DOI:
10.1039/D5QI00110B
(Review Article)
Inorg. Chem. Front., 2025,
12, 3018-3040
Structural diversity and photocurrent responses of multi-component chalcogenidometalates
Received
12th January 2025
, Accepted 4th March 2025
First published on 4th March 2025
Abstract
Multi-component chalcogenidometalates have garnered significant attention due to their promising applications in solar energy conversion devices, including photodetectors, solar cells, and photocatalysts. Photocurrent response is not only a fundamental property of photodetectors but also serves as a key indicator of the solar energy conversion efficiency in potential semiconductor devices. Despite the growing interest, a clear and universal guideline for designing chalcogenide materials with excellent photocurrent response remains elusive, primarily due to the substantial variations in their chemical compositions and crystal structures. In this review, we present a comprehensive compilation of reported multi-component chalcogenidometalates, including main group chalcogenides with binary and ternary anionic frameworks, and discuss their photocurrent response performance. Additionally, we also highlight other special chalcogenide systems, focusing on their photocurrent response characteristics. For the first time, we systematically summarize the intricate relationships between chemical composition, crystal structure, electronic band structure, and photocurrent response in these materials. Finally, we believe that this review provides a valuable structural perspective on the photocurrent response of multi-component chalcogenidometalates, offering useful insights for the design and application of advanced solar energy conversion materials.
1. Introduction
Chalcogenidometalates have gained widespread attention for their promising applications in solar energy conversion technologies due to their semiconductor properties. These materials can generally be classified into two categories based on the composition of their basic building units (BBUs). The first category includes binary chalcogenides composed of a single transition metal, such as MoQ2, WQ2, CdQ, and HgQ (where Q = S, Se, or Te). The second category encompasses compounds with main group metal atoms like Ga, In, Ge, Sn, Sb, and As, most of which coordinate with transition-metal-based (TM-based) BBUs to form binary or ternary multi-component anionic structures. The incorporation of additional structural and functional units can significantly alter the electronic structures and physical properties of these materials, distinguishing them from the original binary anion compounds. By selecting appropriate structural or functional units, it is possible to design multifunctional materials tailored to specific applications. In general, multi-component chalcogenidometalates are composed of organic or inorganic charge-balancing cations and metal-containing anion skeletons, which exhibit a wide range of network topologies. These include zero-dimensional (0D) clusters, one-dimensional (1D) chains, two-dimensional (2D) layers, and three-dimensional (3D) frameworks. The diversity in their structures and compositions has led to extensive exploration of their potential in various fields, such as nonlinear optics,1 thermoelectrics,2 superconductors,3 magnetism,4 photocatalysis,5 ion exchange,6 and photoelectric devices.7
A wide range of materials have been investigated for solar energy conversion, including silicon/carbon-based compounds, oxides, and simple chalcogenides, with optical band gaps ranging from 1.23 to 3.1 eV. Silicon-based solar cells, as first-generation materials, typically demonstrate relatively high conversion efficiency, with a photocurrent density around 35 μA cm−2.8 However, since silicon naturally occurs as SiO2 in sand and gravel, its purification requires significant energy input, and the yield is low. Additionally, converting silicon ingots into wafers is a challenging process. As a result, finding alternative solar cell materials could significantly reduce costs. Most metal oxides, such as TiO2 (3.0 eV),9 ZnO (3.2 eV),10 and SrTiO3 (3.25 eV),11 have wide band gaps, limiting their absorption to ultraviolet light only. For example, TiO2 nanowire arrays produce a photocurrent density of 3 μA cm−2, which can increase to 0.76 mA cm−2 through carbon/nitrogen co-implantation.12 Perovskite solar cells typically exhibit a photocurrent density of around 20 μA cm−2.13 In contrast, chalcogenides, with their narrower band gaps, are more effective at absorbing visible light and have been extensively explored for solar energy conversion. Notable chalcogenide materials include CdTe and CuInGaSe (CIGS), which are widely used in commercial solar cells. CdTe thin-film solar cells typically show a photocurrent density between 20 and 25 mA cm−2,14 while CIGS thin-film solar cells generally have a photocurrent density around 30 mA cm−2, with high-performance variants exceeding 35 mA cm−2 in laboratory settings.15 Among various metal chalcogenides, multi-component chalcogenidometalates offer diverse network topologies and tunable optical band structures due to their adjustable chemical composition, making them promising candidates for high-performance solar energy conversion materials.
Despite the large number of multi-component chalcogenidometalates that have been synthesized and studied, a comprehensive summary on the design and synthesis of high-performance chalcogenides for solar energy conversion applications remains lacking. According to the Shockley–Queisser criteria,16 the optimal band gap for a light-absorbing material is around 1.3 eV. The Spectroscopic Limited Maximum Efficiency (SLME) theory17 provides a calculable metric for selecting suitable photovoltaic absorbers, specifically for the IpIIIqVIr chalcopyrite materials, through a more detailed analysis of their band structures. However, these criteria have proven to be insufficient, as numerous compounds with diverse chemical compositions, structural characteristics, and electronic band structures have been developed in recent decades, and not all of them adhere to these criteria in terms of their solar energy conversion efficiency. Photocurrent response is a fundamental property of photoelectric devices and serves as a critical indicator of the solar energy conversion efficiency of potential semiconductor materials. This work, therefore, focuses on multi-component chalcogenidometalates and their photocurrent response properties, aiming to provide insights for the development of advanced materials for solar energy conversion technologies.
In this review, we present a comprehensive collection and discussion of reported multi-component chalcogenidometalates, which are classified into four distinct structural types: 0D clusters, 1D chains, 2D layers, and 3D frameworks. These materials belong to five crystal systems and a total of 26 space groups. A statistical analysis of the multi-component chalcogenidometalates with reported photocurrent response experiments is provided, as shown in Fig. 1. Notably, the majority of these materials fall within the monoclinic crystal system, accounting for approximately 50%, and more than 55% exhibit photocurrent densities ranging from 2 to 50 μA cm−2. The details of the synthetic conditions, crystal systems, space groups, structural dimensions, optical band gaps, and photocurrent densities are summarized in Table 1 in the following sections.18–54 Additionally, we systematically investigate and summarize the relationships between chemical composition, structural characteristic, electronic band structure, and photocurrent performance. This analysis offers new insights into the design and optimization of chalcogenidometalates for enhanced photocurrent responses. It is our hope that this review will serve as a valuable resource for advancing the understanding and study of photocurrent behaviors in multi-component chalcogenidometalates, facilitating their development for future applications in solar energy conversion and related technologies.
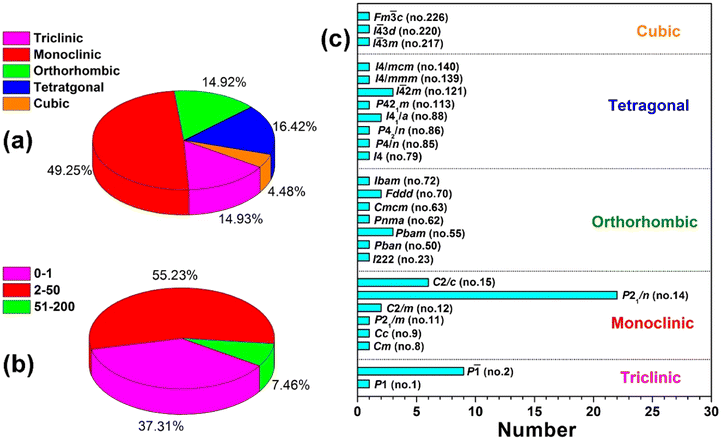 |
| Fig. 1 Distributions of the reported chalcogenidometalates in (a) crystal systems, (b) photocurrent density intervals (ranging from 0–1 μA cm−2, 2–50 μA cm−2, and 51–200 μA cm−2), and (c) space groups. | |
Table 1 A summary of the reported multi-component chalcogenidometalates exhibiting photocurrent responses
No. |
Formula |
Crystal system |
Space group |
Dimension |
Synthetic method |
Band gap (eV) |
Photocurrent density (μA cm−2) |
Ref. |
1 |
[Mn2(en)4Ge2S6]n |
Monoclinic |
P21/c (no. 14) |
1D |
Solvothermal synthesis |
3.27 |
1.2 |
18
|
2 |
[Mn2(dap)4Ge2S6]n |
Monoclinic |
P21/c (no. 14) |
1D |
Solvothermal synthesis |
2.87 |
1.9 |
18
|
3 |
[V(en)2(ea)]2[Ge2Se6] |
Monoclinic |
P21/n (no. 14) |
0D |
Solvothermal synthesis |
2.44 |
30.8 |
18
|
4 |
[V(teta)(ea)]2[Ge2Se6] |
Monoclinic |
P21/n (no. 14) |
0D |
Solvothermal synthesis |
2.47 |
16.1 |
18
|
5 |
[V2(en)6(μ-O)][Ge2Se6] |
Triclinic |
P (no. 2) |
0D |
Solvothermal synthesis |
1.66 |
22.8 |
18
|
6 |
[VII(dien)2]2[Sn2Se6] |
Monoclinic |
P21/c (no. 14) |
0D |
Solvothermal synthesis |
2.11 |
28 |
19
|
7 |
[Co(dien)2]2[Sn2Se6] |
Monoclinic |
P21/n (no. 14) |
0D |
Solvothermal synthesis |
N/A |
50 |
20
|
8 |
[Co(dien)2]2[Sn2S6] |
Monoclinic |
P21/n (no. 14) |
0D |
Solvothermal synthesis |
N/A |
15 |
20
|
9 |
[Ni(dien)2]2[Sn2S6] |
Monoclinic |
P21/n (no. 14) |
0D |
Solvothermal synthesis |
N/A |
10 |
20
|
10 |
[(Ni0.4Zn0.6)(en)6] [Sn2Se6] |
Monoclinic |
P21/n (no. 14) |
0D |
Solvothermal synthesis |
N/A |
35 |
20
|
11 |
[(Mn1.67Zn0.33)(en)6] [Sn2Se6] |
Monoclinic |
P21/n (no. 14) |
0D |
Solvothermal synthesis |
N/A |
20 |
20
|
12 |
[Zn(tren)2H]SbSe4 |
Monoclinic |
P21/n (no. 14) |
0D |
Solvothermal synthesis |
2.07 |
7.25 |
21
|
13 |
[Ni(1,2-dap)3]2[Zn(1,2-dap)]Sb2Se8 |
Monoclinic |
P21/n (no. 14) |
0D |
Solvothermal synthesis |
1.95 |
9.75 |
21
|
14 |
[Zn(tren)]2Sb2Se5 |
Monoclinic |
C2/c (no. 15) |
0D |
Solvothermal synthesis |
2.26 |
1 |
22
|
15 |
[Zn(tepa)H]2Sb2S6 |
Tetragonal |
I41/a (no. 88) |
0D |
Solvothermal synthesis |
1.80 |
1 |
22
|
16 |
Mn(en)3Sb2S4 |
Monoclinic |
P21/c (no. 14) |
1D |
Solvothermal synthesis |
2.20 |
0.75 |
23
|
17 |
Co(en)3Sb2Se4 |
Monoclinic |
P21/c (no. 14) |
1D |
Solvothermal synthesis |
2.25 |
0.6 |
23
|
18 |
{[(Ga10S17)(H+-AEP)2](H+-AEP)2} |
Orthorhombic |
Pban (no. 50) |
1D |
Solvothermal synthesis |
2.48 |
2.89 |
24
|
19 |
Ba3HgGa2S7 |
Monoclinic |
P21/c (no. 14) |
0D |
Solid-state reaction |
3.64 |
12.2 |
25
|
20 |
Ba2AgInS4 |
Orthorhombic |
Pnma (no. 62) |
2D |
Solvothermal synthesis |
1.98 |
0.02 |
26
|
21 |
[H3dien][Ag5Sn4Se12]·3H2O |
Tetragonal |
P 21m (no. 113) |
3D |
Solvothermal synthesis |
1.82 |
109 |
27
|
22 |
[Cu2GeSe5]·[Mn(H+en)2(en)] |
Monoclinic |
P21/c (no. 14) |
1D |
Solvothermal synthesis |
1.69 |
0.78 |
28
|
23 |
[Cu2SnSe5]·[Mn(H+en)2(en)] |
Monoclinic |
P21/c (no. 14) |
1D |
Solvothermal synthesis |
1.49 |
3.83 |
28
|
24 |
(NH4)4Ag12Sn7Se22 |
Monoclinic |
C2/c (no. 15) |
3D |
Solvothermal synthesis |
1.21 |
40 |
29
|
25 |
BaAg2GeS4 |
Tetragonal |
I 2m (no. 121) |
3D |
Solvothermal synthesis |
2.21 |
2.25 |
26
|
26 |
Cs2CdGeSe4 |
Orthorhombic |
Fddd (no. 70) |
1D |
Solvothermal synthesis |
2.41 |
180 |
30
|
27 |
Cs2Hg2GeSe5 |
Tetragonal |
I4 (no. 79) |
0D |
Solvothermal synthesis |
1.92 |
65 |
30
|
28 |
Ba3Ag2Sn2S8 |
Cubic |
I 3d (no. 220) |
3D |
Solvothermal synthesis |
2.02 |
0.2 |
26
|
29 |
BaAg2SnS4 |
Orthorhombic |
I222 (no. 23) |
3D |
Solvothermal synthesis |
1.88 |
2.25 |
26
|
30 |
Cs2Ag2SnS4 |
Orthorhombic |
Ibam (no. 72) |
2D |
Solvothermal synthesis |
2.72 |
0.2 |
31
|
31 |
Cs2MnSnSe4 |
Orthorhombic |
Fddd (no. 70) |
2D |
Solvothermal synthesis |
1.90 |
45 |
32
|
32 |
Rb2MnSnSe4 |
Monoclinic |
P21/n (no. 14) |
2D |
Solvothermal synthesis |
1.61 |
80 |
32
|
33 |
K2HgSnSe4 |
Tetragonal |
I4/mcm (no. 140) |
1D |
Solvothermal synthesis |
2.01 |
1.74 |
33
|
34 |
Na6Cu8Sn3Se13 |
Cubic |
Fm c (no. 226) |
3D |
Solvothermal synthesis |
1.76 |
3.32 |
33
|
35 |
Rb2AgAsS4 |
Triclinic |
P (no. 2) |
1D |
Solvothermal synthesis |
2.65 |
0.78 |
34
|
36 |
RbAg2AsS3 |
Triclinic |
P (no. 2) |
2D |
Solvothermal synthesis |
2.30 |
1.5 |
34
|
37 |
Cs2Ag6As2S7 |
Monoclinic |
P21/m (no. 11) |
2D |
Solvothermal synthesis |
2.10 |
3.83 |
34
|
38 |
Cs3CuAs4S8 |
Monoclinic |
C2/c (no. 15) |
2D |
Solvothermal synthesis |
2.26 |
5 |
35
|
39 |
Cs3CuAs4Se8 |
Monoclinic |
C2/c (no. 15) |
2D |
Solvothermal synthesis |
1.83 |
5 |
35
|
40 |
Cs2ZnAs4S8 |
Tetragonal |
I41/a (no. 88) |
3D |
Solvothermal synthesis |
2.24 |
3 |
36
|
41 |
Cs2CdAsSe5 |
Tetragonal |
P42/n (no. 86) |
0D |
Solvothermal synthesis |
2.06 |
4 |
37
|
42 |
[(NH4)Cs]CdAs4S8 |
Tetragonal |
P4/n (no. 85) |
