DOI:
10.1039/D4QO01828A
(Review Article)
Org. Chem. Front., 2025,
12, 328-345
Convergent synthetic approaches to polycyclic aromatic dicarboximides
Received
30th September 2024
, Accepted 11th November 2024
First published on 12th November 2024
Abstract
Polycyclic aromatic dicarboximides (PADIs) as represented by naphthalene and perylene bis(dicarboximides) are useful molecules for a variety of functional materials and remain important synthetic targets for organic chemists. In this review, we summarize recent developments in the convergent synthetic approaches using palladium-catalysed annulation of peri-activated naphthalimides. Contrary to conventional linear synthetic methods where a single starting material is converted into a target molecule through a stepwise manner, the herein discussed convergent synthetic method enables rapid extension of the π-scaffold by combining multiple polyaromatic substrates into one. This method opened new possibilities for π-extended dicarboximides with so far up to five imide groups. Further exploration of annulation strategies may allow the preparation of previously inaccessible PADIs with fascinating molecular and material properties.
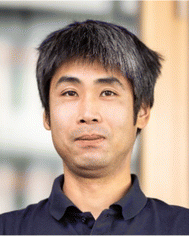 Kazutaka Shoyama | Kazutaka Shoyama received his chemistry education at the University of Tokyo, Japan, where he obtained his MSc and PhD degrees in 2013 and 2016, respectively, under the supervision of Prof. Eiichi Nakamura. Afterwards he worked as a postdoctoral fellow and later as a research associate with Prof. Frank Würthner at the University of Würzburg, Germany, focusing on the synthesis of π-extended polycyclic aromatic dicarboximides and being responsible for crystallographic analyses at the synchrotron facility. In 2024, he began his independent carrier at the University of Würzburg. His current research interests include the design and synthesis of crystalline π-conjugated molecules and their solid-state chemistry. |
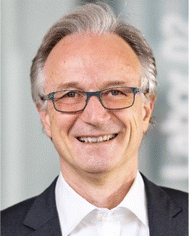 Frank Würthner | Frank Würthner received his education at the University of Stuttgart, Germany and at MIT in Cambridge, MA, USA. In 1995 he started his independent research on functional dyes and supramolecular dye chemistry at BASF in Ludwigshafen and the University of Ulm. In 2002, he became a professor at the University of Würzburg. His research interests include the synthesis of novel π-scaffolds and their application in organic electronics, the construction of complex supramolecular architectures, the mechanistic elucidation of self-assembly processes, and the investigation of light-induced processes in dye-based nanosystems. He has published >600 papers and has been listed since 2014 as a highly cited researcher. He is an elected member of the National Academy of Sciences Leopoldina, Germany and serves since 2015 in the editorial board of Organic Chemistry Frontiers. |
10th anniversary statement of Frank Würthner
As an Associate Editor of Organic Chemistry Frontiers (https://doi.org/10.1039/C5QO90034D) for the fields of supramolecular chemistry and functional materials from 2015 to 2024, I had the great pleasure to contribute to the development of the journal in its first decade. It is a great satisfaction for me that the journal has accomplished its goal to become a leading and indispensable one in the field of organic chemistry. This became possible by the strong commitment of the whole editorial team led by Prof. Shengming Ma together with executive editors Dr Daping Zhang and Dr Wenjun Liu, and excellent personal relationships established during annual board meetings. The latter taking place together with OCF symposia in Oxford, Kunming, Lanzhou, and Changwon were unforgettable experiences for me. Other particularly enjoyable tasks were the handling of two themed issues on novel π-electron molecular scaffolds (2016, https://doi.org/10.1039/C7QO90019H) and in celebration of the 75th birthday of Julius Rebek, Jr., my postdoctoral supervisor (2019, https://doi.org/10.1039/C9QO90040C), as well as publishing my own research, e.g. our highly cited review on perylene dyes (https://doi.org/10.1039/C8QO01368C). I wish the journal success and further growth, and will continue to contribute as an author, guest editor of special issues, and editorial board member.
|
1. Introduction
Polycyclic aromatic dicarboximides (PADIs) are a class of organic molecules composed of a π-conjugated core structure and one or more dicarboximide (–C(
O)–N(R)–C(
O)–) moieties.1–4 In principle, these imide groups can be attached to polycyclic aromatic hydrocarbons (PAHs) either as five or as six-membered rings. In this review, we focus on six-membered rings and structures obtained from naphthalene dicarboximide units (Fig. 1a). With the capability of being reducible at modest negative potential, naphthalene dicarboximides play an important role as subunits of n-type organic semiconductors applied in organic transistors and solar cells, in battery materials, as organic radical anions or in photoredox catalysis. When being annulated to π-scaffolds such as PAHs, they endow them with unique molecular properties such as long-wavelength absorption/fluorescence, and (multiple) reversible redox processes in the range of interest for many applications. In Fig. 1b this is exemplified by the archetype molecule of the PADI family, the perylene bis(dicarboximide) (PBI).5 Thus, by means of two imide substituents attached to the peri-positions of perylene, the electronic structure of perylene changes from being easy to oxidize to being easy to reduce. For the solid state, this change leads to the transition from p-type to n-type organic semiconductor materials. As an important asset, the delocalization of the negative charge between the two carbonyl groups in the dicarboximide subunit and the aromatic scaffolds provides rather stable radical anions.6 These favourable electronic properties of PADIs, together with the decrease of the optical gap and gain in oscillator strength imparted by the imide units as exemplified in Fig. 1b, established a whole class of colorants with excellent thermal, chemical and photochemical stability.7 As all these favourable properties are related to the electron-withdrawing character imparted by the imide units to these molecules it is accordingly highly warranted to consider the expansion of this class of functional molecules towards larger nanographenes8–10 equipped with multiple imide subunits.
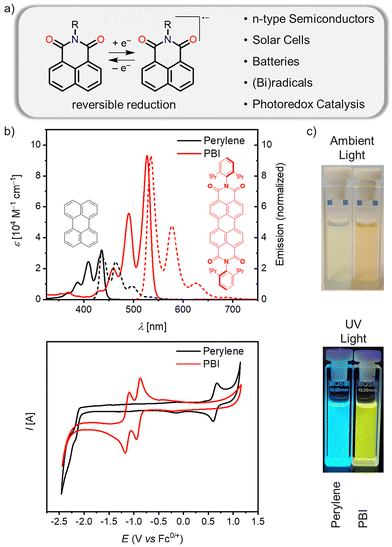 |
| Fig. 1 (a) Chemical structure of naphthalene dicarboximide as the function-imparting unit of all PADIs. (b) The UV/Vis absorption and luminescence spectra, and cyclic voltammetry traces of perylene and PBI (equipped with 2,6-diisopropylphenyl imide substituents) for illustration of the impact of two attached imide units to the polycyclic aromatic framework. (c) Photographs of perylene and PBI solutions under ambient and UV light, respectively. | |
1.1 Brief history of PADIs
The chemistry of PADIs has a rich history dating back to the mid-19th century, at which time the empirical formula of the smallest PADI, naphthalimide (3, Fig. 2), was first predicted.11 The discovery of naphthalimide led to the exploration of PADIs with larger aromatic systems and additional dicarboximide groups, such as the first PBI derivatives reported by Kardos in 1913.12 The intense colour of PBI derivatives, with 4 in Fig. 2 being the pristine unsubstituted molecule in this class, attracted particular interest from the pigment industry. This interest spurred the development of PBI chemistry in the 1950s, making a significant milestone in the field. The structure of PBI consists of a perylene core flanked by two imide groups, which confer exceptional thermal and photochemical stability, as well as strong absorption in the visible region. They are also recognized as stable, highly fluorescent dyes.7,14
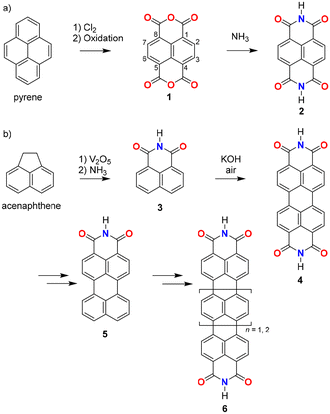 |
| Fig. 2 Known synthetic pathways for naphthalene bis(dicarboximides), perylene bis(dicarboximides), and higher rylene bis(dicarboximides).7,20 | |
The introduction of PBI marked a turning point in the chemistry of PADIs, expanding the scope of these compounds from simple dyes to versatile materials with applications in organic electronics, photovoltaics, and molecular sensors.15 The extended π-conjugation of the perylene core, combined with the electron-accepting nature of the imide groups, made PBIs particularly attractive for use in organic semiconductors as n-type materials. Their ability to undergo reversible redox processes further enhanced their suitability for electronic applications, including their use as active materials in batteries16 and photoredox catalysis.17
Building on the success of PBIs, researchers have since explored a wide range of larger and more complex aromatic dicarboximides. This effort includes the development of rylene bis(dicarboximides) (6), which are derived from even larger aromatic cores such as terrylene and quaterrylene.3,18 These compounds extend the π-conjugation even further, resulting in materials with lower bandgaps, intense absorption in the near-infrared region, and enhanced charge transport properties.
The exploration of these larger aromatic dicarboximides has been driven by the need to tailor the electronic and optical properties of the materials for specific applications. For example, the development of rylene bis(dicarboximides) has been particularly rewarding by the stepwise shift of the absorption maxima far into the infrared region by each additional peri-annulated naphthalene unit, being of interest for harvesting solar energy and for near infrared emission.19 Today, the field of PADIs continues to evolve, with ongoing research focused on expanding the structural diversity and functional properties of these materials.
1.2 Conventional syntheses of PADIs
The synthetic pathways for the archetypical PADIs, naphthalene and perylene bis(dicarboximides), are summarized in Fig. 2. Vollmann et al. reported a typical synthetic pathway for naphthalene bis(dicarboximides) where pyrene was used as the starting material, undergoing chlorination/oxidation steps to reach 1,4,5,8-naphthalenetetracarboxylic acid-1,8:4,5-dianhydride (1).20 Dianhydride 1 was then imidized by ammonia or amine derivatives to give naphthalene-1,8:4,5-bis(dicarboximide) (2). The conventional synthetic pathway for perylene bis(dicarboximides) resembles that for naphthalene bis(dicarboximides), except acenaphthalene was used as the starting material to produce naphthalene mono(dicarboximide) 3, which is then dimerized in the presence of potassium hydroxide and air to give perylene-3,4:9,10-bis(dicarboximide) (4).13 Further conversion of imide 4 to perylene mono(dicarboximide) 5 allows for the extension to ter- or quaterrylene bis(dicarboximides) 6.
