DOI:
10.1039/D4TB01740D
(Paper)
J. Mater. Chem. B, 2025,
13, 1412-1423
Multifunctional hydroxyquinoline-derived turn-on fluorescent probe for Alzheimer's disease detection and therapy†
Received
5th August 2024
, Accepted 9th December 2024
First published on 10th December 2024
Abstract
Understanding molecular motifs that can interfere with amyloid fibrillation through non-covalent interactions is essential for addressing abnormal protein aggregation and associated human diseases. The pursuit of efficient diagnostic and treatment approaches for Alzheimer's disease (AD) has resulted in the development of M8HQ, a multifaceted small molecule turn-on probe derived from 8-hydroxyquinoline with versatile capabilities. M8HQ shows a strong affinity for amyloid beta (Aβ) fibrils, and its ability to target lysosomes enhances therapeutic precision by localizing within these organelles. This localization is essential for restoring cellular balance and maintaining LAMP1 expression, both of which are crucial for addressing AD. It also displays the ability to disaggregate Aβ fibrils and inhibit their formation, thus addressing therapeutic processes in AD progression. M8HQ further blocks reactive oxygen species (ROS)-mediated apoptosis, providing neuroprotective effects. Additionally, it chelates metal ions like Cu(II) and Fe(III), mitigating metal-induced aggregation and oxidative stress. Molecular docking and simulation studies have elucidated the interactions between M8HQ and Aβ, confirming its binding efficacy and stability. These combined properties highlight M8HQ's potential as a comprehensive diagnostic and therapeutic tool for AD.
Introduction
Alzheimer's disease (AD), recognized as the prevailing form of dementia, is currently a worldwide issue of concern. As the world's population ages, dementia becomes more prevalent, with AD accounting for 50–80% of all cases.1 Disease development is assumed to be influenced by various factors, either individually or in combination. According to the amyloid cascade theory, neurodegeneration is mainly caused by amyloid-β (Aβ) peptides, which are distinguished by several pathological indicators, including Aβ. Plaques and neurofibrillary tangles (NFTs) are two prominent characteristics of AD.2–4 The difficulty in determining the source of this neurological issue makes it challenging to find a remedy. Aβ is a brain protein formed by the amyloid precursor protein (APP) proteolysis. APP cleavage by α-/γ-secretases results in shorter, non-amyloidogenic Aβ peptides (ca. 25 residues) with no self-recognition sequence, whereas β-/γ-secretases produce longer, amyloidogenic Aβ40 and Aβ42 (ca. 90% and 9%) soluble monomers, respectively.5 Aβ40/Aβ42 monomers aggregate into oligomers, protofibrils, and fibrils to create thick senile plaques (SP), a histopathological hallmark of AD brains containing foreign species such as proteins and metal ions.6 Fluorescence imaging probes are frequently employed in clinical studies and diagnosing AD.7 More precisely, fluorescent compounds like thioflavin T (ThT) can easily stain Aβ plaques and tau tangles, allowing for microscopic imaging of brain regions.8 The buildup of Aβ plaques and tau tangles occurs before brain shrinkage, with a time gap of at least ten years. Despite the limited association between Aβ plaque levels and cognitive impairments and the fact that tau tangles are not exclusive to AD, these two factors are still considered the most reliable indicators for the early detection of AD. Neurotoxicity from Aβ, mainly through soluble Aβ oligomers, leads to neuronal atrophy and death.9,10 Additional biomarkers have been proposed, and a wide range of small-molecule fluorescence probes are currently under investigation.11
Metals can affect Aβ toxicity through various mechanisms, such as accelerating Aβ aggregation and creating/stabilizing toxic oligomers and Fenton-like reactions at Aβ-bound redox active metal ions Cu and Fe ions.12,13 Overproduction of reactive oxygen species (ROS) by Cu(I/II) and/or Fe(II/III)-Aβ may damage biomolecules and overwhelm antioxidant mechanisms, resulting in oxidative stress and inflammation.12,14 Metal chelators are considered prospective therapy options for AD, owing to the metal ion hypothesis and the potential role of metal–Aβ in AD.6 Although the role of metal–Aβ species in AD pathogenesis is unclear, chemical approaches may offer a potential remedy. Small molecules for this purpose can be developed using rational structure-based design or by selecting natural products and derivatives. Small molecules target metal–Aβ species and change their interaction with Aβ, resulting in benign, unstructured aggregates rather than harmful oligomers.15 Furthermore, these ligands can alter the metal centre geometry of metal–Aβ complexes, lowering ROS levels. Chemical studies can identify chemical interactions between metal ions and Aβ, contributing to AD pathogenesis.16 Exogenous ligands can affect metal–Aβ reactivity, potentially lowering toxicity and establishing it as a therapeutic target.
One of the initial effects of AD pathogenesis is oxidative stress in neuronal cells.17 Overproduction of ROS from the metal–Aβ complex damages DNA and proteins. Additionally, the interaction between Aβ peptide and mitochondria increases oxidative stress. Aβ peptides bind with outer mitochondrial membrane import channels, preventing protein intake for the electron transport chain, causing mitochondrial malfunction and damage, a characteristic of Aβ toxicity in neurons. Mitochondrial damage leads to increased ROS levels, reduced mitochondrial membrane potential (MMP), oxidative phosphorylation impairment, disrupted electron transport chain, and caspase-mediated cell death. Preventing ROS-induced mitochondrial damage is crucial for modifying AD pathogenesis.18 Antioxidant substances reduce ROS and associated toxicity. Our design for M8HQ aims to effectively control metal-independent and metal-dependent Aβ aggregation, ROS production, and mitochondrial damage.
AD is characterized by Aβ peptides forming plaques that impair homeostasis and kill neurons. Lysosomal malfunction can result from Aβ toxicity. The buildup of autophagic vacuoles and decreased cellular waste breakdown show this. LAMP-1, a lysosomal health marker, is commonly dysregulated in these diseases.19 Changes in LAMP-1 expression or function can indicate lysosomal stress and malfunction in amyloid poisoning. LAMP-1 is a crucial protein on lysosome membranes, degrading and recycling biomolecules. LAMP-1 and amyloid toxicity-induced lysosomal dysfunction are essential in neurodegenerative disorders like Alzheimer's.20 Lysosomal failure in AD leads to the buildup of misfolded proteins such Aβ and tau, worsening neurodegeneration. Amyloid intoxication disrupts LAMP-1, which is essential to lysosomal function and the pathophysiology of neurodegenerative illnesses like Alzheimer's. The relationship between amyloid toxicity and LAMP-1 emphasises the necessity of lysosomal integrity in avoiding and treating these diseases.