2D |
Solvothermal synthesis |
2.36 |
0.75 |
36
|
43 |
AgCdAsS3 |
Monoclinic |
C2/c (no. 15) |
3D |
Solid-state reaction |
2.20 |
0.38 |
38
|
44 |
AgHgAsS3 |
Monoclinic |
Cc (no. 9) |
3D |
Solid-state reaction |
1.90 |
0.7 |
38
|
45 |
[pipH2]0.5[Ag2SbS3] |
Monoclinic |
P21/n (no. 14) |
2D |
Solvothermal synthesis |
2.07 |
0.43 |
39
|
46 |
[pipH2]0.5[Ag2SbSe3] |
Monoclinic |
P21/n (no. 14) |
2D |
Solvothermal synthesis |
1.95 |
0.495 |
39
|
47 |
Mn(tren)GaSbS4 |
Triclinic |
P (no. 2) |
1D |
Solvothermal synthesis |
2.08 |
7.5 |
46
|
48 |
Fe(tren)GaSbS4 |
Triclinic |
P (no. 2) |
1D |
Solvothermal synthesis |
1.62 |
3 |
46
|
49 |
[Mn(en)3]CdSb2S4 |
Monoclinic |
C2/c (no. 15) |
1D |
Solvothermal synthesis |
2.58 |
2.59 |
41
|
50 |
K3Mn2Sb3S8 |
Tetragonal |
I 2m (no. 121) |
3D |
Solvothermal synthesis |
1.61 |
7 |
42
|
51 |
Rb3Mn2Sb3S8 |
Tetragonal |
I 2m (no. 121) |
3D |
Solvothermal synthesis |
1.58 |
7 |
42
|
52 |
BaCuSbS3 |
Orthorhombic |
Pbam (no. 55) |
3D |
Solvothermal synthesis |
2.0 |
0.06 |
43
|
53 |
BaCuSbSe3 |
Orthorhombic |
Pbam (no. 55) |
3D |
Solvothermal synthesis |
1.6 |
0.03 |
43
|
54 |
Rb2ZnSb4S8 |
Triclinic |
P1 (no. 1) |
2D |
Solvothermal synthesis |
1.88 |
10 |
21
|
55 |
Rb2CuSb7S12 |
Triclinic |
P (no. 2) |
3D |
Solvothermal synthesis |
1.54 |
10 |
44
|
56 |
KCu2SbS3 |
Triclinic |
P (no. 2) |
2D |
Reactive flux method |
1.7 |
2.5 |
45
|
57 |
SrCuSbS3 |
Orthorhombic |
Pbam (no. 55) |
3D |
Molten salt method |
1.9 |
150 |
46
|
58 |
KCuZnS2 |
Tetragonal |
I4/mmm (no. 139) |
2D |
Solvothermal synthesis |
1.98 |
0.75 |
47
|
59 |
Ba2Cu2Cd2S5 |
Monoclinic |
C2/m (no. 12) |
2D |
Solvothermal synthesis |
2.21 |
0.40 |
48
|
60 |
Cs2ZnP2Se6 |
Triclinic |
P (no. 2) |
1D |
Solid-state reaction |
2.67 |
0.18 |
49
|
61 |
Lu5GaS9 |
Triclinic |
P (no. 2) |
3D |
Solid-state reaction |
2.34 |
0.15 |
50
|
62 |
Yb6Ga4S15 |
Monoclinic |
C2/m (no. 12) |
3D |
Solid-state reaction |
2.11 |
0.06 |
50
|
63 |
[(Ba19Cl4)(Ga6Si12O42S8)] |
Orthorhombic |
Cmcm (no. 63) |
2D |
Solid-state reaction |
4.65 |
1.25 |
51
|
64 |
Zn4B6O12S |
Cubic |
I 3m (no. 217) |
3D |
Solid-state reaction |
3.46 |
2.1 |
52
|
65 |
Sr2Sb2O2S3 |
Monoclinic |
P21/c (no.14) |
1D |
Solid-state reaction |
2.44 |
1.1 |
53
|
66 |
Sr2Sb2O2Se3 |
Monoclinic |
P21/c (no. 14) |
1D |
Solid-state reaction |
1.75 |
1.95 |
53
|
67 |
Sr6Cd2Sb6S10O7 |
Monoclinic |
Cm (no. 8) |
2D |
Solid-state reaction |
2.01 |
2.0 |
54
|
2. Mechanism and measurement of photoelectric property
In 1887, the German physicist Heinrich Hertz first observed the photoelectric effect during experiments aimed at validating the wave theory of light. This groundbreaking discovery played a pivotal role in the development of quantum theory. In 1902, Wilhelm Lenard expanded on Hertz's findings, describing the photoelectric effect as a phenomenon in which electrons in metals absorb the energy of incident light and escape from the material's surface. However, this phenomenon could not be explained by the prevailing theories of that time. It wasn't until 1905 that Albert Einstein proposed his photon hypothesis, offering a clear and robust explanation for the photoelectric effect. According to Einstein, the photoelectric effect occurs when a semiconductor is bombarded by photons of energy (hv). The semiconductor absorbs this photon energy, leading to the generation of corresponding electrical effects.55
Einstein's equation for the photoelectric effect, which encapsulates the energy transfer between light and electrons, is expressed as follows:
and Critical Frequency:
hv: photon energy,
Ws: metal surface work function,
Ep = 1/2
Mv2: the kinetic energy of photoelectron.
When a photon strikes the surface of a semiconductor, its energy is transferrs to an electron in the material. This energy helps the electron overcome the attractive forces of the metal surface, allowing it to escape. The remaining energy is then converted into the kinetic energy of the emitted photoelectron. The photocurrent, which is generated by the absorption of light energy, results from the excitation of electrons and holes within the material. Upon absorption, electrons in the semiconductor transition from the valence band to the conduction band. The generated charge carriers then move towards the surface of the semiconductor. If an external circuit is connected, this movement of charge carriers results in the flow of an electric current. The photocurrent density is defined as the ratio of the photocurrent to the illuminated area of the photoelectrode under a specific light source, and it is a critical parameter for evaluating the photoelectric effect in photoelectrochemical experiments.
In photoelectric response experiments, the standard three-electrode system is commonly used, and these experiments are typically conducted on an electrochemical workstation. The semiconductor powder is coated onto indium tin oxide (ITO) glass, which serves as the working electrode. The reference electrode is usually a saturated mercury/mercury chloride (Hg/HgCl2) or silver/silver chloride (Ag/AgCl2) electrode, while a platinum (Pt) electrode is commonly employed as the counter electrode. The electrolyte solution is typically a standard aqueous Na2SO4 solution, which provides the necessary ionic conductivity for the system. The photocurrent density, a key parameter in photoelectric experiments, can be expressed using the following equation:
| Jp = jmax × (ηabs × ηsep × ηinj) | (3) |
Jp: actual measured photocurrent density,
jmax: the maximum theoretical photocurrent density of a semiconductor photoelectrode,
ηabs: light absorption efficiency of semiconductor photoelectrode,
ηsep: charge separation efficiency of semiconductor photoelectrode,
ηinj: surface charge injection efficiency of semiconductor photoelectrode.
56
In parallel, the photoelectric conversion efficiency can be quantified using the following formula:
| 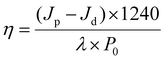 | (4) |
Jp: photocurrent density,
Jd: dark current density,
λ: incident monochromatic light wavelength,
P0: incident light intensity on the surface of the photoelectrode.
57
From eqn (3) and (4), it is evident that the photoelectric conversion efficiency (η) is directly proportional to the photocurrent density (Jp). This photocurrent density, in turn, is influenced not only by the light absorption and utilization efficiency of the photoelectrode, but also by the separation efficiency of the photogenerated charge carriers both internally and at the interface. The latter is a crucial factor in determining the photoelectrochemical properties of the system. The transient photocurrent response is an important tool for evaluating the separation efficiency of photo-induced electron–hole pairs within the photoelectrode. A higher photocurrent typically indicates a more efficient separation of these charge carriers. The process of photoelectrochemical conversion involves the absorption of light by semiconductor materials, which excites electrons (or holes) and drives them to participate in chemical reactions, leading to the formation of new substances. This process is influenced by multiple factors, including the semiconductor's ability to capture light, the generation and migration of photogenerated charge carriers, and the catalytic reactions occurring at the surface of the photoelectrode. Each of these factors plays a significant role in determining the overall efficiency of photoelectric conversion and, by extension, the performance of the photoelectrode in generating current and facilitating chemical transformations.
The photocurrent tests in this review are most taken using three-electrode mode in Na2SO4 aqueous solution under 300 W Xe simulated visible light with λ ≥ 420 nm. However, it is a common phenomenon that different compounds are tested under distinct bias voltages in the range of about 0–1.0 V. Generally, higher bias voltage brings larger photocurrent density value, because higher potential can restrain carrier trapping effect induced by interface defects through the induced electric field and thus retard the recombination rate of electrons and holes.53,54
3 Crystal structures and band gaps of main group chalcogenidometalates
3.1 Crystal structures of main group chalcogenidometalates with binary anionic frameworks
The common main group metals that constitute chalcogenides include Ga and In from group 13, Ge and Sn from group 14, and As and Sb from group 15. These metals predominantly form anion structures with chalcogen elements such as S or Se. In the presence of templating agents like organic amines, ammonium salts, or surfactants, the main group metals are coordinated with S or Se atoms, resulting in the formation of polymeric ions. When the coordination ability of these oligomeric metal ions is limited, charge balancing is achieved by incorporating cationic clusters. These clusters are typically composed of TM-based ions and organic molecules, leading to the creation of organic-hybrid compounds with a binary anionic framework. These compounds generally exhibit a 0D cluster structure and possess remarkable optical and photoelectric properties.
3.1.1 Crystal structures of group 14 chalcogenidometalates (Ge, Sn) with binary anionic frameworks.
The BBUs of Ge/Sn-chalcogenides are typically the tetrahedral [MQ4] unit, where M represents Ge or Sn, and Q denotes S or Se. In the presence of templating agents such as organic amines, ammonium salts, or surfactants, these tetrahedral [MQ4] units can aggregate to form polytetrahedral structures, denoted as [MxQy]z−, through μ-Q2− bridging bonds. These units can link via corner-sharing or edge-sharing interactions, resulting in a wide variety of hybrid chalcogenidometalates with diverse dimensionalities and structural configurations. Recent studies have highlighted a significant correlation between the basic structural motifs of Ge/Sn-chalcogenides and their photoelectric properties, suggesting that the arrangement and connectivity of the [MQ4] units play a crucial role in determining the performance of these materials in optoelectronic applications.
According to H. Luo et al. (2015), five hybrid main-group chalcogenides—[Mn2(en)4Ge2S6]n (1), [Mn2(dap)4Ge2S6]n (2), [V(en)2(ea)]2[Ge2Se6] (3), [V(teta)(ea)]2[Ge2Se6] (4), and [V2(en)6(μ-O)][Ge2Se6] (5)—were synthesized via a solvothermal method.18 In compounds 1 and 2, the dimeric [Ge2S6]4− anions form 1D chain-like structures, where the Mn-containing complex cations are interconnected with the anions (Fig. 2a and b). Compounds 3 and 4 are based on [Ge2Se6]4−, where two [GeSe4] units are connected by edge-sharing, with V-containing complex cations completing the structures (Fig. 2c and d). The cation in compound 5 is [V2(en)6(μ-O)]4+, with ethylenediamine (en) acting as a monodentate ligand (Fig. 2e). Notably, compounds 3–5 exhibit excellent photocurrent responses, demonstrating their potential as efficient photosensitizers. The rare low-valent V2+ complex cation selenide, [V(dien)2]2[Sn2Se6]24− (6), which contains [Sn2Se6]4− anions and [V(dien)2]2+ complex cations (Fig. 2f), shows a reproducible photocurrent response of approximately 2.0 μA cm−2.19 This suggests that photo-generated electrons from the Se-4p orbitals can rapidly transfer to the FTO electrode, generating an anodic current. The UV–Vis absorption data for compound 6 (Table 1) indicates an experimental band gap of 2.11 eV, further supporting its photocurrent-generating potential. 1–2 show very low responsiveness in comparison with that of 4–6. This result means that 4–6 could be a good photosensitizer.
 |
| Fig. 2 The crystal structure of compounds 1–6 (a–f). | |
Additionally, the photocurrent responses of five novel isostructural TM–Sn–Q 0D cluster structures (TM = transition metals; Q = S, Se) (7–11), reported by Menghe Baiyin in 2022, are both rapid and repeatable.20 Among these, [Co(dien)2]2[Sn2Se6] (7) exhibits a current density of nearly 50 μA cm−2, which surpasses that of most chalcogenidostannates, indicating superior electron–hole pair generation and separation efficiency. These findings suggest that chalcogenidostannates containing transition metal based complexes may exhibit enhanced photocurrent responses compared to other chalcogenides (see subsequent chapters). This improvement may be attributed to the decrease in atomic radius of group 14 elements across the period, as well as the corresponding reduction in electronegativity, which facilitates the migration of more free electrons into the external circuit, thereby producing a larger photocurrent. Moreover, TM-based complexes contribute to the formation of unusual structures through their unique spatial arrangements, providing synergistic effects with the inorganic frameworks.