Other synthetic efforts for PADIs with non-rylene core structures can be characterized by linear approaches (Fig. 3). Jiang and Wang,21 as well as Nuckolls22 employed oligomerization of perylene bis(dicarboximide) derivatives (7 and 8). They first prepared PBI derivatives with appropriate functional groups to facilitate the formation of oligomers in subsequent steps. Wudl and coworkers reported synthesis of decacyclene tris(dicarboximide) (9), which was obtained via Friedel–Crafts carbamylation of the decacyclene core followed by imidization.23 Tetrakis(dicarboximide) 10 was produced by Stępień and coworkers where an acenaphthalene dicarboximide was first converted to the corresponding acenaphthalenone dicarboximide, which then acted as a monomer unit and underwent cyclotetramerization.24
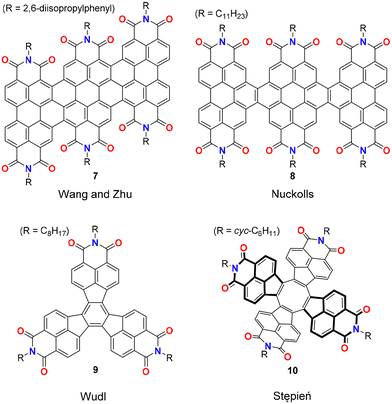 |
| Fig. 3 Representative PADIs that possess non-rylene core structures.21–24 | |
From the viewpoint of synthetic chemistry, these pathways align more closely with linear synthetic approach, where the starting material is functionalized in a stepwise manner to reach the target product. Thus, it is hard with such synthetic approaches to produce PADIs characterized by uncommon π-scaffolds, i.e. those not relying on rylene-based core structures, as exemplified by the contributions from Wudl and Stępień.23,24 On the contrary, convergent synthetic approaches where two components that together build up the structure of the target molecule are synthesized separately and connected at a later step would tremendously reduce the synthetic cumbersomeness and also contribute in enabling production of new unique PADIs bearing previously unknown π-scaffolds. For the connection of two or more aromatic substrates, as required in convergent approaches, transition metal-catalysed cross-coupling reactions provide a valuable toolbox.25 In this review, we describe recent developments in convergent synthetic approaches for PADIs using Pd-catalysed annulation as a key step for combining two or more components that build up the targeted PADI structures.
2. Synthetic methodology
Annulation, a chemical operation involving the formation of two or more chemical bonds to create an additional ring,26,27 is a valuable method to achieving a convergent synthetic approach to PADIs. Fig. 4 shows two possible modes for introduction of imide moieties through such convergent approaches. The simpler mode is a [3 + 3] annulation, where two peri-regions each comprising three carbons face each other to form a six-membered ring (Fig. 4, left). This reaction mode can also be performed in a cascade manner to involve two [3 + 3] annulations and a [2 + 2 + 2] annulation, as in Fig. 4, right. In this way, π-extended PADIs can be formed from two PAH starting materials: one containing the core π-scaffold, highlighted with blue filling, and the other bearing a dicarboximide moiety, coloured green in Fig. 4.
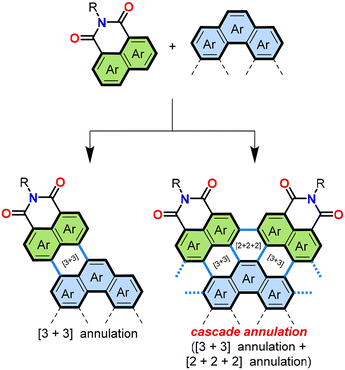 |
| Fig. 4 Annulation concept of convergent synthetic approaches for PADIs. | |
Our group started to examine the aforementioned convergent approach about ten years ago combining naphthalene mono(dicarboximide) as the imide-containing π-conjugated structure and pyrene as the π-core. We hypothesized that tetraimide 11 (Fig. 5) could be obtained by combining naphthalimide and pyrene with additional functional groups, i.e., readily available dibromonaphthalene dicarboximide 12 and pyrene tetra(boronic acid) pinacol ester 13. This retrosynthetic analysis was experimentally tested using two different combinations of substrates, as shown in Fig. 6.28 One combination involved the pinacol ester of naphthalimide and the tetrabromide of pyrene (14 and 15), while the other used opposite functionalities, with dibromo-substituents on naphthalimide and the pinacol ester on pyrene (12 and 13). The successful synthesis of tetrakis(dicarboximide) 11 was achieved with the latter combination of substrates. Although the former combination of substrates successfully yielded intermediate 16, oxidative cyclization of it proved difficult. Without contemplating about mechanistic details (Fig. 7) it seems logical that the second step of dehydrobromination should be more favourable than dehydrogenation, in particular in the presence of base.
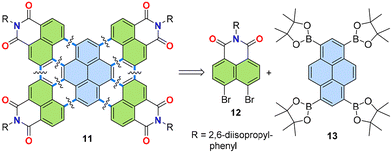 |
| Fig. 5 Retrosynthetic analysis of tetrakis(dicarboximide) 11 using a convergent synthetic approach. | |
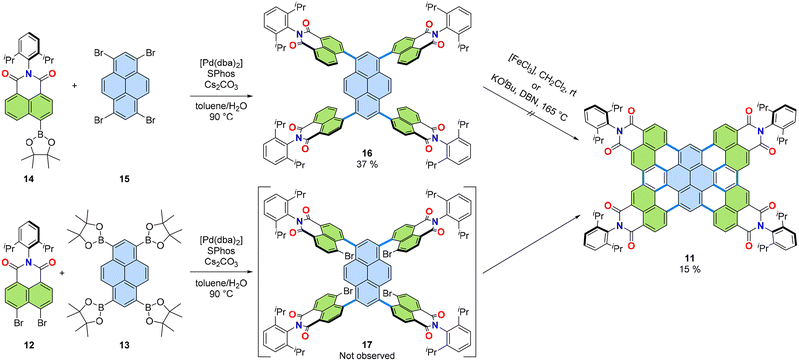 |
| Fig. 6 Two synthetic approaches tried for tetrakis(dicarboximide) 11. dba: dibenzylideneacetone. SPhos: dicyclohexyl(2′,6′-dimethoxy[1,1′-biphenyl]-2-yl)phosphane. DBN: 1,5-diazabicyclo(4.3.0)non-5-ene.28 | |
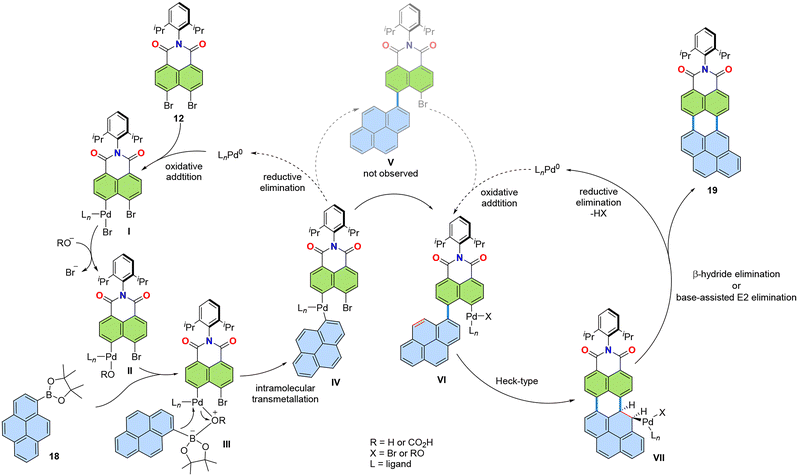 |
| Fig. 7 Proposed reaction mechanism of the palladium-catalysed [3 + 3] annulation of dibromide 12 and boronic ester 18.30 | |
The successful synthesis of 11 from 12 and 13 utilized standard Suzuki–Miyaura cross-coupling conditions: palladium(0) precursor Pd(dba)2 and Buchwald ligand SPhos29 as the catalyst/ligand combination, caesium carbonate as the base, toluene/water as the solvent at 90 °C. This reaction formed product 11 in 15% isolated yield, a high overall yield considering the formation of 10 C–C bonds in one pot. Interestingly, the reaction proceeded without yielding the expected intermediate product 17. The overall reaction can be explained by a cascade of four [3 + 3] annulations and two [2 + 2 + 2] annulations as shown in Fig. 4.
Surprisingly, during this cascade annulation, no intermediate containing unreacted bromide substituents on one of the naphthalimide substrates was observed. To investigate this further, a one-fold cascade annulation was also performed using pyrene mono boronic acid pinacol ester 18 (Fig. 7).30 In this case as well, the expected intermediate product V was not observed. Instead, imide 19 was the only product obtained during the reaction. Based on these observations, the reaction mechanism proposed in Fig. 7 was suggested for this cascade [3 + 3] annulation. The mechanism involves common Pd-catalysis steps: oxidative addition of Pd0 to 12 to form intermediate I, ligand exchange from Br to −OR to form II, followed by transmetallation through intermediates III to IV. The expected product V after reductive elimination wasn't observed, leading to the assumption that IV converts directly to VI in a concerted manner. Intermediate VI, due to the high double bond character of the 4,5-positions on pyrene, undergoes Heck-type insertion to form VII, which then releases product 19via either β-hydride elimination or base-assisted E2 elimination.
After these initial results in convergent synthetic approaches for PADIs, a more detailed investigation of the reaction conditions was carried out.31 As the model system, naphthalene-1-boronic acid pinacol ester 20 and dibromonaphthalimide 12 were used as the organometallic and halide coupling partners, respectively. Parameters such as ligand, additive, solvent, and concentration were varied to determine the optimal reaction conditions. The results are summarized in Table 1. Overall, the ligand played a major role, with tricyclohexylphosphine proving to be the most effective ligand. Further experiments showed that using 1-chloronaphthalene32 as the solvent and conducting the reaction in the absence of additives yielded the best result, with a yield of 88%.
Table 1 Optimization of reaction conditions for [3 + 3] annulation of 12 and 2031
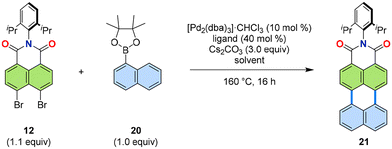
|
Entrya |
Ligand |
Additive (amount) |
Solvent |
Conc. (M) |
Yieldb (%) |
20 (0.04 mmol), 12 (1.1 equiv.), [Pd2(dba)3]·CHCl3 (10 mol%), ligand (40 mol%), Cs2CO3 (3.0 equiv.), solvent, 160 °C, 16 h.
NMR yield using 1,1,2,2-tetrachloroethane as internal standard.
Isolated yield. o-DCB: o-dichlorobenzene, 1-ClNaph: 1-chloronaphthalene, SPhos: 2-dicyclohexylphosphino-2′,6′-dimethoxybiphenyl, IPr: 1,3-bis(2,6-diisopropylphenyl)imidazol-2-ylidene.
|
1 |
SPhos |
— |
o-DCB |
0.03 |
<5 |
2 |
P(o-tolyl)3 |
— |
o-DCB |
0.03 |
11 |
3 |
P(tBu)3·HBF4 |
— |
o-DCB |
0.03 |
12 |
4 |
IPr·HCl |
— |
o-DCB |
0.03 |
42 |
5 |
P(C6F5)3 |
— |
o-DCB |
0.03 |
<5 |
6 |
PCy3·HBF4 |
— |
o-DCB |
0.03 |
50 |
7 |
PCy3·HBF4 |
— |
o-DCB |
0.1 |
17 |
8 |
PCy3·HBF4 |
— |
o-DCB |
0.01 |
57 |
9 |
PCy3·HBF4 |
Bu4NCl (0.5 equiv.) |
o-DCB |
0.03 |
24 |
10 |
PCy3·HBF4 |
Bu4NBr (0.5 equiv.) |
o-DCB |
0.03 |
19 |
11 |
PCy3·HBF4 |
— |
1-ClNaph |
0.03 |
88c |
12 |
PCy3·HBF4 |
— |
1-ClNaph |
0.01 |
86c |
13 |
PCy3·HBF4 |
H2O (100 equiv.) |
1-ClNaph |
0.03 |
65 |
With aforementioned information in hand, the chemistry of convergent synthetic approaches for PADIs was further developed. In the following sections, a variety of substrates were applied to this annulation protocol. The scope of this annulation includes simple one- and two-fold annulations (Section 3) and more complex three-, four-, and five-fold annulations (Section 4). It is also important to note that for higher densities of imide moieties in multi-fold annulations, the key to success was overcoming the low solubility of the product by introducing bulky N-substituents. For the introduction of bulky meta-terphenyl groups, a specifically developed imidization was essential, as detailed in Section 4.1.
