DOI:
10.1039/D4EN01226G
(Perspective)
Environ. Sci.: Nano, 2025,
12, 2507-2515
Towards the next decade for research on the environmental impact of nanoscale zerovalent iron on microorganisms
Received
31st December 2024
, Accepted 7th April 2025
First published on 9th April 2025
Abstract
Nanoscale zerovalent iron (NZVI) is among the most widely used nanomaterials in environmental applications. With such an increased use of NZVI, there is a need to understand its potential impact on microorganisms, vital components of various ecosystems. Significant progress has been made in elucidating the molecular mechanisms underlying NZVI toxicity in model bacteria, while recent research has shifted toward evaluating its long-term impacts on environmental microbial systems. Herein, we first revisit a range of studies on NZVI–single microorganism interactions under aerobic and anaerobic conditions in an effort to make general conclusions regarding the NZVI toxicity mechanism at the cellular level. Additionally, a comprehensive overview is provided on the effects of NZVI on complex bacterial consortia in natural aquatic and soil environments, as well as in wastewater treatment systems. Finally, we discuss future research directions for sustainable and environmentally friendly application of NZVI.
Environmental significance
Nanoscale zerovalent iron (NZVI) is one of the engineered nanomaterials intentionally introduced into ecosystems for environmental remediation. Understanding its interactions with microorganisms and its potential toxicity is essential for sustainable applications of NZVI. Herein, we analyze the current state of knowledge on the NZVI's impacts at cellular and community levels, showing that while NZVI adversely affects single bacteria, bacterial communities exhibit compositional and functional resilience, recovering from initial negative effects following its application. This perspective also emphasizes future research directions focused on environmentally relevant microorganisms in real-world settings to build NZVI application strategies that balance remediation effectiveness with ecological safety.
|
1. Introduction
Nanoscale zerovalent iron (NZVI), which combines the eco-friendly and high reductive properties of ZVI (Fe(0)) with enhanced particle reactivity due to its large specific surface area at the nanoscale, is one of the most widely utilized materials for wastewater treatment and soil and groundwater remediation over the past few decades.1 In 1995, Glavee et al. was the first to synthesize NZVI powder by reducing Fe(II) ions with borohydride.2 Soon after, in 1997, Wang and Zhang applied NZVI to transform trichloroethylene and polychlorinated biphenyls, demonstrating its promising potential for environmental applications.3 Since then, numerous research papers and patents have been filed for practical use of on-site contaminant removal, not just laboratory-scale fundamental research.4 However, several shortcomings are identified during its application. NZVI tends to aggregate and rapidly undergoes surface passivation in the presence of water or oxygen, which limits its reactivity and mobility.5 To address these issues, NZVIs with diverse compositions have been developed. Representative surface modification materials include metals,6–8 sulfides,9–12 and polymers13–17 (Fig. 1a), with dozens of different NZVI compositions currently developed for diverse applications. Surface-modified NZVIs have been reported to degrade recalcitrant pollutants through radical generation, enhance electrical conductivity, and improve mobility within aquifers.1,5,10,14
 |
| Fig. 1 (a) Key breakthroughs in the synthesis of NZVIs. (b) Publications on the toxicity of NZVIs to pure bacterial cultures and bacterial communities based on Web of Science data. | |
From an ecotoxicological perspective, NZVI is a highly intriguing material, as it is one of the engineered nanomaterials intentionally introduced into the environment through well-defined and predictable exposure pathways.18 This has driven extensive research into its toxicity, safety, and persistence in ecosystems.19 Any advancements in NZVI synthesis or the expansion of their compositional and structural diversity exert various toxicological impacts on living organisms.20 For studies regarding NZVIs and their interaction with microorganisms, bacteria are among the most important organisms due to their small size and importance as key players in ecosystem processes. Surface modification of NZVI with stabilizing or reactive agents can directly influence the nano-bio interface and microbial toxicity by altering the surface charge and dissolution of NZVIs.21–24 Nevertheless, a notable time gap existed between the synthesis of NZVIs and their ecotoxicological evaluation in microorganisms (Fig. 1b). For example, while the synthesis of bimetallic NZVI was reported in 1998,6 its toxicity assessment was not conducted until the early 2010s.22 In 2014, we compared the bacterial toxicity of bimetallic NZVIs and bare NZVI, and demonstrated that bare NZVI was more toxic than Pd/NZVI, which is the most reactive to contaminants.25 However, in recent years, it has become evident that the knowledge gap between the two is steadily shrinking due to the growing interest in nanoparticle toxicity.
