Optimal size of Fe3O4 nanoparticles for different crops depends on the unique nanoscale microstructure of plant leaves under rainy conditions†
Received
19th August 2024
, Accepted 23rd October 2024
First published on 7th November 2024
Abstract
Metal-based nanoparticles (NPs) have garnered attention as a potential micronutrient nano-fertilizer. Most studies have focused on the effects of individual NP size on environmental risks and the uptake, translocation, and biological progress of NPs in plants. However, there is a lack of research on the effects of NPs of different sizes and their interactions with the nanoscale layers of plant leaves (hereafter, nanosheets), which may affect adhesion ability, anti-leaching properties, release rate, and fertilizer efficiency. In this study, various sizes (10, 20, 50, 100 nm, and 10 μm) of Fe3O4-NPs (Fe3O4-NPs) were applied to peanut (Fe strategy I, dicotyledon) and maize (Fe strategy II, monocotyledon) leaves to quantitatively compare their fertilization efficiency and anti-leaching effects. The optimal size for different crop leaves differed due to the distinct microstructures of the nanosheets on the leaf surface. In peanut, the optimal size was 50 nm, resulting in superior dry weight (1.32 g per plant), leaf iron concentration (483 μg g−1 DW), and adhesion amount (0.039 mg per plant). For maize, the optimal size was found to be 100 nm, leading to increased dry weight (1.98 g per plant), leaf iron concentration (258 μg g−1 DW), and adhesion amount (0.061 mg per plant). A model was developed to simulate the force and work exerted by Fe3O4-NPs of different sizes on leaf nanosheets, resulting in the optimal size consistent with the experimental findings. These findings will guide the selection of the optimized NP size for different leaves, thereby enhancing the efficiency of nano-fertilizer utilization and facilitating the development of new types of nano-fertilizers.
Environmental significance
Currently, there is a prevailing consensus that nano-fertilizers and nano-pesticides can effectively adhere to plant leaves, thereby reducing leaching and mitigating environmental risks. However, the interaction mechanisms and size-dependent behaviors of nanomaterials on the microstructure of plant leaves remain unexplored. In this study, we found that the optimal nanoparticle size for different crops depends on the unique nanoscale microstructure of plant leaves, and a specific model was developed to validate these results. These findings clearly indicate that optimizing nanoparticle size may enhance the retention of applied nanoparticles on leaf surfaces, thereby mitigating their environmental dispersal and associated risks.
|
1. Introduction
Iron (Fe) is a vital micronutrient in plant growth, crop yield, and nutrient fortification, as it plays direct roles in the synthesis of chlorophyll, transport of photosynthetic electrons, synthesis of key enzymes, and other metabolic processes.1–3 Dicotyledonous plants (such as peanuts, soybeans, citrus, and pear) exhibit lower Fe absorption efficiency due to their inability to secrete mugineic acids, leading to physiological Fe deficiency that necessitates a correction through Fe supplementation.4,5 However, conventional Fe fertilizers (e.g., FeSO4 and chelate Fe fertilizers) exhibit certain limitations despite their rapid efficiencies.6 For example, the susceptibility of Fe2+ oxidation to Fe3+ by environmental influences, such as rainfall, leads to potential leaching loss, short-duration fertilizers, and the frequent need for reapplication, which increases labor costs. Therefore, new materials and innovative technologies are needed to overcome the limitations associated with traditional Fe fertilizers and traditional agriculture.7–9
Nanotechnology brings a revolutionary perspective to the field of modern agriculture offering potential solutions for overcoming the limitations associated with conventional fertilizers and addressing the challenges posed by a changing global climate and escalating food demand.10–13 A nano-Fe fertilizer is a foliate fertilizer that exhibits remarkable attributes, including stability, resistance to oxidation, a low application dosage, strong adhesion, and long-lasting efficacy. Moreover, it offers additional advantages.14–16 For example, Fe3O4 nanoparticles (NPs) increase the Fe content in the crease tissue and endosperm components of wheat grains, demonstrating their potential to enhance grain quality.17 Root nodules induced by Fe-based nanomaterials (NMs) regulate flavonoids to improve the growth and fruit quality of Glycine max.18 Furthermore, Fe and its composite/combined metal-oxide NMs mitigate heavy metal contamination and remediate soil pollution.19,20 Superparamagnetic Fe oxide NPs loaded with usnic acid as bioherbicide nanocarriers mitigate soil pollution, facilitate the homeostatic behavior of usnic acid, and minimize disruption to the microbial community of the soil, thus promoting sustainable agricultural production and environmental benefits.21 Fe-based NPs have gained increasing attention due to the abundant Fe resources, as well as their significant role in promoting sustainable agriculture and facilitating environmental remediation.22–24
The presence of various particle sizes within a specific type of NM is significant, as the size-dependent effect of nanomaterials (NMs) plays a pivotal role in governing the transformation, transportation, accumulation, and bioavailability of NPs and their corresponding ions within plants.25 This is because there are various physiological size thresholds and in vivo size-dependent interactions that impose physical barriers on the ingestion pathways.26 Some studies have demonstrated that smaller NMs exhibit enhanced fertilizer efficacy at an appropriate fertilization dosage.27,28 For example, smaller zinc oxide particles (40 nm vs. 200 nm) are absorbed and migrated at the highest rates in wheat cells, thereby highlighting the size effect.29 In a study that compared the effects of spraying leaves with different sizes of γ-Fe2O3 NMs (4–15, 8–30, and 40–215 nm) on the growth and physiology of soybean seedlings found that the smallest ones (4–15 nm, 30 mg L−1) had the most significant promoting effect.30 However, other studies have shown that smaller NMs tend to penetrate cells more easily and are more phytotoxic at elevated dosages.31 The optimal size range remains uncertain, and it is unclear whether smaller sizes are always advantageous. Definitive conclusions regarding the size optimization have yet to be reached.
The duration that NMs adhere to leaf surfaces plays a crucial role in determining fertilizer efficiency. This process is affected by the size of the NMs and the microstructure of leaves. The leaf surfaces of plants exhibit distinct nanoscale layers of various sizes and distributions (hereafter, nanosheets). These tiny structures collectively form a topographical landscape resembling “small hill” and “concave void space” (see Fig. S1 and S2†), exhibiting a pronounced manifestation of the “lotus leaf effect”. This type of biomimetic nanostructure has also been investigated in hydrophobic and self-cleaning materials.32–34 Notably, a variety of crop types exhibit significant variation in leaf microstructure. For example, the height/void size of the nanosheets of peanut and maize leaves are 172/622 nm and 115/942 nm, respectively (Fig. S1, S2 and Table S1†). Importantly, different microstructures on a leaf will significantly affect the interactive forces between nanoscale materials and the nanosheets of the leaf surface, thereby affecting their anti-leaching capacity, adsorption efficiency, and migration within leaves. However, there has been a lack of research on the interaction between NPs of different sizes and leaf nanosheets, as well as the relationship between the size effect and anti-leaching ability.
Selection of an appropriate Fe3O4 particle size is crucial, considering the potential interactions with different leaf microstructures. In this study, we selected peanut and maize leaves with two distinct leaf nanosheet structures and applied Fe3O4 particles of various nano- and micron-level sizes to compare fertilizer efficiency and anti-leaching effects. The optimal size range was determined by calculating the force/work of Fe3O4 particles on the leaf surfaces using different nanosheet models and validating the fertilizer efficiency of the Fe3O4 NPs with different particle sizes in peanut and maize cultivation experiments. This research aimed to guide further optimization and application of NMs or nano-carrier fertilizers to address the challenges posed by rainy and other extreme weather environments.