3. Mono- and diimides
The newly developed [3 + 3] annulation is a useful synthetic method for achieving the convergent synthesis of PADIs. This method was applied to a variety of substrate combinations to synthesize new PADI derivatives that had previously been inaccessible through conventional linear synthetic approaches.
3.1 Annulation with simple PAHs
Various PAHs were applied as the boronic ester coupling partners in the annulation to produce new PADIs (Fig. 8a).31,33,34 Thus, expansion from naphthalene as for 21 to pyrene (for 19), coronene (for 22) and other PAHs, as well as heteroatom-containing PAHs, such as benzothiophene (for 23) and quinoline (for 24), was successfully achieved with the [3 + 3] annulation. The reaction scope was further expanded to include two-fold annulations, as demonstrated with 25, using pyrene-1,6-diboronic ester as the boronic acid coupling partner.
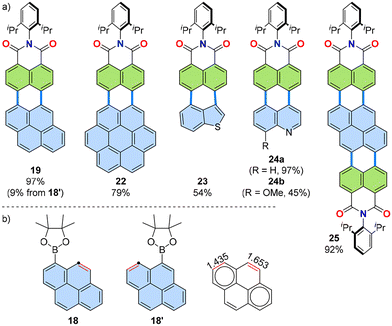 |
| Fig. 8 (a) Representative products of [3 + 3] annulation of simple aromatic substrates with naphthalimide 12. (b) Structures of two isomers of pyrene boronic ester (18 and 18′), as well as the Wiberg bond order35 of pyrene. The reacting double bond is coloured red and the reacting carbon atom shown as dot.31,34 | |
As discussed in Section 2 (Fig. 7), this [3 + 3] annulation presumably proceeds via a Heck-type mechanism involving an η2-coordinated intermediate (i.e., VII in Fig. 7). This assumption suggests that the double bond character of the coordinated site (marked as a red double bond in the structure of VI in Fig. 7) plays a critical role determining the reactivity of the boronic ester substrate during annulation. When applied to the reaction, the two isomeric pyrene boronic esters 18 and 18′, resulted in markedly different yields for the same product, 19: the former gave a yield of 97% while the latter yielded only 9%. The corresponding double bond character of the reaction site as evaluated by the Wiberg bond order,35 being inversely correlated to the aromaticity of the ring, explained this discrepancy: for 18, the bond order was high at 1.653, whereas for 18′, it was only 1.435 (Fig. 8b). This discussion will also be relevant to another annulation mode, the [3 + 2] mechanism, which is covered later in Section 3.5.
3.2 Tetrachloroperylene as the imide substrate
The [3 + 3] annulation of boronic esters and dibromides was also extended to a larger dibromide coupling partner, tetrachloroperylene dicarboximide 26.36,37 This dibromide was prepared via a Hunsdiecker-type reaction of tetrachloroperylene bis(anhydride).38 Essentially the same reaction conditions used for the synthesis of 21 were applied to yield π-extended tetrachloroperylene dicarboximide 27 from naphthalene boronic ester 20 and tetrachloroperylene dicarboximide 26 (Fig. 9a). The boronic acid substrate was also varied to phenanthrene (for 28), pyrene (for 29), coronene (30), naphthalimide (31), and others. Additionally, the ester and dibromo functionalities were reversed to prepare terrylene dicarboximide 34 from dicarboximide boronic ester 32 and dibromonaphthalene 33 (Fig. 9b), showcasing the robustness of this annulation protocol across various substrate combinations.33 These results demonstrate the versatility of this method with respect to both boronic acid and dibromo substrates.
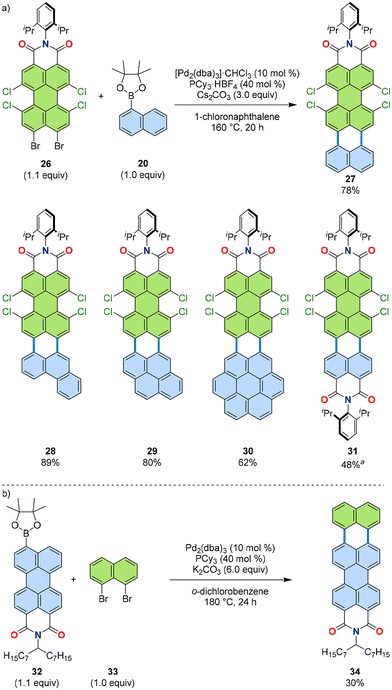 |
| Fig. 9 (a) Reaction scheme (top) and representative products (bottom) of [3 + 3] annulation of simple aromatic substrates with perylene dicarboximide 26. (b) Reaction scheme of perylene dicarboximide boronic ester 32 and dibromide 33 for terrylene dicarboximide 34. a Pd(PPh3)2Cl2, Cs2CO3, 1-chloronaphthalene, 140 °C, 12 h. dba: dibenzylideneacetone.33,36,37 | |
3.3 [3 + 3] annulation with anilines, indoles, and carbazole
Further exploration of the [3 + 3] annulation of aromatic imides and PAHs enabled the use of aniline derivatives, as well as indoles and carbazole, as PAH substrates (Fig. 10).39 Following optimization of reaction conditions, an alternative naphthalimide substrate 35 with chloro-boronic ester substituents was employed as the imide coupling partner. Under optimized conditions, naphthalimide 35 was successfully reacted with aniline, indole, and carbazole derivatives 36 for one-fold annulation and dihaloaniline derivatives 37 for two-fold annulation. A representative set of products is shown in Fig. 10a, along with their isolated yields. For the introduction of carbazole, the combination of dibromide 12 and di-tert-butylcarbazole 43 was used to produce 44 under slightly modified conditions (Fig. 10b).40
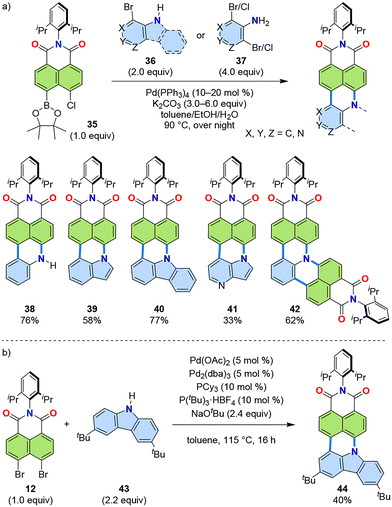 |
| Fig. 10 (a) Reaction scheme (top) and representative products (bottom) of the [3 + 3] annulation of amine-containing aromatic substrates with naphthalimide 35. (b) Reaction of dibromide 12 and carbazole 43 for PADI 44.39,40 | |
3.4 [3 + 2] annulation with thiophenes and indoles
The successful annulation of heterocycles, as demonstrated for 23 and 24, and 38–42, suggests that the method should also be applicable to boronic esters of other heterocycles, such as thiophene and indole. Thus, the reaction conditions were optimised to enable the [3 + 2] annulation of thiophene-1-boronic esters and indole-1-boronic esters (Fig. 11a).41 To prevent double Suzuki–Miyaura cross-coupling of heterocyclic substrates (46) to naphthalimide, a two-step procedure was introduced. Therefore, chloro-bromo-naphthalimide (45a), instead of dibromo-naphthalimide, was used to allow a stepwise Suzuki–Miyaura coupling/direct arylation cascade. The first step, Suzuki–Miyaura coupling, was conducted at 90 °C with the P(m-tolyl)3 ligand (highlighted as orange bonds in Fig. 11a). The second step, direct arylation, was successively performed with the addition of tetrabutylammonium chloride (highlighted as green bonds in Fig. 11a). This additive is often used to increase the reactivity of direct arylation.42 These modifications allowed for the preparation of heterocycle-annulated products such as 47–51, as shown in Fig. 11a. Additionally, a [3 + 2] annulation that led to pyrrole ring formation was achieved by reacting dibromide 12 with indole boronic ester 52, yielding pyrrolizine 53 (Fig. 11b).43
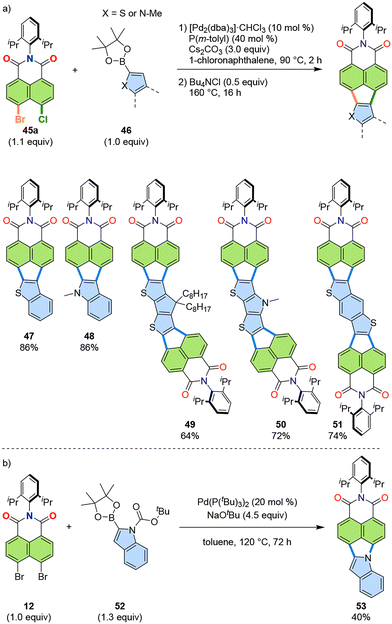 |
| Fig. 11 (a) Reaction scheme (top) and representative products (bottom) of the [3 + 2] annulation of naphthalimide 45a and heterocycles 46. (b) Reaction of dibromide 12 and indole boronic ester 52 for PADI 53. dba: dibenzylideneacetone.41,43 | |
3.5 Formation of five-membered rings by [3 + 2] annulation
Heterocyclic substrates underwent the [3 + 2] annulation mode, yielding the corresponding products. This result suggests that the [3 + 2] annulation mode should also be possible with non-heterocyclic PAHs. The [3 + 3] annulation mode achieved for 19 and 21–25 (Table 1 and Fig. 8) required a second C–C bond formation site with a high degree of double-bond character, suggesting that the Heck-type reaction mechanism was involved (Fig. 7, VI to VII). It was hypothesized that using a bulky dative ligand, such as 1,8-diazabicyclo[5.4.0]undec-7-ene (DBU), as the base would enable the sterically favoured [3 + 2] annulation mode. As expected, the reaction using DBU as the base led to the formation of products with five-membered rings (54–58, Fig. 12a).44
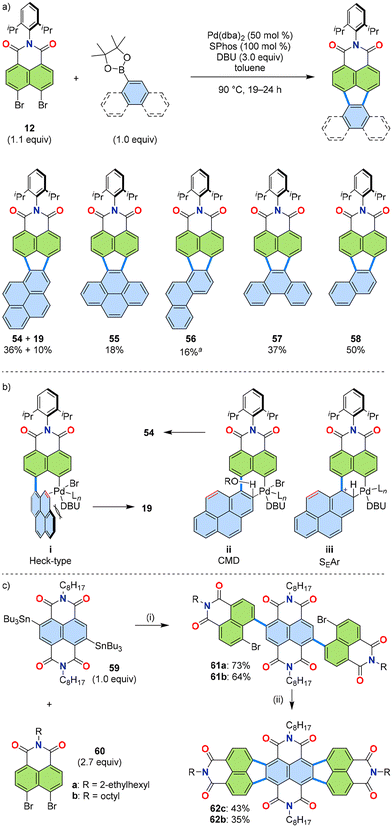 |
| Fig. 12 (a) Reaction scheme (top) and representative products (bottom) of the [3 + 2] annulation of naphthalimide 12 and PAH boronic esters. (b) Assumed reaction intermediates for three possible reaction mechanisms: Heck-type, concerted metallation–deprotonation (CMD), and electrophilic aromatic substitution (SEAr). (c) An applied [3 + 2] annulation of bis(stannyl)imide 59 and dibromide 60 for PADI 62. Conditions: (i) Pd(PPh3)4 (22 mol%), CuI (44 mol%), toluene, rt, 12 h; (ii) Pd(PPh3)2Cl2 (20 mol%), DBU (4.0 equiv.), dimethylacetamide, 160 °C, 24 h. a Similar to the case for 54, the six-membered ring product was isolated in 2% yield. dba: dibenzylideneacetone, SPhos: dicyclohexyl(2′,6′-dimethoxy[1,1′-biphenyl]-2-yl)phosphane, DBU: 1,8-diazabicyclo[5.4.0]undec-7-ene.44,45 | |
As discussed in Section 3.1, there are three possible reaction mechanisms for the Pd-catalysed annulation discussed here. For the [3 + 3] annulation mode, taking the example of the reaction of pyrene-1-boronic ester 18 with dibromide 12, the Heck-type intermediate (i, Fig. 12b, see also VII in Fig. 7) is presumably the active intermediate during annulation, as it leads to the formation of a six-membered ring, yielding 19. For the [3 + 2] annulation mode, however, the other two possible reaction mechanisms—concerted metallation–deprotonation (CMD, ii) or electrophilic aromatic substitution (SEAr, iii)—should participate in product formation, as they lead to the formation of a five-membered ring, yielding 54 (Fig. 12b).
