < 0.05. Additionally, tools used for drawing related images include TBtools45 and an online Venn diagram drawing website (https://bioinformatics.psb.ugent.be/webtools/Venn/).
3. Results and discussion
3.1. ZnO NPs promote apple callus growth
In plant growth research, the determination of growth curves is a fundamental and important tool. It not only helps researchers monitor and quantify the growth rate and stages of plant growth, but also reveals the plant's response to environmental changes and external stress factors.46 Here, the growth curve assay showed that treatments with 200 ppm, 500 ppm and 1000 ppm ZnO NPs all promote the growth of apple callus cells compared to the CK. 500 ppm ZnO NPs most effectively promote the growth of apple callus cells (Fig. 1A). The OD values of the 500 ppm ZnO NP treatment group are higher than those of other groups. By fitting growth curve equations, the exponential growth onset time points for the groups at 200 ppm, 500 ppm, and 1000 ppm are 23.6 h, 16.14 h, and 21.25 h (Table 1). This result indicated that the 500 ppm ZnO NP treatment group enters the exponential growth phase earlier than the other two groups. Therefore, it is speculated that treatment with 500 ppm ZnO NPs has the most significant promoting effect on apple callus growth.
 |
| Fig. 1 The growth curves and physiology experiment of apple callus cells following treatment with varying concentrations of ZnO NPs. (A) Growth curves of apple callus cells treated with different concentrations of ZnO NPs for 24 h. (B–D) The content of MDA and the activity of SOD and POD in apple callus cells treated with different concentrations of ZnO NPs for 24 h. Error bars represent the standard error of the mean (n = 3), and ‘*’ indicates p < 0.05. | |
Table 1 Nonlinear regression analysis of the growth curves of the apple callus cells with varying concentrations of ZnO NPs
Concentration |
Formula |
R
2
|
ln B/k |
200 ppm |
Y = 1.781/(1 + 3.494 × 10−0.053t) |
0.996 |
23.60 |
500 ppm |
Y = 1.552/(1 + 2.633 × 10−0.06t) |
0.972 |
16.14 |
1000 ppm |
Y = 1.751/(1 + 2.893 × 10−0.05t) |
0.988 |
21.25 |
The concentration of MDA in cells can indicate the cellular oxidative stress response.47 In this study, compared to the CK, the MDA content in ZnO NP treatment groups was significantly reduced. The MDA content exhibited a trend of initial decrease followed by an increase, with the greatest reduction observed in the group treated with 500 ppm. After treatment with 500 ppm ZnO NPs for 12 hours, the MDA content in apple callus cells decreased by 46.7%. At 16 h, when the apple callus cells entered the exponential growth phase, the MDA content in the 500 ppm treatment group remained lower than that in the other concentration groups, and was 22% lower than that in CK (Fig. 1B). In Coriandrum sativum L., foliar application of 400 ppm ZnO NPs led to a 72% reduction in MDA content, likely due to the enhancement of antioxidant enzyme activity, which helps alleviate oxidative stress.48 Similarly, in Mentha piperita L. grown in cadmium-contaminated soil, the application of TiO2 NPs also reduced MDA levels, indicating that nanoparticles may mitigate oxidative stress by boosting the plant's antioxidant defense mechanisms.49 These findings suggest that nanoparticle treatments may play a crucial role in enhancing plant resilience to oxidative damage across different species.
The antioxidant status of the cells was monitored by assessing the activities of SOD and POD enzymes.50,51 In this study, compared to the CK, the SOD and POD activities in all treatment groups significantly increased. The overall trend showed an initial increase followed by a decrease. The SOD activity showed no significant differences among the treatment groups with varying concentrations, reaching its peak between 12 and 16 h. At 16 h, when the apple callus cells in the 500 ppm treatment entered the exponential growth phase, SOD activity increased by 66.5% compared to the CK group. The POD activity peaked at 12 h and then declined. At 12 h, the POD activity in all treatment groups was very similar, reaching 142% of the CK group. At 16 h, during the exponential growth phase, the POD activity in all treatment groups decreased but remained significantly higher than the CK, with an increase of 35.6% compared to CK (Fig. 1C and D). In a study investigating the effects of ZnO NPs on Hibiscus syriacus, treatments with 30 ppm and 50 ppm ZnO NPs increased the activities of SOD and POD in the petals, suggesting that ZnO NPs can enhance antioxidant efficiency and the antioxidant capacity of the flowers.52 Based on the above studies and results, it can be inferred that ZnO NPs may reduce oxidative stress by strengthening the antioxidant defense mechanism.