2. Materials and methods
2.1 Nano-iron material information and characterization
Fe3O4-NPs (10/50/100 nm, purity 99.5%, density 4.8–5.1 g mL−1) were obtained from Shanghai Macklin Biochemical Technology Co., Ltd. (Macklin, Shanghai, China). Other NPs of 20 nm (purity 99.5%, density 4.8–5.1 g mL−1) and 10 μm (purity 99%, density 1.8 g mL−1) were purchased from Shanghai Maogon Nano Technology Co., Ltd. (Maogon, Shanghai, China). These nano-Fe materials are primitive and stable, without any additional surface modification substances, avoiding the surface charge and electrostatic effects. The morphology and size of the nano-Fe materials were characterized via transmission electron microscopy (TEM, FEI Tecnai G2 F30, FEI, Lausanne, Switzerland) and scanning electron microscopy (SEM, SU8082, Hitachi Japan, Tokyo, Japan), while the size distribution statistics of the Fe3O4 particles were analyzed with Nano Measurer 1.2 software.
2.2 Experimental design and sample treatment analysis
Information and cultivation of peanut and maize seeds.
The peanut (Arachis hypogaea L.) variety Luhua no. 8 was obtained from Shandong Peanut Research Institute in China and the maize (Zea mays L.) variety Zhengdan 958 was obtained from the Chinese Academy of Agricultural Sciences. Firstly, peanut and maize seeds were sterilized with 3% H2O2 (AR) for 30 minutes, followed by immersion in deionized water for 6 hours. Subsequently, they were transferred to a plastic container containing moist filter paper and subjected to germination under dark conditions at 25 °C for 3 days. The germinated peanut and maize seeds (3–5 cm radicles) were transferred to a hydroponic system containing 1/2 strength modified Hoagland solution (pH 6.0–6.5, refer to Table S2† for the composition of the modified Hoagland solution). The hydroponic system was continuously aerated and placed in an automatic plant growth chamber for 10 days under controlled cultivation conditions: day and night cycle of 16/8 hours, temperature maintained at 25 °C, humidity set at 50%, and light photon flux density of 100 μmol m−2 s−1. Finally, 12 healthy growing peanut and maize seedlings were selected. The cotyledons were removed, and then they were transferred to plastic culture pots with 10 L iron-free modified Hoagland solution.
Experimental treatment.
In this experiment, the treatments were Fe starvation (CT, foliar spray of 0.1% Tween 80, as a control) and leaf application of Fe materials of varying particle size to Fe-starved plants. The particle sizes ranged from 10 nm, 20 nm, 50 nm, and 100 nm for Fe3O4-NPs in the nanoscale range to 10 μm for Fe3O4-NPs in the micron-scale range. They were labeled Fe3O4-10 nm, Fe3O4-20 nm, Fe3O4-50 nm, Fe3O4-100 nm and Fe3O4-10 μm, respectively. The concentration of the Fe fertilizers was 300 mg L−1 Fe equivalent (i.e. 414 mg L−1 Fe3O4-NPs), in reference to the recommended agricultural dosage.35 Fe fertilizers were sprayed three times on the leaves once every 10 days (Fig. S3†), with a volume of 1.67, 3.33, and 5 mL per plant for each successive application on days 23, 33, and 43, respectively, based on previous research to ensure optimal leaf surface wetting without runoff.35 An aerated Fe-deficient nutrient solution was renewed every 10 days, and the peanut/maize plants were harvested after growing for 53 days.
Simulated rainfall experiment.
After 1 h of foliar fertilization following the aforementioned experiments, simulated rainfall was induced by spraying 100 mL water onto the leaves of six plants. The volume of simulated rainfall was 10 times that of the fertilizer applied (10 mL), following the methods described by Li et al.36 and Chen et al.35 The rainfall height of the spray is approximately 20 cm above the plants. To better simulate the actual conditions of crop fields, the simulated rainfall was repeated every fifth day from the first day of applying the foliar fertilizer (i.e., days 23, 28, 33, 38, 43, and 48 after sowing) (Fig. S3†). Peanut/maize samples were harvested on day 53 after sowing.
Plant sampling.
A minimum of 12 plantlets was used for each treatment. The plant samples were collected and rinsed with 0.1 M HCl (twice), followed by distilled water (once) to remove the attached nano-Fe particles on the leaf surface; the washing method was effective for removing Fe NPs attached to the leaf surface according to a previous experiment.35 At least four seedlings were selected from each experiment for the peanut growth, biomass, and nutrient analyses. Six seedlings were used for the leaf SPAD and photosynthesis tests, while the remaining seedlings were used to characterize the leaf tissue and for other analyses. The primary phenotype of Fe-deficient plants is interveinal chlorosis of young leaves (Fig. 4 and S12†). However, this symptom may not be apparent in older leaves as it developed when the seedlings were cultivated in 1/2 strength modified Hoagland solution (Fig. S11/S13†). Therefore, we separately tested the old leaves (leaves of seedlings grown in 1/2 strength nutrient solution) and the young leaves (leaves produced in seedlings grown in Fe-free nutrient solution, and without cotyledons).
Plant analysis.
The ESI† provides a detailed analysis of the plant parameters, including root biomass, aboveground biomass, morphology, nutrient analysis of the tissues, SPAD/photosynthesis parameters of the leaves, and statistical analysis of the nanosheet structure and stomatal size in leaves.
2.3 Adhesion/anti-leaching tests and the contact angle model of nano-Fe particles on leaf surfaces
Adhesion/anti-leaching test.
After 10 days of Fe starvation, the plants were subjected to a leaf adhesion and anti-leaching assessment of the nano-Fe particles. Briefly, the test is divided into four stages. See the ESI† and Fig. S4 for more details. Three crucial quantification parameters were considered: the effective spraying amount was the difference in Fe content between the leaves of plants in state A and those in state B; the leaching amount was the difference in Fe content between the leaves of plants in state B and those in state C; the effective adhesion amount was the difference in Fe content between the leaves of plants in state C and those in state D.
Contact angle model.
Briefly, a tiny nano-Fe fertilizer droplet on a leaf exhibits three types of surface tension (i.e., γsg for the solid–gas interface tension, γsl for the solid–liquid interface tension, and γlg for the liquid–gas interface tension). γsg and γlg form the θ angle, and these three surface tensions comply with the wetting equation (Fig. S6†). The smaller the θ value, the more easily the Fe fertilizer solution adheres to leaves. The adhesion work (Wa) was quantified and calculated by measuring γlg and θ to facilitate a better comparison of the adhesive abilities of the different Fe3O4 particles (refer to the ESI†).