In the presence of DBU, it is assumed that DBU coordinates to the Pd centre, which hinders the formation of the Heck-type intermediate i due to steric hinderance. Consequently, the reaction favours the other two intermediates, ii or iii, resulting in the formation of 54. Indeed, by substituting the base Cs2CO3 with bulky DBU, the reaction could be switched to yield 54 as the major product in 36% yield, whereas 19 was isolated in only 10% yield. The reaction using Cs2CO3 under otherwise identical conditions yielded 19 as the sole product in 50%.
When an isomeric pyrene-4-boronic ester 18′ instead of 18 was used, the reaction gave 55 as the sole product through the formation of a five-membered ring. This observation can be explained by the absence of a C–C bond at the reaction site with high double bond character (as discussed in Section 3.1 and Fig. 8b), further substantiating the proposed explanation on the switch in reaction mechanism from six- to five-membered ring formation when DBU was used as the base.
An application of this [3 + 2] annulation mode was demonstrated by combining bis(stannyl)imide 59 with dibromide 60 (Fig. 12c).45 The products 62, designed for n-type semiconductor applications, were synthesized in two steps from these two starting materials. First, a Stille coupling yielded 61, followed by ring closure using DBU as the base, giving 62 in yields of 35–43%.
3.6 Synthesis of non-alternant PADI
Different from alternant PAHs, non-alternant PAHs show unique photophysical properties. Thus, it was expected that the annulation using a non-alternant isomer of naphthalene, namely azulene, would produce new PADIs that exhibit both the properties of azulene and imides. The readily available diboronic ester of azulene 63 was successfully applied to the [3 + 3] annulation with naphthalimide 12 to give the doubly naphthalimide-annulated azulene derivative 64 (Fig. 13).46 Interestingly, this azulene derivative showed the intrinsic azulene structural properties, which is in stark contrast to other known π-extended azulene derivatives to date.47–49 Thus, azulene 64 was the first intrinsically π-extended azulene, which was corroborated by aromaticity analysis using nucleus-independent chemical shift (NICS) and anisotropy of the induced current density (ACID), as well as the long-wavelength absorption measured by UV/Vis absorption spectroscopy. Azulene 64 was further functionalized via bromination to produce 65, followed by nucleophilic substitutions with morpholine (66) or methoxide (67).
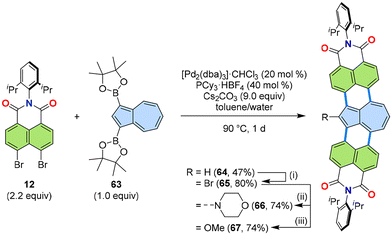 |
| Fig. 13 Reaction scheme of the [3 + 3] annulation of naphthalimide 12 and azulene diboronic ester 63. (i) N-Bromosuccinimide (2 equiv.), THF, rt, 21 h. (ii) morpholine (10 equiv.), CH2Cl2, rt, 4.5 h. (iii), n-BuLi (10 equiv.), THF/MeOH, rt. dba: dibenzylideneacetone.46 | |
3.7 Synthesis of curved PADIs
The [3 + 3] annulation strategy is also a useful method to produce non-planar PADIs. By using a bowl-shaped PAH boronic ester, such as corannulene mono- or di-boronic ester (68 or 69), bowl-shaped PADIs were synthesized (Fig. 14). One-fold [3 + 3] annulation was flawlessly carried out to yield 70 by using the reaction conditions developed for the model system, as presented in Table 1.31 Due to the low reactivity of non-planar PAHs, the two-fold annulation for 71a had to be optimized to include the two-step approach similar to the [3 + 2] annulation for thiophene- or indole-containing PADIs 47–51 (Section 3.4), using bromo-chloro-naphthalimide 45 as the coupling partner and Bu4NCl as an additive for the promotion of the second step.50 A derivative of 71a with highly soluble trialkylphenyl substituents (71b) showed self-assembly into dimers where the two bowls are stacked together at close contact.51 Another derivative of 71a with alkyl side chain (71c) was proven to be useful for an active material in organic solar cells as an n-type semiconductor.52
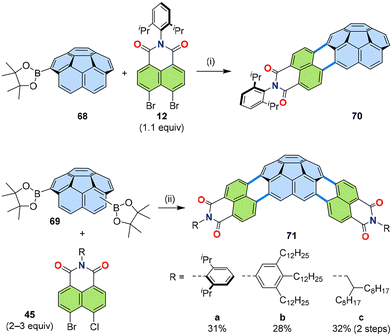 |
| Fig. 14 Reaction scheme of the [3 + 3] annulation of naphthalimide 12 or 45 and corannulene mono- or di-boronic esters 68 or 69, respectively. (i) [Pd2(dba)3]·CHCl3 (10 mol%), PCy3·HBF4 (40 mol%), Cs2CO3 (3.0 equiv.), 1-chloronaphthalene, 160 °C, 16 h. (ii) For 71a: [Pd2(dba)3]·CHCl3 (20 mol%), P(o-tolyl)3 (80 mol%), Cs2CO3 (4.5 equiv.), water (100 equiv.), o-dichlorobenzene, 90 °C, 2 h, then added Bu4NCl (1.0 equiv.), 160 °C, 24 h; for 71b: [Pd2(dba)3]·CHCl3 (5 mol%), P(m-tolyl)3 (20 mol%), Cs2CO3 (6 equiv.), 1-chloronaphthalene, 100 °C, 1 d; for 71c: (1) [Pd2(dba)3]·CHCl3 (5 mol%), P(m-tolyl)3 (20 mol%), Cs2CO3 (6 equiv.), 1-chloronaphthalene, 110 °C, 2 d, (2) [Pd2(dba)3]·CHCl3 (10 mol%), P(1-Ad)3 (30 mol%), Cs2CO3 (6 equiv.), Bu4NCl (0.5 equiv.), 1-chloronaphthalene, 170 °C, 16 h. dba: dibenzylideneacetone, 1-Ad: 1-adamantyl.31,50–52 | |
3.8 Synthesis of chiral PADIs
Helicenes are another class of non-planar PAHs that also give rise to chiral properties, such as circular dichroism (CD) and circularly polarized luminescence (CPL). Two non-racemizing helicene boronic esters, [5]helicene 72 and [6]helicene 73, were subjected to two-fold [3 + 3] annulation with naphthalimide 12 to furnish chiral PADIs 74 and 75, respectively (Fig. 15).53 These chiral PADIs showed red CPL up to 655 nm, which is a longer wavelength than that of most chiral emitting organic molecules, and high CPL brightness up to 66 M−1 cm−1.
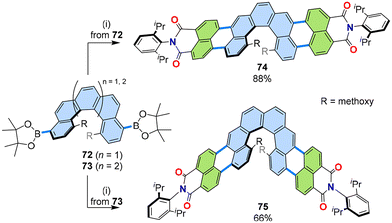 |
| Fig. 15 Reaction scheme of the [3 + 3] annulation of naphthalimide 12 and [n]helicene diboronic esters 72 and 73. (i) 12 (2.5 equiv.), [Pd2(dba)3]·CHCl3 (20 mol%), PCy3·HBF4 (80 mol%), Cs2CO3 (6.0 equiv.), 1-chloronaphthalene, 160 °C, 2 d. dba: dibenzylideneacetone.53 | |
3.9 Core-twisted quaterrylenes
Extended rylenes, such as quaterrylenes, are proven to possess intriguing NIR absorption properties owing to their extended π-core. The developed [3 + 3] annulations were expanded to the synthesis of contorted quaterrylenes by modifying the substrate combination. Instead of peri-dibromide of imides, the perylene derivative 76, which has two sets of peri-dibromo moieties on both sides (Fig. 16), could be used to undergo a two-fold [3 + 3] annulation with boronic ester 77 to give quaterrylene 79 (Fig. 16).54,55 Interestingly, this quaterrylene has four congested p-tert-butylphenyl groups at the bay positions of the middle two naphthalene units, creating a large twist in the middle of the rylene scaffold. This twisted interlocked structure resulted in the preservation of chirality even at elevated temperature and afforded for 79a intriguing double helical self-assembled J-aggregates.55 The interlocked chiral tetraphenyl-PBI core recently also proved to be advantageous for PBI-based organic photovoltaic materials.56
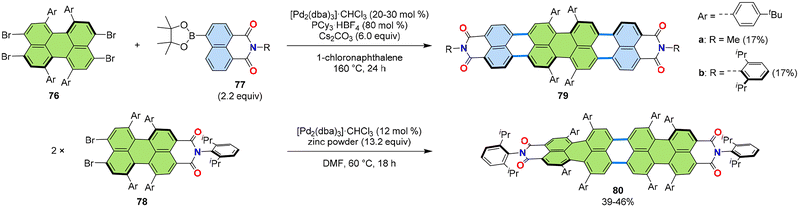 |
| Fig. 16 Reaction scheme of the [3 + 3] annulation for the synthesis of quaterrylene derivatives 79 and 80. dba: dibenzylideneacetone.54,55 | |
Another synthetic approach to quaterrylene bis(dicarboximide) was demonstrated using the homo-coupling of perylene imide 78. In the presence of a palladium(0) catalyst and zinc powder, dimerization of enantiopure 78 proceeded, yielding the homo-coupled product 80. The combination of Pd-catalysed Ullmann-type coupling and the dibromo-substrate was key to achieving a smooth reaction at relatively low temperature, preventing racemization of the substrate. This contrasts with the use of Scholl reaction with non-halogenated substrate in strongly acidic media or in the presence of oxidants,57 which typically requires higher reaction temperatures and would have caused racemization. This quaterrylene showed an end-to-end twist of up to 76°, giving rise to strong circular dichroism.