3.1.2 Crystal structures of group 15 chalcogenidometalates (As, Sb) with binary anionic frameworks.
In comparison to the group 14, metal elements from the group 15 of the periodic table often exhibit a broader range of coordination modes, owing to the presence of stereochemically active lone pairs. These lone pairs contribute to the flexibility and diversity in the coordination environment of these elements.58 The anionic frameworks of binary group 15 chalcogenidometalates are primarily composed of a series of polymeric ions, [MxQy]z− (M = As, Sb; Q = S, Se). These frameworks are formed by [MQ3] trigonal and [MQ4] tetrahedral units, which serve as the BBUs of the structure. These unique coordination motifs not only influence the structural characteristics of these compounds but also have a significant impact on their physical properties. According to existing studies, photoresponsive characterizations have predominantly been carried out on chalcogenides that incorporate inorganic–organic hybrid cations. For instance, the 0D [Zn(tren)2H]SbSe4 (12) comprises trigonal bipyramidal Zn2+ ions and [SbSe4] clusters, while the unique [Ni(1,2-dap)3]2Zn(1,2-dap)Sb2Se8 (13) features Zn2+ ions that coordinate with both organic amines and Se atoms (Fig. 3a and b).21 In 2023, two novel chalcogenides were synthesized incorporating [Zn(tren)]2+ and [Zn(tepa)H]2+ cations. These materials showcase 0D cluster structures based on the binary anionic frameworks [Sb2Se5]n2n− and [Sb2S6]n3n−, respectively, both of which are built from the primary [SbQ4] (Q = S, Se) unit (Fig. 3c and d).22 Additionally, the same research group solvothermally synthesized Mn(en)3Sb2S4 (16) and Co(en)3Sb2Se4 (17), which are composed of Mn/Co complex cations and 1D anionic chains (Fig. 3e).23
 |
| Fig. 3 The structure of compounds 12 (a), 13 (b), 14 (c), 15 (d), and 16 (e) (16 and 17 are isostructural). | |
Among these compounds, 12 and 13 exhibit notably stronger photocurrent responses compared to others, indicating superior photogenerated electron–hole pair transfer and separation efficiency. The enhanced activity of the lone pair electrons of Sb3+ in compounds 12 and 13 contributes to a redshift in the ultraviolet absorption edge, thereby reducing the band gap. A narrower band gap allows for a broader spectral absorption range and a higher absorption rate across the solar spectrum, ultimately leading to the generation of more photogenerated charge carriers, which significantly enhances the photoconductivity effect. It indicated that compound 12 and 13 have a potential development prospect in the field of solar cell materials.
3.2 Crystal structures of main group chalcogenidometalates with ternary anionic frameworks
The incorporation of transition metals into the anionic frameworks of main group chalcogenidometalates significantly enhances their structural complexity and imparts distinctive properties, sparking considerable interest in their study. Compared to chalcogenidometalates with simpler binary anionic frameworks, the introduction of ternary mixed anions leads to more intricate polyhedral distortions. These distortions not only contribute to the development of stronger polarity but also induce greater anisotropy in the materials. As a result, ternary anion frameworks often exhibit exceptional photoelectronic properties. This is attributed to the combination of metal atoms with varying sizes and electronegativities, which creates a rich interplay of electronic states and structural variations that can be finely tuned for specific applications.
3.2.1 Crystal structures of group 13 chalcogenidometalates (Ga, In) with ternary anionic frameworks.
The basic building unit of Ga/In chalcogenides is the tetrahedral [MQ4] unit (M = Ga, In; Q = S, Se). Under solvothermal conditions, and in the presence of templating agents such as organic amines, quaternary ammonium salts, or other nitrogen-containing organic compounds, multiple [MQ4] tetrahedra can aggregate into larger polytetrahedral structures, forming [MxQy]z− clusters. These clusters can exhibit various topologies, such as hypertetrahedral clusters (Tn clusters), pentagonal supertetrahedral clusters (Pn clusters), hatched supertetrahedral clusters (Pn clusters), and other complex supertetrahedral configurations. The formation of these diverse superclusters allows for the synthesis of a wide range of inorganic–organic hybrid materials and inorganic chalcogenidometalates with varying dimensionalities, spanning from 1D to 3D architectures. For instance, in the 1D structure {[(Ga10S17)(H+-AEP)2](H+-AEP)2} (18), a T3-[GaS] cluster is coordinated to two organic AEP ligands through neutral amine groups that bond to two corner Ga atoms, forming a unique molecular assembly (Fig. 4a).24 In contrast, the 0D Ba3HgGa2S7 (19) features Ba2+ counterions, and the anionic framework displays a [Ga2S2] parallelogram formed between two [GaS4] tetrahedra, which further links to the [Hg2Ga4S14]12− string (Fig. 4b).25 Moving to the two-dimensional Ba2AgInS4 (20), the structure is built from [AgS4] and [InS4] tetrahedra that are connected through corner-sharing interactions, resulting in a layered 2D framework (Fig. 4c).26
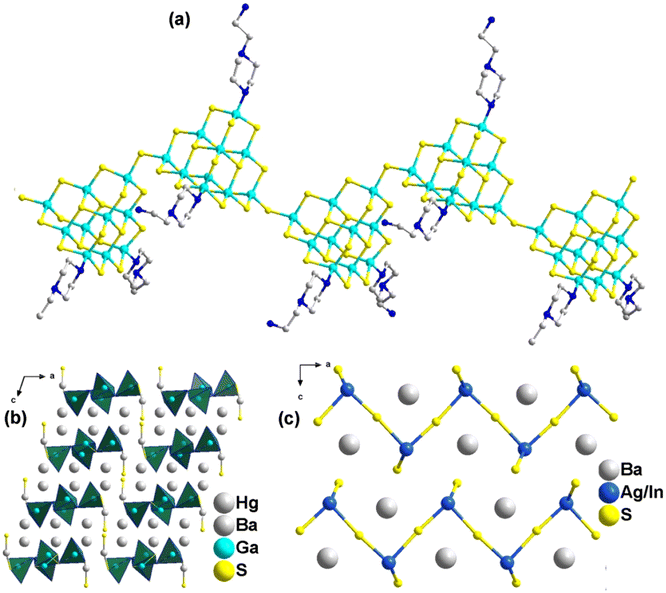 |
| Fig. 4 The structure of compound 18 (a), 19 (b) and 20 (c). | |
Among these ternary chalcogenides, Ba3HgGa2S7 (19) demonstrates the strongest photocurrent response, reaching approximately 12.2 μA cm−2 (Table 1). The enhanced photocurrent response can be attributed to the presence of [HgS2] units within the structure, where the large polarizable Hg atoms—due to their fully occupied d10 configuration—contribute to the formation of built-in electric fields via Jahn–Teller distortion.59 This phenomenon promotes efficient photogenerated charge separation, stabilizes in-plane carriers, and reduces the formation of photogenerated carrier complexes, which collectively enhance the photocurrent response. UV-Vis absorption spectra reveal distinct band gaps for the materials: 2.48 eV for compound 18, 3.64 eV for compound 19, and 1.98 eV for compound 20 (Table 1). Notably, compound 19 exhibits the largest band gap, which is likely due to its unique chemical composition and electronic structure. The [HgS2] units within Ba3HgGa2S7 (19) primarily contribute to the valence band, resulting in a relatively wide band gap. However, 19 exhibits a stronger photocurrent response compared to the other two compounds, which further illustrates the significant impact of large polarizable Hg atoms on photocurrent property. Therefore, 19 provides an opportunity for discovering new chalcogenides with promising photocurrent properties.
3.2.2 Crystal structures of group 14 chalcogenidometalates (Ge, Sn) with ternary anionic frameworks.
As outlined in section 3.1.1, the BBUs of Ge/Sn-chalcogenidometalates are the tetrahedron [MQ4] (where M = Ge, Sn and Q = S, Se). A series of polymeric thio/selenidogermanates with ternary anionic frameworks, which exhibit stability in air, have been synthesized by incorporating transition metals as linkers for the [SnQ4] units. A representative compound featuring protonated organic amine cations is the organic–inorganic silver-rich selenidostannate [H3dien][Ag5Sn4Se12]·3H2O (21) (Fig. 5a).27 The core structure of compound 21 comprises discrete [Sn2Se6] clusters and [Ag5Se8]n chains, which interconnect to form a 3D [Ag5Sn4Se12]n3n− framework with 1D channels running through it. Similar to compound 21, two isostructural compounds, [Cu2MSe5]·[Mn(H+-en)2(en)] (M = Ge (22), Sn (23)), feature 1D chains of [Cu2MSe5]4− anions, and their band gaps were found to be 1.69 eV for compound 22 and 1.49 eV for compound 23 (Fig. 5b).28 The near-infrared-triggered photoelectric response observed in compound 23 highlights its potential for application in photoelectric sensors. Compared to compounds 21 and 22, the band gaps of compounds 23 and (NH4)4Ag12Sn7Se22 (24) display a notable red shift (1.49 and 1.21 eV, respectively), suggesting their potential for near-infrared photoelectronic applications (Table 1).29 Additionally, the photocurrent response demonstrates highly repeatable photovoltage behavior, further validating the near-infrared photoelectronic capabilities of compounds 23 and 24. Compound 21, in particular, exhibits a rapid and stable photocurrent response with a current intensity as high as 109 μA cm−2, indicating highly efficient carrier separation under light irradiation. Data suggests that, in most cases, tin selenides exhibit superior photocurrent responses compared to germanium selenides, as the heavier Sn atom typically results in a lower conduction band energy, which is more favorable for charge carrier movement.28
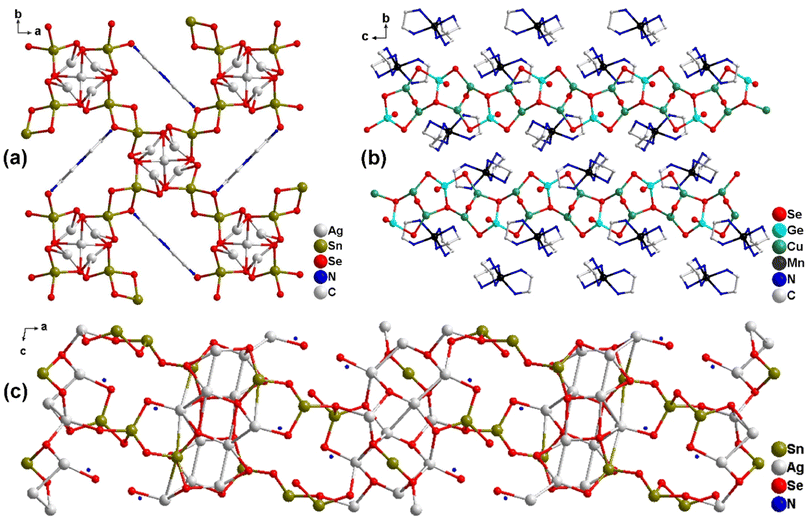 |
| Fig. 5 The structure of compound 21 (a), 23 (b) and 24 (c). (22 and 23 are isostructural). | |
Inorganic multi-compounds involving alkali or alkaline earth metals as equilibrium cations filling the ternary germanium/tin-anion frameworks have also been explored. In the case of BaAg2GeS4 (25),26 the 3D anionic [Ag2GeS4]2− framework consists of [AgS4] tetrahedra and an infinite [AgS2] layer, where Ge4+ ions are embedded between the AgS2 layers, sharing corners with the [AgS4] tetrahedra (Fig. 6a). Other noteworthy examples include Cs2CdGeSe4 (26) and Cs2Hg2GeSe5 (27), both synthesized under solvothermal conditions.30 Compound 26 is composed of 1D infinite chains of {[GeCdSe4]2−} anions, which consist of [GeSe4] and [CdS4] tetrahedra (Fig. 6b). Compound 27, on the other hand, features a 0D cluster structure where four [HgSe4] tetrahedra form an 8-membered ring via vertex-sharing. This 8-membered ring contributes to the formation of [GeHgSe5]n2n− clusters in conjunction with [GeSe4] tetrahedra (Fig. 6c). As shown in Table 1, the band gap (Eg) of compound 27 is smaller than those of compounds 25 and 26, suggesting a broader absorption range. The photocurrent densities for compounds 25, 26, and 27 are 0.02, 180, and 65 μA cm−2, respectively, indicating that compounds 26 and 27 exhibit significantly better photoelectron-hole transfer and separation efficiency than compound 25. These results support the idea that transition metals with a d10 electron configuration in the anion skeletons can enhance photocurrent response properties, as discussed in section 3.2.1.