2.4 Comparative analysis of the force/work of different sizes of Fe3O4 particles on peanut and maize leaves
Based on TEM (Fig. 2) and previous XRD data,35 we determined that Fe3O4 particles possess a cubic structure. Assuming that the surface of a leaf is inclined at an angle of 45° from the ground, we developed a model wherein the Fe3O4 particles slid on the leaf's surface, following the approach described by Li et al.36 According to this characterization, peanut had nanosheets with a height of 172 nm and a void size of 622 nm (Fig. S1 and Table S1†); for maize, these values were 115 nm and 942 nm, respectively (Fig. S2 and Table S1†). Therefore, six hypothetical scenarios were classified (Fig. 1 and S7†): (1) when the size of Fe3O4 particles exceeded 700 nm (i.e., 10 μm), they would remain on top of the peanut leaf nanosheets but could fall into the void positions; (2) when their size exceeded 1000 nm (i.e., 10 μm), they would remain on top of the maize leaves and would not fall into the void positions; (3) when they were <700 nm (i.e., 10/20/50/100 nm), the height of the nanosheets of peanut leaves increased while the void size decreased and thus NPs were subject to a strong blocking effect, making it difficult for them to slide down; (4) when they were <1000 nm, the NPs would slide down from the void positions and suffered a low blocking effect; (5) when the nanosheets of peanut leaves were taller (172 nm) and overlapped two 100 nm Fe3O4-NPs, the work performed by the second Fe3O4-NPs to overcome friction is crucial and directly impacts the stability of other particles; (6) when the nanosheets of the maize leaves were shorter (115 nm) and overlapped only one 100 nm Fe3O4-NP, the work performed by the first Fe3O4-NPs to overcome friction is crucial and directly impacts the stability of other particles. For comprehensive insight into the force and work analyses, as well as the associated calculations, refer to the ESI.†
 |
| Fig. 1 Schematic diagram illustrating the force/work analysis of the Fe3O4-NPs of different sizes on various crop leaf nanosheets. Force analysis at the surface of (A) peanut leaf nanosheets (size: a > 700 nm, which is also 10 μm), (B) maize leaf nanosheets (size: a > 1000 nm, which is also 10 μm), (C) peanut leaf nanosheets (size: a < 700 nm, which is 10–100 nm), and (D) maize leaf nanosheets (size: a < 1000 nm, which is 10–100 nm). Work analysis at the surface of (E) peanut leaf nanosheets (size: a < 700 nm, which is 10–100 nm), and (F) maize leaf nanosheets (size: a < 1000 nm, which is also 10–100 nm). The relevant calculations and the force and work analyses are provided in the ESI.† | |
2.5 Statistical analysis of the experimental data
Experiments were repeated with a minimum of four duplicate samples (n ≥ 4). All data were analyzed using analysis of variance (ANOVA) and IBM SPSS 19.0 software (SPSS Inc., Chicago, IL, USA), and are presented as mean ± standard deviation. One-way ANOVA was used to assess the effect of different types of nano-Fe fertilizers on the dependent variables. Duncan's multiple range test was used to detect the differences. A p-value < 0.05 was considered significant.
3. Results
3.1 Material characterization
TEM and SEM characterization revealed that the Fe3O4-NPs had a cubic shape (Fig. 2), in line with previous XRD results.35 The average sizes of Fe3O4-10 nm, Fe3O4-20 nm, Fe3O4-50 nm, Fe3O4-100 nm and Fe3O4-10 μm were 12 nm, 17 nm, 42 nm, 91 nm and 9 μm, respectively. These results demonstrate that the sizes of the NPs met the basic size requirements for the experiments, specifically within the ranges of 10–100 nm and 10 μm, confirming that the main elements of these materials were Fe and O, with no other elements. Hydrated particle sizes of 0.1% Tween 80, Fe3O4-10 nm, Fe3O4-20 nm, Fe3O4-50 nm, Fe3O4-100 nm and Fe3O4-10 μm were 25 ± 3 nm, 379 ± 11 nm, 416 ± 23 nm, 1210 ± 70 nm, 1393 ± 44 nm and 2060 ± 421 nm, respectively. And zeta potential values of Fe3O4-10 nm, Fe3O4-20 nm, Fe3O4-50 nm, Fe3O4-100 nm and Fe3O4-10 μm were −14.7 ± 2.4 mV, −15.4 ± 2.5 mV, −18.2 ± 1.4 mV, −15.9 ± 1.9 mV, and −17.3 ± 2.2 mV respectively. Their values were similar to that of 0.1% Tween 80 (−15.3 ± 2.0 mV).
 |
| Fig. 2 TEM and SEM, size distribution, and EDS characterization of the different sizes of the Fe3O4-NPs: 10 nm (A1, A2, and A3), 20 nm (B1, B2, and B3), 50 nm (C1, C2, and C3), 100 nm (D1, D2, and D3), and 10 μm (E1, E2, and E3). | |
3.2 Peanut and maize growth, Fe concentration, and photosynthesis are affected by the different sizes of Fe3O4-NPs
Under simulated rainfall conditions, the fertilizer efficiency of Fe3O4-10 μm was the lowest, and no significant differences in the aboveground biomass or plant height were observed between the peanut/maize group (Fe3O4-10 μm) and the control group (Fe deficiency treatment) as shown in Fig. 3. Spraying Fe3O4-50 nm on the peanut leaves increased the aboveground biomass and plant height. However, spraying Fe3O4-100 nm enhanced the aboveground biomass and height of maize. These results indicate that Fe3O4 NPs of 50 nm and 100 nm are the optimal sizes for promoting growth in peanut and maize plants under simulated rainfall conditions.
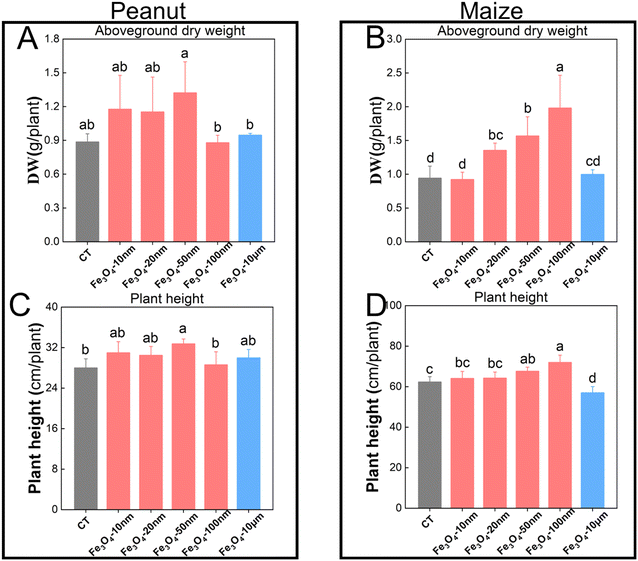 |
| Fig. 3 Effects of different sizes of Fe3O4-NPs on the growth status of peanut/maize seedlings 53 days after sowing under simulated rainfall conditions: aboveground dry weight (A) and plant height (C) of peanut plants; aboveground dry weight (B) and plant height (D) of maize plants CT-0.1% Tween 80 solution, DW, dry weight. Mean ± standard deviation (n = 4) values shown in the bar diagram followed by different letters are significantly different according to Duncan's multiple range test (p < 0.05). | |
Fe concentration, SPAD, and photosynthesis in leaves are key indicators for assessing Fe deficiency, particularly in young leaves. Spraying the leaves with Fe3O4-NPs (10–100 nm) significantly increased the Fe concentration of young peanut and maize leaves under the simulated rainfall conditions (Fig. 4), consistent with previous experimental results.35 Notably, Fe3O4-10 μm did not significantly increase the Fe concentration due to its larger size impeding passage through the stomata and hindering access to mesophyll cells. This may be attributable to the small stomatal openings of peanut/maize with widths of less than 2 μm (Fig. S1/S2 and Table S1†). Similarly, Fe3O4-50 nm had the greatest effect on increasing Fe concentration in peanut leaves, while Fe3O4-100 nm had the greatest effect on maize. Although SPAD and photosynthesis both increased (Fig. 4), the increases were not as significant as that of Fe concentration. This is because the entry of Fe3O4-NPs into the leaves cannot be timely degraded and bio-utilized; the aggregates of undegraded Fe NPs in these leaves have been characterized by TEM-EDS in previous studies.35 These results are directly related to the adhesion amount and anti-leaching ability of the Fe3O4-NPs on the leaves of different crops.