3.10 Annulation of dibromonaphthalene dicarboxylic acid diester with PAH boronic ester
As described in Section 3.2, the dibromo coupling partner could be varied for the developed [3 + 3] annulation. Not only electron-deficient imide dibromides but also more electron-rich dicarboxylic acid diesters could undergo annulation. Thus, a series of boronic esters 82 were successfully annulated with dibromo-diesters 81, yielding perylene diesters 83–86 (Fig. 17a). These diesters could be further functionalized into perylene dicarboximides bearing an acid moiety at the N-substituent (87), which functioned as amphiphiles for supramolecular polymerization in water.58 A double annulation of diester 81 was achieved with naphthalene diboronic ester 88 to synthesize terrylene tetraester 89 (Fig. 17b).33
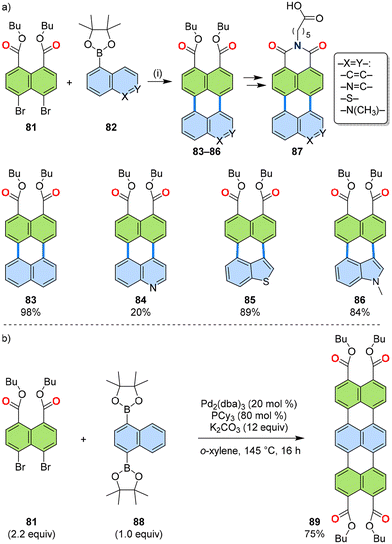 |
| Fig. 17 (a) Reaction scheme of the [3 + 3] annulation of 1,8-dibromonaphthalene 81 and PAH boronic esters 82. (i) Pd2(dba)3 (10 mol%), PCy3 (40 mol%), K2CO3 (6.0 equiv.), o-xylene/o-dichlorobenzene, 145–180 °C, 24–48 h. (b) A two-fold [3 + 3] annulation of dibromide 81 and diboronic ester 88. dba: dibenzylideneacetone.33,58 | |
3.11 Annulation of dibromonaphthalene or dibromoacenaphthene with PAH boronic esters
Although the main focus of this review is on synthetic methods for PADIs, we deem it appropriate to make an exception here to highlight the broader scope of these peri-dihalonaphthalene annulations. Thus, the [3 + 3] annulations designated for PADIs have also proven valuable for synthesizing electron-rich PAHs, especially those with unsymmetric structures that have been difficult to access through conventional oxidative coupling reactions. For instance, annulation of peri-dibrominated PAHs, such as 1,8-dibromonaphthalene 33 or 5,6-dibromoacenaphthene 90, with PAH boronic esters was successfully carried out to produce π-extended unsymmetrical PAHs 91–100 (Fig. 18).59
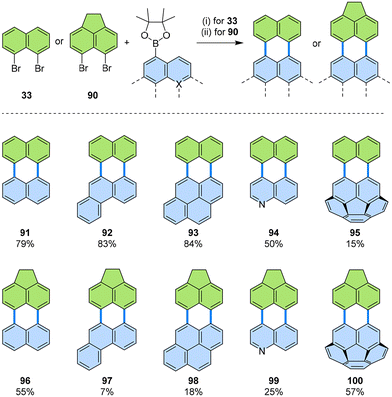 |
| Fig. 18 Reaction scheme of the [3 + 3] annulation of 1,8-dibromonaphthalene 33 or 5,6-dibromoacenaphthene 90 and PAH boronic esters. (i) 33 (1.1 equiv.), PAH boronic ester (1.0 equiv.), [Pd2(dba)3]·CHCl3 (10 mol%), PCy3·HBF4 (40 mol%), Cs2CO3 (3.0 equiv.), 1-chloronaphthalene, 160 °C, 16 h. (ii) 90 (1.0 equiv.), PAH boronic ester (1.1 equiv.) [Pd(PPh3)4] (20 mol%), Cs2CO3 (3.0 equiv.), mesitylene, 120 °C, 2–5 d. For 99 the reaction time was shortened to 16 h. dba: dibenzylideneacetone.59 | |
Caution must be exercised with these substrate systems because different dibromo coupling partners required different reaction conditions. While dibromide 33 could be reacted under standard conditions for PADIs, with [Pd2(dba)3]·CHCl3 as the catalyst and 1-chloronaphthalene as the solvent at a high reaction temperature of 160 °C, its acenaphthene derivative 90 gave optimal yields with Pd(PPh3)4 as the catalyst/ligand and a milder reaction temperature of 120 °C in mesitylene. Nevertheless, a variety of products could be furnished with 33 or 90 as the dibromo coupling partner for pure hydrocarbons (91–93 and 96–98), nitrogen-containing π-extended quinolines (94 and 99), and bowl-shaped PAHs (95 and 100).
4. Multiimides (triimides, tetraimides, and pentaimide)
For the synthesis of multiimides bearing larger π-cores and more than two imide groups, special care must be taken to ensure sufficient solubility of the product and the reaction intermediates for good reactivity. Otherwise, the reaction would yield insufficiently reacted products, i.e., only partially annulated products or products missing some of the desired C–C bonds. Therefore, in addition to the standard 2,6-diisopropylphenyl substituent, meta-terphenyl substituents were considered as the imide substituents. For the preparation of these imide precursors, i.e., derivatives of 12 with bulkier N-substituents, a new imidization method was necessary to overcome low reactivity of bulky aniline derivatives in imidization reaction.
4.1 Imidization using bulky aniline derivatives
Imidization of naphthalene anhydrides 102–104 with bulky anilines 101 was inefficient or failed when common reaction conditions for the conversion of rylene dicarboxylic acid anhydride and amine to rylene dicarboximide were applied, i.e. refluxing in acetic acid or reacting at high temperatures in the presence of zinc acetate in imidazole. Thus, it was hypothesized that deprotonation of the aniline substrate by base followed by addition of dicarboxylic acid anhydride would facilitate the reaction through nucleophilic addition of deprotonated aniline to anhydride (Fig. 19).60 Detailed investigations revealed that the reaction of aniline 101d with a strong base, lithium diisopropylamide (LDA), followed by an addition of 103 did give the product 106d but only in low yield. It was found that the main product of such reactions was an isoimide, which then converted to imide 106 upon the addition of water (an example case of the reaction of 101a and 103 is shown in Fig. 19b). The optimal conditions for the imidization of anhydrides 102–104 with bulky aniline derivatives 101 consist of three steps, (1) addition of n-BuLi for deprotonation of aniline 101, (2) addition of the anhydride 102–104 to form the isoimide intermediate, and (3) addition of water to facilitate the formation of the rearranged final product. Under optimized conditions, the desired products 105–107 were successfully obtained, which were otherwise inaccessible.61,62
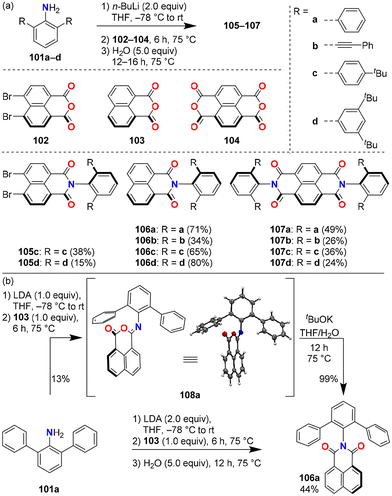 |
| Fig. 19 Reaction scheme of the imidization developed for bulky N-substituents. (a) Optimized reaction conditions of base-assisted imidization using bulky meta-terphenyl amines 101a–d, product structures, and isolated yields. (b) Proposed reaction mechanism supported by an isolated intermediate 108a. LDA: lithium diisopropylamide.60–62 | |
4.2 Triimides
With successful preparation of peri-dibromonaphthalimides 105, it could be expected that large PAHs with multiple imides could be obtained due to the high solubility provided by the aggregation-prohibiting bulky imide substituents. Coronene tris(boronic ester) 109 was annulated with naphthalimide 105d, which contains 2,6-bis(3,5-di-tert-butylphenyl)phenyl-substituents, to give nanographene tris(dicarboximide) 110 (Fig. 20).62 This nanographene undergoes dimerization and complexation with a hexabenzocoronene derivative, resulting in sequestration-enhanced fluorescence, which diminished quenching by oxygen. Another tris(dicarboximide) 112 was synthesized via a three-fold [3 + 2] annulation from subphthalocyanine tris(boronic ester) 111 and 12.63 The curvature derived from subphthalocyanine scaffold gave rise to high solubility, which presumably contributed to the successful reaction, even with standard diisopropylphenyl substituents at the imide nitrogen. An analogous subphthalocyanine with alkyl substituents instead of diisopropyl substituents for 112 was shown to be a superior n-type semiconductor material for bulk-heterojunction organic solar cells.
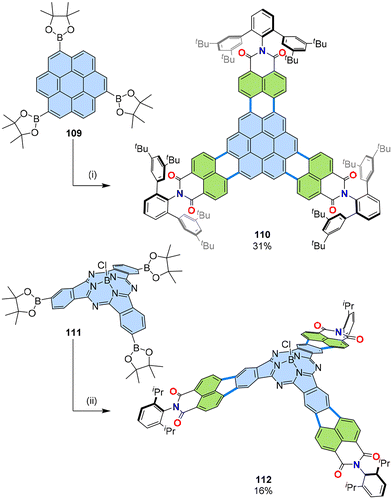 |
| Fig. 20 Synthesis of PAH tris(dicarboximides) 110 and 112. (i) 105d (6.0 equiv.), [Pd2(dba)3]·CHCl3 (80 mol%), PCy3·HBF4 (80 mol%), Cs2CO3 (5.0 equiv.), 1-chloronaphthalene, 180 °C, 2 d. (ii) 12, [Pd2(dba)3]·CHCl3 (15 mol%), PCy3·HBF4 (120 mol%), Cs2CO3 (3.5 equiv.), 1-chloronaphthalene, 160 °C, microwave reactor. dba: dibenzylideneacetone.62,63 | |
4.3 Tetraimide
Derivatization of our original tetrakis(dicarboximide) 11 (Fig. 6) was carried out using optimized reaction conditions and naphthalimide derivatives 105 with bulky N-substituents (Fig. 21) through cascade [3 + 3] and [2 + 2 + 2] annulations.61,64 With 2,6-bis(para-tert-butylphenyl)phenyl substituents tBuPh-11 self-assembled in the presence of PAHs such as coronene into multilayer stacks ranging from di-, tri-, tetra-, hexa-, and poly-layers. Such precise formation of multilayer PAHs was enabled by the cavity created by the four imide substituents on both sides of tetrakis(dicarboximide) tBuPh-11.61 Nanographene tBuPh-11 also proved useful in forming supramolecular complexes with other guests such as porphyrins and phthalocyanines, enabling solubilization of poorly soluble guests65 or guest-sensitized phosphorescence.66
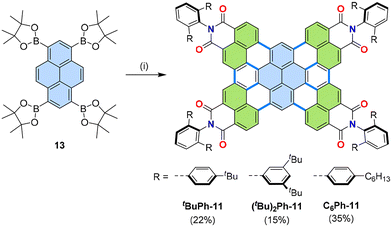 |
| Fig. 21 Synthesis of tetrakis(dicarboximides) tBuPh-11, (tBu)2Ph-11, and C6Ph-11. (i) 105c, 105d, or N-[2,6-bis(4-hexylphenyl)phenyl]-4,5-dibromo-naphthalene-1,8-dicarboximide (4.4 equiv.), [Pd2(dba)3]·CHCl3 (40 mol%), PCy3·HBF4 (160 mol%), Cs2CO3 (12 equiv.), 1-chloronaphthalene, 160 °C, 20–72 h. dba: dibenzylideneacetone.61,62,64 | |
Further variation of N-substituents to 2,6-bis[3,5-di(tert-butyl)phenyl]phenyl for (tBu)2Ph-11 and 2,6-bis(para-hexylphenyl)phenyl for C6Ph-11 enabled the application of these imides as n-type semiconductors for solar cells.64 It is interesting to note that PAHs with such bulky substituents could be used as the active material in organic solar cells, as such substituents, used to enhance solubility, were previously thought to act only as impeding layers. This discrepancy could be explained by the formation of dimers and interactions with donor polymers in the thin films.