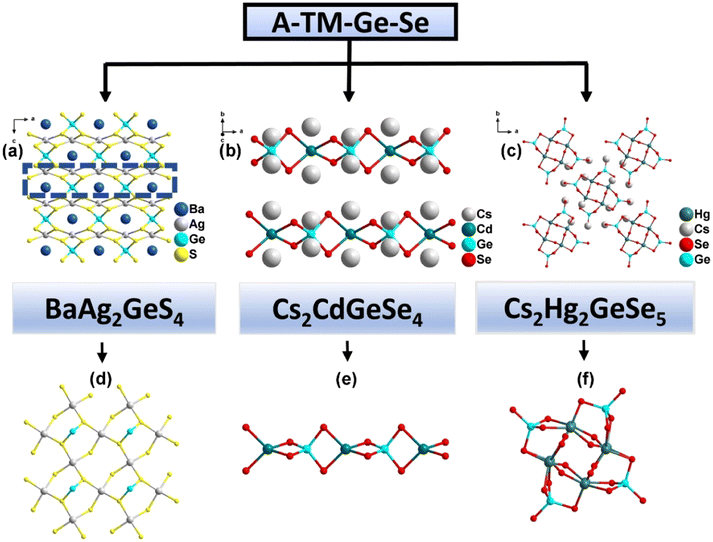 |
| Fig. 6 The structure of compounds 25 (a and d), 26 (b and e), and 27 (c and f). | |
In the category of A–Ag–Sn–S compounds, Ba3Ag2Sn2S8 (28) features a 3D [Ag2Sn2S8]6− framework constructed by [AgS4] and [SnS4] units through vertex-sharing (Fig. 7a).26 BaAg2SnS4 (29)16 and Cs2Ag2SnS4 (30)21 both possess outstanding optical performance. For 29, vertex-sharing of [AgS4] tetrahedra help form the [AgS2] 2D layer, while Sn tetrahedra connect [AgS2] layers and build a 3D [Ag2SnS4]2− skeleton (Fig. 7b). For compound 30, [AgS2]n3n− chains which formed by [AgS4] tetrahedra are connected via Sn4+ ions and generating a 2D layered anion (Fig. 7c). The different structural dimensions of 29 and 30 mainly due to the types of charge-balancing agent. Among the A–Ag–Sn–S compounds, the photocurrent density of 29 (2.25 μA cm−2) indicates a good transfer and separation efficiency of photogenerated electron–hole pairs under simulated solar light illumination, which may be related to its Eg of 1.88 eV (Table 1). Band gap plays an important role in photocurrent response. When a certain compound has a smaller band gap, it is easier for electrons to transition across the band gap, resulting in more electrons and holes, enhancing the photocurrent activity. The good photoelectric responsive properties of compound 29 under simulated solar light illumination make it promising as a photoelectric device.
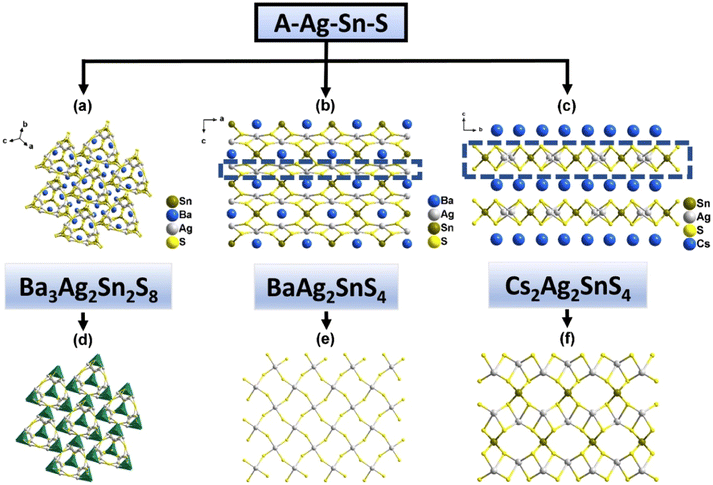 |
| Fig. 7 The structure of compounds 28 (a and d), 29 (b and e), and 30 (c and f). | |
Typical examples of tin-selenides include the family of A2TSnSe4 (A = alkali metal; T = Mn, Hg). Recent work on the photocurrent response performances of Cs2MnSnSe4 (31) and Rb2MnSnSe4 (32) supported that the tin-selenides had a strong photocurrent response.32 For compound 31, edge-sharing occurs between [MnSe4] and [SnSe4] to form the 1D anionic chain [MnSnSe4]n2− (Fig. 8a). The structure of 32 features 2D [MnSnSe4]n2− anionic network, which is composed of [Mn2Sn2Se10] cluster connected with four other adjacent units (Fig. 8b). Furthermore, it is clear that the charge-balanced Rb+/Cs+ are both located between the anions. The Eg of 31 and 32 are 1.90 eV and 1.61 eV, respectively (Table 1). The photocurrent densities of 31 and 32 are 45 μA cm−2 and 80 μA cm−2, indicating two p-type semiconductors. The narrow bandgap and high photocurrent response make compound 32 an alternative material for solar cells. Another example in the family of A2TSnSe4 (A = alkali metal; T = Mn, Hg) is K2HgSnSe4 (33), which features 1D [HgSnSe4]n2n− anions but a much weaker photo response (1.74 μA cm−2), close to that of Na6Cu8Sn3Se13 (34) (3.32 μA cm−2) contains 3D [Cu8Sn3Se13]6− anionic framework which balanced by Na+ ions (Fig. 8c and d).33 A conclusion can be drawn through the research of the A2TSnSe4 (A = alkali metal; T = Mn, Hg) family that crystal structure has a vital influence on optical properties and photocurrent response properties of compounds.
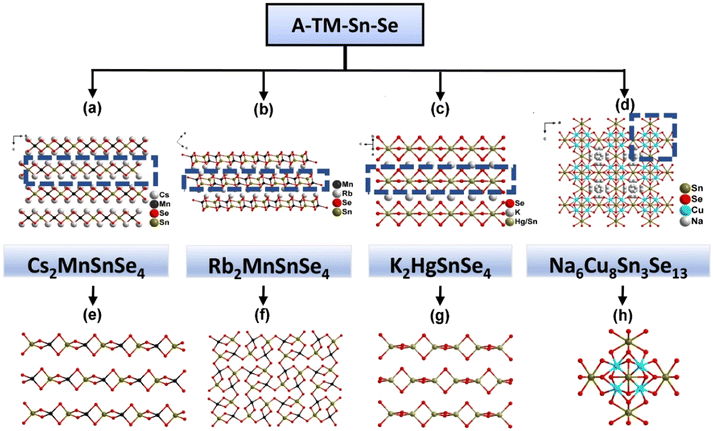 |
| Fig. 8 The structure of compounds 31 (a and e), 32 (b and f), 33 (c and g) and 34 (d and h). | |
3.2.3 Crystal structures of group 15 chalcogenidometalates (As, Sb) with ternary anionic frameworks.
The basic building unit of thio/selenidoarsenates(V) is mainly tetrahedral [AsQ4]3− (Q = S, Se), which is usually linked to transition metal polyhedra via edge/vertex-sharing to form compounds with a variety of structures. In 1D Rb2AgAsS4 (35),24 the As5+ ion coordinated with four S2− ions in a nearly ideal tetrahedral configuration and formed a [AgAsS4]2− dimer unit with AgS4 distorted tetrahedra as found in Fig. 9a. Trivalent thio/selenidoarsenates(III) mainly adapt to the trigonal pyramid [AsIIIS3] unit. In the 2D layered structure of RbAg2AsS3 (36),34 the [AsIIIS3] unit is stabilized by connecting with [AgS4]. Cs2Ag6As2S7 (37) also possesses a 2D anionic layer with corner-sharing [AsIIIQ3] and [AgS3] units and the mono-layered [Ag3AsS4]n2n− form a double-layered structure via the connection of S2− ions (Fig. 9c).34 The photocurrent responses of 35–37 enhance along with their Eg values decreasing from 2.65 to 2.10 eV. According to the density of states (DOS) calculation by density functional theory (DFT), valence bands of 35–37 are mainly composed of Ag-4d and S-3p states, but due to the valence change of As ion, As5+ with unoccupied 4s states in 35 causes the predomination of As-4s and S-3p states in conduction band bottom, while As3+ with filled 4s orbitals in 36 and 37 causes the predomination of As-4p and S-3p states in conduction band bottom. Thus, band structure changes caused by As5+/As3+ ions affected the photocurrent responses of the compounds. Moreover, the increasing of Ag/As ratios of 35–37 indicated that a higher proportion of Ag+ can improve the photocurrent responses through adjusting band structure.
 |
| Fig. 9 The structure of compounds 35 (a and d), 36 (b and e), and 37 (c and f). | |
As displayed in Fig. 10a, Cs3CuAs4Q8 (Q = S, Se) (38, 39)35 2D layer structure was formed by eight [AsQ3] and two [CuQ3] units, which connected to form a [Cu2As8Q18] cluster through vertex-sharing. These [Cu2As8Q18] clusters are interlinked to construct a double-layered 2D [CuAs4S8]3− anion. The open structure of a double-layered anion provides enough space for 4 independently located Cs+ cations (Fig. 10a). 3D Cs2ZnAs4S8(40) compound was obtained by replacing the transition metal Cu by Zn, which is constructed by Cs+ cations, [ZnS4] tetrahedra, and 1D [As4S8]4− chains consist of corner-sharing [As4S9] groups (Fig. 10b).36 The structure of Cs2CdAsSe5(41) is formed of windmill-like [Cd4As4Se16(Se2)2]8− clusters and charge balancing Cs+ cations (Fig. 10c).37 The crystal structure of [(NH4)Cs]CdAs4S8 (42) is composed of regular [CdS4] and tetranuclear [As4S8] clusters, the BBUs form the two-dimensional [CdAs4S8]2− layer filled with the dispersed (NH4)+ or Cs+ ions (Fig. 11a).26 The above anions of four thio/selenidoarsenates(III) possess an identical stoichiometry of 1–4–8, but they are significantly distinct in structural features from 3D to 0D while the photocurrent responses (Table 1) of 38–41 occur in a narrow range from 0.75 to 5 μA cm−2, indicating that the anion dimension has little influence on their photocurrent responses. Nevertheless, selenides 39 and 41 still display better photocurrent response properties and narrower band gaps than the other three sulfides.
 |
| Fig. 10 The structure of compounds 38 (a and d), 40 (b and e), and 41 (c and f). (38 and 39 are isostructural). | |
 |
| Fig. 11 The structure of compounds 42 (a), 43 (b), 44 (c), 45 (d), 47 (e), and 49 (f). (45 and 46 are isostructural, 47 and 48 are isostructural). | |
Double d10 cations with a variety of different coordination modes could be introduced to obtain thioarsenites AgMAsS3 (M = Cd, Hg) (43, 44). In these two compounds, the building units contain d10 cations are [AgS4]/[HgS4] in AgHgAsS3 (Fig. 11c) and [AgS5]/[CdS6] in AgCdAsS3 (Fig. 11b), respectively.38 The non-centrosymmetric (NCS) AgHgAsS3 show stronger photocurrent responses compared to centrosymmetric (CS) AgCdAsS3, which indicates that built-in electric field generated by polar structure could be beneficial for photogenerated electrons/holes separating. Meanwhile, the smaller band gap of AgHgAsS3 helps absorb a wider range of light.
The first-principles calculations of density of states elaborate the valence band of 44 mainly contains Ag-4d and S-3p states and shows strong stereochemical lone-pair activity. The conduction band bottom is composed of As-4p, S-3p, and Hg-5d states. Other reported trivalent thio/selenidoarsenates(III) such as RbAg2AsS3 (36), Cs2Ag6As2S7 (37), Cs2ZnAs4S8 (40) and [(NH4)Cs]CdAs4S8 (42) etc. exhibit the same feature of the band structure. Therefore, the essential motifs which dictates the band gap of AgHgAsS3 are distorted [HgQ4] (Q = S, Se) and [AsQ4] (Q = S, Se) units, in which charge-balancing cations are rarely involved.
The transition metals (Cu+, Ag+, Cd2+, Hg2+) can further integrate with [SbS3] units in thioantimonates to build the TM–Sb–Q (Q = S, Se) ternary anions. A series of organic hybrid thioantimonates with ternary anionic frameworks, which are stable in air, have been obtained by using transition metals as linkages for the [SbS3] units. [pipH2]0.5[Ag2SbS3] (45)39 has a 2D layered structure and it is isostructural with [pipH2]0.5[Ag2SbSe3] (46) (Fig. 11d).39 Compounds Mn(tren)GaSbS4 (47)40 and Fe(tren)GaSbS4 (48)40 are also isostructural, which featured a 1D chain formed by the pyramidal [SbS3] unit and slightly distorted tetrahedral [GaS4] unit (Fig. 11e). However, these isostructural compounds exhibit different photocurrent densities due to their band structure and carrier separation efficiency. In addition, [Mn(en)3]CdSb2S4 (49)41 features 1D anionic chains and [Mn(en)3]2+ charge-balancing complex cations (Fig. 11f).