Herein, we present a perspective from the key findings regarding the toxicological effects of NZVI on microorganisms over the past decade. In addition to discussing the mechanism underlying NZVI toxicity at cellular and community levels in various environmental settings, our perspective suggests future directions of research in this field.
2. Ecotoxicological impacts of NZVIs
Previous studies assessing NZVI-dependent bacterial toxicity have primarily utilized just a few, relatively well-characterized, model organisms. However, more recently, there has been a push to examine the long-term impacts of NZVI exposure on the bacterial community structure and function across ecosystems. This section will carefully elaborate the underlying mechanisms associated with the toxicological impacts of NZVIs on both single bacteria and bacterial communities.
2.1. Toxicity of NZVI on pure cultures
Over the past decade, numerous investigations have evaluated the toxicity of NZVI on single microorganisms using in vitro toxicity assays. Most of them confirmed significant adverse effects on cell viability, growth, membrane integrity, and biological activity, and the extent of toxicity differed with the oxygen levels and the specific microorganisms tested.26
Upon application, NZVIs are exposed to varying oxygen levels in the environment; for instance, oxygen concentrations are higher in water than in soil. Oxygen levels determine the oxidation state of iron, and thereby the means by which cells acquire it.27 Under aerobic conditions, NZVI reacts with molecular oxygen to generate various extracellular reactive oxygen species (ROS) and form a surface oxide layer (eqn (1)–(4)).28
| Fe0 + O2 → Fe2+ + H2O2 | (1) |
| Fe2+ + O2 → Fe3+ + HO2˙/O2−˙ | (2) |
| Fe2+ + HO2˙/O2−˙ → Fe3+ + H2O2 | (3) |
| Fe2+ + H2O2 → Fe3+ + OH− + ˙OH | (4) |
These ROS are likely to engage in uncontrolled oxidation reactions with cell wall components and, after diffusion, with intracellular biological molecules (
e.g., proteins, lipids, DNA). This leads to an increase in oxidative damage, adversely affecting bacterial survival.
29,30 The extent of cellular damage in response to ROS is influenced by the type of microorganism with different cell wall structures.
31–33 A number of studies comparing the toxicity of bare and modified NZVIs on Gram-negative (
Escherichia coli, Pseudomonas fluorescens, Pseudomonas aeruginosa) and Gram-positive bacteria (
Staphylococcus aureus,
Bacillus subtilis) have shown that Gram-positive strains are more tolerant to NZVIs than Gram-negative strains.
34–38 Gram-positive bacteria have a thick, tightly-structured peptidoglycan layer whilst Gram-negative bacteria have a thin peptidoglycan layer covered by an outer lipid membrane consisting of lipopolysaccharide (LPS). ROS can react with both lipids and peptidoglycan, thus causing structural changes in the cell envelope. However, the outer membrane of Gram-negative bacteria, which have a higher lipid content, is more susceptible to oxidation and envelope damage than the peptidoglycan layer of Gram-positive bacteria.
31,32 The thick peptidoglycan layer of Gram-positive bacteria also limits ROS diffusion into the cell, leading to lower intracellular oxidative stress compared to Gram-negative bacteria.
39 Moreover, the negatively charged LPS in the outer membrane of Gram-negative bacteria may exhibit a higher affinity for Fe
2+ ions released from NZVI, potentially enhancing Fe
2+ uptake and promoting subsequent ROS generation, which provides another possible explanation for the greater susceptibility of Gram-negative bacteria to NZVI-induced toxicity.
Another often-suggested mechanism contributing to NZVI toxicity is membrane disruption following the adhesion of NZVI to the cell membrane.40,41 In 2014, we demonstrated that the direct contact of bimetallic NZVI with E. coli had a more pronounced impact on the NZVI cytotoxicity compared to the generation of endogenous ROS.25 Similarly, surface modification of NZVI with polymers or natural organic matter (NOM) reduces toxicity by limiting the physical contact between the particles and cell membranes.23,42 The importance of particle adhesion to bacteria in NZVI toxicity has been highlighted in previous studies.34,41 Consequently, the interaction between NZVI and bacteria varies greatly depending on the envelope composition of Gram-negative and Gram-positive bacteria. The thick peptidoglycan layer in the cell walls of Gram-positive bacteria can reduce NZVI adhesion,38 while lipoteichoic acid, another cell wall component, can form chemical bonds with NZVI, further inhibiting its penetration into the cell.43 Thus, the observed difference in inactivation efficacy between Gram-negative and Gram-positive bacteria in the NZVI system may be attributed to different reactions of ROS and the extent of NZVI adsorption to the cell wall.