4.4 Pentaimide
So far, the greatest number of imides that could be introduced by a single operation of [3 + 3] annulation is five. Corannulene pentakis(boronic ester) 113 could be annulated with naphthalimide 45a to give pentakis(dicarboximide) 115.67 Due to cumbersome purification of the mixture obtained by one-pot reaction (Fig. 22, step (i)), the reaction was divided into two steps (Fig. 22, steps (ii) and (iii)) to apply the best phosphine ligands for the respective steps and ease the purification of product 115. The structure of pentaimide 115 was unambiguously confirmed by X-ray crystallographic analysis of the obtained single crystals. This pentaimide undergoes reversible six-fold reduction processes as confirmed by cyclic and square wave voltammetry. From the structural point of view 115 constituted the first example for a bottom-up synthesis of a carbon nanocone.67,68
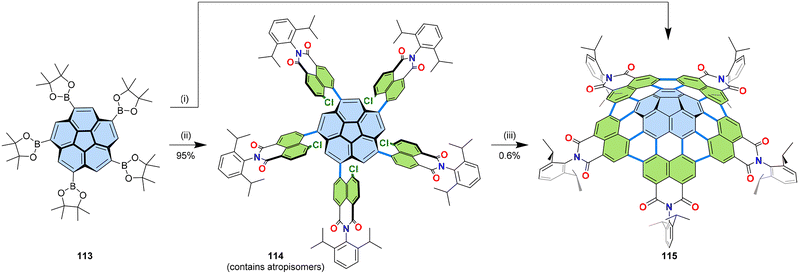 |
| Fig. 22 Synthesis of PAH pentakis(dicarboximides) 115. (i) 113 (1.0 equiv.), 45a (5.5 equiv.), [Pd2(dba)3]·CHCl3 (50 mol%), PBu(1-Ad)2 (200 mol%), Cs2CO3 (15 equiv.), 1-chloronaphthalene, 160 °C, 3 d, c(113) = 0.03 M. (ii) 113 (1.0 equiv.), 45a (5.5 equiv.), [Pd2(dba)3]·CHCl3 (12.5 mol%), P(m-tolyl)3 (50 mol%), Cs2CO3 (15 equiv.), 1-chloronaphthalene, 110 °C, 3 days, c(113) = 0.03 M. (iii) 114 (1.0 equiv.), [Pd2(dba)3]·CHCl3 (50 mol%), P(1-Ad)3 (200 mol%), Cs2CO3 (15 equiv.), 1-chloronaphthalene, 170 °C, 3 days, c(114) = 0.03 M. dba: dibenzylideneacetone, 1-Ad: 1-adamantyl.67 | |
5. Functional properties
This review discussed the synthetic methodologies that have enabled the creation of an entirely new class of electron deficient polycyclic aromatic compounds. Compared to other electron-withdrawing substituents, dicarboximides offer several advantages. They are planar, which allows for the extension of π-conjugation, and delocalization of negative charge across the two carbonyl groups prevents excessive electron density, thus avoiding high reactivity. Due to these features, a significant number of PADIs bearing two imide groups have found commercial applications, in particular as high-grade colour pigments,7 but also in more specialized applications such as fluorescent and laser dyes.13,14 Additionally, PADIs have shown great promise as n-type organic semiconductors,15 and their fully planar acceptor–donor–acceptor π-scaffolds exhibit strong π–π-stacking interactions.2 This has led to the establishment of two major research fields around the year 2000: PADI-derived organic electronic materials69 and PADI-based supramolecular dye chemistry.2 In these fields, PADIs have been incorporated into diverse molecular architectures such as foldamers,70 cyclophanes,71 catenanes,72 and cages.73 Furthermore, PADIs are also highly valued in various areas of chemistry, including radical chemistry74,75 and photothermal conversion.73,76
Accordingly, this review would be incomplete without discussing at least briefly the optical and redox properties of the new, larger PADIs bearing multiple imide units. Fig. 23 illustrates two key examples: first, the electrochemical reduction of the pentaimide nanocone 115 (Fig. 23a), and second, the UV/Vis absorption spectra of three newly synthesized PADIs compared with a PBI derivative (Fig. 23b).
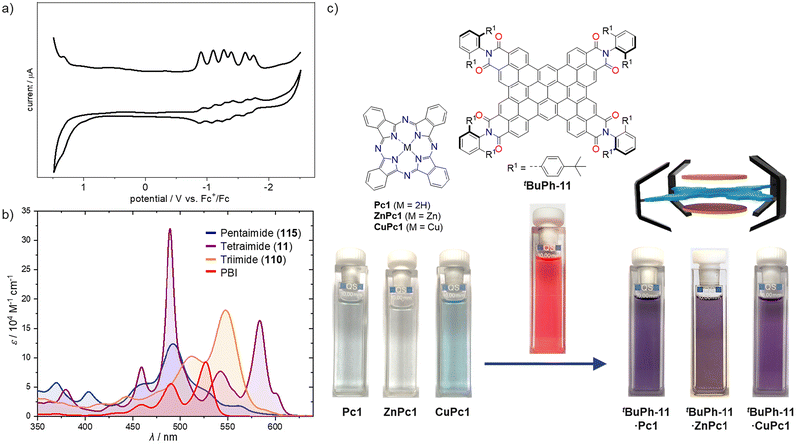 |
| Fig. 23 (a) Cyclic and square wave voltammograms of pentaimide 115 in dichloromethane at room temperature using tetrabutylammonium hexafluorophosphate as the electrolyte referenced against the ferrocene/ferrocenium redox couple. Adapted with permission from ref. 67, copyright 2019 American Chemical Society. (b) UV/Vis absorption spectra of triimide 110, tetraimide 11, and pentaimide 115 in comparison to PBI (bearing 2,6-diisopropylphenyl imide substituents) in dichloromethane (110 and 115) or chloroform (PBI, 11) at room temperature. (c) Extraction of copper phthalocyanine pigments into solution by tetraimide tBuPh-11. Adapted from ref. 65 under a Creative Commons CC BY 4.0 license. | |
With a first reduction potential of −0.90 V (vs. ferrocene/ferrocenium redox couple), pentaimide 115 is reduced with similar ease to core-unsubstituted PBI with the same 2,6-diisopropylphenyl groups (E1/2 = −1.00 V).77 The reduction potential for tetraimide 11, at E1/2 = −0.99 V, falls within the same range, corroborating the idea that similar reduction potentials are obtained when the ratio of imide units to PAH π-surface is comparable. These electron-deficient aromatic compounds exhibit high stability, suggesting their potential utility as n-type organic semiconductors, in battery materials, or for other applications as demonstrated by their more traditional long known counterparts. With regard to organic semiconductor applications, evidence of their effectiveness has already been shown in polymer solar cell devices fabricated with tetraimide 11 derivatives and subphthalocyanine 112.63,64
Regarding applications in batteries, capacitors, or other redox technologies, the electrochemical data for pentaimide 115 presented in Fig. 23 are particularly noteworthy. This C80N5 π-scaffold exhibits six reduction processes in the narrow potential range from −0.90 V to −1.75 V, indicating a much better reducibility than fullerene, which can accumulate five electrons in the potential range from −0.98 V to −3.26 V.78 Based on these observations, the first five electrons are likely loaded onto the five imide units of 115, with the final electron occupying the central five-membered ring. Likewise, tetraimide 11 shows only four reductions between −0.99 V and −1.68 V within the accessible potential window in dichloromethane.
Turning to optical properties, Fig. 23b reveals more complex absorption spectra for the multiimides compared to conventional bis(dicarboximides). Given the two- or even three-dimensional structures of 11 and 115, it is unsurprising that a greater number of optical transitions possess oscillator strength, compared to rylene bis(dicarboximides), whose absorption properties are dominated by the lowest energy S0 → S1 transition polarized along the long molecular axis (N–N direction). The exploration of the possibilities presented by such unconventional chromophores is still in its infancy, with few examples including the sensitized phosphorescence of tBuPh-1166 and the still notable fluorescence observed in 115.67
As for their supramolecular properties, recent research has demonstrated entirely new perspectives originating from nanographene tri- and tetraimides. Whilst PBIs were the perfect molecules for studying self-assembly processes that lead to functional dye aggregates in dilute solutions, organogel materials, and liquid crystals,79,80 they did not offer sufficient binding strengths for host–guest complexes.81 However, these highly desired supramolecular systems can now be achieved more easily with the larger π-surfaces provided by nanographene triimide 110 and tetraimide tBuPh-11.46,61 A particularly striking example is the capability of tBuPh-11 to extract phthalocyanines from their solid pigment form into solution (Fig. 23c).65 This demonstrates that the complete coverage of the π-surface of a phthalocyanine by nanographene tetraimide creates stronger π–π-interactions than the cohesive forces between phthalocyanines in their solid-state pigment particles.
6. Conclusions
For over a century, the synthesis of PADIs has primarily relied on linear synthetic approaches, where a single starting material is converted into the final product through multiple steps. However, the novel annulation strategies reviewed here have contributed to the possibilities of convergent synthesis, enabling access to PADIs with larger π-cores (often referred to as “nanographenes”), structures that were previously inaccessible through conventional linear approaches. These newly available structures include, most notably, multiimides with large symmetrical π-core architectures (Section 4).
Palladium catalysis has proved useful in the synthesis of these PADIs from aromatic boronic acids and dibromoarenes, either by [3 + 3] or [3 + 2] annulation, occasionally accompanied by a cascade of [3 + 3] and [2 + 2 + 2] annulations. Depending on the substrate, fine-tuning was necessary to reach optimal yields. Unlike in case of Pd-catalysed Buchwald–Hartwig aminations, where often specifically tailored ligands are needed, the combination of [Pd2(dba)3]·CHCl3 as the Pd source and PCy3·HBF4 as the ligand provided a general method with good yields of PADI products in most cases. This method has been applied to the synthesis of various PADIs, ranging from simple one-fold annulation for monoimides to two-, three-, four-, and five-fold annulations for the respective bis-, tris-, tetrakis-, and pentakis-(dicarboximides) (Fig. 23).
Unique properties could be integrated into the PADI products, such as long-wavelength absorption/emission, CD/CPL, multi-fold reductions, supramolecular complexation, and n-type semiconducting properties. Further expansion of the substrate combinations for the annulation strategy would unlock new capabilities in PADI-based materials, paving the way for their use in a wide range of cutting-edge applications. The continued exploration of innovative synthetic strategies, combined with a focus on scalability and application-driven design, will be key to advancing the field of PADI chemistry in the years to come.
Author contributions
K. S.: conceptualization, funding acquisition, writing – original draft, writing – review & editing; F. W.: conceptualization, funding acquisition, writing – original draft, writing – review & editing.