In this work, M8HQ, a multifunctional hydroxyquinoline and morpholine-functionalized small molecule, was developed to diagnose and treat AD. M8HQ has a significant affinity for amyloid beta (Aβ) fibrils, a key pathogenic hallmark of AD, and targets lysosomes to improve cellular homeostasis. It supports lysosomal function by localizing in lysosomes and maintaining LAMP1 expression at normal levels. Furthermore, M8HQ effectively disaggregates and inhibits Aβ fibril formation, addressing crucial AD progression pathways. The Scheme 1 representation serves as a conceptual framework for our study, illustrating the amyloid fibril formation pathway and M8HQ's ability to disaggregate the fibrils and chelation of Cu2+ and Fe3+ ions, which prevent the formation of Aβ-metal complexes. By doing so, it helps reduce oxidative stress and protects neuronal cells from apoptosis. This visual representation aligns with our findings and integrates various pathways discussed in the manuscript, including Aβ aggregation, metal ion-induced oxidative stress, apoptosis via mitochondrial dysfunction, the neuroprotective role of M8HQ and the lysosome targeting ability. M8HQ's binding efficacy and stability have been confirmed through molecular docking and molecular dynamics simulation experiments with Aβ40, demonstrating improved effectiveness compared to the FDA-approved drug rivastigmine. These qualities imply that M8HQ has the potential to serve as a comprehensive tool for diagnosing and treating AD, opening up new avenues for multifactorial AD treatment.
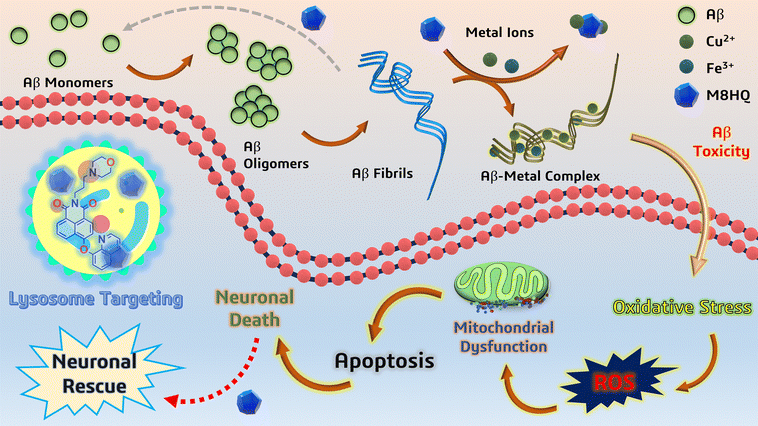 |
| Scheme 1 Schematic representation of neuronal damage pathways and neuroprotection by the multifunctional molecule M8HQ, along with its ability to target lysosome. | |
Results and discussion
The rationale for probe design
To integrate known functions from multiple molecular scaffolds into a single entity, rational design is the foundation for probe design. We synthesized M8HQ, a fluorescent small molecule that interacts with the Aβ peptide. Hydroxyquinoline, a common Aβ-interacting unit, is crucial for creating many Aβ-therapeutic medicines.15 To create a fluorescent probe napthalimide molecular framework was used as the main skeleton. The napthalimide core interacts with Aβ via π–π stacking, while the morpholine group is recognised for lysosome targeting.21 (Scheme 2) Its multi-functionality as metal ion chelators and amyloid aggregation modulators to avoid metal-mediated neurotoxicity makes this probe intriguing and worth investigating.
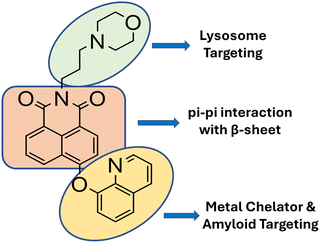 |
| Scheme 2 Overview of rational design approaches for synthesizing the multipotent molecule M8HQ against AD-related pathogenic conditions. | |
Photophysical and biophysical study:
The absorption and emission spectra of the M8HQ molecule were thoroughly investigated. The UV-Vis absorption spectra display the absorbance of 20 μM M8HQ in several solvents, including methanol, acetonitrile, DMF (dimethylformamide), DMSO (dimethyl sulfoxide), water, and 10 mM PBS. The measured peaks reveal how the solvent environment impacts M8HQ's electronic transitions. Acetonitrile shows the highest absorbance, while water and PBS show lower absorbance. This suggests that M8HQ has higher solubility and different electronic interactions in more organic solvents compared to aqueous ones (Fig. S1, ESI†). It was observed that the molecule exhibits an absorption spectrum of 360 nm in a soluble state and 370 in an aggregated state. The emission spectra of M8HQ with different water fractions have also been performed (Fig. S2, ESI†). The fluorescence intensity of M8HQ varies significantly with pH, suggesting its fluorescent properties are sensitive to the protonation state influenced by the environment's pH. At acidic pH, M8HQ shows the highest fluorescence intensity. As pH increases to 3, fluorescence intensity decreases but remains high (Fig. S3, ESI†). Further increases in pH result in a noticeable decrease in fluorescence intensity, but a significant red shift is observed for pH 7.4. At pH 9, fluorescence intensity is lowest. The peak emission wavelength remains relatively stable, though intensity changes with pH. This pH-dependent fluorescence behaviour may be useful for pH-sensitive probe applications. The UV-Vis absorption spectra of M8HQ (20 μM) with different concentrations of Aβ40 peptide (10 to 100 μM) were studied (Fig. S4, ESI†). As Aβ40 concentration rises, the absorbance spectrum increases. These variations reveal interactions between M8HQ and Aβ40, which might indicate binding or structural changes in the presence of Aβ. As Aβ concentration increases, M8HQ's electronic environment may alter owing to complex formation or aggregation effects, leading to higher absorbance values. Fig. 1(A) displays fluorescence spectra of M8HQ and Aβ40 fibrils at different concentrations (2.5 to 20 μM). The interaction between M8HQ and Aβ40 fibrils leads to a concentration-dependent fluorescence intensity, peaking at 436 nm. There is a significant shift in the maximum peak observed after the addition of Aβ40 fibrils, changing from 445 nm to 436 nm. There is a 3-fold increase in fluorescence intensity with a 1
:
1 ratio of Aβ40 fibrils (20 μM) and M8HQ, confirming a strong interaction and the molecule's turn-on behaviour. Significant changes are observed in the fluorescence intensity for the interaction between M8HQ and Aβ40 monomer (Fig. S5, ESI†). As the concentration of Aβ16–21 increases, the fluorescence intensity of M8HQ also increases. Additionally, a slight red shift was observed in the maxima (Fig. S6, ESI†). Further, to check the interaction with metal ions, we have studied different metal ions like Zn2+, Cu2+, Ca2+, Mn2+, Fe2+, Al3+ and Fe3+, but it shows interaction with Cu2+ and Fe3+ only (Fig. S7 and S8, ESI†). From the fluorescence studies, it was observed that beyond 200 μM Cu2+, the fluorescence intensity saturated, suggesting that M8HQ binds Cu2+ ions up to 10 times its concentration. This behaviour implies that the molecule has a robust chelation capacity, even at relatively lower concentrations of M8HQ. The calculated LOD indicates that the fluorescence-based detection of Cu2+ ions is limited to concentrations of 900 nM or higher (Fig. S9, ESI†). To confirm the interaction of Cu2+ with M8HQ, isothermal titration calorimetry (ITC) study was also performed, and good binding interactions were found with maximum binding constant (K) 6.56 × 104 M−1 (Fig. S10, ESI†). The Fig. 1(B) illustrates the effect of M8HQ on the formation of amyloid fibrils induced by Cu2+, as measured by ThT fluorescence intensity. In the absence of M8HQ, the ThT intensity of Aβ40 in the presence of Cu2+ is significantly higher than that of Aβ40 alone, indicating that Cu2+ promotes extensive fibril formation. However, when M8HQ is added to the mixture of Aβ40 and Cu2+, there is a notable decrease in ThT fluorescence intensity. This suggests that M8HQ interferes with the Cu2+-induced fibrillation process. 