3.2. ZnO NPs efficiently enter the nucleus and cytoplasm
To further investigate the efficiency of ZnO NPs, apple callus cells were treated with zinc particles with different concentrations (50 ppm ZnSO4/5 h, 500 ppm ZnO NPs/16 h, 500 ppm ZnO BPs/16 h). IPC-OES experimental results showed that the zinc content in the ZnO NP treated group was almost 100 times that in the other groups (Fig. 2A). The SEM results showed that there were no significant changes observed on the surface of cells between ZnO NP treated apple calli and CK (Fig. 2B, a and b). However, there was substantial particle accumulation on the cell surface after ZnO BP treatment (Fig. S1†). The diameter of plant cell wall pores is generally 5–20 nm, allowing ZnO NPs, with a diameter of 20 nm, to pass through. In contrast, ZnO BPs, with a diameter larger than that of the cell wall pores, cannot penetrate.53 Compared to the ZnO BPs, ZnO NPs have a larger specific surface area, which increases their contact area with the cell membrane, potentially enhancing the cellular uptake of more particles.54 These features may also increase their interaction with the cell membrane, thereby facilitating their internalization through mechanisms such as endocytosis.55 Additionally, Zn2+ ions need to bind to transport proteins such as ZIP family transporters or natural resistance associated macrophage proteins56 to enter plant cells. This leads to the rate at which Zn2+ ions enter plant cells being affected by the quantity and transport efficiency of transport proteins on the cell membrane. Therefore, ZnO NPs can more readily enter apple callus cells than ZnO BPs and Zn2+ ions.
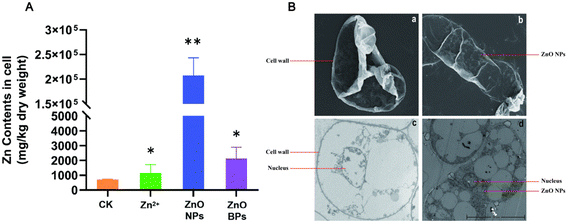 |
| Fig. 2 Zinc elemental content and subcellular localization in apple callus cells. (A) Zinc elemental content in apple callus cells of the treatment group with particles of varying sizes. (B) SEM images of apple callus cells treated with the CK (a) and 500 ppm ZnO NPs (b). TEM images of apple callus cells treated with the CK (c) and 500 ppm ZnO NPs (d). ‘*’ indicates p < 0.05, ‘**’ indicates p < 0.01. | |
A previous study has demonstrated that the surface charge of nanoparticles or the released Zn2+ ions may interact with DNA or nuclear proteins, further facilitating their migration into the nucleus.57 Here, the TEM results showed that ZnO NPs accumulated significantly in apple callus cells, primarily localized in the cytoplasm and nucleus (Fig. 2B, c and d). These findings indicate that ZnO NPs can penetrate apple callus cells and progressively migrate toward the nucleus, ultimately exerting their effects in both the cytoplasm and nucleus.
3.3. ZnO NPs affect the gene expression of apple
To further investigate the mechanism by which ZnO NPs promote the growth of apple calli, transcriptome analysis was performed on calli treated with 500 ppm ZnO NPs for 16 h. The Q score (quality score) is an indication of the overall base call accuracy. For base quality analysis, the Q20 score reached over 97%, and the Q30 score was around 92%. Compared to the reference genome, about 95% of the sequences could be mapped, and the GC content was almost consistent between treatment groups. These results indicate that the transcriptome data obtained from the apple calli treated with ZnO NPs are of high quality and reliable for further analysis of the underlying mechanisms promoting growth (Table S2†). In the PCA analysis, it was found that the gene differences within the groups were minimal, while the gene differences between the groups were substantial. This indicates that under the same treatment conditions, the gene expression among samples exhibits similarity. Transcriptome analysis revealed a total of 20
802 common genes detected in both the ZnO NP treatment group and the CK group, with 1487 unique genes in the CK group and 811 unique genes in the ZnO NP treatment group (Fig. 3B, Table S3†). Compared with the CK group, 7450 genes were significantly up-regulated and 2972 genes were down-regulated in the ZnO NP treatment group (Fig. 3C, Table S4†). Subsequently, GO analysis and KEGG enrichment analysis were performed on these differentially expressed genes (DEGs).