 |
| Fig. 4 Effects of different sizes of Fe3O4-NPs on the physiological parameters of peanut/maize leaves 53 days after sowing under simulated rainfall conditions: Fe concentration (A), SPAD (C), and Pn (E) of young peanut leaves; Fe concentration (B), SPAD (D), and Pn (F) of young maize leaves. CT, 0.1% Tween 80 solution, DW, dry weight, Pn, net photosynthetic rate. Mean ± standard deviation (n = 6) shown in the bar diagram followed by different letters are significantly different according to Duncan's multiple range test (p < 0.05). | |
3.3 Adhesion and anti-leaching effects of Fe-NPs
The 20–50 nm Fe3O4-NPs had the greatest adhesion (0.044 and 0.039 mg per plant) and the lowest leaching (0.00062 and 0.0015 mg per plant) on peanut leaves (Fig. 5). SEM-EDS results show that Fe3O4-NPs (range 20–50 nm) attached to the surface of the peanut leaves were the strongest under rainfall conditions. That is, this size class had the best adhesion and the strongest anti-leaching ability to cope with rainfall; the performance of Fe3O4-10 μm was relatively poor.
 |
| Fig. 5 Effects of different sizes of Fe3O4-NPs on adhesion and anti-leaching of peanut leaves: the effective foliar application amount (A), the leaching amount (B), and the effective adhesion amount (C). SEM images of Fe3O4-NP deposits on peanut leaves 53 days after sowing under simulated rainfall conditions: CT (D); 10 nm (E); 20 nm (F); 50 nm (G); 100 nm (H); 10 μm (I). The Fe–Kα energy spectrum distribution pattern was obtained by EDS below the corresponding SEM: CT (d); 10 nm (e); 20 nm (f); 50 nm (g); 100 nm (h); 10 μm (i). CT, 0.1% Tween 80 solution. Mean ± standard deviation (n = 6) shown in the bar diagram followed by different letters are significantly different according to Duncan's multiple range test (p < 0.05). | |
The results demonstrated that Fe3O4-NPs within the size range of 50–100 nm had the highest amount of adhesion (0.059 mg per plant and 0.061 mg per plant) and the lowest amount of leaching (0.024 mg per plant and 0.012 mg per plant) on maize leaves (Fig. 6B/C). SEM-EDS characterization results further confirmed that Fe3O4-NPs, ranging from 50 to 100 nm in size, displayed the most pronounced signal when attached to the surface of maize leaves under rainfall conditions (Fig. 6D–I). These results suggested that Fe3O4-NPs with a size range of 50–100 nm possessed superior adhesion ability and robust anti-leaching properties for protecting maize leaves against rainfall. In contrast, Fe3O4-10 μm demonstrated comparatively inferior performance.
 |
| Fig. 6 Effects of different sizes of Fe3O4-NPs on adhesion and anti-leaching of maize leaves: the effective foliar application amount (A), the leaching amount (B), and the effective adhesion amount (C). SEM images of Fe3O4-NP deposits on maize leaves 53 days after sowing under simulated rainfall conditions: CT (D); 10 nm (E); 20 nm (F); 50 nm (G); 100 nm (H); 10 μm (I). The Fe–Kα energy spectrum distribution pattern was obtained by EDS below the corresponding SEM: CT (d), 10 nm (e), 20 nm (f), 50 nm (g), 100 nm (h), 10 μm (i). CT, 0.1% Tween 80 solution. Mean ± standard deviation (n = 6) shown in the bar diagram followed by different letters are significantly different according to Duncan's multiple range test (p < 0.05). | |
3.4 Comparative analysis of the contact angle/adhesion work of different Fe3O4 particles on peanut and maize leaves
The smallest contact angles, θ, of the Fe3O4-NPs, within the 50–100 nm size range when sprayed onto peanut leaves were 77° and 81°, respectively. Furthermore, they exhibited the greatest adhesion work at values of 59 mN m−1 and 53 mN m−1, respectively (Fig. 7). These results indicate that the nano-Fe fertilizer (range 20–50 nm) provides good wetting and enhances adhesion to the leaf surface when sprayed onto peanut leaves. However, spraying Fe3O4-100 nm on maize leaves resulted in the smallest contact angle (80°) and the highest adhesion work (54 mN m−1), as shown in Fig. 8. Thus, this size is best for maize. These results are consistent with the fertilizer efficiency, adhesion, and anti-leaching effects.
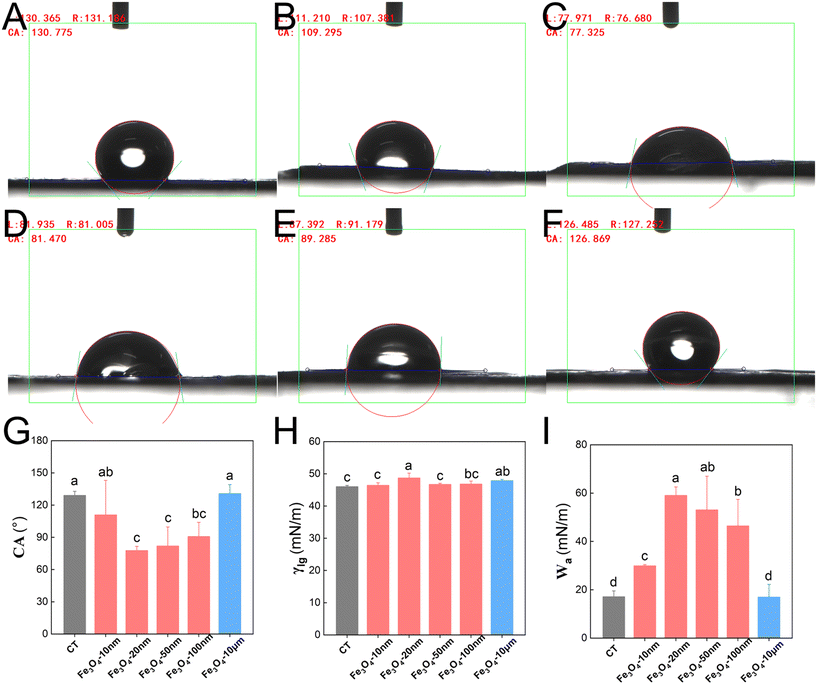 |
| Fig. 7 Contact angle images of the 0.5 μL nano-Fe fertilizer on peanut leaves: 0.1% Tween 80 (A); Fe3O4-NPs-10 nm (B); Fe3O4-NPs-20 nm (C); Fe3O4-NPs-50 nm (D); Fe3O4-NPs-100 nm (E); Fe3O4-NPs-10 μm (F). Contact angle (G), surface tension (H) and adhesion work (I) of different foliar fertilizers on peanut leaves. Mean ± standard deviation (n = 5) shown in the bar diagram followed by different letters are significantly different according to Duncan's multiple range test (p < 0.05). CT, 0.1% Tween 80 solution. | |
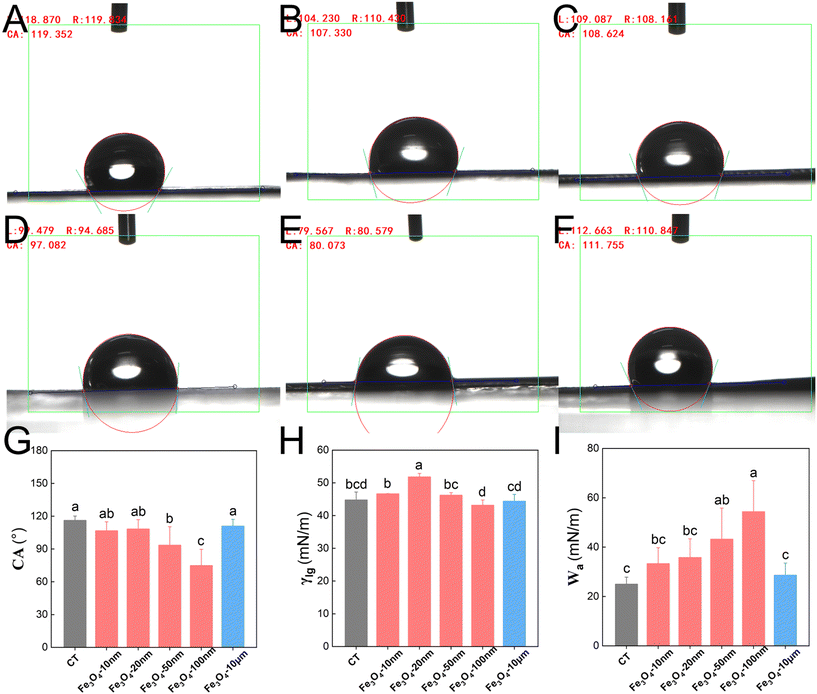 |
| Fig. 8 Contact angle images of the 0.5 μL nano-Fe fertilizer on maize leaves: 0.1% Tween 80 (A); Fe3O4-NPs-10 nm (B); Fe3O4-NPs-20 nm (C); Fe3O4-NPs-50 nm (D); Fe3O4-NPs-100 nm (E); Fe3O4-NPs-10 μm (F). Contact angle (G), surface tension (H) and adhesion work (I) of different foliar fertilizers on peanut leaves. Mean ± standard deviation (n = 5) shown in the bar diagram followed by different letters are significantly different according to Duncan's multiple range test (p < 0.05). CT, 0.1% Tween 80 solution. | |