100 μM Cu2+ solution was used in the reaction for this study. The decrease in intensity also indicates that M8HQ can disaggregate fibrils formed in the presence of Cu2+. The lowest fluorescence intensity observed in the sample containing only Aβ40 serves as a baseline, demonstrating minimal fibril formation under physiological conditions. Additionally, the reduced intensity suggests that M8HQ contributes to the disaggregation of existing fibrils along with preventing new fibril formation. Overall, the data confirm M8HQ's strong interaction with Aβ40 in the presence of Cu2+, highlighting its potential as an anti-aggregation therapeutic for amyloid-related disorders. The MALDI spectra confirmed the metal chelation ability of the multifunctional probe M8HQ, demonstrating the development of a complex between M8HQ and Cu2+ (Fig. S11, ESI†). This complex has a distinct peak, highlighting M8HQ's binding affinity for Cu2+; the peak corresponds to the molecular weight of the M8HQ molecule with the addition of two Cu2+ ions. Further XPS study was also done to check the interaction of Cu2+ and M8HQ. From the Fig. S12 (ESI†) it was clearly seen there is significant interaction present. In Fig. 1(C), the fluorescence intensity of M8HQ rises with increasing concentrations of Fe3+ ions, from 20 μM to 200 μM, demonstrating a turn-on behaviour. This shows that Fe3+ ions interact with M8HQ, most likely via complex formation, increasing its fluorescence. Such concentration-dependent turn-on reveals M8HQ's sensitivity to Fe3+ ions and suggests its possible application as a fluorescence-based sensor for detecting Fe3+ concentrations. The limit of detection (LOD) was found 80 nM from the fluorescence study of 20 μM M8HQ with Fe3+ at different concentrations (Fig. S13, ESI†). To check the interaction between Aβ40 fibrils and M8HQ, a time-resolved photoluminescence (TRPL) study has been performed. From the time-resolved fluorescence decay curves for M8HQ, the data indicate that Aβ40 fibril has a considerable impact on the fluorescence lifetime of the M8HQ molecule (Fig. 1(D)). The native M8HQ molecule has a lifetime of 2.25 ns. After the addition of Aβ40, this increases to 3.35 ns, indicating effective binding. A slight change occurs after adding Aβ16–21, with M8HQ showing a lifetime of 2.56 ns (Fig. S14–S16, ESI†). DFT studies are vital in the structural interaction between the molecule and protein. Here, we performed density functional theory (DFT) calculations at the B3LYP/631G(d,p) level to dig deeper into the electronic structures of M8HQ. The excited state energy levels of the highest occupied molecular orbital (HOMO) and the lowest unoccupied molecular orbital (LUMO) for M8HQ are −5.44 eV and −2.12 eV, respectively. Consequently, the calculated band gap is 3.32 eV (see Fig. S17, ESI†). For Aβ16–21, the ground state energy level of the HOMO is −6.51 eV, while the LUMO is −0.65 eV. The energy difference between the excited state HOMO of M8HQ and the ground state HOMO of Aβ16–21 is very small, indicating a potential for electron transfer from the ground state HOMO of Aβ16–21 to the excited state HOMO of M8HQ (Fig. S18, ESI†). This suggests a possible interaction between these two compounds.
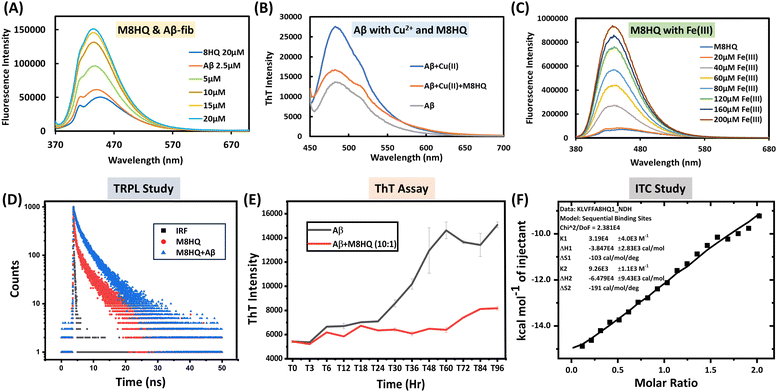 |
| Fig. 1 Photophysical and biophysical studies M8HQ with Aβ40, Aβ16–21, Cu2+ and Fe3+. (A) Fluorescence study of M8HQ with Aβ40 fibrils shows a 3-fold increase in intensity in a 1 : 1 ratio, (B) interaction of M8HQ in metal-mediated Aβ40 toxicity, (C) fluorescence spectra of M8HQ with Fe3+ showing turn on behaviour, (D) time-resolved fluorescence spectroscopy (TRPL) studies demonstrate that the lifetime increases after addition of Aβ40 to the solution of M8HQ. (E) Therapeutic efficacy of M8HQ determined by the ThT assay. M8HQ (1 μM) with Aβ40 (10 μM) at 37 °C for 96 h in PBS (pH 7.4, 10 mM). T0 means time at 0 h, T3 means time at 3 h, and so on. Data are presented as the mean ± SD (standard deviation), n = 3, (F) ITC analysis of Aβ(16-21) with M8HQ showing binding interaction and reaction pathway. The data were recorded at pH 7.0 at 37 °C. | |
Inhibition of Aβ40 accumulation and disaggregation of Aβ40 aggregates
Aβ40 aggregates build up in the brain during the advanced stage of AD and must be removed to start a successful treatment. Small molecules that can dissolve produced Aβ40 aggregates are anticipated to significantly decrease the amyloid burden. The capacity of M8HQ to dissolve pre-existing Aβ40 fibrillar aggregates was assessed. ThT binding assay was performed to evaluate the inhibition of Aβ40 aggregation by the M8HQ. The Aβ40 (10 μM) was incubated alone and independently with 1 μM of M8HQ at 37 °C for 96 h in PBS (pH 7.4, 10 mM). ThT (20 μM) was added to these solutions, and fluorescence was monitored at 480 nm (Fig. 1(E)). The ThT assay between the M8HQ and Aβ40 confirms that the accurate dose (10%) of M8HQ can inhibit the fibrillation process of Aβ40. Up to 48 h, the inhibition property was also studied, and the dose-dependent inhibitory effect of the M8HQ molecule was found (Fig. S19, ESI†). Furthermore, the disaggregation study also tells us that the M8HQ molecule can break down the aggregated Aβ40 fibrils to its monomeric form (Fig. S20, ESI†). ITC was done to check the binding interaction between M8HQ and Aβ16–21 (Fig. 1(F)). The thermodynamic profile displays the binding affinity and stoichiometry under physiological conditions (pH 7.4, 37 °C), revealing that Aβ16–21 binds with M8HQ in a 2
:
1 ratio with a maximum binding constant (K) of 3.19 × 104 M−1, an enthalpy change (ΔH) of −3.847 × 104 kcal mol−1, and a Gibbs free energy change (ΔG) of −3.466 × 104 kcal mol−1. Hence, this reaction is exothermic in nature (Fig. 1(F)). These data detail the interactions between M8HQ, Aβ16–21 peptides, Aβ40 monomer, Aβ40 fibrils and Cu2+, revealing insights into the putative mechanism of M8HQ modulating Aβ aggregation, which is relevant to AD research. This understanding is crucial for exploring the therapeutic potential of M8HQ in amyloid diseases like Alzheimer's.