 |
| Fig. 3 Transcriptome analysis of the CK and 500 ppm ZnO NP treatment group. (A) PCA analysis of CK and ZnO NP treatment groups. (B) Venn plot of the number of DEGs between CK and ZnO NP treatment groups. (C) Volcano plot of up-regulated and down-regulated gene expression in the ZnO NP treatment group. (D) Top 20 bubble plot of KEGG enrichment analysis for DEGs. (E) Bar plot of GO Analysis for DEGs. | |
GO analysis categorizes and describes gene functions, allowing researchers to assign differentially expressed genes to predefined categories and thereby understand their roles and the biological processes they are involved in within the cell.58 The results of GO enrichment analysis indicated that most DEGs were distributed among the three main GO categories: cellular component (CC), biological process (BP), and molecular function (MF). After ZnO NP treatment, most DEGs were found in the BP and CC categories. GO analysis showed that within the biological process category, DEGs were mainly enriched in metabolic processes, cellular processes, and single-organism processes. Within the cellular component category, DEGs were primarily enriched in the cell, cell part, and membrane. In the molecular function category, DEGs were mainly enriched in catalytic activity, binding, and transporter activity (Fig. 3E).
KEGG analysis focuses on the roles of genes in biological metabolic pathways and signal transduction pathways.59 KEGG pathway analysis identified 137 distinct pathways, and the top 20 pathways were analyzed. These DEGs were predominantly enriched in metabolic pathways, biosynthesis of secondary metabolites, fatty acid biosynthesis, one-carbon pool by folate, and DNA replication pathways (Fig. 3D). Zinc is an important signaling molecule that acts as a cofactor in various enzymes, particularly DNA-binding proteins, and plays roles in maintaining genetic stability, regulating transcription and translation, and promoting cell differentiation and proliferation.60,61 Therefore, we speculate that ZnO NPs may affect cell growth by influencing the expression of genes related to DNA replication.
Multiple studies have demonstrated that ZnO NPs are involved in regulating the expression of TFs in plants. For instance, in Solanum lycopersicum, ZnO NP treatment up-regulated the expression of several TFs, including MKK2, bHLH, WRKY1, EREB, HsfA1a, and R2R3MYB. Similarly, in Oryza sativa L., ZnO NP treatment significantly up-regulated the expression of TFs that regulate responses to cold stress, thereby enhancing rice's resistance to low-temperature stress.62,63 In this study, we found 584 TFs in the DEGs, including 202 TFs containing zinc finger motifs (Table S5†).
We found a gene, MdCDF2, which was significantly up-regulated under ZnO NP treatment, encoding a type of zinc finger-containing TF (Fig. 5A). MdCDF2 contains a conserved Dof (DNA binding with one finger) domain at its N-terminus, which comprises a single C2C2-type zinc finger structure. The CDF (cycling of Dof factor) proteins, part of the Dof family, contain three conserved sequence elements in their C-terminal region. These CDF proteins can participate in protein–protein interactions and bind to plant-specific promoters to regulate gene expression.64 The CDF family has been shown to play critical roles in plant responses to environmental stimuli, including regulating flowering in response to the photoperiod and increasing tolerance to abiotic stresses. In Arabidopsis and Solanum lycopersicum, overexpression of the CDF3 gene has been reported to enhance biomass and regulate genes related to plant growth.65
3.4.
MdCDF2 binding to the promoter regions of several genes associated with DNA replication
The CDF proteins specifically bind to the AAAAAG sequence.43 The promoter analysis showed that MdCDF2 might interact with the promoters of three DEGs related to DNA replication (MdClpB1, MD01G1182200, and MD12G1082300) (Fig. 4A). The transcriptome data and qRT-PCR results showed that after ZnO NP treatment, the expression levels of MdCDF2 and MdClpB1 increased by 2-fold and 8-fold, respectively, while the expression levels of MD01G1182200 and MD12G1082300 significantly decreased (Fig. 4B). Based on GO enrichment analysis, MdClpB1, MD01G1182200, and MD12G1082300 are implicated in DNA replication processes. Although detailed functional annotations for these genes are not available in the NCBI, their enrichment in DNA replication pathways suggests that they may play roles in key processes such as DNA strand synthesis, replication initiation, or the stabilization of replication machinery. Further experimental validation is required to confirm their precise roles in promoting DNA replication in apple calli.