By directly introducing alkali metal as the structure-directing agent, the novel Mn-based thioantimonate A3Mn2Sb3S8 (A = K, Rb) (50, 51)42 which belongs to the non-centrosymmetric space group were reported. Both compounds exhibit unique 3D open frameworks constructed by [Mn8S32] and [Sb3S7] units (Fig. 12a) and display the same rapid and consistent photocurrent responses, which indicate that the charge-balanced cations rarely affect the Eg and photocurrent responses. Other quaternary thioantimonates exhibit photocurrent response properties are BaCuSbS3 (52), BaCuSbSe3 (53) (Fig. 12b),43 Rb2ZnSb4S8 (54) (Fig. 12c),21 and Rb2CuSb7S12 (55)44 which featured a 3D or 2D framework with [CuS3] and [SbS3] triangular pyramids (Fig. 12e), and KCu2SbS3 (56)45 with the layered structure of two single heavily distorted graphene-like layers based on the [Cu2SbS3]− building unit (Fig. 12f). Another important example is SrCuSbS3 (57) (Fig. 12d),46 which is isostructural with 52 and 53, and consists of 3D [CuSbS3]2− extended framework and Sr2+ ions with Eg of 1.91 eV. When Sr2+ ions are partially replaced by Pb2+ ions, the Eg can be decreased to 1.63 eV, and the largest photocurrent density was tested as 162.9 μA cm−2 at 3 V for Sr0.85Pb0.15CuSbS3. Pb2+ doping decreased the Eg of SrCuSbS3 and created excellent solar light absorption photocatalytic materials. Compared to that of ionic Sr–S bonds in SrCuSbS3, the more delocalized electrons of Pb–S bonds efficiently narrow the Eg of the doping compounds. In addition, the introduction of Pb-6s/6p states around the Fermi level provides photo-generated electrons/holes separation and transport with enough mobility in optoelectronic processes.
 |
| Fig. 12 The structure of compounds 50 (a), 52 (b), 54 (c), 55 (e), 56 (f), and 57 (d). (50 and 51 are isostructural, 52 and 53 are isostructural). | |
4 Crystal structures and band gaps of other special chalcogenides
Multinary transition metal sulfides are renowned for their rich structural diversity and remarkable physical and chemical properties, which primarily arise from the various coordination modes they can adopt with S2− ions.60 However, the photocurrent response properties of transition metal chalcogenides remain relatively underexplored. Among the existing studies, we have identified several chalcogenides incorporating transition metals, whose anionic frameworks are predominantly 2D in nature, and these materials exhibit excellent photocurrent response characteristics. Typically, transition metal ions such as Zn2+ and Cd2+ exhibit coordination environments that include trigonal pyramidal [ZnS4] and octahedral [ZnS6] motifs. In particular, the compound KCuZnS2 (58)47 (Fig. 13a) presents an intriguing case, where both Cu+ and Zn2+ ions occupy the same crystallographic site. In this structure, each [CuS4] and [ZnS4] tetrahedron is connected to the other units via edge-sharing, resulting in a unique 2D layered arrangement. Similarly, another compound, Ba2Cu2Cd2S5 (59)48 (Fig. 13b), features a layered anionic framework of [Cu2Cd2S5]4−, with Cd2+ ions coordinated in tetrahedral geometry. Both of these compounds exhibit rapid and consistent photocurrent response profiles, indicating their strong potential for optoelectronic applications. The experimentally determined Eg of compounds 58 and 59 are 1.98 eV and 2.21 eV, respectively (Table 1). These values suggest that these materials are promising candidates for use in photoelectric devices, where their photocurrent response properties are of paramount importance. In our view, there is a critical need to develop and explore new transition metal chalcogenides with superior photocurrent response properties to further advance the field of energy conversion and optoelectronics.
 |
| Fig. 13 The structure of compounds 58 (a) and 59 (b). | |
In addition to the compounds discussed earlier, recent studies have revealed that certain chalcogenide systems with unique structures exhibit notable photocurrent properties. The crystal structure of Cs2ZnP2Se6 (60)49 is illustrated in Fig. 14. It features a 1D chain structure composed of [ZnP2Se2]2− chains that extend along the c-axis, interspersed with Cs+ cations. Two types of ethane-like motifs are observed, where the [P2Se6]4− groups and the [ZnSe4]6− units, which have four distinct bond lengths, intersect and share Se atoms, forming the 1D [ZnP2Se2]2− anionic chain. The optical absorption properties of the material are primarily governed by transitions between the valence band and conduction band near the Fermi level. Thus, the contributions of the [ZnSe4] and [P2Se6] groups are crucial to the material's optical characteristics. Compound Lu5GaS9 (61),50 shown in Fig. 15a, has the highest rare-earth (RE)/Ga molar ratio reported thus far in the RE/Ga/S system. All Lu atoms are six-fold coordinated in distorted [LuS6] octahedra. Remarkably, discrete dimeric [(GaS4)2] tetrahedra serve as the central species in the 3D anionic Lu–S channels. This structure plays a pivotal role in the material's photocurrent response. In Fig. 15b, Yb6Ga4S15 (62)50 features a distinct 3D framework based on 2D Yb–S layers, which are composed of two different corner-sharing substructures. These layers are further interconnected by 1D [GaS4] double-tetrahedron chains extending along the c-axis. Both compounds exhibit high efficiency in transferring photo-generated electrons and in separating electron–hole pairs. However, compound 61 demonstrates an even stronger transient photocurrent response, approximately three times larger than that of compound 62.
 |
| Fig. 14 The structure of compounds 60. | |
 |
| Fig. 15 The structure of compounds 61 (a) and 62 (b). | |
Recently, oxychalcogenides with mixed-anion groups have attracted significant attention as an important class of optoelectronic functional materials.61 This is due to their ability to combine the beneficial properties of oxides and chalcogenides through a chemical substitution strategy.62 As shown in Fig. 16a, compound [(Ba19Cl4)(Ga6Si12O42S8)](63),51 features a unique architecture in which two [GaO2S2], four [GaO3S], and four [Si2O7] units apex-share to form a [Si8Ga6O32S8] circular cluster. These clusters are interconnected to form a 2D wavelike layer along the ac-plane, facilitated by a disordered [Si2O7] unit that shares corners with O atoms. Between these layers, discrete Ba2+ cations and Cl− anions occupy interstitial spaces. Notably, compound 63 represents the first heteroanionic chalcogenide to exhibit a photocurrent response, marking a significant breakthrough in the field. Subsequently, other oxychalcogenides, including Zn4B6O12S (64),52 Sr2Sb2O2Q3 (Q = S (65), Se (66))53 and Sr6Cd2Sb6S10O7 (67)54 have been found to exhibit photocurrent responses. Compound 64 crystallizes in the noncentrosymmetric cubic structure. As shown in Fig. 16b, twenty-four vertex-sharing [BO4]5− tetrahedra form a sodalite cage, while four [ZnSO3]6− tetrahedra containing Zn–O and Zn–S bonds inside the cage, help restricting the rotation of rigid [BO4]5− tetrahedra. Compounds 65 and 66, show in Fig. 16c, adopt structures composed of double chains of edge-sharing [SbSe4O] square-based pyramids. In Fig. 16d, compound 67 exhibits the polar space group Cm with 2∞[CdSb2OS5]4− layers composed of [CdS3]4− tetrahedra and tetragonal pyramids of antimony [SbOS2]3−/[SbS3]3. The pseudochains built from [SbO4]5− units fill the spaces between the layers as well as Sr2+. Among these oxychalcogenides, compound 64 generate the highest photocurrent response (2.1 μA cm−2) with a relatively wide band gap of 3.46 eV, which is inconsistent with the general trend that narrower band gap always brings better photocurrent response. The same result occurs in 63 (∼1.25 μA cm−2, 4.65 eV). The special coordination environments of cations in these structures, namely the cations bond with both O2− and Q2−, contribute to the formation of polar units and thus the built-in electric field, can enhance the efficiency of e−/h+ separation. Therefore, these oxychalcogenides emerge as potential photocatalysts, offering improved photocurrent responses under visible light or ultraviolet illumination compared to oxides.
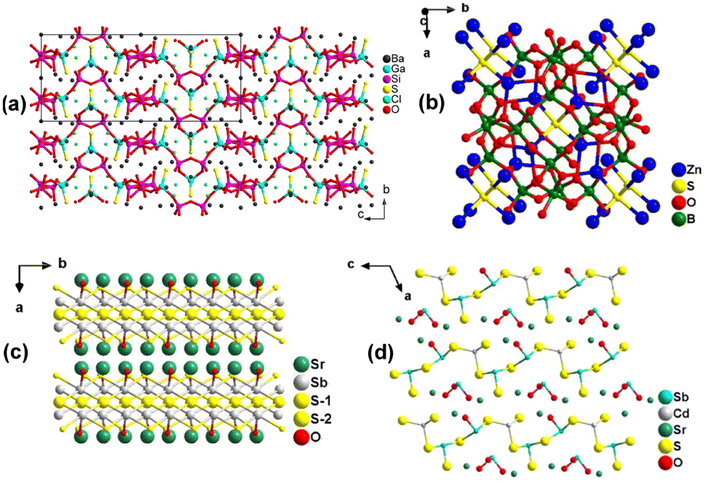 |
| Fig. 16 The structure of compounds 63 (a), 64 (b), 65 (c) and 67 (d). (65 and 66 are isostructural). | |
5 Discussions
After a systematic analysis of their structural characteristics and properties, several key conclusions have emerged that may serve as valuable guidance for future research in the field, the details are discussed below:
(1) Effect of band gap: from a statistical perspective, the Eg of the multi-component chalcogenidometalates discussed in this review can be categorized into three distinct ranges: <1.5 eV, 1.5–2.5 eV, and >2.5 eV. As illustrated in Fig. 17, the photocurrent density values within the 1.5–2.5 eV range are notably higher than those in the other two ranges. This trend clearly indicates that, in most cases, multi-component chalcogenidometalates with narrower Eg values exhibit larger photocurrent densities. This can be attributed to the fact that compounds with smaller Eg have a broader spectral absorption range, enabling them to absorb photons at lower energies. Moreover, these materials are more effective at minimizing carrier recombination, which further enhances their ability to efficiently capture photons and generate a higher number of photo-generated carriers.
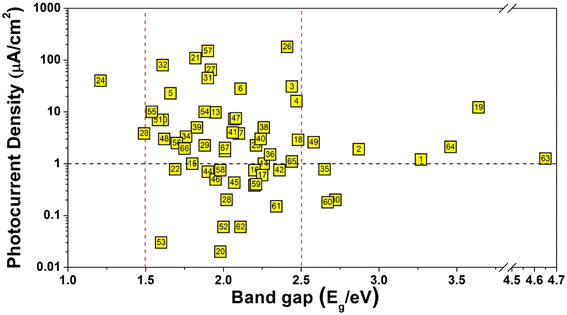 |
| Fig. 17 A comparison of the banggap (Eg/eV) and photocurrent density (μA cm−2) of the multi-component chalcogenidometalates presented in Table 1. | |
(2) Impact of space groups: statistical analysis of Table 1 reveals that materials with high symmetry, such as tetragonal and orthorhombic crystal systems, generally exhibit higher photocurrents compared to those with lower symmetry, like triclinic or monoclinic crystal systems. This can be primarily attributed to the fact that high-symmetry space groups tend to have a regular electron cloud distribution and strong absorption of photons at specific wavelengths, which facilitates the generation of more carriers and enhances the photocurrent response. Furthermore, structures with higher crystalline symmetry typically exhibit stronger electron internally scattering rates, which contribute to higher charge mobility.63 Notably, the cubic crystal system, despite having the highest symmetry, shows a relatively low photocurrent. This can be attributed to the absence of an intrinsic electric field and a directional separation mechanism, both of which are crucial for effectively separating the photogenerated h+/e− and suppressing charge carrier recombination.64 This issue can be addressed through heterojunctions or surface modifications. Therefore, an appropriately symmetrical crystal system can significantly enhance the photocurrent response. Of course, slight differences in the statistical data may stem from the influence of complex factors related to the chalcogenides and deviations caused by the limited sample size.
(3) Effect of elements: compared to group 15 metals, group 14 metal chalcogenides tend to exhibit stronger photocurrent responses. This behavior can be attributed to the periodic trends in atomic radius and electronegativity. As the atomic radius decreases within the same period and electronegativity weakens, the ease of electron escape increases, resulting in a more significant movement of electrons into the external circuit and consequently, larger photocurrents. Chalcogenide elements play a crucial role in the photoelectric properties, as demonstrated by the significantly higher photocurrent densities observed in several selenium-based multi-component chalcogenidometalates, such as Rb2MnSnSe4 (80 μA cm−2), [H3dien][Ag5Sn4Se12]·3H2O (109 μA cm−2), and Cs2CdGeSe4 (180 μA cm−2). Therefore, it is reasonable to hypothesize that tellurium-based multi-component chalcogenidometalates may exhibit even superior photocurrent performance.
(4) Effect of polarization effects: non-centrosymmetric Hg-based chalcogenides demonstrate relatively stronger photocurrent responses, which are not only due to their polar structures but also because of the large polarization effects arising from the Jahn–Teller distortion of Hg. Additionally, the presence of Hg-containing functional motifs significantly influences the band structure, further enhancing the photocurrent response. Moreover, the polar structure could also be obtained in heteranionic structures generated from diverse chemical bonds formed by different anions (O2−, S2−, Cl−etc.).
(5) Impact of anion dimension: the dimensionality of the anion framework appears to have a minimal effect on the photocurrent response. Similarly, complex cations that act as charge- and space-compensating ions do not exhibit a pronounced impact. However, it is noteworthy that compounds with 3D anion structures tend to exhibit superior photocurrent densities. This is due to the more efficient charge transfer within the 3D extended network, as compared to 2D or 0D anions, which may hinder charge mobility.
6 Conclusions and prospects
In conclusion, this review comprehensively summarized the photocurrent response performances of novel chalcogenidometalates synthesized and reported thus far and offer several key conclusions have emerged that may serve as valuable guidance for future research in the field. It is important to highlight that the 67 compounds reviewed here were specifically multi-component metal chalcogenides for which photocurrent response experiments have been conducted. Although a diverse range of novel structural chalcogenides has been synthesized and studied, there remains a significant gap in photocurrent response data for many of these materials. To further accelerate the development of solar energy conversion materials based on chalcogenidometalates, it is crucial to expand the experimental database, not only for newly synthesized materials but also for previously reported multi-component metal chalcogenides. This data is essential for both performance evaluation and theoretical modeling. Moreover, the introduction of new elements or advanced doping strategies into the anionic frameworks of chalcogenidometalates could have synergistic effects on the crystal and electronic structures, enabling the tuning of band gaps and enhancing photocurrent responses. Finally, the discovery of novel chalcogenidometalates with improved photocurrent properties will benefit from rational design of their components and structures, with increased application of theoretical calculations to predict and optimize performance. We hope that this review offers a comprehensive perspective on the photocurrent response properties of multi-component chalcogenidometalates and stimulates further research into the design and development of high-performance solar energy conversion materials.