Conflicts of interest
There are no conflicts to declare.
Acknowledgements
We acknowledge all co-workers and collaboration partners whose name appear in the reference list for their contribution in our research in the field of synthesis of polycyclic aromatic dicarboximides. We thank the Deutsche Forschungsgemeinschaft (DFG, German Research Foundation) for financial support (Grant No. WU 317/20-1, and WU 317/20-2 ).
References
- H. Langhals, Cyclic Carboxylic Imide Structures as Structure Elements of High Stability. Novel Developments in Perylene Dye Chemistry, Heterocycles, 1995, 40, 477 CrossRef CAS
.
- F. Würthner, Perylene bisimide dyes as versatile building blocks for functional supramolecular architectures, Chem. Commun., 2004, 1564–1579 RSC
.
- Y. Avlasevich, C. Li and K. Müllen, Synthesis and applications of core-enlarged perylene dyes, J. Mater. Chem., 2010, 20, 3814–3826 RSC
.
- C. Huang, S. Barlow and S. R. Marder, Perylene-3,4,9,10-tetracarboxylic Acid Diimides: Synthesis, Physical Properties, and Use in Organic Electronics, J. Org. Chem., 2011, 76, 2386–2407 CrossRef CAS PubMed
.
- A. Nowak-Król and F. Würthner, Progress in the synthesis of perylene bisimide dyes, Org. Chem. Front., 2019, 6, 1272–1318 RSC
.
- S. K. Lee, Y. Zu, A. Herrmann, Y. Geerts, K. Müllen and A. J. Bard, Electrochemistry, Spectroscopy and Electrogenerated Chemiluminescence of Perylene, Terrylene, and Quaterrylene Diimides in Aprotic Solution, J. Am. Chem. Soc., 1999, 121, 3513–3520 CrossRef CAS
.
-
K. Hunger and M. U. Schmidt, Industrial Organic Pigments, 4. Ed, Wiley/VCh, 2018 Search PubMed
.
- A. Narita, X.-Y. Wang, X. Feng and K. Müllen, New advances in nanographene chemistry, Chem. Soc. Rev., 2015, 44, 6616–6643 RSC
.
- Y.-F. Wu, L. Zhang, Q. Zhang, S.-Y. Xie and L.-S. Zheng, Multiple [n]helicenes with various aromatic cores, Org. Chem. Front., 2022, 9, 4726–4743 RSC
.
- H. V. Anderson, N. D. Gois and W. A. Chalifoux, New advances in chiral nanographene chemistry, Org. Chem. Front., 2023, 10, 4167–4197 RSC
.
- A. Laurent, Theorie der organischen Verbindungen, J. Prakt. Chem., 1836, 8, 201–218 CrossRef
.
- M. Kardos, Über einige Aceanthrenchinon- und 1.9-Anthracen-Derivate, Ber. Dtsch. Chem. Ges., 1913, 46, 2086–2091 CrossRef CAS
.
- G. Seybold and G. Wagenblast, New perylene and violanthrone dyestuffs for fluorescent collectors, Dyes Pigm., 1989, 11, 303–317 CrossRef
.
- M. G. Ramírez, S. Pla, P. G. Boj, J. M. Villalvilla, J. A. Quintana, M. A. Díaz-García, F. Fernández-Lázaro and Á. Sastre-Santos, 1,7-Bay-Substituted Perylenediimide Derivative with Outstanding Laser Performance, Adv. Opt. Mater., 2013, 1, 933–938 CrossRef
.
- A. Nowak-Król, K. Shoyama, M. Stolte and F. Würthner, Naphthalene and perylene diimides – better alternatives to fullerenes for organic electronics?, Chem. Commun., 2018, 54, 13763–13772 RSC
.
- J. C. Russell, V. A. Posey, J. Gray, R. May, D. A. Reed, H. Zhang, L. E. Marbella, M. L. Steigerwald, Y. Yang, X. Roy, C. Nuckolls and S. R. Peurifoy, High-performance organic pseudocapacitors via molecular contortion, Nat. Mater., 2021, 20, 1136–1141 CrossRef CAS PubMed
.
- C. Rosso, G. Filippini and M. Prato, Use of Perylene Diimides in Synthetic Photochemistry, Eur. J. Org. Chem., 2021, 1193–1200 CrossRef CAS
.
- T. Weil, T. Vosch, J. Hofkens, K. Peneva and K. Müllen, The Rylene Colorant Family—Tailored Nanoemitters for Photonics Research and Applications, Angew. Chem., Int. Ed., 2010, 49, 9068–9093 CrossRef CAS PubMed
.
- L. Chen, C. Li and K. Müllen, Beyond perylene diimides: synthesis, assembly and function of higher rylene chromophores, J. Mater. Chem. C, 2014, 2, 1938–1956 RSC
.
- H. Vollmann, H. Becker, M. Corell and H. Streeck, Beiträge zur Kenntnis des Pyrens und seiner Derivate, Justus Liebigs Ann. Chem., 1937, 531, 1–159 CrossRef CAS
.
- W. Jiang, Y. Li and Z. Wang, Tailor-Made Rylene Arrays for High Performance n-Channel Semiconductors, Acc. Chem. Res., 2014, 47, 3135–3147 CrossRef CAS PubMed
.
- Y. Zhong, B. Kumar, S. Oh, M. T. Trinh, Y. Wu, K. Elbert, P. Li, X. Zhu, S. Xiao, F. Ng, M. L. Steigerwald and C. Nuckolls, Helical Ribbons for Molecular Electronics, J. Am. Chem. Soc., 2014, 136, 8122–8130 CrossRef CAS PubMed
.
- T. V. Pho, F. M. Toma, M. L. Chabinyc and F. Wudl, Self-Assembling Decacyclene Triimides Prepared through a Regioselective Hextuple Friedel–Crafts Carbamylation, Angew. Chem., Int. Ed., 2013, 52, 1446–1451 CrossRef CAS PubMed
.
- R. Kumar, P. J. Chmielewski, T. Lis, D. Volkmer and M. Stępień, Tridecacyclene Tetraimide: An Easily Reduced Cyclooctatetraene Derivative, Angew. Chem., Int. Ed., 2022, 61, e202207486 CrossRef CAS PubMed
.
- Y. Segawa, T. Maekawa and K. Itami, Synthesis of Extended π-Systems through C–H Activation, Angew. Chem., Int. Ed., 2015, 54, 66–81 CrossRef CAS PubMed
.
- W. Hagui, H. Doucet and J.-F. Soulé, Application of Palladium-Catalyzed C(sp2)–H Bond Arylation to the Synthesis of Polycyclic (Hetero)Aromatics, Chem, 2019, 5, 2006–2078 CAS
.
- H. Ito, Y. Segawa, K. Murakami and K. Itami, Polycyclic Arene Synthesis by Annulative π-Extension, J. Am. Chem. Soc., 2019, 141, 3–10 CrossRef CAS PubMed
.
- S. Seifert, K. Shoyama, D. Schmidt and F. Würthner, An Electron-Poor C64 Nanographene by Palladium-Catalyzed Cascade C−C Bond Formation: One-Pot Synthesis and Single-Crystal Structure Analysis, Angew. Chem., Int. Ed., 2016, 55, 6390–6395 CrossRef CAS PubMed
.
- S. D. Walker, T. E. Barder, J. R. Martinelli and S. L. Buchwald, A Rationally Designed Universal Catalyst for Suzuki–Miyaura Coupling Processes, Angew. Chem., Int. Ed., 2004, 43, 1871–1876 CrossRef CAS PubMed
.
- S. Seifert, D. Schmidt and F. Würthner, A cross-coupling-annulation cascade from peri-dibromonaphthalimide to pseudo-rylene bisimides, Org. Chem. Front., 2016, 3, 1435–1442 RSC
.
- K. Shoyama, M. Mahl, S. Seifert and F. Würthner, A General Synthetic Route to Polycyclic Aromatic Dicarboximides by Palladium-Catalyzed Annulation Reaction, J. Org. Chem., 2018, 83, 5339–5346 CrossRef CAS PubMed
.
- R. S. Ruoff, D. S. Tse, R. Malhotra and D. C. Lorents, Solubility of fullerene (C60) in a variety of solvents, J. Phys. Chem., 1993, 97, 3379–3383 CrossRef CAS
.
- D. Uersfeld, S. Stappert, C. Li and K. Müllen, Practical Syntheses of Terrylene Chromophores from Naphthalene and Perylene Building Blocks, Adv. Synth. Catal., 2017, 359, 4184–4189 CrossRef CAS
.
- X. Zhao, X. Chen, Z. Yuan, H. Zhang, G. Luo, Y. Hu and Y. Chen, Construction of rylene near-infrared absorption dyes with azaperylene monoimide, Dyes Pigm., 2020, 173, 107930 CrossRef
.
- K. B. Wiberg, Application of the pople-santry-segal CNDO method to the cyclopropylcarbinyl and cyclobutyl cation and to bicyclobutane, Tetrahedron, 1968, 24, 1083–1096 CrossRef CAS
.
- M. Mahl, K. Shoyama, J. Rühe, V. Grande and F. Würthner, Tetrachlorinated Polycyclic Aromatic Dicarboximides: New Electron-Poor π-Scaffolds and NIR Emitters by Palladium-Catalyzed Annulation Reaction, Chem. – Eur. J., 2018, 24, 9409–9416 CrossRef CAS PubMed
.
- K. Chen, N. Xue, G. Liu, Y. Liu, J. Feng, W. Jiang and Z. Wang, Sila-annulated terrylene diimides for balanced ambipolar transporting, Chin. Chem. Lett., 2023, 34, 107884 CrossRef CAS
.
- Y. Zagranyarski, L. Chen, Y. Zhao, H. Wonneberger, C. Li and K. Müllen, Facile Transformation of Perylene Tetracarboxylic Acid Dianhydride into Strong Donor–Acceptor Chromophores, Org. Lett., 2012, 14, 5444–5447 CrossRef CAS PubMed
.
- X. Tian, K. Shoyama and F. Würthner, Nitrogen-doped polycyclic aromatic hydrocarbons by a one-pot Suzuki coupling/intramolecular SNAr reaction, Chem. Sci., 2023, 14, 284–290 RSC
.
- K. Bartkowski, P. Z. Crocomo, M. A. Kochman, D. Kumar, A. Kubas, P. Data and M. Lindner, Tandem rigidification and π-extension as a key tool for the development of a narrow linewidth yellow hyperfluorescent OLED system, Chem. Sci., 2022, 13, 10119–10128 RSC
.
- K. Shoyama, M. Mahl, M. A. Niyas, M. Ebert, V. Kachler, C. Keck and F. Würthner, Palladium-Catalyzed [3+2] Annulation of Naphthalimide Acceptors and Thiophene Donors, J. Org. Chem., 2020, 85, 142–149 CrossRef CAS PubMed
.
- D. Alberico, M. E. Scott and M. Lautens, Aryl−Aryl Bond Formation by Transition-Metal-Catalyzed Direct Arylation, Chem. Rev., 2007, 107, 174–238 CrossRef CAS PubMed
.
- K. Bartkowski, A. K. Gupta, T. Matulaitis, M. Morawiak, E. Zysman-Colman and M. Lindner, Using pyrrolizine-fused bipolar PAHs as a new strategy towards efficient red and NIR emissive dyes, Org. Chem. Front., 2023, 11, 755–760 RSC
.