Under aerobic conditions, however, ROS-induced oxidative stress and membrane disruption appear to be interrelated processes that contribute to NZVI toxicity, rather than affecting each separately.26 Given that ROS-induced oxidative stress occurs within cells, nanoparticles should be internalized through the cell membrane, resulting in membrane damage during the process.
Despite NZVI being the most widely employed reductant for in situ remediation of groundwater where anaerobic conditions prevail, plenty of studies focus on its toxicity in aerobic environments. Notably, NZVI has demonstrated greater toxicity toward Gram-negative bacteria, such as E. coli and P. putida, under anaerobic conditions compared to aerobic conditions.30,44–46 Given the lack of extracellular ROS production in the absence of oxygen, anaerobic bacterial inactivation should be attributed to other mechanisms. First, the bactericidal mechanism of NZVI is associated with physical disruption caused by its attachment to the bacterial cell membrane.30,43,47 The absence of oxygen prevents the formation of a substantial oxide layer on the NZVI surface. This lack of passivation enables more direct interactions between NZVIs and bacterial cell membranes, resulting in greater membrane disruption and increased toxicity. Under anaerobic conditions, iron primarily exists in its soluble, reduced Fe2+ form (eqn (5)), and can be taken up directly by bacteria via dedicated pathways (e.g., the FeoABC system).48,49
| Fe0 + 2H2O → Fe2+ + H2 + 2OH− | (5) |
Intracellular Fe
2+ causes cellular damage by displacing other divalent metals in metalloproteins, reducing various molecules to produce additional bactericidal compounds, and generating organic radicals, such as glutathione radicals.
21,50 Furthermore, intracellular Fe
2+ can trigger excessive ROS production within the cytoplasm through the iron-catalyzed Haber–Weiss reaction (
eqn (4)).
51 According to Wang
et al.'s study,
46 it was shown that more ROS were detected inside the
E. coli cells after being exposed to NZVI anaerobically than aerobically, and the intracellular ROS level was positively correlated with intracellular Fe
2+ concentration and bacterial inactivation efficiency. Taken together, the toxicity mechanism of NZVIs may not be unique compared to that of other nanomaterials and may be a combination of multiple mechanisms. Although the relative contribution of each mechanism may vary depending on the types of NZVI and microorganisms, membrane disruption and oxidative stress caused by both intracellular and extracellular ROS are considered the main bactericidal mechanisms under aerobic conditions, whereas membrane disruption, Fe
2+ release, and Fe
2+-induced intracellular oxidative stress likely dominate under anaerobic conditions (
Fig. 2).
 |
| Fig. 2 Proposed bacterial inactivation mechanisms of NZVI under aerobic and anaerobic conditions. | |
An increasing body of evidence suggests that bacteria possess a range of defense mechanisms to cope with NZVI-induced stress by regulating specific enzyme activity and gene expression related to antioxidant defense and damage repair.52 Key cellular antioxidant defense systems include enzymes such as superoxide dismutase and catalase. These enzymes are found to be upregulated to counterbalance the presence of intracellular and extracellular ROS and thereby prevent them from causing oxidative damage, in response to ZVI exposure.53–55 On the other hand, membrane rupture caused by NP exposure may induce alterations in the expression of membrane proteins and transport-associated proteins, contributing to the repair and maintenance of cell envelope integrity.56 For example, although envelope gene regulation was not examined in that study, partial repair of the E. coli cell membrane following NZVI-induced damage in the presence of a nutrient supply has been reported.57 Nevertheless, NZVI-induced bacterial toxicity involves a combination of ROS generation, biomolecule damage, and cell membrane disruption (Fig. 2), making it challenging for bacteria to mount an immediate and comprehensive defense against all simultaneous stressors.