3.5 Force/work analysis of Fe3O4 particles of varying size on peanut and maize leaves
The interaction between the size of Fe3O4 particles and leaf nanosheets had a direct influence on the anti-leaching effect, which affects fertilizer efficiency and size optimization. Fe3O4-10 μm remained on top of the nanosheets as its size exceeded the void size (942 nm), thereby impeding descent into void positions (Fig. 1A/B). The van der Waals force (Fvdw) was too small compared to gravity (G) (Tables 1 and 2). Consequently, the primary factors affecting the system were G and the impulsive force (Fi), with Fvdw being disregarded during calculations. The force analysis must satisfy inequality eq-S12 (μ ≤ 1) in the ESI† for the Fe3O4 particles to slide off the leaf surface. The coefficient of friction (μ) for rubber-cast Fe is 0.8 according to ASTM G115, which complies with the slip condition. Thus, our theoretical calculation supports the result that Fe3O4 particles (10 μm) readily slid off the leaf surface under simulated rainfall conditions, displaying minimal adhesion and poor resistance to leaching (Fig. 5 and 6).
Table 1 Force/work analysis of the Fe3O4 particles of different sizes on peanut leaves
Force/work types |
10 nm |
20 nm |
50 nm |
100 nm |
10 μm |
NA: Data not available; Fvdw: van der Waals force; G: gravity; Fb: buoyancy force; W(n=1): the work of the first Fe3O4 NP along peanut leaf nanosheets; W(n=2): the work of the second Fe3O4 NP along peanut leaf nanosheets; the relevant calculations, and the force and work analyses are provided in the ESI.† |
F
vdw/N |
2.08 × 10−10 |
4.17 × 10−10 |
1.04 × 10−9 |
2.08 × 10−9 |
2.81 × 10−13 |
G/N |
4.85 × 10−20 |
3.88 × 10−19 |
6.06 × 10−18 |
4.85 × 10−17 |
1.76 × 10−11 |
F
b/N |
0.98 × 10−20 |
0.78 × 10−19 |
1.22 × 10−18 |
0.98 × 10−17 |
0.98 × 10−11 |
W
(n=1)/J |
2.78 × 10−17 |
5.40 × 10−17 |
1.23 × 10−16 |
2.03 × 10−16 |
NA |
W
(n=2)/J |
2.62 × 10−17 |
4.73 × 10−17 |
8.08 × 10−17 |
3.67 × 10−17 |
NA |
Table 2 Force/work analysis of Fe3O4 particles with different sizes on maize leaves
Force/work types |
10 nm |
20 nm |
50 nm |
100 nm |
10 μm |
NA: Data not available; Fvdw: van der Waals forces; G: gravity; Fb: buoyancy force; W(n=1): the work of the first Fe3O4 NP along peanut leaf nanosheets; the relevant calculations, as well as the force and work analyses, are provided in the ESI.† |
F
vdw/N |
2.08 × 10−10 |
4.17 × 10−10 |
1.04 × 10−9 |
2.08 × 10−9 |
6.29 × 10−13 |
G/N |
4.85 × 10−20 |
3.88 × 10−19 |
6.06 × 10−18 |
4.85 × 10−17 |
1.76 × 10−11 |
F
b/N |
0.98 × 10−20 |
0.78 × 10−19 |
1.22 × 10−18 |
0.98 × 10−17 |
0.98 × 10−11 |
W
(n=1)/J |
1.83 × 10−17 |
3.50 × 10−17 |
7.50 × 10−17 |
1.08 × 10−16 |
NA |
NPs in the 10–100 nm size range, applied to peanut, were subject to a high blocking effect (Fig. 1C). The dominant force was Fvdw, as supported by the force analysis calculations (Table 1), indicating that it is significantly larger compared to G. Therefore, it can be inferred that there is a direct correlation between its magnitude and the reduced likelihood of slip for Fe3O4 NPs in water droplets. The NPs primarily showed anti-friction work as they slid upward along the nanosheets of the leaf surface at a constant speed (Fig. 1E). As the height of the nanosheets allowed for the overlap of two 100 nm NPs, we focused on W(n=2). In particular, when the second NP (100 nm) slid, it caused other trapped NPs in the interstitial space of the nanosheets to slide down with it. The work values for the second NPs within the 10–100 nm range were calculated to be 2.62 × 10−17 J, 4.73 × 10−17 J, 8.08 × 10−17 J, and 3.67 × 10−17 J, respectively, according to the work formula eq-S16 in the ESI† (Table 1). Among them, Fe3O4-50 nm does the most work and is the least likely to slip. Therefore, our results agree with the aforementioned level of effective adhesion (Table 1, Fig. 5 and 6).
The presence of Fe3O4-NPs on maize leaves was attributed to the larger void size (942 nm) and lower height (115 nm) of the nanosheets. Thus, the NPs exhibited diagonal downward sliding along the empty spaces between the nanosheets (Fig. 1D). The force analysis must satisfy inequality eq-S18 to slip from the surface of the maize leaf, as shown in the ESI.† According to the data presented in Table 2, Fvdw was significantly larger than G. Consequently, we further simplified eq-S16, resulting in eq-S17 (Fvdwμ ≤ Fi sin
45° (1 – μ)). Because Fi sin
45° (1 – μ) is a specific range value in eq-S17, it can be inferred that Fvdw is the dominant force. As the height of the nanosheets on the surface of the maize leaf (115 nm) only allows for one 100 nm NP to overlap, we focused on W(n=1) (Fig. 1). The larger the size within the range of 10–100 nm, the greater the Fvdw, Ff, and W(n=1), which makes it less likely for particles to slip off the leaf surface. These results are consistent with the level of effective adhesion (Table 2, Fig. 5 and 6).