Crystal structure analysis of M8HQ
The single crystal structure provides a comprehensive understanding of the molecular packing and photophysical properties of M8HQ, which are critical for its stability and binding potential. Additionally, this structure was used as a basis for molecular docking and simulation studies to predict interactions with biological targets. The molecular interactions within the crystal structure of M8HQ reveal a π–π stacking contact of 3.605 Å between adjacent aromatic rings, with a nearly perpendicular alignment of 89.93° (Fig. S21, ESI†). The unit cell of the M8HQ crystal illustrates the molecular organization and various intermolecular interactions, measured at 2.465 Å, 2.785 Å, and 2.610 Å, which contribute to the integrity of the crystal and the arrangement of M8HQ molecules across different crystallographic planes. These planes are indicated by distinct Miller indices, coloured in red, blue, and green. An enlarged view of the crystal packing highlights the regular pattern of M8HQ molecules within the crystal lattice. The head-to-tail arrangement of the molecular stacks suggests possible J-type aggregation of the molecules. Overall, Fig. S21 and Table S1 (ESI†) provide a comprehensive analysis of the crystal structure of M8HQ, detailing the molecular connections, unit cell characteristics, crystallographic orientations, and extended packing configurations that define its structural properties.
MD simulation and binding free energy analysis
The strength and the stability of M8HQ with target proteins were studied based on RMSD (root means square deviation), RMSF (root means square fluctuations), number of H-bonds, and pair distance and compared with the control inhibitor. The average values of the MD parameters were tabulated in Table. The RMSD of the protein backbone provides insight into the protein stability; a higher deviation in RMSD indicates a less stable structure. We have observed the lowest RMSD in the M8HQ bounded target protein compared to the control inhibitor bounded and unbound state (Fig. 2(A)). The deviation in backbone RMSD was observed between 0.5 nm and 1 nm for all three cases. The residual level fluctuation of the target protein was also analyzed with M8HQ and a control inhibitor. Higher fluctuations were observed in the residues from 30 to 50 of target protein upon binding of M8HQ and inhibitor compared to the unbound state. This data confirms those residues’ involvement in the ligand interaction (Fig. 2(B)). In addition to the protein stability, the strength of the binding was also investigated using the number of H-bonds formed and the distance of the pairing between ligands and protein. The number of H-bonds formed by M8HQ and the control inhibitor varied between 1 and 2. However, the persistence of the formed H-bond during 100 ns simulation is higher in M8HQ than in the control inhibitor (Fig. 2(C)). In addition, the distance between M8HQ and control inhibitor pairing with target proteins is also lesser than 0.24 nm over 100 ns simulation, which portrays the strongest interaction (Fig. 2(D)). This data suggests that the formed H-bonds are good enough to bind the ligand molecules inside the binding pocket. The snapshot of the protein–ligand complexes with 20 ns time interval also confirms the interaction of M8HQ and control inhibitor with target protein throughout 100 ns simulation (Fig. 2(E) and (F)). Binding free energy analysis was also performed to study the intermolecular forces involved in the protein–ligand interactions. In this study, the forces involved in the compound interactions, such as van der Waals, electrostatic, polar solvation, and SASA, were tabulated in Table 1 using the MMPBSA binding free energy method. M8HQ attained the lowest binding free energy with the target protein (−99.620 kJ mol−1), which is better than the control (−69.908 kJ mol−1). Following MDS and binding free energy analysis, M8HQ can be a potent inhibitor for amyloid β protein compared to the control inhibitor.
 |
| Fig. 2 (A) Root means square deviation (RMSD) of protein backbone upon binding M8HQ and rivastigmine over 100 ns. (B) Root means square fluctuation (RMSF) of each target protein residue upon binding M8HQ and rivastigmine over 100 ns. (C) Number of hydrogen bonds formed by the M8HQ and rivastigmine with target protein over 100 ns period. (D) Distance between the target protein and ligands (M8HQ and rivastigmine) over 100 ns simulation. (E) and (F) Binding conformation of M8HQ and rivastigmine in the binding pockets of target proteins with 20 ns time interval. The ligand molecules are depicted in different colours inside the binding pocket based on the time frame: red – 0th ns, green – 20th ns, blue – 40th ns, magenta – 60th ns, cyan – 80th ns, orange – 100th ns. | |
Table 1 Binding free energy analysis of M8HQ and control inhibitor with target protein
Different interaction energy (kJ mol−1) |
M8HQ |
Control |
van der Waals energy |
−162.921 |
−98.510 |
Electrostatic energy |
−25.555 |
−13.071 |
Polar solvation energy |
107.278 |
54.452 |
SASA energy |
−18.422 |
−12.778 |
Binding energy |
−99.620 |
−69.908 |
Further, the molecular docking study of M8HQ was also performed with target protein 42 residues amyloid β (PDB ID: 2NAO) and good binding interactions were observed (Fig. S22 and S23, ESI†).