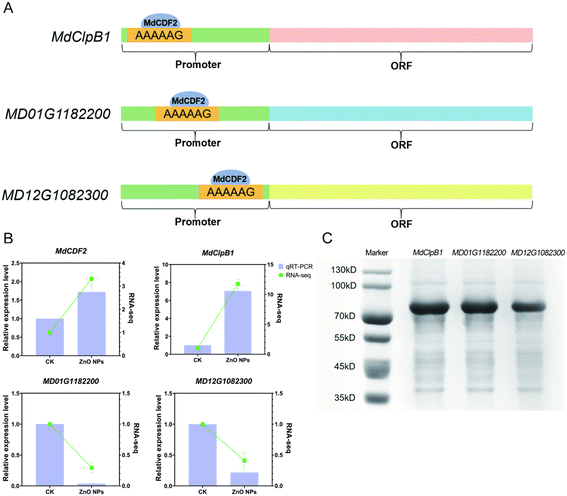 |
| Fig. 4
MdCDF2 regulates gene expression by binding to gene promoter regions. (A) A schematic diagram illustrating MdCDF2 binding to the promoter regions of several genes associated with DNA replication. (B) Relative expression levels of qRT-PCR and RNA-seq. Error bars indicate ±S.D. of biological triplicates. (C) DNA-pull down validation of MdCDF2 binding to MdClpB1, MD01G1182200 and MD12G1082300 promoter regions. | |
To verify whether MdCDF2 can bind to the promoter regions of MdClpB1, MD01G1182200, and MD12G1082300, a DNA pull-down assay was performed. Streptavidin-coated magnetic beads were used to specifically bind the biotin-labeled DNA probe, followed by incubation with MdCDF2 proteins and then used mass spectrometry analysis. As shown in Fig. 4C, a distinct band was observed at a molecular weight of approximately 70–80 kD, consistent with the expected size. Mass spectrometry analysis further corroborated that the protein in the distinct band is MdCDF2 (Table S6†). These results suggest that MdCDF2 can bind to the promoter regions of MdClpB1, MD01G1182200 and MD12G1082300, thereby regulating gene expression and subsequently influencing DNA replication and cell growth.
4. Conclusion
This study has determined that ZnO NPs with a diameter of 20 nm can efficiently enter apple callus cells and promote cell growth. After entering the cells, ZnO NPs accumulate extensively in the cytoplasm and nucleus. Upon migrating into the nucleus, ZnO NPs may influence transcriptional regulation by upregulating the expression of the transcription factor MdCDF2. MdCDF2, in turn, promotes the expression of MdClpB1 while inhibiting MD01G1182200 and MD12G1082300, enhancing DNA replication and accelerating cell proliferation in apple callus cells. In addition, ZnO NPs improve the antioxidant capacity of the cells by reducing the MDA content and increasing the activity of SOD and POD, ultimately promoting the growth of apple callus cells (Fig. 5).
 |
| Fig. 5 The molecular mechanism diagram of ZnO NPs promoting the growth of apple callus cells. | |
While the study provides compelling evidence for the regulatory role of MdCDF2, it primarily focuses on promoter binding and expression analysis. Functional assays, such as gene knockout or overexpression studies, could further validate the causal relationships between MdCDF2 activity and the observed phenotypic outcomes. Despite these limitations, this study holds significant importance. It not only advances our understanding of how MdCDF2 regulates gene expression related to DNA replication and cell growth, but also provides a potential molecular target for enhancing plant growth and stress resistance. The findings from this study could have far-reaching applications in improving the yield and resilience of economically important crops, opening new avenues for agricultural biotechnology.
Data availability
The datasets generated and/or analyzed during the current study are available from the corresponding author upon reasonable request. The sequencing data have been deposited in the NCBI Sequence Read Archive (SRA) under accession number NCBI SRA: SUB14689169.
Author contributions
Yuxiao Yi: investigation, validation, visualization, and writing – original draft. Xiaowei Li: resources and investigation. Qing Wang: investigation, validation, and data curation. Tongtong Guo: validation and data curation. Changjian Xie: methodology, resources, supervision, and validation. Fengtang Yang: supervision conceptualization, and writing – review & editing. Jianing Xu: methodology, funding acquisition, conceptualization, and writing – review & editing.
Conflicts of interest
The authors declare that they have no known competing financial interests or personal relationships that could have appeared to influence the work reported in this paper.
Acknowledgements
This work was supported by the National Natural Science Foundation of China (Grant No. 12105163, 32001987) and the Natural Science Foundation of Shandong Province (Grant No. ZR2020QD133, ZR2020MC142). We are grateful to Guangzhou Genedenovo Biotechnology Co., Ltd. for assisting in sequencing and bioinformatics analysis.
References
- A. Cornille, F. Antolín, E. Garcia, C. Vernesi, A. Fietta, O. Brinkkemper, W. Kirleis, A. Schlumbaum and I. Roldán-Ruiz, A multifaceted overview of apple tree domestication, Trends Plant Sci., 2019, 24, 770–782 CrossRef CAS PubMed.
- G. Piva, V. Farina, G. Sortino, G. Gianguzzi and G. Liguori, Effects of zinc foliar nutrition on ‘gala’ apple (Malus domestica borkh) fruit quality, Bulg. J. Agric. Sci., 2017, 23, 213–218 Search PubMed.