Author contributions
Chang Liu: supervision, conceptualization, writing – review & editing. Wenjing Tian: investigation, writing – original draft. Mao-Yin Ran: formal analysis, visualization. Pan Gao: formal analysis, writing – review & editing. Panpan Jing: visualization. Yi Liu: writing – review & editing. Hua Lin: conceptualization, writing – review & editing.
Data availability
No primary research results, software or code have been included and no new data were generated or analysed as part of this review.
Conflicts of interest
There are no conflicts to declare.
Acknowledgements
This work was supported by the National Natural Science Foundation of China (Grants No. 52202142, 52171277, and 22175175), the Science and Technology Serving Enterprise Project in University and Colleges of Xi'an Science and Technology Bureau (Grant No. 24GXFW0002), the Doctoral Scientific Research Startup Foundation of Shaanxi University of Science and Technology (2018BJ-07) and Fujian Science & Technology Innovation Laboratory for Optoelectronic Information of China (2021ZR118).
References
-
(a) I. Chung and M. G. Kanatzidis, Metal Chalcogenides: A Rich Source of Nonlinear Optical Materials, Chem. Mater., 2013, 26, 849–869 CrossRef;
(b) F. Liang, L. Kang, Z. Lin, Y. Wu and C. Chen, Analysis and prediction of mid-IR nonlinear optical metal sulfides with diamond-like structures, Coord. Chem. Rev., 2017, 333, 57–70 CrossRef CAS;
(c) H. Chen, M. Y. Ran, W. B. Wei, X. T. Wu, H. Lin and Q. L. Zhu, A comprehensive review on metal chalcogenides with three-dimensional frameworks for infrared nonlinear optical applications, Coord. Chem. Rev., 2022, 470, 214706 CrossRef CAS;
(d) H.-D. Yang, M.-Y. Ran, W.-B. Wei, X.-T. Wu, H. Lin and Q.-L. Zhu, Recent advances in IR nonlinear optical chalcogenides with well-balanced comprehensive performance, Mater. Today Phys., 2023, 35, 101127 CrossRef CAS;
(e) J.-X. Zhang, P. Feng, M.-Y. Ran, X.-T. Wu, H. Lin and Q.-L. Zhu, Ga-based IR nonlinear optical materials: synthesis, structures, and properties, Coord. Chem. Rev., 2024, 502, 215617 CrossRef CAS;
(f) X. Liu, Y.-C. Yang, M.-Y. Li, L. Chen and L.-M. Wu, Anisotropic structure building unit involving diverse chemical bonds: a new opportunity for high-performance second-order NLO materials, Chem. Soc. Rev., 2023, 52, 8699–8720 RSC;
(g) J.-X. Zhang, M.-Y. Ran, X.-T. Wu, H. Lin and Q.-L. Zhu, An overview of Mg-based IR nonlinear optical materials, Inorg. Chem. Front., 2023, 10, 5244–5257 RSC;
(h) W. Zhou and S.-P. Guo, Rational Design of Novel Promising Infrared Nonlinear Optical Materials: Structural Chemistry and Balanced Performances, Acc. Chem. Res., 2024, 57, 648–660 CrossRef CAS PubMed.
-
(a) H. Lin, H. Chen, J.-N. Shen, L. Chen and L.-M. Wu, Chemical Modification and Energetically Favorable Atomic Disorder of a Layered Thermoelectric Material TmCuTe2 Leading to High Performance, Chem. – Eur. J., 2014, 20, 15401–15408 CrossRef CAS PubMed;
(b) H. Lin, G.-J. Tan, J.-N. Shen, S.-Q. Hao, L.-M. Wu, N. Calta, C. Malliakas, S. Wang, C. Uher, C. Wolverton and M. G. Kanatzidis, Concerted Rattling in CsAg5Te3 Leading to Ultralow Thermal Conductivity and High Thermoelectric Performance, Angew. Chem., Int. Ed., 2016, 55, 11431–11436 CrossRef CAS PubMed;
(c) R. Woods-Robinson, Y. Han, H. Zhang, T. Ablekim, I. Khan, K. A. Persson and A. Zakutayev, Wide band gap chalcogenide semiconductors, Chem. Rev., 2020, 120, 4007–4055 CrossRef CAS PubMed;
(d) B. Jiang, Y. Yu, J. Cui, X. Liu, L. Xie, J. Liao, Q. Zhang, Y. Huang, S. Ning and B. Jia, High-entropy-stabilized chalcogenides with high thermoelectric performance, Science, 2021, 371, 830–834 CrossRef CAS PubMed;
(e) A. Giri, G. Park and U. Jeong, Layer-structured anisotropic metal chalcogenides: recent advances in synthesis, modulation, and applications, Chem. Rev., 2023, 123, 3329–3442 CrossRef CAS PubMed;
(f) N. H. Li, Q. Zhang, X. L. Shi, J. Jiang and Z. G. Chen, Silver Copper Chalcogenide Thermoelectrics: Advance, Controversy, and Perspective, Adv. Mater., 2024, 36, 2313246 Search PubMed;
(g) P.-F. Liu, X. Li, J. Li, J. Zhu, Z. Tong, M. Kofu, M. Nirei, J. Xu, W. Yin, F. Wang, T. Liang, L. Xie, Y. Zhang, D. J. Singh, J. Ma, H. Lin, J. Zhang, J. He and B.-T. Wang, Strong low-energy rattling modes enabled liquid-like ultralow thermal conductivity in a well-ordered solid, Natl. Sci. Rev., 2024, 11, nwae216 CrossRef PubMed;
(h) H. Chen, M.-Y. Ran, L.-H. Li, X.-T. Wu and H. Lin, [Cs14Cl][Tm71Se110]: an unusual salt-inclusion chalcogenide containing different valent Tm centers and ultralow thermal conductivity, Chin. J. Struct. Chem., 2024, 43, 100397 CrossRef CAS.
-
(a) L. Malavasi and S. Margadonna, Structure–properties correlations in Fe chalcogenide superconductors, Chem. Soc. Rev., 2012, 41, 3897–3911 RSC;
(b) Q. Si, R. Yu and E. Abrahams, High-temperature superconductivity in iron pnictides and chalcogenides, Nat. Rev. Mater., 2016, 1, 1–15 Search PubMed;
(c) N. Zaki, G. Gu, A. Tsvelik, C. Wu and P. D. Johnson, Time-reversal Symmetry Breaking in the Fe-chalcogenide Superconductors, Proc. Natl. Acad. Sci. U. S. A., 2021, 118, e2007241118 CrossRef CAS PubMed;
(d) R. M. Fernandes, A. I. Coldea, H. Ding, I. R. Fisher, P. J. Hirschfeld and G. Kotliar, Iron pnictides and chalcogenides: a new paradigm for superconductivity, Nature, 2022, 601, 35–44 CrossRef CAS PubMed.
-
(a) M. Enayat, Z. Sun, U. R. Singh, R. Aluru, S. Schmaus, A. Yaresko, Y. Liu, C. Lin, V. Tsurkan and A. Loidl, Real-space imaging of the atomic-scale magnetic structure of Fe1+yTe, Science, 2014, 345, 653–656 CrossRef CAS PubMed;
(b) Y.-J. Zheng, Y.-F. Shi, C.-B. Tian, H. Lin, L.-M. Wu, X.-T. Wu and Q.-L. Zhu, An Unprecedented Pentanary Chalcohalide with the Mn Atoms in Two Chemical Environments: Unique Bonding Characteristics and Magnetic Properties, Chem. Commun., 2019, 55, 79–82 RSC;
(c) S. Licciardello, J. Buhot, J. Lu, J. Ayres, S. Kasahara, Y. Matsuda, T. Shibauchi and N. E. Hussey, Electrical resistivity across a nematic quantum critical point, Nature, 2019, 567, 213–217 CrossRef CAS PubMed;
(d) H. Liu and Y. Xue, van der Waals epitaxial growth and phase transition of layered FeSe2 nanocrystals, Adv. Mater., 2021, 33, 2008456 CrossRef CAS PubMed.
-
(a) C. Coughlan, M. Ibanez, O. Dobrozhan, A. Singh, A. Cabot and K. M. Ryan, Compound copper chalcogenide nanocrystals, Chem. Rev., 2017, 117, 5865–6109 CrossRef CAS PubMed;
(b) Y. Zhu, L. Wang, Y. Liu, L. Shao and X. Xia,
In situ Hydrogenation Engineering of ZnIn2S4 for Promoted visible-light Water Splitting, Appl. Catal., B, 2019, 241, 483–490 CrossRef CAS;
(c) Y. Wang, B. Ren, J.-Z. Ou, K. Xu, C. Yang, Y. Li and H. Zhang, Engineering Two-dimensional Metal Oxides and Chalcogenides for Enhanced Electro- and Photocatalysis, Sci. Bull., 2021, 66, 1228–1252 CrossRef CAS PubMed;
(d) L. Wu, Y. Li, G.-Q. Liu and S.-H. Yu, Polytypic metal chalcogenide nanocrystals, Chem. Soc. Rev., 2024, 53, 9832–9873 RSC.
-
(a) Y.-J. Gao, H.-Y. Sun, J.-L. Li, X.-H. Qi, K.-Z. Du, Y.-Y. Liao, X.-Y. Huang, M.-L. Feng and M. G. Kanatzidis, Selective Capture of Ba2+, Ni2+, and Co2+ by a Robust Layered Metal Sulfide, Chem. Mater., 2020, 32, 1957–1963 CrossRef CAS;
(b) W.-A. Li, J.-R. Li, B. Zhang, H.-Y. Sun, J.-C. Jin, X.-Y. Huang and M.-L. Feng, Layered Thiostannates with Distinct Arrangements of Mixed Cations for the Selective Capture of Cs+, Sr2+, and Eu3+ Ions, ACS Appl. Mater. Interfaces, 2021, 13, 10191–10201 CrossRef CAS PubMed;
(c) C. Gu, H.-M. Xu, S.-K. Han, M.-R. Gao and S.-H. Yu, Soft chemistry of metastable metal chalcogenide nanomaterials, Chem. Soc. Rev., 2021, 50, 6671–6683 RSC;
(d) K. Saito, M. Morita, T. Okada and M. Ogawa, Designed functions of oxide/hydroxide nanosheets via elemental replacement/doping, Chem. Soc. Rev., 2024, 53, 10523–10574 RSC.
-
(a) S.-L. Ju, W. Bai, L.-M. Wu, H. Lin, C. Xiao, S.-T. Cui, Z. Li, S. Kong, Y. Liu, D.-Y. Liu, G.-B. Zhang, Z. Sun and Y. Xie, Evidence for Itinerant Carriers in Anisotropic Narrow-gap Semiconductor by Angle-Resolved Photoemission Spectroscopy, Adv. Mater., 2018, 30, 1704733 CrossRef PubMed;
(b) R. Woods-Robinson, Y. Han, H. Zhang, T. Ablekim, I. Khan, K. A. Persson and A. Zakutayev, Wide Band Gap Chalcogenide Semiconductors, Chem. Rev., 2020, 120, 4007–4055 CrossRef CAS PubMed;
(c) Y. Wang, S. R. Kavanagh, I. Burgués-Ceballos, A. Walsh, D. O. Scanlon and G. Konstantatos, Cation Disorder Engineering Yields AgBiS2 Nanocrystals with Enhanced Optical Absorption for Efficient Ultrathin Solar Cells, Nat. Photonics, 2022, 16, 235–241 CrossRef CAS;
(d) H. Yang, Z. Ma and Q. Wang, Shortwave-Infrared Silver Chalcogenide Quantum Dots for Optoelectronic Devices, ACS Nano, 2024, 18, 30123–30131 CrossRef CAS PubMed;
(e) W. Jiang, S. Wu, D. Xu, L. Tu, Y. Xie, P. Pasqués-Gramage, P. G. Boj, M. A. Díaz-García, F. Li and J. Wu, Stable Xanthene Radicals and Their Heavy Chalcogen Analogues Showing Tunable Doublet Emission from Green to Near-infrared, Angew. Chem., Int. Ed., 2025, 138, e202418762 Search PubMed.
- D. Paul and D. Devaprakasam, A Novel Approach to Enhance Performance, Stability and Longevity of the Solar Cells by Mitigating UV-induced Degradation with Monolayer-Boosted Nano-TiO2 Coatings, Sol. Energy, 2025, 285, 113118 CrossRef CAS.
- K. Hashimoto, H. Irie and A. Fujishima, TiO2 Photocatalysis: A Historical Overview and Future Prospects, Jpn. J. Appl. Phys., 2005, 44, 8269 CrossRef CAS.
- H. P. Maruska and A. K. Ghosh, Photocatalytic Decomposition of Water at Semiconductor Electrodes, Sol. Energy, 1978, 20, 443–458 CrossRef CAS.
- K. van Benthem, C. Elsässer and R. French, Bulk Electronic Structure of SrTiO3: Experiment and Theory, J. Appl. Phys., 2001, 90, 6156–6164 CrossRef CAS.
- X. Y. Song, W. Q. Li, D. He, H. G. Wu, Z. J. Ke, C. Z. Jiang, G. M. Wang and X. H. Xiao, The “Midas Touch” transformation of TiO2 nanowire arrays during visible light photoelectrochemical performance by carbon/nitrogen coimplantation, Adv. Energy Mater., 2018, 8, 1800165 CrossRef.