Morphological alteration of Aβ
Field emission transmission electron microscopy (FETEM) was used to examine the morphology of Aβ40 and Cu2+-induced Aβ40 aggregates in the absence and presence of M8HQ. At an optimal concentration in water, M8HQ forms distinct nanodots, with the inset highlighting the individual spherical nanodots (Fig. 3(A)). The nanodot of M8HQ and Aβ40 monomer formed a coaggregate structure, indicating initial aggregation (Fig. 3(B)). Aβ40 fibrils were obtained after incubating 50 μM of Aβ40 at 37 °C for 96 h in PBS (pH 7.4, 10 mM). Aβ40 fibrils are characterized by their elongated and fibrous morphology (Fig. 3(C)). The interaction of M8HQ with Aβ40 fibrils reveals a disrupted or altered fibril structure due to the presence of M8HQ (Fig. 3(D)). At higher concentrations of M8HQ, it forms larger aggregate structures, ranging from 300 to 500 nm in size. These aggregates have also been found to be effective in disrupting the amyloid fibril structure (Fig. S24, ESI†). The SAED (selected area electron diffraction) pattern indicates the crystallinity of M8HQ when combined with Aβ40 aggregates, confirming that the compound's crystallinity was maintained after complex formation, as evidenced by the sharp diffraction rings. (Fig. 3(E)). Cu2+ ions and M8HQ interact with Aβ40 fibrils, leading to a more dispersed clustering of Aβ fibrils. This results in the formation of smaller, less organized aggregates instead of large, dense cluster morphologies compared to the native fibrils. (Fig. 3(F)). Overall, these images suggest that M8HQ interacts with Aβ40 in various forms, potentially altering its aggregation state and structure, which could have implications for understanding its role in amyloid-related diseases.
 |
| Fig. 3 FETEM images of (A) M8HQ in optimal concentration in water forming tiny nanodots, inset showing that molecule has a diameter of 15–17 nm, (B) M8HQ with Aβ40 monomer showing the co-aggregate formation, (C) Aβ40 fibril formed after incubation of 4 days in 10 mM PBS buffer at 37 °C. (D) M8HQ with Aβ40 fibril shows that the nanodots of M8HQ stuck to the surface of amyloid fibrils, (E) SAED pattern of M8HQ molecule with Aβ40 aggregate showing crystallinity of M8HQ remains in the coaggregate (F) Aβ40 fibril with Cu2+ and M8HQ forming smaller, less organized aggregates structure compared to native fibrils. All the images have been taken repeatedly to confirm the formations. | |
Determination of the cytotoxicity of the compound M8HQ:
IMR32 cells were cultured as monolayers. Once they reached confluency, The cells were seeded on 96-well plates (5000 cells per well). Then, the cells were incubated for 24 h and successively treated with different concentrations of the drug Darifenacin hydrobromide and the compound M8HQ. In triplicates. After incubation of 48 h, the drug-containing media in the wells were replaced by a media-containing MTT reagent and additionally incubated for 1.5 h. Next, the MTT reagent containing media was further replaced with DMSO and the plates were incubated in the dark for 15 minutes to facilitate the solubilization of the formazan crystals. Absorbance was recorded with a microplate reader. The absorbance data was analyzed using the software GraphPad Prism to determine the IC50 values of the drug and the compound on different cell lines. The data showed that the compound has no significant cell killing till 12 μM concentration and showed very little cytotoxicity at up to 20 μM concentration (Fig. 4(A)). Cell viability assay of M8HQ up to 100 μM concentration was also performed in HeLa, HEK 293, and IMR 32 cell lines, respectively and very little toxicity was observed (Fig. S25, ESI†).
 |
| Fig. 4 The impact of M8HQ on cell viability, cellular imaging and ROS generation in IMR32 cells. (A) Cell viability assay results show the effect of various concentrations of M8HQ on cell viability. (B) Fluorescence microscopy images showing M8HQ internalization within the cells at different time points (0 h, 2 h, and 6 h). (C) Bar graph illustrating the fold change in intracellular ROS generation for control, Aβ-treated, and Aβ + M8HQ treated cells. (D) Table summarizing the fold change in cellular ROS generation for cells treated with Aβ alone and Aβ with M8HQ. The analysis was carried out using the GraphPad Prism software, and the experimental data were expressed as ± SEM. Additionally, a one-way ANOVA test was used to assess the correlations among the groups. The p-value < 0.05 (*) is statistically significant, whereas p < 0.001 (***) and p < 0.0001 (****) are considered to be highly significant. | |
Cellular uptake study
Since the M8HQ molecule didn’t show any significant cytotoxicity at lower concentrations, we now studied the ability of the compound to be uptaken by the cells. In order to do this, a cellular uptake study was performed by treating IMR32 cells with M8HQ and incubating for 0 h, 1 h, 2 h, 4 h, 6 h, and 8 h (Fig. S26, ESI†). Images depicted that the compound was taken up significantly by the IMR32 cells (Fig. 4(B)). Further, the fluorescence property of the compound may facilitate future bioimaging studies.
Study of the decreased ability of Aβ mediated ROS generation in the presence of M8HQ
In order to understand the potential of M8HQ to reduce the ROS-generating ability of Aβ fibrils in diseased neuronal cells, an ROS generation assay was performed. Cellular ROS levels were detected using 2′,7′-dichlorodihydrofluorescein diacetate (DCFDA)-based flow cytometry analysis.22 The results showed that upon treatment with amyloid-beta for 4 h, the cellular ROS levels increased to almost 3.8 folds in IMR32 cells compared to the untreated cells (Fig. S27, ESI†). On the other hand, M8HQ treatment upregulated the cellular ROS level up to 1.4 folds only compared to untreated cells. Whereas, upon treatment with M8HQ along with amyloid-beta, the cellular ROS level decreased to 0.98 folds with respect to untreated cells, suggesting a great potential of the compound to inhibit the ROS generation ability of toxic Aβ fibrils within the affected cells (Fig. 4(C) and (D)).
Mitochondrial membrane potential (MMP) detection assay
Intracellular ROS levels greatly affect the integrity of mitochondria, leading to the depolarization of the organelle membrane. To evaluate the integrity of the mitochondrial membrane post-treatment, MMP was detected using rhodamine 123 dye. Due to its cationic nature, the dye binds and remains distributed along the negative membrane potential across the inner mitochondrial membrane of respiring mitochondria.23
Flow cytometry-based tests display how M8HQ affects MMP and death in cells treated with amyloid-beta (Aβ). Fig. 5(A) shows untreated cells with a single high-fluorescence peak, indicating healthy MMP. Conversely, Aβ-treated cells show a widened peak with lower fluorescence intensity, indicating mitochondrial membrane potential loss (Fig. 5(B)). In Fig. 5(C), M8HQ-treated cells had a fluorescence peak comparable to untreated cells, showing they do not influence mitochondrial membrane potential. Aβ + M8HQ treatment results in a fluorescence peak similar to untreated cells, demonstrating that M8HQ reduces the drop in MMP caused by Aβ (Fig. 5(D)). Thus, M8HQ can reduce the toxic effects of Aβ fibrils within the cells.