2.2. Environmental impacts of NZVI on microbial communities
The interactions of NZVI with microbial communities—key drivers of ecosystem functioning and contaminant degradation—remain a critical area of investigation. To ensure sustainable remediation practices, it is crucial to evaluate the environmental impacts of NZVI. Several studies have shown that NZVI influences microbial communities in diverse and often matrix-dependent ways, affecting their diversity, structure, and functionality across various environments ranging from aquatic and terrestrial ecosystems to wastewater treatment plants (WWTPs) (Fig. 3).58–61
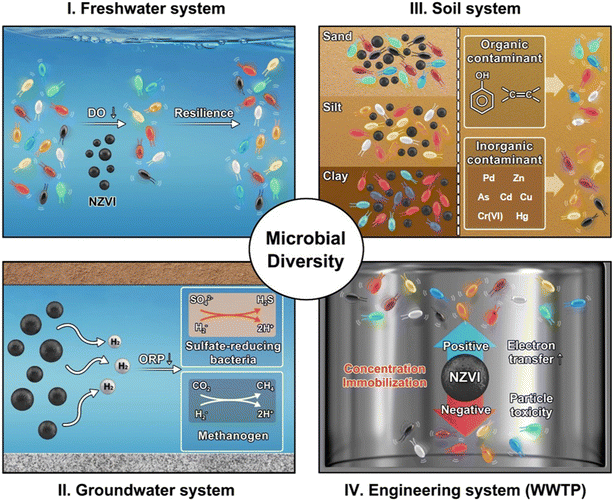 |
| Fig. 3 Schematic diagram of microbial community shifts and adaptations induced by NZVIs across various environmental matrices. | |
NZVI reacts with water and oxygen, altering oxidation–reduction potential (ORP) and dissolved oxygen (DO) levels in waters. For instance, the addition of 100 mg L−1 of NZVI into river water initially caused a decline in ORP and DO, which later stabilized as bacterial communities adapted. Despite an increase in bacterial cell abundance and activity, the bacterial community demonstrated resilience, with minimal changes observed in diversity indices and rank-abundance patterns.58 This indicates that microbial communities in freshwater environments can adapt to moderate NZVI exposure without significant functional loss. In contrast, significant shifts in bacterial diversity have been observed in groundwater following NZVI injection, without any adverse impact on total bacterial abundance. Specifically, populations of sulfate reducers and methanogens increased, driven by the reduction in ORP value and hydrogen production resulting from the anaerobic reaction of NZVI with water.62
In soil environments, the impacts of NZVI are strongly influenced by soil characteristics and NZVI type. Sandy-loam soils, which have lower organic matter content, are more vulnerable to NZVI-induced declines in bacterial abundance, richness, and enzymatic activity compared to clay-loam soils with higher organic content. This highlights the buffering capacity of organic matter and clay in mitigating NZVI's impacts.59 In contaminated soil, NZVI injection disrupts the bacterial community composition, leading to a reduction in pollutant biodegradation efficiency during relatively short-term monitoring.63 However, long-term observations following NZVI application reveal an increase in the abundance of bacteria capable of degrading pollutants within soil communities, eventually enhancing pollutant degradation.64,65 Unlike bare NZVI, the introduction of modified NZVIs, such as biochar-supported NZVI66,67 and sulfidized NZVI,66,68,69 into soil can enhance bacterial diversity in the native communities rather than reduce it.
Conflicting effects depending on the concentration of NZVI are commonly observed in wastewater treatment processes. In anaerobic digesters, a low dose of NZVI could enhance methane production by releasing hydrogen, a critical electron donor for hydrogenotrophic methanogenesis.61 In contrast, excessive NZVI concentrations inhibit methane production by creating high hydrogen partial pressures that favor alternative anaerobic pathways or by elevating Fe(II) concentrations to toxic levels to the microbial community.70 Similarly, low concentrations of NZVI in denitrifiers and sequencing biofilm reactors are shown to enhance process efficiency due to an enrichment of bacterial species involved in denitrification and pollutant biodegradation within the community.71–73 In addition to NZVI concentration, particle size is a key factor influencing microbial toxicity within bacterial communities. It is shown that ZVI particle size significantly affects anaerobic methane production: 48 μm ZVI at 2 g L−1 increased the methane yield by 84.12%, whereas 100 nm ZVI at 0.5 g L−1 led to only a 37.32% increase. ZVI particles with a range of 4–48 μm also enhance microbial abundance, enzyme activity, and direct interspecies electron transfer, while reducing nanoscale cell lysis.74
Unique microbial traits, e.g., gene transfer, dormancy, dispersal ability, or speciation rate, underpinning microbial community assembly are intrinsically linked to microbial resilience against disturbances,75 including NZVI exposure. Although the impact of NZVI on microbial communities varies depending on a range of factors, such as environmental matrices, NZVI type, its concentration, and the presence of contaminants, similar concentration-dependent responses have been observed in both natural and engineered ecosystems. While low doses of NZVI can enhance microbial diversity and activities beneficial for bioremediation, higher doses often disrupt the community structure and function. On the other hand, surface modifications of NZVI significantly influence its transformation behavior in soil environments, thereby affecting the structure and function of soil microbial communities. For instance, the aging of polymer-coated NZVI into iron oxides has been linked to shifts in microbial composition, particularly among iron-reducing and iron-oxidizing bacteria (e.g., Bacillus, Shewanella). The type of polymer coating material affects the aging kinetics, with polyacrylic acid (PAA) accelerating and carboxymethyl cellulose (CMC) retarding, ultimately leading to distinct microbial responses.76 Similarly, sulfidized NZVI undergoes surface transformations, resulting in the formation of both iron oxides and sulfur-containing minerals, including pyrite and iron polysulfides. These transformations are attributed to notable changes in the microbial community structure such as an increased abundance of magnetotactic bacteria and enrichment of sulfur-cycling microorganisms. Collectively, these observations underscore the reciprocal interactions between NZVI surface chemistry and soil microbial ecology, emphasizing the ecological importance of surface modifications.60