- A. Paliwal, K. P. S. Zanoni, C. Roldán-Carmona, N. Rodkey and H. J. Bolink, Vacuum-Deposited Bifacial Perovskite Solar Cells, ACS Energy Lett., 2024, 9, 4587–4595 CrossRef CAS PubMed.
- C. S. Ferekides, U. Balasubramanian, R. Mamazza, V. Viswanathan, H. Zhao and D. L. Morel, CdTe thin film solar cells: device and technology issues, Sol. Energy, 2004, 77, 823–830 CrossRef CAS.
- T. Zhang, Y. Yang, D. Liu, S. C. Tse, W. Cao, Z. Feng, S. Chen and L. Qian, High efficiency solution-processed thin-film Cu(In,Ga)(Se,S)2 solar cells, Energy Environ. Sci., 2016, 9, 3674–3681 RSC.
- L.-P. Yu and A. Zunger, Identification of Potential Photovoltaic Absorbers Based on First-Principles Spectroscopic Screening of Materials, Phys. Rev. Lett., 2012, 108, 068701 CrossRef PubMed.
- M.-S. Hybertsen and S.-G. Louie, First-principles Theory of Quasiparticles: Calculation of Band Gaps in Semiconductors and Insulators, Phys. Rev. Lett., 1985, 55, 1418–1421 CrossRef CAS PubMed.
- H.-Y. Luo, J. Zhou and S. Cao, The Rare Examples of Chalcogenogermanates Combined with Trivalent Vanadium Complexes, Dalton Trans., 2019, 48, 10907–10914 RSC.
- S. Cao, Y. Chen, X. Liu, J. Zhou and J. Liu, The First Selenidostannate Directed by Low-Valent Vanadium(II) Complex: Photocurrent Response and Magnetic Properties, Inorg. Chem. Commun., 2021, 133, 108862 CrossRef CAS.
- X. Tian, G. Teri, M. Shele, E. Namila, L. Qi, M. Liu and M.-H. Baiyin, Five New TM-Sn-Q (TM = Transition Metals; Q = S, Se) Photoelectrocatalytic Materials, Polyhedron, 2022, 217, 115729 CrossRef CAS.
- M. Shele, X. Tian and M. Baiyin, Solvothermal Synthesis and Properties of Three Antimony Chalcogenides Containing Transition Metal Zinc, J. Solid State Chem., 2021, 302, 122401 CrossRef CAS.
- L. Zhang, H. Zhao, X. Liu, G. Teri and M. Baiyin, Syntheses, Crystal Structure, and Photoelectric Properties of Two Zn-Based Chalcogenidoantimonates Zn–Sb–Q (Q = S, Se), Phys. Chem. Chem. Phys., 2023, 25, 29709–29717 RSC.
- L. Zhang, N. Li, G. Teri, M. Shele, E. Namila, S. Bai and M. Baiyin, Syntheses, Crystal Structure and Properties of Two Chalcogenidoantimonates Mn(En)3Sb2S4 and Co(En)3Sb2Se4, Polyhedron, 2023, 241, 116470 CrossRef CAS.
- J. Tang, X. Wang, P. Sun, J. Wu, J. Li, Z. Wang and T. Wu, The First Observation That Metal Chalcogenide Supertetrahedral Cluster Is Corner-Coordinated by Neutral Amine Group, J. Solid State Chem., 2022, 308, 122935 CrossRef CAS.
- X. Huang, S.-H. Yang, W. Liu and S.-P. Guo, Ba3HgGa2S7: A Zero-Dimensional Quaternary Sulfide Featuring a Unique [Hg2Ga4S14]12− String and Exhibiting a High Photocurrent Response, Inorg. Chem., 2022, 61, 12954–12958 CrossRef CAS PubMed.
- Y. Liu, Y. Li, J. Zhao, R. Zhang, M. Ji, Z. You and Y. An, Solvothermal Syntheses, Characterizations and Semiconducting Properties of Four Quaternary Thioargentates Ba2AgInS4, Ba3Ag2Sn2S8, BaAg2MS4 (M = Sn, Ge), J. Alloys Compd., 2020, 815, 152413 CrossRef CAS.
- Y. Tian, J. Zhang, W. Wang and D. Jia, Ternary 3D Framework [Ag5Sn4Se12]3−: Solvothermal Synthesis, Crystal Structure and Photoelectric Properties of an Organic Silver-Rich Selenidostannate Hybrid, Inorg. Chem. Commun., 2021, 131, 108799 CrossRef CAS.
- Y. Zhang, D. Hu, H. Yang, J. Lin and T. Wu, Synthesis, Crystal Structure, near-IR Photoelectric Response of Two 1-D Selenides: [Cu2MSe5]·[Mn(H+-En)2(En)] (M = Ge, Sn), J. Solid State Chem., 2017, 251, 61–64 CrossRef CAS.
- K.-Z. Du, X.-H. Qi, M.-L. Feng, J.-R. Li, X.-Z. Wang, C.-F. Du, G.-D. Zou, M. Wang and X.-Y. Huang, Synthesis, Structure, Band Gap, and Near-Infrared Photosensitivity of a New Chalcogenide Crystal, (NH4)4Ag12Sn7Se22, Inorg. Chem., 2016, 55, 5110–5112 CrossRef CAS PubMed.
- C. Cui, H. Pan, L. Wang and M.-H. Baiyin, Transition Metal Germanium Chalcogenide Materials: Solvothermal Syntheses, Flexible Crystal, Structures, and Photoelectric Response Property, Cryst. Growth Des., 2024, 24, 1227–1234 CrossRef CAS.
- Y. Li, X. Song, Y. Liu, Y. Guo, Y. Sun, M. Ji, Z. You and Y. An, Syntheses, Structures, and Photocatalytic Properties of Open-Framework Ag-Sn-S Compounds, Dalton Trans., 2020, 49, 11708–11714 RSC.
- G. Teri, X. Liu, N. Li, M. Shele, E. Namila, L. Qi, S. Bai and M. Baiyin, Strong Photoelectric Properties of Mnerties of Open-Framework Ag-Sn-S Compounds Crystal, (NHRich Selenidostann, Eur. J. Inorg. Chem., 2023, 26, e202200607 CrossRef CAS.
- G. Teri, N. Li, S. Bai, E. Namila and M.-H. Baiyin, Synthesis, Crystal Structure, Photocatalysis, Photocurrent Response: One-Dimensional K2HgSnSe4 and Three-Dimensional Na6Cu8Sn3Se13, CrystEngComm, 2021, 23, 6079–6085 RSC.
- Y. Li, X. Song, Y. Zhong, Y. Guo, M. Ji, Z. You and Y. An, Temperature Controlling Valance Changes of Crystalline Thioarsenates and Thioantimonates, J. Alloys Compd., 2021, 872, 159591 CrossRef CAS.
- C. Liu, H.-D. Yang, P.-P. Hou, Y. Xiao, Y. Liu and H. Lin, Cs3CuAs4Q8(Q = S, Se): Unique Two-Dimensional Layered Inorganic Thioarsenates with the Lowest Cu-to-As Ratio and Remarkable Photocurrent Responses, Dalton Trans., 2022, 51, 904–909 RSC.
- C. Zhang, S.-H. Zhou, Y. Xiao, H. Lin and Y. Liu, Interesting Dimensional Transition through Changing Cations as the Trigger in Multinary Thioarsenates Displaying Variable Photocurrent Response and Optical Anisotropy, Inorg. Chem. Front., 2022, 9, 5820–5827 RSC.
- X. Chen, S.-H. Zhou, C. Zhang, H. Lin and Y. Liu, A Novel Bifunctional Thioarsenate Based on Unprecedented Molecular [Cd4As8Se16(Se2)2]8− Cluster Anions, Chem. Commun., 2023, 59, 12124–12127 RSC.
- J. Tang, F. Liang, W. Xing, C. Tang, W. Yin, B. Kang and J. Deng, AgMAsS3 (M = Cd, Hg): Double d10 Cation-Containing Thioarsenites(III) Exhibiting High Photocurrent Response and Moderate Second Harmonic Generation, Inorg. Chem., 2023, 62, 14739–14747 CrossRef CAS PubMed.
- H. Wang, J.-M. Yu, N. Wang, L.-L. Xiao, J.-P. Yu, Q. Xu, B. Zheng, F.-F. Cheng and W.-W. Xiong, Two Silver Chalcogenidoantimonates Synthesized in Piperazine and Their High Performances for Visible-Light Driven Cr(VI) Reduction, J. Solid State Chem., 2021, 300, 122276 CrossRef CAS.
- N. Li, L. Zhang, J. Chen, G. Teri, M. Shele, E. Namila, S. Bai and M. Baiyin, Two New Gallium(III)-Thioantimonates TM(Tren)GaSbS4 (TM = Mn, Fe): Syntheses, Crystal Structure and Properties, Inorg. Chem. Commun., 2022, 146, 110214 CrossRef CAS.
- L. Zhang, F. Qi, M. Shele, E. Namila, L. Qi and M.-H. Baiyin, Solvothermal Synthesis and Characterization of One-Dimensional [Mn(En)3]CdSb2Se5, J. Chem. Crystallogr., 2023, 53, 145–151 CrossRef CAS.
- Y. Xiao, M.-M. Chen, Y.-Y. Shen, P.-F. Liu, H. Lin and Y. Liu, A3Mn2Sb3S8 (A = K and Rb): A New Type of Multifunctional Infrared Nonlinear Optical Material Based on Unique Three-Dimensional Open Frameworks, Inorg. Chem. Front., 2021, 8, 2835–2843 RSC.
- C. Liu, P. Hou, W. Chai, J. Tian, X. Zheng, Y. Shen, M. Zhi, C. Zhou and Y. Liu, Hydrazine-Hydrothermal Syntheses, Characterizations and Photoelectrochemical Properties of Two Quaternary Chalcogenidoantimonates(III): BaCuSbQ3 (Q = S, Se), J. Alloys Compd., 2016, 679, 420–425 CrossRef CAS.
- Y. Xiao, S.-H. Zhou, R. Yu, Y. Shen, Z. Ma, H. Lin and Y. Liu, Rb2CuSb7S12: Quaternary Antimony-Rich Semiconductor Featuring a Three-Dimensional Open Framework and Exhibiting an Intriguing Photocurrent Response, Inorg. Chem., 2021, 60, 9263–9267 CrossRef CAS PubMed.
- R. Wang, X. Zhang, J. He, C. Zheng, J. Lin and F. Huang, Synthesis, Crystal Structure, Electronic Structure, and Photoelectric
Response Properties of KCu2SbS3, Dalton Trans., 2016, 45, 3473–3479 RSC.
- X. Zhang, J. He, R. Wang, K. Bu, J. Li, C. Zheng, J. Lin and F. Huang, Enhancement of Solar Energy Absorption and Optoelectronic Properties of SrCuSbS3 by Lead Doping, Sol. RRL, 2018, 2, 1800021 CrossRef.
- Y. Liu, L. Geng, Z. Xue, Z. Song, D. Xuan, S. Song and Z. Zhao, Syntheses, Crystal Structures, Photocatalysis, and Photoelectric Responses of Quaternary Sulfides ACuZnS2 (A = K, Rb, Cs), Inorg. Chem. Commun., 2022, 146, 110108 CrossRef CAS.
- Y. Liu, X. Song, Y. Guo, Y. Zhong, Y. Li, Y. Sun, M. Ji, Z. You and Y. An, Mild Solvothermal Syntheses and Characterizations of Two Layered Sulfides Ba2Cu2Cd2S5 and Ba3Cu4Hg4S9, J. Alloys Compd., 2020, 829, 154586 CrossRef CAS.
- M. Y. Li, X. Y. Xie, X. T. Wu, X. F. Li and H. Lin, Quaternary Selenophosphate Cs2ZnP2Se6 Featuring Unique One-dimensional Chains and Exhibiting Remarkable Photo-electrochemical Response, Chin. J. Struct. Chem., 2021, 40, 246–255 CAS.
- H. Lin, J. N. Shen, W. W. Zhu, Y. Liu, X. T. Wu, Q. L. Zhu and L. M. Wu, Two new phases in the ternary RE–Ga–S systems with unique interlinkage of GaS4 building units: synthesis, structure, and properties, Dalton Trans., 2017, 46, 13731–13738 RSC.
- Y. F. Shi, X. F. Li, Y. X. Zhang, H. Lin, Z. J. Ma, L. M. Wu, X. T. Wu and Q. L. Zhu, [(Ba19Cl4)(Ga6Si12O42S8)]: a Two-Dimensional Wide-Band-Gap Layered Oxysulfide with Mixed-Anion Chemical Bonding and Photocurrent Response, Inorg. Chem., 2019, 58, 6588–6592 CrossRef CAS PubMed.
- W.-F. Zhou, W.-D. Yao, R.-L. Tang, G. Xue and S.-P. Guo, Second-order Nonlinear Optical and Photoelectric Properties of Zn4B6O12S, J. Alloys Compd., 2021, 867, 158879 CrossRef CAS.
- S. Al Bacha, S. Saitzek, P. Roussel, M. Huvé, E. E. McCabe and H. Kabbour, Low Carrier Effective Masses in Photoactive Sr2Sb2O2Q3 (Q = S, Se): The Role of the Lone Pair, Chem. Mater., 2023, 35, 9528–9541 CrossRef CAS.
- S. Al Bacha, S. Saitzek, E. E. McCabe and H. Kabbour, Photocatalytic and Photocurrent Responses to Visible Light of the Lone-Pair-Based Oxysulfide Sr6Cd2Sb6S10O7, Inorg. Chem., 2022, 61, 18611–18621 CrossRef CAS PubMed.