 |
| Fig. 5 Mitochondrial membrane potential detection assay and apoptosis assay results for untreated cells, Aβ-treated cells, M8HQ-treated cells, and Aβ + M8HQ treated cells. (A)–(D) demonstrates the mitochondrial membrane potential detection assay. (E)–(H) shows the apoptotic and non-apoptotic cell population in the presence of Aβ-treated cells, M8HQ-treated cells, and Aβ + M8HQ-treated cells. | |
Analysis of apoptotic cell death
Intracellular ROS generation, followed by a change in mitochondrial membrane potential, leads to apoptotic cell death.24 To study if the cells were dying through apoptosis in the presence of Aβ, we performed an apoptotic cell population detection assay. It was observed that in the presence of Aβ, 76% of cells showed apoptotic death. In Fig. 5(E), live, low-apoptosis untreated cells dominate the lower left quadrant (Q3). Aβ-treated cells exhibit a considerable increase in early and late apoptosis in the upper right (Q2) and lower right (Q4) quadrants (Fig. 5(F)). Fig. 5(G) shows that most M8HQ-treated cells survive just like untreated cells. Finally, Fig. 5(H) indicates that Aβ + M8HQ treatment results in fewer apoptotic cells than Aβ-treated cells, indicating M8HQ's protective impact against Aβ-induced apoptosis. Together, these results suggest that M8HQ can protect cells from Aβ-induced mitochondrial dysfunction and apoptosis, highlighting its potential therapeutic role in conditions associated with amyloid-beta toxicity in Alzheimer's disease.
Colocalization study
Since the structure of the molecule is reported to have a possibility to target the lysosomes, to check if this particular molecule has the property as well or not, a cellular colocalization study was performed.25 Upon treating the cells with M8HQ and staining the cells with lysotracker dye, the confocal microscope images clearly depicted the colocalization of the compound with the lysosome-targeting staining dye. The PCC value of the co-localization study was calculated to be 0.812 (Fig. 6). Thus, this study indicated the capability of the compound to target lysosomes. These images suggest that M8HQ can enter cells and possibly localize in lysosomes, as indicated by the overlap of the green and blue fluorescence signals. This colocalization is critical for understanding the intracellular distribution and potential lysosomal targeting properties of the M8HQ probe intriguing and worth investigating.
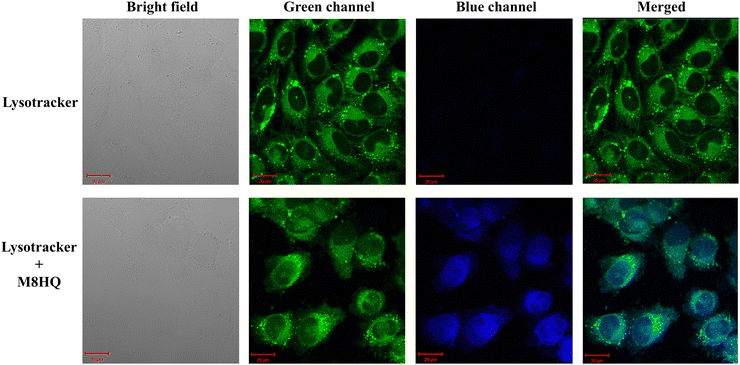 |
| Fig. 6 Cellular localization and co-staining of M8HQ with lysotracker. (PCC value- 0.812) IMR32 cells were seeded for 24 h till cell attachment, then 20 μM M8HQ was added and incubated for 6 h. Then, cells were processed with lysotracker green as per the manufacturer's protocol. Images were captured at a magnification of 63× using a confocal microscope. | |
Study of intracellular protein expression
Aβ plaque is known to increase the LAMP1 (lysosome associated membrane protein-1) expression in AD. Since increased LAMP-1 deposition is linked to increased amyloid plaque deposition in AD, we further studied if our lysosome targeting molecule was able to maintain a lower level of LAMP1 expression post-treatment. Upon the treatment of IMR32 cells with Aβ40, intracellular LAMP1 expression increased 1.56-fold. Whereas, when the cells were treated with Aβ40 in the presence of M8HQ or with M8HQ only, no significant change in LAMP1 expression was observed compared to the untreated cells (Fig. 7). This suggested that the molecule not only targeted the intracellular lysosomes but also facilitated the maintenance of the expression of LAMP1 protein to its normal level.
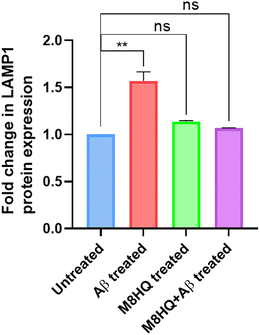 |
| Fig. 7 Change LAMP1 protein expression in IMR32 cells for untreated cells, Aβ-treated cells, M8HQ-treated cells, and Aβ + M8HQ treated cells. | |
Experimental
Synthesis of M8HQ
A solution of 4-bromo-1,8-naphthalic anhydride (277 mg, 1 mmol) in ethanol (5 mL) was prepared. Then, 3-morpholinopropylamine (1.2 mmol) was added to the solution at room temperature. The mixture was subjected to heating at a reflux temperature for a duration of 12 hours. Subsequently, the product was cooled to the ambient temperature and subjected to evaporation while maintaining a lowered pressure. The solution was subjected to chloroform extraction and rinsed with water. Subsequently, it was dehydrated using anhydrous Na2SO4 and then made more concentrated. Then, the compound underwent purification using column chromatography over silica gel, with a 20% ethyl acetate in hexane solution as the eluent. This process resulted in the isolation of pure MNI. M8HQ was produced using a chemical reaction called nucleophilic phenoxide substitution. This reaction involved combining MNI with 8-hydroxyquinoline in the presence of a weak basic, potassium carbonate. (Scheme S1, ESI†) Ultimately, the raw product underwent purification using column chromatography. The final pure product obtained was solid yellow powder which was characterised using 1H NMR and 13C NMR spectroscopy (Fig. S28–S31, ESI†). HPLC analysis was done to check the analytical purity of the sample (Fig. S32, ESI†).
Cell toxicity and BBB permeability prediction
To assess the blood–brain barrier (BBB) efficacy, the toxicity of the synthesized molecule M8HQ and other properties in relation to AD, three different prediction software programs were utilized: Molinspiration Cheminformatics Software, ProTox 3.0, and SwissADME. The results indicated that the molecule demonstrates favourable outcomes in both efficacy and toxicity assessments (see Fig. S33–S35, ESI†).
Molecular dynamics simulation
The molecular dynamics of the compound M8HQ with target protein (40 residues amyloid β PDB ID: 2M4J) were studied using GROMACS 2019.6. The behavioural changes in the target protein's dynamics upon binding compound M8HQ and control inhibitor (rivastigmine) were analyzed using the CHARMM27 force field.26 The protein-M8HQ and protein-rivastigmine complex were retained in a rectangular box with a 1.2 nm distance from the box edge. Systems were solvated with the TIP3P water model, followed by the neutralization of the net charge of the complex system with sodium and chlorine ions. Gromacs module “genion” was used for the neutralization of the system. Subsequently, energy minimization (until the maximum force <10 kJ mol−1), the canonical ensemble, and the isobaric–isothermal ensemble were performed with the steepest descent algorithm, Velocity-rescale thermostat, and Berendsen pressure coupling, respectively. The final molecular dynamics simulation of the complexes was carried out up to 100 ns. After the MD run, the system was analyzed for its stability and strength using MD parameters such as root means square deviation (RMSD), root means square fluctuations (RMSF), number of H-bonds, and pair distance.