- S. Seifert, D. Schmidt, K. Shoyama and F. Würthner, Base-Selective Five- versus Six-Membered Ring Annulation in Palladium-Catalyzed C–C Coupling Cascade Reactions: New Access to Electron-Poor Polycyclic Aromatic Dicarboximides, Angew. Chem., Int. Ed., 2017, 56, 7595–7600 CrossRef CAS PubMed
.
- X. Cui, C. Xiao, L. Zhang, Y. Li and Z. Wang, Polycyclic aromatic hydrocarbons with orthogonal tetraimides as n-type semiconductors, Chem. Commun., 2016, 52, 13209–13212 RSC
.
- B. Pigulski, K. Shoyama and F. Würthner, NIR-Absorbing π-Extended Azulene: Non-Alternant Isomer of Terrylene Bisimide, Angew. Chem., Int. Ed., 2020, 59, 15908–15912 CrossRef CAS PubMed
.
- H. Xin, B. Hou and X. Gao, Azulene-Based π-Functional Materials: Design, Synthesis, and Applications, Acc. Chem. Res., 2021, 54, 1737–1753 CrossRef CAS PubMed
.
- Y. Han, Z. Xue, G. Li, Y. Gu, Y. Ni, S. Dong and C. Chi, Formation of Azulene-Embedded Nanographene: Naphthalene to Azulene Rearrangement During the Scholl Reaction, Angew. Chem., Int. Ed., 2020, 59, 9026–9031 CrossRef CAS PubMed
.
- X. Zhang, Y. Huang, J. Zhang, W. Meng, Q. Peng, R. Kong, Z. Xiao, J. Liu, M. Huang, Y. Yi, L. Chen, Q. Fan, G. Lin, Z. Liu, G. Zhang, L. Jiang and D. Zhang, Dicyclohepta[ijkl,uvwx]rubicene with Two Pentagons and Two Heptagons as a Stable and Planar Non-benzenoid Nanographene, Angew. Chem., Int. Ed., 2020, 59, 3529–3533 CrossRef CAS PubMed
.
- K. Shoyama, D. Schmidt, M. Mahl and F. Würthner, Electron-Poor Bowl-Shaped Polycyclic Aromatic Dicarboximides: Synthesis, Crystal Structures, and Optical and Redox Properties, Org. Lett., 2017, 19, 5328–5331 CrossRef CAS PubMed
.
- R. Renner, M. Stolte and F. Würthner, Self-Assembly of Bowl-Shaped Naphthalimide-Annulated Corannulene, ChemistryOpen, 2020, 9, 32–39 CrossRef CAS PubMed
.
- K. Menekse, R. Renner, B. Mahlmeister, M. Stolte and F. Würthner, Bowl-Shaped Naphthalimide-Annulated Corannulene as Nonfullerene Acceptor in Organic Solar Cells, Org. Mater., 2020, 02, 229–234 CrossRef CAS
.
- X. Tian, K. Shoyama, B. Mahlmeister, F. Brust, M. Stolte and F. Würthner, Naphthalimide-Annulated [n]Helicenes: Red Circularly Polarized Light Emitters, J. Am. Chem. Soc., 2023, 145, 9886–9894 CrossRef CAS PubMed
.
- B. Mahlmeister, M. Mahl, H. Reichelt, K. Shoyama, M. Stolte and F. Würthner, Helically Twisted Nanoribbons Based on Emissive Near-Infrared Responsive Quaterrylene Bisimides, J. Am. Chem. Soc., 2022, 144, 10507–10514 CrossRef CAS PubMed
.
- B. Mahlmeister, T. Schembri, V. Stepanenko, K. Shoyama, M. Stolte and F. Würthner, Enantiopure J-Aggregate of Quaterrylene Bisimides for Strong Chiroptical NIR-Response, J. Am. Chem. Soc., 2023, 145, 13302–13311 CrossRef CAS PubMed
.
- B. Mahlmeister, R. Renner, O. Anhalt, M. Stolte and F. Würthner, Axially chiral bay-tetraarylated perylene bisimide dyes as non-fullerene acceptors in organic solar cells, J. Mater. Chem. C, 2021, 10, 2581–2591 RSC
.
- M. Grzybowski, B. Sadowski, H. Butenschön and D. T. Gryko, Synthetic Applications of Oxidative Aromatic Coupling—From Biphenols to Nanographenes, Angew. Chem., Int. Ed., 2020, 59, 2998–3027 CrossRef CAS PubMed
.
- L. Đorđević, H. Sai, Y. Yang, N. A. Sather, L. C. Palmer and S. I. Stupp, Heterocyclic Chromophore Amphiphiles and their Supramolecular Polymerization, Angew. Chem., Int. Ed., 2023, 62, e202214997 CrossRef PubMed
.
- B. Pigulski, M. Ximenis, K. Shoyama and F. Würthner, Synthesis of polycyclic aromatic hydrocarbons by palladium-catalysed [3 + 3] annulation, Org. Chem. Front., 2020, 7, 2925–2930 RSC
.
- M. Mahl, K. Shoyama, A. Krause, D. Schmidt and F. Würthner, Base-Assisted Imidization: A Synthetic Method for the Introduction of Bulky Imide Substituents to Control Packing and Optical Properties of Naphthalene and Perylene Imides, Angew. Chem., Int. Ed., 2020, 59, 13401–13405 CrossRef CAS PubMed
.
- M. Mahl, M. A. Niyas, K. Shoyama and F. Würthner, Multilayer stacks of polycyclic aromatic hydrocarbons, Nat. Chem., 2022, 14, 457–462 CrossRef CAS PubMed
.
- B. Pigulski, K. Shoyama, M.-J. Sun and F. Würthner, Fluorescence Enhancement by Supramolecular Sequestration of a C54-Nanographene Trisimide by Hexabenzocoronene, J. Am. Chem. Soc., 2022, 144, 5718–5722 CrossRef CAS PubMed
.
- J. Labella, K. Shoyama, D. Guzmán, T. Schembri, M. Stolte, T. Torres and F. Würthner, Naphthalimide-Fused Subphthalocyanines: Electron-Deficient Materials Prepared by Cascade Annulation, ACS Mater. Lett., 2023, 5, 543–548 CrossRef CAS
.
- K. Menekse, M. Mahl, J. Albert, M. A. Niyas, K. Shoyama, M. Stolte and F. Würthner, Supramolecularly Engineered Bulk-Heterojunction Solar Cells with Self-Assembled Non-Fullerene Nanographene Tetraimide Acceptors, Sol. RRL, 2023, 7, 2200895 CrossRef CAS
.
- M. A. Niyas, K. Shoyama and F. Würthner, C64 Nanographene Tetraimide—A Receptor for Phthalocyanines with Subnanomolar Affinity, Angew. Chem., Int. Ed., 2023, 62, e202302032 CrossRef CAS PubMed
.
- M. A. Niyas, S. Garain, K. Shoyama and F. Würthner, Room-Temperature Near-Infrared Phosphorescence from C64 Nanographene Tetraimide by π-Stacking Complexation with Platinum Porphyrin, Angew. Chem., Int. Ed., 2024, 63, e202406353 CrossRef CAS PubMed
.
- K. Shoyama and F. Würthner, Synthesis of a Carbon Nanocone by Cascade Annulation, J. Am. Chem. Soc., 2019, 141, 13008–13012 CrossRef CAS PubMed
.
- Z.-Z. Zhu, Z.-C. Chen, Y.-R. Yao, C.-H. Cui, S.-H. Li, X.-J. Zhao, Q. Zhang, H.-R. Tian, P.-Y. Xu, F.-F. Xie, X.-M. Xie, Y.-Z. Tan, S.-L. Deng, J. M. Quimby, L. T. Scott, S.-Y. Xie, R.-B. Huang and L.-S. Zheng, Rational synthesis of an atomically precise carboncone under mild conditions, Sci. Adv., 2019, 5, eaaw0982 CrossRef CAS PubMed
.
- F. Würthner, Plastic Transistors Reach
Maturity for Mass Applications in Microelectronics, Angew. Chem., Int. Ed., 2001, 40, 1037–1039 CrossRef
.
- V. Dehm, M. Büchner, J. Seibt, V. Engel and F. Würthner, Foldamer with a spiral perylene bisimide staircase aggregate structure, Chem. Sci., 2011, 2, 2094–2100 RSC
.
- F. Schlosser, M. Moos, C. Lambert and F. Würthner, Redox-switchable Intramolecular π−π-Stacking of Perylene Bisimide Dyes in a Cyclophane, Adv. Mater., 2012, 25, 410–414 CrossRef PubMed
.
- T. A. Barendt, L. Ferreira, I. Marques, V. Félix and P. D. Beer, Anion- and Solvent-Induced Rotary Dynamics and Sensing in a Perylene Diimide [3]Catenane, J. Am. Chem. Soc., 2017, 139, 9026–9037 CrossRef CAS PubMed
.
- B. Lü, Y. Chen, P. Li, B. Wang, K. Müllen and M. Yin, Stable radical anions generated from a porous perylenediimide metal-organic framework for boosting near-infrared photothermal conversion, Nat. Commun., 2019, 10, 767 CrossRef PubMed
.
- G. Heywang, L. Born, H. Fitzky, T. Hassel, J. Hocker, H. Müller, B. Pittel and S. Roth, Radical Anion Salts of Naphthalenetetracarboxylic Acid Derivatives—a Novel Class of Electrically Conducting Compounds, Angew. Chem., Int. Ed. Engl., 1989, 28, 483–485 CrossRef
.
- Y. Kumar, S. Kumar, K. Mandal and P. Mukhopadhyay, Isolation of Tetracyano-Naphthalenediimide and Its Stable Planar Radical Anion, Angew. Chem., Int. Ed., 2018, 57, 16318–16322 CrossRef CAS PubMed
.
- L. Cui, Y. Jiao, A. Wang, L. Zhao, Q. Dong, X. Yan and S. Bai, Regulating morphologies and near-infrared photothermal conversion of perylene bisimide via sequence-dependent peptide self-assembly, Chem. Commun., 2018, 54, 2208–2211 RSC
.
- R. Renner, M. Stolte, J. Heitmüller, T. Brixner, C. Lambert and F. Würthner, Substituent-dependent absorption and fluorescence properties of perylene bisimide radical anions and dianions, Mater. Horiz., 2021, 9, 350–359 RSC
.
- L. Echegoyen and L. E. Echegoyen, Electrochemistry of Fullerenes and Their Derivatives, Acc. Chem. Res., 1998, 31, 593–601 CrossRef CAS
.
- F. Würthner, C. R. Saha-Möller, B. Fimmel, S. Ogi, P. Leowanawat and D. Schmidt, Perylene Bisimide Dye Assemblies as Archetype Functional Supramolecular Materials, Chem. Rev., 2016, 116, 962–1052 CrossRef PubMed
.
- M. Hecht and F. Würthner, Supramolecularly Engineered J-Aggregates Based on Perylene Bisimide Dyes, Acc. Chem. Res., 2021, 54, 642–653 CrossRef CAS PubMed
.
- S. Soldner, O. Anhalt, M. B. Sárosi, M. Stolte and F. Würthner, Donor–acceptor complex formation by social self-sorting of polycyclic aromatic hydrocarbons and perylene bisimides, Chem. Commun., 2023, 59, 11656–11659 RSC
.
|
This journal is © the Partner Organisations 2025 |
Click here to see how this site uses Cookies. View our privacy policy here.