- D. A. Hyson, A comprehensive review of apples and apple components and their relationship to human health, Adv. Nutr., 2011, 2, 408–420 CrossRef CAS PubMed.
- A. Koutsos, K. M. Tuohy and J. A. Lovegrove, Apples and cardiovascular health—is the gut microbiota a core consideration?, Nutrients, 2015, 7, 3959–3998 CrossRef CAS PubMed.
- J. Boyer and R. H. Liu, Apple phytochemicals and their health benefits, Nutr. J., 2004, 3, 5 CrossRef PubMed.
- Y. Sun, Y. Lu, Z. Wang and M. Li, Production efficiency and change characteristics of China's apple industry in terms of planting scale, PLoS One, 2021, 16, e0254820 CrossRef CAS PubMed.
- H. J. Zhou, R. F. Korcak and M. Faust, Development of Fe chlorosis and nutrition of apple seedlings under deficient Fe, Mn and Zn supply, Sci. Hortic., 1985, 27, 233–240 CrossRef CAS.
- M. R. Broadley, P. J. White, J. P. Hammond, I. Zelko and A. Lux, Zinc in plants, New Phytol., 2007, 173, 677–702 CrossRef CAS PubMed.
- H. Motte, S. Vanneste and T. Beeckman, Molecular and environmental regulation of root development, Annu. Rev. Plant Biol., 2019, 70, 465–488 CrossRef CAS PubMed.
- A. Jalal, E. F. Júnior and M. C. M. Teixeira Filho, Interaction of zinc mineral nutrition and plant growth-promoting bacteria in tropical agricultural systems: A review, Plants, 2024, 13 Search PubMed.
- R. Hajiboland and H. Amirazad, Drought tolerance in zn-deficient red cabbage (Brassica oleracea l. Var. Capitata f. Rubra) plants, Hortic. Sci., 2010, 37 Search PubMed.
- F. S. Sadoogh, H. Shariatmadari, A. H. Khoshgoftarmanesh and M. R. Mosaddeghi, Adjusted nutrition of tomato with potassium and zinc in drought stress conditions induced by polyethylene glycol 6000 in hydroponic culture, Journal of Science and Technology of Greenhouse Culture, 2014, 5, 67–81 Search PubMed.
- M. Eslami, H. Dehghanzadeh, M. Jafarzade and R. Aminian, The effect of zinc on yield and yield components of sunflower (Helianthus.annuus L.) under drought stress, Scientific Journal of Crop Science, 2014, 3(6), 61–65 Search PubMed.
- H. Kaur and N. Garg, Zinc toxicity in plants: A review, Planta, 2021, 253, 129 CrossRef CAS PubMed.
- C. Stanton, D. Sanders, U. Krämer and D. Podar, Zinc in plants: Integrating homeostasis and biofortification, Mol. Plant, 2022, 15, 65–85 CrossRef CAS PubMed.
- H. Balafrej, D. Bogusz, Z.-E. A. Triqui, A. Guedira, N. Bendaou, A. Smouni and M. Fahr, Zinc hyperaccumulation in plants: A review, Plants, 2020, 9, 562 CrossRef CAS PubMed.
- P. I. Michael and M. Krishnaswamy, The effect of zinc stress combined with high irradiance stress on membrane damage and antioxidative response in bean seedlings, Environ. Exp. Bot., 2011, 74, 171–177 CrossRef CAS.
- M. Shen, W. Liu, A. Zeb, J. Lian, J. Wu and M. Lin, Bioaccumulation and phytotoxicity of ZnO nanoparticles in soil-grown Brassica chinensis l. And potential risks, J. Environ. Manage., 2022, 306, 114454 CrossRef CAS PubMed.
- N. Garg and H. Kaur, Influence of zinc on cadmium-induced toxicity in nodules of pigeonpea (Cajanus cajan l. Millsp.) inoculated with Arbuscular mycorrhizal (am) fungi, Acta Physiol. Plant., 2012, 34, 1363–1380 CrossRef CAS.
- N. Garg and H. Kaur, Response of antioxidant enzymes, phytochelatins and glutathione production towards Cd and Zn stresses in
Ajanus cajan (l.) millsp. Genotypes colonized by arbuscular mycorrhizal fungi, J. Agron. Crop Sci., 2013, 199, 118–133 CrossRef CAS.
- N. Garg and H. J. J. o. P. N. Kaur, Impact of cadmium-zinc interactions on metal uptake, translocation and yield in pigeonpea genotypes colonized by Arbuscular mycorrhizal fungi, J. Plant Nutr., 2013, 36, 67–90 CrossRef CAS.