4. Discussion
4.1 Size effect of NMs critically influences plant growth, nutrient enrichment, and photosynthetic efficiency
The size effect of NMs plays a pivotal role in determining fertilizer efficiency in practical agricultural applications, owing to the size exclusion limits imposed by barriers to NP uptake. Uptake in foliar applications is affected by two intake pathways: the cuticle (effective size range: 0.6–4.8 nm) and the stomata (effective size range: ∼10 μm).37 In this study, the stomatal opening of the peanut and corn leaves was 2 μm (i.e., the width size of stomata on the leaf epidermis, see Fig. S1/S2 and Table S1†), which fully satisfies the entry of nanoscale Fe3O4-NPs (10–100 nm) but makes it difficult for microscale Fe3O4 particles (10 μm) to enter (Table S1†). The foliar application of the 10–20 nm NPs resulted in better fertilizer efficiency on both crops under non-rainfall conditions (Fig. S8, S10, and S12†). Furthermore, the micron-sized NPs had a noticeably inferior fertilization effect compared to the nano-sized ones. Similar results have been reported in other studies. For instance, a study that compared nano- and micron-scale copper particles found that only the smaller nano-copper exhibited increased copper accumulation on barley grains.38 Foliar spraying of γ-Fe2O3 NMs with varying sizes (4–15, 8–30, and 40–215 nm) on soybean seedlings revealed that the smallest size of γ-Fe2O3 NMs (4–15 nm) had the greatest impact on plant growth and nitrogen fixation capacity.30 The growth of rice seedlings has also been found to be differentially influenced by platinum39 and zinc oxide31 NPs of different sizes, but not under simulated rainfall conditions. It should be noted that the optimal sizes for peanut and maize under rainfall conditions were 50 nm and 100 nm, respectively (Fig. 3 and 4). This was attributed to the reduced interactions between the smaller NPs and the nanosheets on the leaves, which makes them more prone to sliding off. These findings highlight the interaction between the NPs and the microstructure of the leaf surface, particularly during rainfall conditions.
4.2 The nanosheets on the surface of the leaves affect the size optimization of NMs
A significant knowledge gap exists regarding the interactions between NPs and the nanosheets of leaves, with only a few studies focusing on the impact of leaf microstructure. For example, Li et al.40 reported that the accumulation of nano-ZnO in the non-glandular trichoid area of sunflower leaves is 2.9 times higher than that of other epidermal tissues, potentially due to stronger relative interactions between non-glandular trichomes and NPs resulting in enhanced adsorption and uptake. Additionally, they observed a decrease in the trichoid density on sunflower leaves and changes in the composition and structure of the adaxial leaf surface due to zinc deficiency, possibly serving as the primary factor contributing to lower zinc absorption.41 The microscopic trichomes on the leaves of sunflower are remarkably large, with a size of 30–100 μm, but it is sufficient to meet the absorption effects of nano-zinc. Furthermore, the peanut/corn nanosheets and nano-iron are both at the same nanoscale and will be more affected by the interaction. The most intuitive phenotype is that these different structures display diverse lotus leaf effects and hydrophobicity, which affect the degree of wetting. In this study, the contact angle of 0.1% Tween 80 on peanut leaves (130°) was significantly larger than that of maize (119°), due to their different nanosheet structures (Fig. 7 and 8). The interactions between the NPs and nanosheets directly affected their adhesion capability and resistance to leaching. The unique interplay between surface microstructure and NMs was comprehensively analyzed through force and work measurements (Tables 1 and 2), and the quantitative results were consistent with the adhesion and anti-leaching results (Fig. 5 and 6). These results demonstrate that different nanosheet structures have different effects on the retention of NPs on the leaf surface. Moreover, when exploring the optimization of nanomaterial shapes, it is imperative to consider the distinct leaf nanosheets of different crops. Therefore, optimized NPs that are tailored to match the specific leaf microstructure of a given plant will attach more firmly to the surfaces of leaves, show stronger leaching resistance and utilization rate, and thus lead to superior fertilizer efficacy especially under extreme weather conditions.
4.3 The optimized NM design effectively adapts to extreme weather conditions
We found that the optimized sizes of Fe3O4-NPs were 50 and 100 nm for peanut and maize leaves, respectively. These NPs displayed remarkable anti-leaching ability, increased biomass, high leaf Fe concentrations, and enhanced photosynthetic efficiency under simulated rainfall conditions (Fig. 3–8). Zhang et al.42 reported that the transport efficiency of 6 nm star-shaped polymers to young leaves was three times higher than that of 35 nm star-shaped polymers, indicating preferential facilitation of symbiotic unloading in young leaves by smaller star-shaped polymers. NMs can be optimized not only in terms of size but also by shape, charge, and hydrophobicity. Deng et al.43 showed that regulating the charge and shape of nanoscale CuO into a negatively charged nano-peak shape significantly enhances the inhibitory effect against Fusarium and nutrient bio-enhancement in tomatoes and watermelons. Hu et al.44 investigated the effect of the ζ potential (−52.3 mV to +36.6 mV) and hydrodynamic size (1.7–18 nm) of hydrophilic NPs on transport efficiency and pathways to specific leaf cells and organelles, and revealed that hydrophilic NPs with a diameter of 11 nm and a positive charge of +15 mV exhibit superior foliar delivery efficiencies in guard cells (100%), the extracellular space (90.3%), and chloroplasts (55.8%) of cotton and maize plants. The oxidation-resistant ferrous foliar fertilizer (ORFFF) was optimized by Li et al.,45 using micro/nano-structured hollow silicon as the carrier, combined with ferrous and vitamin C. The optimized ORFFF nano-fertilizer had intelligent control and foliar delivery functions, including anti-oxidation, high foliar adhesion efficiency, nutrient slow-release ability, pH-controlled release ability, and enhanced biosecurity. The optimal design for NMs is developed towards intelligent release, multi-functionality, and precision delivery, which offers more advantages when considering extreme weather in future nano-agricultural applications.
Conclusion
We found that the optimal size of Fe3O4-NPs for peanut leaves was 50 nm, which led to the highest anti-leaching ability. This was attributed to their higher height, more widespread distribution, and stronger interactions between the nanosheets and the Fe3O4-NPs. These Fe3O4-NPs showed a superior fertilizing effect under simulated rainfall conditions, including enhanced adhesion capacity, biomass, Fe concentration, and net photosynthetic rate. For maize, the optimal size was 100 nm, attributed to their lower height and much lower frequency distribution of the nanosheets, resulting in fewer interactions with Fe3O4-NPs. Overall, our results show that the optimal NP size for achieving the best anti-leaching effect was influenced by the leaf microstructure. These results will guide the design of more intelligent and multi-functional nano-fertilizers and nano-carrier fertilizers.
Data availability
All data generated or analyzed in this study are included in this published article and its ESI.†
Author contributions
Dunyi Liu: conceptualization, funding acquisition, writing – review & editing. Lingyun Chen: data curation, project administration, writing & editing. Wanru Qing, Xiaoxiao Li, Wenhui Chen and Can Hao: formal analysis, investigation. Xinping Chen: supervision, visualization, writing – review & editing.