MMPBSA – binding free energy analysis
In addition, the binding free energy of the protein-M8HQ was also calculated using the MMPBSA (molecular mechanics Poisson–Boltzmann surface area) method and compared with the control inhibitor complex. MM-PBSA method predicts the binding free energy in terms of van der Waals energy, Electrostatic energy, Polar solvation, and SASA energy from MD trajectories. The binding free energy was calculated with a one ns time interval from the MD trajectory with the help of the g_mmpbsa function.27 The binding free energy (ΔGbind) calculation formula
ΔGbind = ΔEMM + ΔGPBSA − TΔSMM |
where ΔGbind is the calculated average free energy, ΔEMM is the average molecular mechanics’ energy, ΔGPBSA is the solvation free energy, and TΔSMM represents the solute configuration entropy.28
Cell viability assay
IMR32 cells were cultured as monolayers. Once they reached confluency, The cells were seeded on 96-well plates (5000 cells per well). Cells were incubated for 24 hours and then treated with various concentrations of the compound M8HQ: 10 μM, 20 μM, 30 μM, 40 μM, 50 μM, 75 μM, and 100 μM, in triplicates. After a further incubation period of 48 hours, the drug-containing media in the wells were replaced with media containing the MTT reagent and then incubated for an additional 1.5 hours. Next, MTT reagent containing media was further replaced with DMSO and the plates were incubated in the dark for 15 minutes to facilitate the solubilization of the formazan crystals. Absorbance was recorded at 570 nm and 630 nm using a microplate reader. The absorbance data was analyzed using the software GraphPad Prism. The following formula was used to calculate the percentage of viable cells post-treatment.
Cellular uptake study
IMR32 Cells were seeded in 35 mm petriplates. Samples were treated with M8HQ for five different time periods: 0 h, 1 h, 2 h, 4 h, 6 h, and 8 h. Cell images were taken using a fluorescent microscope (ZOE fluorescent cell imager, Bio-Rad).
Colocalization study
Cells were seeded on glass coverslips and incubated for 24 h. Upon attachment, cells were treated with M8HQ for 6 h. Lysosomes of both the treated and untreated cells were stained using a green lysotracker by processing the cells according to the manufacturer's protocol. The cells were fixed with 4% formaldehyde, and images were taken using a confocal microscope. Bright-field and fluorescence microscopy images showing the localization of lysosomes in cells stained with lysotracker alone and lysotracker with M8HQ. Images were captured under bright field illumination and through green and blue fluorescence channels, followed by merging the channels for colocalization analysis.
Reactive oxygen species (ROS) generation assay
Intracellular ROS generation following M8HQ treatment was analyzed using 2′,7′-dichlorofluorescein diacetate (DCFDA) dye. DCFDA is a cell-permeant reagent and in the presence of hydroxyl, peroxyl and some other ROS activities within the cell, it is oxidized to 2′,7′-dichlorofluorescein (DCF), which emits a green fluorescence. For the detection of ROS generation, cells were incubated with 10 μM DCFDA for 30 min followed by being treated with M8HQ, Aβ and M8HQ + Aβ for 4 h. Then the cells were trypsinized and prepared for the analysis using CytoFLEX flow cytometer (Beckman Coulter). This software was further used for data analysis.
Mitochondrial membrane potential detection assay
Rhodamine 123 dye was used to assess the mitochondrial membrane potential post-treatment. IMR32 cells were treated with M8HQ, Aβ and M8HQ + Aβ for 48 h. Then, cells were incubated with rhodamine 123 for 1 h, followed by trypsinization and processing of the cells for further analysis using flow cytometry (BD FACS Melody). Data was further analyzed and represented using FlowJo software and GraphPad Prism.
Apoptosis assay
To determine the apoptotic cell population post-M8HQ, Aβ and M8HQ+ Aβ treatment, cells were seeded and incubated till they were properly attached. Then, all the mentioned groups of compounds were added to the cells, and the cells were further incubated for 48 h. An Annexin-V FITC-containing apoptosis kit was used to mark the apoptotic cell population, which was analysed using a flow cytometer (BD FACS Melody). Data was further analyzed by FlowJo software.
Western blot
To isolate proteins from cells of different treatment groups, RIPA lysis buffer was used. Then, the protein samples were quantified using a kit of BCA reagents. An equivalent amount of different protein samples was loaded on and run through an SDS protein gel. Then the proteins were transferred from gel to a PVDF membrane followed by 2 h incubation with blocking buffer (5% BSA in PBST) and subsequent overnight incubation with LAMP-1 and β-actin 1°-antibody. The membrane was then washed with PBST and further incubated with a secondary antibody for 2 h. The membrane was rewashed with PBST to eliminate the excess unbound antibodies and protein expression signals were developed using a chemiluminescent reagent. Images were acquired using ChemiDoc by Bio-Rad, and the ImageJ software was used to quantify protein bands of the blot.
Conclusions
M8HQ emerges as a promising multifunctional molecule in the fight against AD, offering a comprehensive approach to targeting its multifaceted pathology. By selectively binding to Aβ40 fibrils, M8HQ addresses one of the primary hallmarks of AD. Its lysosome-targeting capability ensures precise localization to these crucial organelles, potentially aiding in restoring cellular homeostasis. Our research demonstrates that M8HQ effectively localizes within lysosomes, sustaining in maintaining LAMP1 expression to normal levels, which generally gets imbalanced in the presence of Aβ, affecting the viability of the cells. Furthermore, M8HQ can disaggregate existing Aβ fibrils and inhibit the fibrillation process, thereby preventing the progression of amyloid pathology. Additionally, M8HQ's metal-chelating properties, particularly its ability to chelate Fe3+ and Cu2+ ions, offer a strategic advantage in mitigating metal-induced aggregation and oxidative stress. This unique combination of targeting Aβ, lysosomal localization, fibril disaggregation, fibrillation inhibition, preventing ROS-mediated cell death, mitochondrial protection and metal ion chelation positions M8HQ as a versatile and effective candidate for AD therapy.
Author contributions
P. G. and P. K. I. designed schemes and experiments; P. G. and S. Mondal synthesized compounds; P. G. performed the biophysical and photophysical experiments; S. Mukhopadhyay and S. S. G. performed cell-culture experiments; P. G., T. K. and S. S. G. performed MD simulation; P.G. compiled and analyzed data, made figures, and wrote the manuscript. All authors reviewed and approved the final version of the manuscript.