- M. S. Sheteiwy, H. Shaghaleh, Y. A. Hamoud, P. Holford, H. Shao, W. Qi, M. Z. Hashmi and T. Wu, Zinc oxide nanoparticles: Potential effects on soil properties, crop production, food processing, and food quality, Environ. Sci. Pollut. Res., 2021, 28, 36942–36966 CrossRef CAS PubMed.
- A. Król, P. Pomastowski, K. Rafińska, V. Railean-Plugaru and B. Buszewski, Zinc oxide nanoparticles: Synthesis, antiseptic activity and toxicity mechanism, Adv. Colloid Interface Sci., 2017, 249, 37–52 CrossRef PubMed.
- L. V. Subbaiah, T. N. Prasad, T. G. Krishna, P. Sudhakar, B. R. Reddy and T. Pradeep, Novel effects of nanoparticulate delivery of zinc on growth, productivity, and zinc biofortification in maize (Zea mays l.), J. Agric. Food Chem., 2016, 64, 3778–3788 CrossRef CAS PubMed.
- M. Faizan, J. A. Bhat, C. Chen, M. N. Alyemeni, L. Wijaya, P. Ahmad and F. Yu, Zinc oxide nanoparticles (ZnO-NPs) induce salt tolerance by improving the antioxidant system and photosynthetic machinery in tomato, Plant Physiol. Biochem., 2021, 161, 122–130 CrossRef CAS PubMed.
- X. Xu, C. Zhao, K. Qian, M. Sun, Y. Hao, L. Han, C. Wang, C. Ma, J. C. White and B. Xing, Physiological responses of pumpkin to zinc oxide quantum dots and nanoparticles, Environ. Pollut., 2022, 296, 118723 CrossRef CAS PubMed.
- Y. Wu, Y. Wang, X. Liu and C. Zhang, Unveiling key mechanisms: Transcriptomic meta-analysis of diverse nanomaterial applications addressing biotic and abiotic stresses in Arabidopsis thaliana, Sci. Total Environ., 2024, 928, 172476 CrossRef CAS PubMed.
- Z. Wang, C. Tang, X. Mi, D. Yao, Z. Chen, C. Guo, Y. Zhao, X. Xue, W. Chang and Y. Li, Zinc oxide nanoparticles alleviated vanadium-induced inhibition by regulating plant hormone signal transduction and phenylpropanoid biosynthesis in maize seedlings (Zea may l.), Environ. Technol. Innovation, 2024, 35, 103696 CrossRef CAS.
- L. Xiang, H. M. Zhao, Y. W. Li, X. P. Huang, X. L. Wu, T. Zhai, Y. Yuan, Q. Y. Cai and C. H. Mo, Effects of the size and morphology of zinc oxide nanoparticles on the germination of Chinese cabbage seeds, Environ. Sci. Pollut. Res., 2015, 22, 10452–10462 CrossRef CAS PubMed.
- D. Singh and A. Kumar, Impact of irrigation using water containing cuo and zno nanoparticles on Spinach Oleracea grown in soil media, Bull. Environ. Contam. Toxicol., 2016, 97, 548–553 CrossRef CAS PubMed.
- D. Lin and B. Xing, Phytotoxicity of nanoparticles: Inhibition of seed germination and root growth, Environ. Pollut., 2007, 150, 243–250 CrossRef CAS PubMed.
- X. Zhang, K. Yin, R. Huo, Z. Wang, S. Fan, Q. Ma, L. Wang, S. Zhai and J. Wang, Phytotoxic effects of different concentrations of zinc species on lettuce, Water, Air, Soil Pollut., 2023, 234, 569 CrossRef CAS.
- R. Vankova, P. Landa, R. Podlipna, P. I. Dobrev, S. Prerostova, L. Langhansova, A. Gaudinova, K. Motkova, V. Knirsch and T. Vanek, Zno nanoparticle effects on hormonal pools in Arabidopsis thaliana, Sci. Total Environ., 2017, 593–594, 535–542 CrossRef CAS PubMed.
- L. Sun, Y. Wang, R. Wang, R. Wang, P. Zhang, Q. Ju and J. Xu, Physiological, transcriptomic, and metabolomic analyses reveal zinc oxide nanoparticles modulate plant growth in tomato, Environ. Sci.: Nano, 2020, 7, 3587–3604 RSC.
- H. Salehi, N. De Diego, A. Chehregani Rad, J. J. Benjamin, M. Trevisan and L. Lucini, Exogenous application of ZnO nanoparticles and ZnSO4 distinctly influence the metabolic response in Phaseolus vulgaris l, Sci. Total Environ., 2021, 778 Search PubMed.