Conflicts of interest
The authors declare no competing interests.
Acknowledgements
This work was supported by the National Key Research and Development Program of China (2023YFD1902200) and Fundamental Research Funds for the Central Universities (SWU-KQ22060). The English in this manuscript has also been checked by two professional editors (M.Sc. or Ph.D./M.D.) and an English language specialist. Please see https://www.textcheck.com/certificate/index/uP1Y7X for the Textcheck Certificate.
References
- J.-F. Briat, C. Dubos and F. Gaymard, Iron nutrition, biomass production, and plant product quality, Trends Plant Sci., 2015, 20, 33–40 CrossRef CAS.
- S. Khan, K. Kaur, V. Kumar and S. Tiwari, Iron transport and homeostasis in plants: current updates and applications for improving human nutrition values and sustainable agriculture, Plant Growth Regul., 2023, 100, 373–390 CrossRef CAS.
- J. Morrissey and M. L. Guerinot, Iron uptake and transport in plants: the good, the bad, and the ionome, Chem. Rev., 2009, 109, 4553–4567 CrossRef CAS PubMed.
- C. Curie, Z. Panaviene, C. Loulergue, S. L. Dellaporta, J. F. Briat and E. L. Walker, Maize yellow stripe1 encodes a membrane protein directly involved in Fe(III) uptake, Nature, 2001, 409, 346–349 CrossRef CAS.
- N. J. Robinson, C. M. Procter, E. L. Connolly and M. L. Guerinot, A ferric-chelate reductase for iron uptake from soils, Nature, 1999, 397, 694–697 CrossRef CAS PubMed.
- S. Shirsat and K. Suthindhiran, Iron oxide nanoparticles as iron micronutrient fertilizer—Opportunities and limitations, J. Plant Nutr. Soil Sci., 2023, 1–24 Search PubMed.
- M. Kah, N. Tufenkji and J. C. White, Nano-enabled strategies to enhance crop nutrition and protection, Nat. Nanotechnol., 2019, 14, 532–540 CrossRef CAS.
- P. Li, Y. Du, L. Huang, N. Mitter and Z. P. Xu, Nanotechnology promotes the R&D of new-generation micronutrient foliar fertilizers, RSC Adv., 2016, 6, 69465–69478 RSC.
- M. Suzuki, A. Urabe, S. Sasaki, R. Tsugawa, S. Nishio, H. Mukaiyama, Y. Murata, H. Masuda, M. S. Aung, A. Mera, M. Takeuchi, K. Fukushima, M. Kanaki, K. Kobayashi, Y. Chiba, B. B. Shrestha, H. Nakanishi, T. Watanabe, A. Nakayama, H. Fujino, T. Kobayashi, K. Tanino, N. K. Nishizawa and K. Namba, Development of a mugineic acid family phytosiderophore analog as an iron fertilizer, Nat. Commun., 2021, 12, 1558 CrossRef CAS PubMed.
- N. Rani, A. Duhan, A. Pal, P. Kumari, R. K. Beniwal, D. Verma, A. Goyat and R. Singh, Are nano-pesticides really meant for cleaner production? An overview on recent developments, benefits, environmental hazards and future prospectives, J. Cleaner Prod., 2023, 411, 137232 CrossRef CAS.
- Y. Sun, H. Zhou, Y. Sun, Q. Wu, H. Chen and D. Chi, The application of nano−clinoptilolite based nitrogen fertilizer mixed with urea promotes nitrogen balance and enhances economic and ecological benefits in paddy fields, J. Cleaner Prod., 2024, 453, 142257 CrossRef CAS.
- Q. Wang, L. Gao, Y. Li, N. Shakoor, Y. Sun, Y. Jiang, G. Zhu, F. Wang, Y. Shen, Y. Rui and P. Zhang, Nano-agriculture and nitrogen cycling: Opportunities and challenges for sustainable farming, J. Cleaner Prod., 2023, 421, 138489 CrossRef.
- X. Yu, H. Pu and D.-W. Sun, Nano light conversion agents: recent advances, future prospects and challenges in enhancing plant green cultivation, J. Cleaner Prod., 2023, 426, 138919 CrossRef CAS.
- C. A. Garza-Alonso, G. Cadenas-Pliego, A. Juárez-Maldonado, J. A. González-Fuentes, G. Tortella and A. Benavides-Mendoza, Fe2O3 nanoparticles can replace Fe-EDTA fertilizer and boost the productivity and quality of Raphanus sativus in a soilless system, Sci. Hortic., 2023, 321, 112261 CrossRef CAS.
- L. Zhao, L. Lu, A. Wang, H. Zhang, M. Huang, H. Wu, B. Xing, Z. Wang and R. Ji, Nano-Biotechnology in Agriculture: Use of Nanomaterials to Promote Plant Growth and Stress Tolerance, J. Agric. Food Chem., 2020, 68, 1935–1947 CrossRef CAS.
- K. Wang, Y. Wang, K. Li, Y. Wan, Q. Wang, Z. Zhuang, Y. Guo and H. Li, Uptake, translocation and biotransformation of selenium nanoparticles in rice seedlings (Oryza sativa L.), J. Nanobiotechnol., 2020, 18, 103 CrossRef CAS PubMed.
- Z. Lv, M. Zhong, Q. Zhou, Z. Li, H. Sun, J. Bai, J. Liu and H. Mao, Nutrient strengthening of winter wheat by foliar ZnO and Fe3O4 NPs: Food safety, quality, elemental distribution and effects on soil bacteria, Sci. Total Environ., 2023, 893, 164866 CrossRef CAS PubMed.
- J. Wang, X. Cao, C. Wang, F. Chen, Y. Feng, L. Yue, Z. Wang and B. Xing, Fe-based nanomaterial-induced root nodulation is modulated by flavonoids to improve soybean (<i>glycine max</i>) growth and quality, ACS Nano, 2022, 16, 21047–21062 CrossRef CAS PubMed.
- A. Emamverdian, A. Ghorbani, Y. Li, N. Pehlivan, J. Barker, Y. Ding, G. Liu and M. Zargar, Responsible mechanisms for the restriction of heavy metal toxicity in plants via the co-foliar spraying of nanoparticles, Agronomy, 2023, 13, 1748 CrossRef CAS.
- J. Lian, L. Cheng, X. Zhai, R. Wu, W. Liu, J. Pan, M. J. I. Shohag, X. Xin, Z. He and X. Yang, Foliar spray of combined metal-oxide nanoparticles alters the accumulation, translocation and health risk of Cd in wheat (Triticum aestivum L.), J. Hazard. Mater., 2022, 440, 129857 CrossRef CAS.
- M. S. Pontes, J. S. Santos, J. L. Da Silva, T. B. A. R. Miguel, E. C. Miguel, A. G. Souza Filho, F. Garcia, S. M. Lima, L. H. Da Cunha Andrade, G. J. Arruda, R. Grillo, A. R. L. Caires and E. Felipe Santiago, Assessing the fate of superparamagnetic iron oxide nanoparticles carrying usnic acid as chemical cargo on the soil microbial community, ACS Nano, 2023, 17, 7417–7430 CrossRef CAS.
- N. Asghar, A. Hussain, D. A. Nguyen, S. Ali, I. Hussain, A. Junejo and A. Ali, Advancement in nanomaterials for environmental pollutants remediation: a systematic review on bibliometrics analysis, material types, synthesis pathways, and related mechanisms, J. Nanobiotechnol., 2024, 22, 26 CrossRef.