Data availability
The data supporting this article have been included as part of the ESI.†
Conflicts of interest
The authors declare no competing financial interest.
Acknowledgements
The authors acknowledge the financial grants from the Department of Science and Technology (DST), New Delhi, India, through the projects DST/CRG/2019/002614, Deity, India no. 5(1)/2022-NANO, ICMR no. 5/3/8/20/2019-ITR, and Max-Planck-Gesellschaft IGSTC/MPG/PG(PKI)/2011A/48. The Department of Chemistry, Centre for Nanotechnology, Central Instrument Facilities (CIF), and Param Ishan, IIT Guwahati are acknowledged for instrument facilities and supercomputer facilities. PG thanks the Prime Minister's Research Fellowship, Ministry of Education, Government of India.
Notes and references
- Alzheimer's Association, Alzheimer's disease facts and figures, Alzheimer’s Dementia, 2023, 19, 1598–1695 CrossRef PubMed
.
- E. Giacobini and G. Gold, Nat. Rev. Neurol., 2013, 9, 677–686 CrossRef CAS PubMed
.
- J. A. Hardy and G. A. Higgins, Science, 1992, 256, 184–185 CrossRef CAS PubMed
.
- J. W. Kelly, Nat. Struct. Biol., 2000, 7, 824–826 CrossRef CAS PubMed
.
- M. P. Murphy and H. LeVine III, J. Alzheimer's Dis., 2010, 19, 311–323 Search PubMed
.
- M. G. Savelieff, A. S. DeToma, J. S. Derrick and M. H. Lim, Acc. Chem. Res., 2014, 47, 2475–2482 CrossRef CAS PubMed
.
- J. Cummings, G. Lee, P. Nahed, M. E. Z. N. Kambar, K. Zhong, J. Fonseca and K. Taghva, Alzheimer's Dement. Transl. Res. Clin. Interv., 2022, 8, e12295 CrossRef PubMed
.
- H. Levine III, Protein Sci., 1993, 2, 404–410 CrossRef PubMed
.
-
P. Ghosh, K. Narang and P. K. Iyer, in Neuroprotection, ed. S. K. Ray, Springer, US, New York, NY, 2024, vol. 2761, pp. 337–354 Search PubMed
.
- P. Ghosh, K. Shokeen, S. Mondal, T. Kandasamy, S. Kumar, S. S. Ghosh and P. K. Iyer, ACS Chem. Neurosci., 2024, 15, 268–277 CrossRef CAS PubMed
.
- B. Bargagna, L. Ciccone, S. Nencetti, M. A. Santos, S. Chaves, C. Camodeca and E. Orlandini, Molecules, 2021, 26, 6015 CrossRef CAS PubMed
.
- P. Baruah, H. Moorthy, M. Ramesh, D. Padhi and T. Govindaraju, Chem. Sci., 2023, 14, 9427–9438 RSC
.
- B. Muthuraj, S. Hussain and P. K. Iyer, Polym. Chem., 2013, 4, 5096–5107 RSC
.
- S. Mondal, Y. Vashi, P. Ghosh, D. Roy, M. Barthakur, S. Kumar and P. K. Iyer, ACS Chem. Neurosci., 2020, 11, 3277–3287 CrossRef CAS PubMed
.
- S. Mondal, Y. Vashi, P. Ghosh, P. Kalita, S. Kumar and P. K. Iyer, ACS Appl. Bio Mater., 2023, 6, 4383–4391 CrossRef CAS PubMed
.
- M. G. Savelieff, G. Nam, J. Kang, H. J. Lee, M. Lee and M. H. Lim, Chem. Rev., 2019, 119, 1221–1322 CrossRef CAS PubMed
.
- C. Guo, L. Sun, X. Chen and D. Zhang, Neural Regener. Res., 2013, 8, 2003–2014 CAS
.
- T. Ashleigh, R. H. Swerdlow and M. F. Beal, Alzheimer's Dementia, 2023, 19, 333–342 CrossRef CAS PubMed
.
- S. Gowrishankar, P. Yuan, Y. Wu, M. Schrag, S. Paradise, J. Grutzendler, P. De Camilli and S. M. Ferguson, Proc. Natl. Acad. Sci. U. S. A., 2015, 112(28), E3699–E3708 CrossRef CAS PubMed
.
- M. Barrachina, T. Maes, C. Buesa and I. Ferrer, Neuropathol. Appl. Neurobiol., 2006, 32, 505–516 CrossRef CAS PubMed
.
- X. Kong, L. Di, Y. Fan, Z. Zhou, X. Feng, L. Gai, J. Tian and H. Lu, Chem. Commun., 2019, 55, 11567–11570 RSC
.
- B. Dong, X. Song, X. Kong, C. Wang, N. Zhang and W. Lin, J. Mater. Chem. B, 2017, 5, 988–995 RSC
.
- G. Juan, M. Cavazzoni, G. T. Sáez and J.-E. O’Connor, Cytometry, 1994, 15, 335–342 CrossRef CAS PubMed
.
- H. Kadowaki, H. Nishitoh, F. Urano, C. Sadamitsu, A. Matsuzawa, K. Takeda, H. Masutani, J. Yodoi, Y. Urano, T. Nagano and H. Ichijo, Cell Death Differ., 2005, 12, 19–24 CrossRef CAS PubMed
.
- B. Dong, X. Song, X. Kong, C. Wang, N. Zhang and W. Lin, J. Mater. Chem. B, 2017, 5, 988–995 RSC
.
- K. Hart, N. Foloppe, C. M. Baker, E. J. Denning, L. Nilsson and A. D. MacKerell, J. Chem. Theory Comput., 2012, 8, 348–362 CrossRef CAS PubMed
.
- R. Kumari, R. Kumar, Open Source Drug Discovery Consortium and A. Lynn, J. Chem. Inf. Model., 2014, 54, 1951–1962 CrossRef CAS PubMed
.
- P. A. Kollman, I. Massova, C. Reyes, B. Kuhn, S. Huo, L. Chong, M. Lee, T. Lee, Y. Duan, W. Wang, O. Donini, P. Cieplak, J. Srinivasan, D. A. Case and T. E. Cheatham, Acc. Chem. Res., 2000, 33, 889–897 CrossRef CAS PubMed
.
Footnote |
† Electronic supplementary information (ESI) available: Reaction scheme, NMR, HPLC, MALDI, single crystal, UV-Vis, fluorescence, TRPL, ITC, XPS, DFT, molecular docking, MTT, confocal microscopy, flow cytometry. CCDC 2374847. For ESI and crystallographic data in CIF or other electronic format see DOI: https://doi.org/10.1039/d4tb01740d |
|
This journal is © The Royal Society of Chemistry 2025 |
Click here to see how this site uses Cookies. View our privacy policy here.