- D. Wong, P. Lee, S. Gao, X. Wang, H. Y. Qi and F. S. Kit, The photoelectric effect: experimental confirmation concerning a widespread misconception in the theory, Eur. J. Phys., 2011, 32, 1059 CrossRef.
- J. Cen, Q. Wu, M. Liu and A. Orlov, Developing new understanding of photoelectrochemical water splitting via in-situ techniques: A review on recent progress, Green Energy Environ., 2017, 2, 100–111 CrossRef.
- W. Zhang, J. Ma, L. Xiong, H.-Y. Jiang and J. Tang, Well-Crystallized α-FeOOH Cocatalysts Modified BiVO4 Photoanodes for Efficient and Stable Photoelectrochemical Water Splitting, ACS Appl. Energy Mater., 2020, 3, 5927–5936 CrossRef CAS.
-
(a) Y.-Y. Li, B.-X. Li, G. Zhang, L.-J. Zhou, H. Lin, J.-N. Shen, C.-Y. Zhang, L. Chen and L.-M. Wu, Syntheses, Characterization, and Optical Properties of Centrosymmetric Ba3(BS3)1.5(MS3)0.5 and Noncentrosymmetric Ba3(BQ3)(SbQ3), Inorg. Chem., 2015, 54, 4761–4767 CrossRef CAS PubMed;
(b) H. Lin, Y. Y. Li, M. Y. Li, Z. J. Ma, L. M. Wu, X. T. Wu and Q. L. Zhu, Centric-to-acentric structure transformation induced by a stereochemically active lone pair: a new insight for design of IR nonlinear optical materials, J. Mater. Chem. C, 2019, 7, 4638–4643 RSC;
(c) C. Liu, Y. Xiao, H. Wang, W. Chai, X. Liu, D. Yan, H. Lin and Y. Liu, One-Dimensional Chains in Pentanary Chalcogenides A2Ba3Cu2Sb2S10 (A = K, Rb, Cs) Displaying a Photocurrent Response, Inorg. Chem., 2020, 59, 1577–1581 CrossRef CAS PubMed;
(d) M. Yan, H.-G. Xue and S.-P. Guo, Recent Achievements in Lone-Pair Cation-Based Infrared Second-Order Nonlinear Optical Materials, Cryst. Growth Des., 2021, 21, 698–720 CrossRef CAS;
(e) C. Liu, S.-H. Zhou, Y. Xiao, C. Zhang, H. Lin and Y. Liu, Aliovalent-cation-substitution-induced structure transformation: a new path toward high-performance IR nonlinear optical materials, J. Mater. Chem. C, 2021, 9, 15407–15414 RSC;
(f) M.-M. Chen, Z. Ma, B.-X. Li, W.-B. Wei, X.-T. Wu, H. Lin and Q.-L. Zhu, M2As2Q5 (M = Ba, Pb; Q = S, Se): A source of infrared nonlinear optical materials with excellent overall performance activated by multiple discrete arsenate anions, J. Mater. Chem. C, 2021, 9, 1156–1163 RSC;
(g) Y. Xiao, M. M. Chen, Y. Y. Shen, P. F. Liu, H. Lin and Y. Liu, A3Mn2Sb3S8 (A = K, Rb): A new type of multifunctional infrared nonlinear optical materials based on unique three-dimensional open frameworks, Inorg. Chem. Front., 2021, 8, 2835–2843 RSC;
(h) H.-J. Zhao, H.-D. Yang, P.-F. Liu and H. Lin, From Cc to P63mc: Structural Variation in La3S2Cl2[SbS3] and La3OSCl2[SbS3] Induced by the Isovalent Anion Substitution, Cryst. Growth Des., 2022, 22, 1437–1444 CrossRef CAS;
(i) F. Xu, X. Xu, B. Li, G. Zhang, C. Zheng, J. Chen and N. Ye, Hg3AsS4X (X = Cl and Br): two Hg-based chalcogenides as long-wave infrared nonlinear optical crystals with superior comprehensive performances, Inorg. Chem. Front., 2024, 11, 2105–2115 RSC;
(j) Y. Zhou, L.-T. Jiang, X.-M. Jiang, B.-W. Liu and G.-C. Guo, Mixed-anion square-pyramid [SbS3I2] units causing strong second-harmonic generation intensity and large birefringence, Chin. Chem. Lett., 2024, 35, 109740 Search PubMed.
-
(a) M. Y. Li, Z. J. Ma, B. X. Li, X. T. Wu, H. Lin and Q. L. Zhu, HgCuPS4: An Exceptional Infrared Nonlinear Optical Material with Defect Diamond-like Structure, Chem. Mater., 2020, 32, 4331–4339 CrossRef CAS;
(b) Y. Chu, P. Wang, H. Zeng, S. Cheng, X. Su, Z. Yang, J. Li and S. Pan, Hg3P2S8: A New Promising Infrared Nonlinear Optical Material with a Large Second-Harmonic Generation and a High Laser-Induced Damage Threshold, Chem. Mater., 2021, 33, 6514–6521 CrossRef CAS;
(c) Y. Chu, H. Wang, T. Abutukadi, Z. Li, M. Mutailipu, X. Su, Z. Yang, J. Li and S. Pan, Zn2HgP2S8: A Wide Bandgap Hg-Based Infrared Nonlinear Optical Material with Large Second-Harmonic Generation Response, Small, 2023, 19, 2305074 CrossRef CAS PubMed;
(d) Y. Chu, H. Wang, Q. Chen, X. Su, Z. Chen, Z. Yang, J. Li and S. Pan, “Three-in-One”: A New Hg-Based Selenide Hg7P2Se12 Exhibiting Wide Infrared Transparency Range and Strong Nonlinear Optical Effect, Adv. Funct. Mater., 2024, 34, 2314933 CrossRef CAS;
(e) A.-Y. Wang, S.-H. Zhou, M.-Y. Ran, X.-T. Wu, H. Lin and Q.-L. Zhu, Regulating the Key Performance Parameters for Hg-based IR NLO Chalcogenides via Bandgap Engineering Strategy, Chin. Chem. Lett., 2024, 35, 109377 CrossRef CAS;
(f) M.-Y. Ran, S.-H. Zhou, W.-B. Wei, B.-X. Li, X.-T. Wu, H. Lin and Q.-L. Zhu, Breaking through the Trade-Off between Wide Band Gap and Large SHG Coefficient in Mercury-based Chalcogenides for IR Nonlinear Optical Application, Small, 2024, 20, 2304563 CrossRef CAS PubMed.
-
(a) S.-P. Guo, Y. Chi and G.-C. Guo, Recent Achievements on Middle and Far-Infrared Second-Order Nonlinear Optical Materials, Coord. Chem. Rev., 2017, 335, 44–57 CrossRef CAS;
(b) M.-M. Chen, H.-G. Xue and S.-P. Guo, Multinary Metal Chalcogenides with Tetrahedral Structures for Second-Order Nonlinear Optical, Photocatalytic, and Photovoltaic Applications, Coord. Chem. Rev., 2018, 368, 115–133 CrossRef CAS; H. Chen, W.-B. Wei, H. Lin and X.-T. Wu, Transition-metal-based chalcogenides: A rich source of infrared nonlinear optical materials, Coord. Chem. Rev., 2021, 448, 214154 Search PubMed;
(c) Y. K. Kang, H. Lee, T. D. C. Ha, J. K. Won, H. Jo, K. M. Ok, S. Ahn, B. Kang, K. Ahn, Y. Oh and M.-G. Kim, Thiostannate Coordination Transformation-Induced Self-Crosslinking Chalcogenide Aerogel with Local Coordination Control and Effective Cs+ Remediation Functionality, J. Mater. Chem. A, 2020, 8, 3468–3480 RSC;
(d) P. Feng, J.-X. Zhang, M.-Y. Ran, X.-T. Wu, H. Lin and Q. L. Zhu, Rare-earth-based chalcogenides and their derivatives: an encouraging IR nonlinear optical material candidate, Chem. Sci., 2024, 15, 5869–5896 RSC.
-
(a) R. Wang, F. Liang, F. Wang, Y. Guo, X. Zhang, Y. Xiao, K. Bu, Z. Lin, J. Yao, T. Zhai and F. Q. Huang, Sr6Cd2Sb6O7S10: Strong SHG Response Activated by Highly Polarizable Sb/O/S Groups, Angew. Chem., Int. Ed., 2019, 58, 8078–8081 CrossRef CAS PubMed;
(b) Y.-F. Shi, W.-B. Wei, X.-T. Wu, H. Lin and Q.-L. Zhu, Recent progress in oxychalcogenides as IR nonlinear optical materials, Dalton Trans., 2021, 50, 4112–4118 RSC;
(c) H. Yan, Y. Matsushita, K. Yamaura and Y. Tsujimoto, La3Ga3Ge2S3O10: An Ultraviolet Nonlinear Optical Oxysulfide Designed by Anion-Directed Band Gap Engineering, Angew. Chem., Int. Ed., 2021, 60, 26561–26565 CrossRef CAS PubMed;
(d) Y. Zhang, H. Wu, Z. Hu and H. Yu, Oxychalcogenides: A Promising Class of Materials for Nonlinear Optical Crystals with Mixed-Anion Groups, Chem. – Eur. J., 2022, 29, e202203597 CrossRef PubMed;
(e) M.-Y. Ran, S.-H. Zhou, B.-X. Li, W.-B. Wei, X.-T. Wu, H. Lin and Q.-L. Zhu, Enhanced Second-Harmonic-Generation Efficiency and Birefringence in Melillite Oxychalcogenides Sr2MGe2OS6 (M = Mn, Zn, and Cd), Chem. Mater., 2022, 34, 3853–3861 CrossRef CAS;
(f) R. Wang, F. Liang, X. Liu, Y. Xiao, Q. Liu, X. Zhang, L.-M. Wu, L. Chen and F. Huang, Heteroanionic Melilite Oxysulfide: A Promising Infrared Nonlinear Optical Candidate with a Strong Second-Harmonic Generation Response, Sufficient Birefringence, and Wide Bandgap, ACS Appl. Mater. Interfaces, 2022, 14, 23645–23652 CrossRef CAS PubMed;
(g) Y.-F. Shi, Z. Ma, B.-X. Li, X.-T. Wu, H. Lin and Q.-L. Zhu, Phase matching achieved by isomorphous substitution in IR nonlinear optical material Ba2SnSSi2O7 with an undiscovered [SnO4S] functional motif, Mater. Chem. Front., 2022, 6, 3054–3061 RSC;
(h) M.-Y. Ran, S.-H. Zhou, W.-B. Wei, B.-X. Li, X.-T. Wu, H. Lin and Q.-L. Zhu, Rational Design of a Rare-Earth Oxychalcogenide Nd3[Ga3O3S3][Ge2O7] with Superior Infrared Nonlinear Optical Performance, Small, 2023, 19, 2300248 CrossRef CAS PubMed;
(i) M.-Y. Ran, S.-H. Zhou, X.-T. Wu, H. Lin and Q.-L. Zhu, The first Hg-based oxychalcogenide Sr2HgGe2OS6: achieving balanced IR nonlinear optical properties through synergistic cation and anion substitution, Mater. Today Phys., 2024, 44, 101442 CrossRef CAS;
(j) M.-Y. Ran, S.-H. Zhou, B.-X. Li, X.-T. Wu, H. Lin and Q.-L. Zhu, Balanced IR Nonlinear Optical Performance Achieved by Cation–Anion Module Co-Substitution in V-Based Salt-Inclusion Oxychalcogenides, Chem. Mater., 2024, 36, 11996–12005 CrossRef CAS.
-
(a) H. Lin, W.-B. Wei, H. Chen, X.-T. Wu and Q.-L. Zhu, Rational design of infrared nonlinear optical chalcogenides by chemical substitution, Coord. Chem. Rev., 2020, 406, 213150 CrossRef CAS;
(b) G. Zou and K. M. Ok, Novel ultraviolet (UV) nonlinear optical (NLO) materials discovered by chemical substitution-oriented design, Chem. Sci., 2020, 11, 5404–5409 RSC;
(c) M.-Y. Ran, A. Y. Wang, W.-B. Wei, X.-T. Wu, H. Lin and Q.-L. Zhu, Recent progress in the design of IR nonlinear optical materials by partial chemical substitution: Structural evolution and performance optimization, Coord. Chem. Rev., 2023, 481, 215059 CrossRef CAS.
-
(a) Y. Z. Pei, X. Y. Shi, A. LaLonde, H. Wang, L. D. Chen and G. J. Snyder, Convergence of electronic bands for high performance bulk thermoelectrics, Nature, 2011, 473, 66–69 CrossRef CAS PubMed;
(b) Y. Y. Li, Y. Diao, X. Y. Wang, X. F. Tian, Y. Hu, B. Zhang and D. F. Yang, Zn4B6O13: Efficient Borate Photocatalyst with Fast Carrier Separation for Photodegradation of Tetracycline, Inorg. Chem., 2020, 59, 13136–13143 CrossRef CAS PubMed.
-
(a) Z. L. Li, Z. Q. Li, C. L. Zuo and X. S. Fang, Application of Nanostructured TiO2 in UV Photodetectors: A Review, Adv. Mater., 2022, 34, 2109083 CrossRef CAS PubMed;
(b) H. T. Huang, J. Wang, Y. Liu, M. Y. Zhao, N. S. Zhang, Y. F. Hu, F. T. Fan, J. Y. Feng, Z. S. Li and Z. G. Zou, Stacking textured films on lattice-mismatched transparent conducting oxides via matched Voronoi cell of oxygen sublattice, Nat. Mater., 2024, 23, 383–390 CrossRef CAS PubMed.
|
This journal is © the Partner Organisations 2025 |
Click here to see how this site uses Cookies. View our privacy policy here.