- S. Jia, Y. Wu, H. Zhou, H. Yan, Y. Liao and H. Mao, Plant tannin foam anchored iron nanoparticles: Efficient and recyclable degradation of tetracycline antibiotics under high salt conditions, J. Cleaner Prod., 2023, 426, 139188 CrossRef CAS.
- C. Zou, T. Lu, R. Wang, P. Xu, Y. Jing, R. Wang, J. Xu and J. Wan, Comparative physiological and metabolomic analyses reveal that Fe3O4 and ZnO nanoparticles alleviate Cd toxicity in tobacco, J. Nanobiotechnol., 2022, 20, 302 CrossRef CAS PubMed.
- H. Wu and Z. Li, Nano-enabled agriculture: How do nanoparticles cross barriers in plants?, Plant Commun., 2022, 3, 100346 CrossRef CAS PubMed.
- M. Xu, Y. Qi, G. Liu, Y. Song, X. Jiang and B. Du, Size-dependent <i>in vivo</i> transport of nanoparticles: implications for delivery, targeting, and clearance, ACS Nano, 2023, 17, 20825–20849 CrossRef PubMed.
- M. Gao, J. Chang, Z. Wang, H. Zhang and T. Wang, Advances in transport and toxicity of nanoparticles in plants, J. Nanobiotechnol., 2023, 21, 75 CrossRef CAS PubMed.
- B. Ruttkay-Nedecky, O. Krystofova, L. Nejdl and V. Adam, Nanoparticles based on essential metals and their phytotoxicity, J. Nanobiotechnol., 2017, 15, 33 CrossRef.
- J. Zhu, J. Wang, X. Zhan, A. Li, J. C. White, J. L. Gardea-Torresdey and B. Xing, Role of charge and size in the translocation and distribution of zinc oxide particles in wheat cells, ACS Sustainable Chem. Eng., 2021, 9, 11556–11564 CrossRef CAS.
- X. Cao, L. Yue, C. Wang, X. Luo, C. Zhang, X. Zhao, F. Wu, J. C. White, Z. Wang and B. Xing, Foliar application with iron oxide nanomaterials stimulate nitrogen fixation, yield, and nutritional quality of soybean, ACS Nano, 2022, 16(1), 1170–1181 CrossRef CAS PubMed.
- Z. Li, W. Yan, Y. Li, Y. Xiao, Y. Shi, X. Zhang, J. Lei, K. Min, Y. Pan, X. Chen, Q. Liu and G. Jiang, Particle size determines the phytotoxicity of ZnO nanoparticles in rice ( <i>oryza sativa</i> l.) revealed by spatial imaging techniques, Environ. Sci. Technol., 2023, 57, 13356–13365 CrossRef CAS PubMed.
- Y.-Y. Chen, S.-F. Ou and H.-W. Chien, Rose petal patterns with “Lotus Effect”, corrosion resistance and photocatalytic bacterial inactivation, J. Ind. Eng. Chem., 2024, 137, 536–546 CrossRef CAS.
- X. He, K. Zhang, X. Xiong, Y. Li, X. Wan, Z. Chen, Y. Wang, X. Xu, M. Liu, Y. Jiang and S. Wang, Prediction of the lotus effect on solid surfaces by machine learning, Small, 2022, 18, 2203264 CrossRef CAS.
- S. Sun, H. Li, Y. Guo, H.-Y. Mi, P. He, G. Zheng, C. Liu and C. Shen, Superefficient and robust polymer coating for bionic manufacturing of superwetting surfaces with “rose petal effect” and “lotus leaf effect”, Prog. Org. Coat., 2021, 151, 106090 CrossRef CAS.
- L. Chen, Z. Hu, W. Chen, Z. Xu, C. Hao, P. Lakshmanan, D. Liu and X. Chen, Comparative study of the effectiveness of nano-sized iron-containing particles as a foliar top-dressing of peanut in rainy conditions, Agric. Water Manag., 2023, 286, 108392 CrossRef.
- W. Li, R. Fan, H. Zhou, Y. Zhu, X. Zheng, M. Tang, X. Wu, C. Yu and G. Wang, Improving the utilization rate of foliar nitrogen fertilizers by surface roughness engineering of silica spheres, Environ. Sci.: Nano, 2020, 7, 3526–3535 RSC.
- J. Lv, P. Christie and S. Zhang, Uptake, translocation, and transformation of metal-based nanoparticles in plants: recent advances and methodological challenges, Environ. Sci.: Nano, 2019, 6, 41–59 RSC.
- I. Jośko, M. Kusiak, K. Różyło, E. Baranowska-Wójcik, M. Sierocka, M. Sheteiwy, D. Szwajgier and M. Świeca, The life cycle study revealed distinct impact of foliar-applied nano-Cu on antioxidant traits of barley grain comparing with conventional agents, Food Res. Int., 2023, 164, 112303 CrossRef.
- Y. Zhou, X. Liu, X. Yang, G. Du Laing, Y. Yang, F. M. G. Tack, M. S. Bank and J. Bundschuh, Effects of platinum nanoparticles on rice seedlings ( <i>oryza sativa</i> l.): size-dependent accumulation, transformation, and ionomic influence, Environ. Sci. Technol., 2023, 57, 3733–3745 CrossRef CAS PubMed.
- C. Li, P. Wang, E. Lombi, J. Wu, F. P. C. Blamey, V. Fernandez, D. L. Howard, N. W. Menzies and P. M. Kopittke, Absorption of foliar applied Zn is decreased in Zn deficient sunflower (Helianthus annuus) due to changes in leaf properties, Plant Soil, 2018, 433, 309–322 CrossRef CAS.
- C. Li, P. Wang, A. van der Ent, M. Cheng, H. Jiang, T. Lund Read, E. Lombi, C. Tang, M. D. de Jonge, N. W. Menzies and P. M. Kopittke, Absorption of foliar-applied Zn in sunflower (Helianthus annuus): importance of the cuticle, stomata and trichomes, Ann. Bot., 2019, 123, 57–68 CrossRef CAS.
- Y. Zhang, L. Fu, S. Li, J. Yan, M. Sun, J. P. Giraldo, K. Matyjaszewski, R. D. Tilton and G. V. Lowry, Star polymer size, charge content, and hydrophobicity affect their leaf uptake and translocation in plants, Environ. Sci. Technol., 2021, 55, 10758–10768 CrossRef CAS.
- C. Deng, C. R. Protter, Y. Wang, J. Borgatta, J. Zhou, P. Wang, V. Goyal, H. J. Brown, K. Rodriguez-Otero, C. O. Dimkpa, R. Hernandez, R. J. Hamers, J. C. White and W. H. Elmer, Nanoscale CuO charge and morphology control Fusarium suppression and nutrient biofortification in field-grown tomato and watermelon, Sci. Total Environ., 2023, 905, 167799 CrossRef CAS.
- P. Hu, J. An, M. M. Faulkner, H. Wu, Z. Li, X. Tian and J. P. Giraldo, Nanoparticle charge and size control foliar delivery efficiency to plant cells and organelles, ACS Nano, 2020, 14, 7970–7986 CrossRef CAS PubMed.
- W. Li, H. Zhou, X. Zhang, Z. Li, Z. Zou, Y. Shen and G. Wang, Oxidation-resistant silicon nanosystem for intelligent controlled ferrous foliar delivery to crops, ACS Nano, 2023, 17, 15199–15215 CrossRef CAS.
|
This journal is © The Royal Society of Chemistry 2025 |
Click here to see how this site uses Cookies. View our privacy policy here.