- H. Kang, T. T. Zhang, L. L. Fu, Y. X. Yao, C. X. You, X. F. Wang and Y. J. Hao, The apple MdCOP1-interacting protein 1 negatively regulates hypocotyl elongation and anthocyanin biosynthesis, BMC Plant Biol., 2021, 21, 15 CrossRef CAS PubMed.
- N. Wang, C. Qu, Y. Wang, H. Xu, S. Jiang, H. Fang, J. Liu, Z. Zhang and X. Chen, MdMYB4 enhances apple callus salt tolerance by increasing MdNHX1 expression levels, Plant Cell, Tissue Organ Cult., 2017, 131, 283–293 CrossRef CAS.
- Z. Yang, C. Deng, Y. Wu, Z. Dai, Q. Tang, C. Cheng, Y. Xu, R. Hu, C. Liu, X. Chen, X. Zhang, A. Li, X. Xiong, J. Su and A. Yan, Insights into the mechanism of multi-walled carbon nanotubes phytotoxicity in Arabidopsis through transcriptome and m6A methylome analysis, Sci. Total Environ., 2021, 787, 147510 CrossRef CAS PubMed.
- D. Kim, B. Langmead and S. L. Salzberg, Hisat: A fast spliced aligner with low memory requirements, Nat. Methods, 2015, 12, 357–360 CrossRef CAS PubMed.
- B. Li and C. N. Dewey, Rsem: Accurate transcript quantification from RNA-seq data with or without a reference genome, BMC Bioinf., 2011, 12, 323 CrossRef CAS PubMed.
- M. I. Love, W. Huber and S. Anders, Moderated estimation of fold change and dispersion for RNA-seq data with deseq2, Genome Biol., 2014, 15, 550 CrossRef PubMed.
- F. Tian, D.-C. Yang, Y.-Q. Meng, J. Jin and G. Gao, Plantregmap: Charting functional regulatory maps in plants, Nucleic Acids Res., 2020, 48, D1104–D1113 CAS.
- S. Yanagisawa and R. J. Schmidt, Diversity and similarity among recognition sequences of dof transcription factors, Plant J, 1999, 17, 209–214 CrossRef CAS PubMed.
- T. L. Bailey, J. Johnson, C. E. Grant and W. S. Noble, The meme suite, Nucleic Acids Res., 2015, 43, W39–W49 CrossRef CAS PubMed.
- C. Chen, Y. Wu, J. Li, X. Wang, Z. Zeng, J. Xu, Y. Liu, J. Feng, H. Chen, Y. He and R. Xia, Tbtools-II: A “one for all, all for one” bioinformatics platform for biological big-data mining, Mol. Plant, 2023, 16, 1733–1742 CrossRef CAS PubMed.
- V. R. Krishnamurthi, I. I. Niyonshuti, J. Chen and Y. Wang, A new analysis method for evaluating bacterial growth with microplate readers, PLoS One, 2021, 16, e0245205 CrossRef CAS PubMed.
- M. W. Davey, E. Stals, B. Panis, J. Keulemans and R. L. Swennen, High-throughput determination of malondialdehyde in plant tissues, Anal. Biochem., 2005, 347, 201–207 CrossRef CAS PubMed.
- V. L. Reddy Pullagurala, I. O. Adisa, S. Rawat, S. Kalagara, J. A. Hernandez-Viezcas, J. R. Peralta-Videa and J. L. Gardea-Torresdey, ZnO nanoparticles increase photosynthetic pigments and decrease lipid peroxidation in soil grown cilantro (Coriandrum sativum), Plant Physiol. Biochem., 2018, 132, 120–127 CrossRef CAS PubMed.
- H. Mohammadi, Z. Kazemi, A. Aghaee, S. Hazrati, R. Golzari Dehno and M. Ghorbanpour, Unraveling the influence of TiO(2) nanoparticles on growth, physiological and phytochemical characteristics of Mentha piperita l. In cadmium-contaminated soil, Sci. Rep., 2023, 13, 22280 CrossRef CAS PubMed.
- C. J. Weydert and J. J. Cullen, Measurement of superoxide dismutase, catalase and glutathione peroxidase in cultured cells and tissue, Nat. Protoc., 2010, 5, 51–66 CrossRef CAS PubMed.
- H. B. Dunford and J. S. Stillman, On the function and mechanism of action of peroxidases, Coord. Chem. Rev., 1976, 19, 187–251 CrossRef CAS.
- X. Wang, J. Chen, L. Hu, J. Zhang, F. Xiao, S. Zhang, F. Shao and L. Huang, Embryological observations on seed abortion in Hibiscus syriacus l. And physiological studies on nutrients, enzyme activity and endogenous hormones, BMC Plant Biol., 2023, 23, 665 CrossRef CAS PubMed.
- F. Schwab, G. Zhai, M. Kern, A. Turner, J. L. Schnoor and M. R. Wiesner, Barriers, pathways and processes for uptake, translocation and accumulation of nanomaterials in plants-critical review, Nanotoxicology, 2016, 10, 257–278 CrossRef CAS PubMed.
- X. Huang, C. Chen, C. Yi and X. Zheng, Smart drug delivery systems based on nanoscale ZnO, Shengwu Yixue Gongchengxue Zazhi, 2018, 35, 324–328 Search PubMed.
- H. Zhang, N. S. Goh, J. W. Wang, R. L. Pinals, E. González-Grandío, G. S. Demirer, S. Butrus, S. C. Fakra, A. Del Rio Flores, R. Zhai, B. Zhao, S. J. Park and M. P. Landry, Nanoparticle cellular internalization is not required for RNA delivery to mature plant leaves, Nat. Nanotechnol., 2022, 17, 197–205 CrossRef CAS PubMed.
- W. Hong and J. I. N. Jiyun, The physiological and molecular mechanisms of zinc uptake, transport, and hyperaccumulation in plants: A review, Zhiwu Yingyang Yu Feiliao Xuebao, 2009, 15, 225–235 Search PubMed.
- M. Kang, Y. Liu, Y. Weng, H. Wang and X. Bai, A critical review on the toxicity regulation and ecological risks of zinc oxide nanoparticles on plants, Environ. Sci.: Nano, 2023, 11 Search PubMed.
- M. Ashburner, C. A. Ball, J. A. Blake, D. Botstein, H. Butler, J. M. Cherry, A. P. Davis, K. Dolinski, S. S. Dwight, J. T. Eppig, M. A. Harris, D. P. Hill, L. Issel-Tarver, A. Kasarskis, S. Lewis, J. C. Matese, J. E. Richardson, M. Ringwald, G. M. Rubin and G. Sherlock, Gene ontology: Tool for the unification of biology. The gene ontology consortium, Nat. Genet., 2000, 25, 25–29 CrossRef CAS PubMed.
- M. Kanehisa and S. Goto, Kegg: Kyoto encyclopedia of genes and genomes, Nucleic Acids Res., 2000, 28, 27–30 CrossRef CAS PubMed.
-
A. Iranbakhsh, Z. Oraghi Ardebili and N. Oraghi Ardebili, in Plant responses to nanomaterials: Recent interventions, and physiological and biochemical responses, ed. V. P. Singh, S. Singh, D. K. Tripathi, S. M. Prasad and D. K. Chauhan, Springer International Publishing, Cham, 2021, pp. 33–93, DOI:10.1007/978-3-030-36740-4_3.
- W. Maret, Zinc in cellular regulation: The nature and significance of “zinc signals”, Int. J. Mol. Sci., 2017, 18, 2285 CrossRef PubMed.
- F. Pejam, Z. O. Ardebili, A. Ladan-Moghadam and E. Danaee, Zinc oxide nanoparticles mediated substantial physiological and molecular changes in tomato, PLoS One, 2021, 16, e0248778 CrossRef CAS PubMed.
- Y. Song, M. Jiang, H. Zhang and R. Li, Zinc oxide nanoparticles alleviate chilling stress in rice (Oryza sativa l.) by regulating antioxidative system and chilling response transcription factors, Molecules, 2021, 26 CAS.
- A. R. Corrales, L. Carrillo, P. Lasierra, S. G. Nebauer, J. Dominguez-Figueroa, B. Renau-Morata, S. Pollmann, A. Granell, R. V. Molina, J. Vicente-Carbajosa and J. Medina, Multifaceted role of cycling dof factor 3 (CDF3) in the regulation of flowering time and abiotic stress responses in arabidopsis, Plant, Cell Environ., 2017, 40, 748–764 CrossRef CAS PubMed.
- B. Renau-Morata, R. V. Molina, L. Carrillo, J. Cebolla-Cornejo, M. Sánchez-Perales, S. Pollmann, J. Domínguez-Figueroa, A. R. Corrales, J. Flexas, J. Vicente-Carbajosa, J. Medina and S. G. Nebauer, Ectopic expression of CDF3 genes in tomato enhances biomass production and yield under salinity stress conditions, Front. Plant Sci., 2017, 8, 660 CrossRef PubMed.
|
This journal is © The Royal Society of Chemistry 2025 |
Click here to see how this site uses Cookies. View our privacy policy here.