Furthermore, the mobility of NZVI within environmental matrices plays an important role in determining microbial exposure. Increased mobility facilitates wider dispersion, potentially exerting more extensive effects on microbial communities by prolonging and expanding the spatial and temporal extent of exposure.77 However, long-term studies indicate that microbial communities frequently demonstrate resilience to NZVI exposure, with adaptations that mitigate initial disruptions. Practical application conditions of NZVI, such as injection methods, frequency, dosage, and environmental heterogeneity, also influence its fate and the patterns of microbial exposure. Variations in these parameters can markedly alter NZVI's spatial distribution, aggregation behavior, and ecological impacts in realistic remediation settings.16 Overall, NZVI has proven to be a valuable material for environmental applications, even when accounting for its environmental impact.
3. Summary and future recommendations
NZVIs have garnered great attention for the toxicity and potential environmental impacts due to their high reactivity, broad potential for environmental applications, and scalable synthesis. To ensure the sustainable use of NZVI in environmental remediation while minimizing ecological risks, several key considerations must be addressed.
Surface modification in NZVIs plays a pivotal role in determining their properties and passivation behavior, directly influencing their toxicity. However, not enough is known about the environmental effects of surface-modified NZVIs at the community level. Most of the work has focused on the impacts of bare NZVI. To gain a better understanding for accurate correlation between surface coatings and environmental microbial processes, systematic investigations using a model microbial community exposed to various types of surface-modified NZVIs are needed. Such information will be of great value for redesigning NZVIs that minimize adverse biological interactions. Moreover, achieving a balance between particle stability and remediation efficacy necessitates the selection of stabilizers that preserve NZVI dispersibility and reactivity, while simultaneously minimizing unintended ecotoxicological effects. Future research should prioritize systematic and comparative investigations into how different stabilizers influence the resilience of microbial communities.
Microbial communities are vital components of both natural and engineered ecosystems. Assessing the impacts of NZVI on microbial communities offers valuable insights into ecological interactions in real-world environments. The effects of NZVI exposure may be subtle, as microbial communities can be highly resilient and can quickly return to their original composition after being disturbed.78 Molecular approaches, such as metagenomics, proteomics, and metabolomics, serve as highly sensitive and efficient tools for revealing the molecular effects of nanoparticles and identifying biological functions disrupted by nanoparticles. Thus, combining omics techniques with conventional methods will enable the detection of those subtle changes and provide advanced evidence of NZVI's environmental impacts on complex biological networks.
While NZVI holds significant promise for environmental remediation, concerns remain regarding its long-term fate and associated ecological risks, particularly in relation to its persistence and interactions within environmental compartments. Processes such as passivation, aggregation, sedimentation, and complexation with NOM can prolong the environmental residence time of NZVI, thereby resulting in unintended ecological effects. Persistent NZVI particles or their transformation products may disrupt microbial communities and higher trophic levels, potentially altering ecosystem functions and biodiversity over time. Microbial community resilience—often assessed through compositional recovery—varies across temporal scales due to differences in microbial life spans and the time lag between functional and structural responses. This complexity highlights the need for long-term, field-scale studies that not only assess NZVI accumulation and transformation but also monitor microbial adaptation and recovery dynamics over extended periods. Integrating comprehensive environmental risk assessments with long-term monitoring will enable future research to optimize the NZVI applications, ensuring a balance between remediation efficacy and ecological safety. A deeper understanding of microbial recovery mechanisms across multiple time scales is essential for the development of sustainable and ecologically responsible NZVI-based remediation strategies that minimize disruption to native microbial ecosystems.
Data availability
No primary research results, software or code have been included and no new data were generated or analysed.
Author contributions
Hakwon Yoon: conceptualization, data curation, and writing – original draft preparation. Jaehwan Kim: conceptualization, data curation, and writing – original draft preparation. Eun-Ju Kim: conceptualization, supervision, writing – review and editing, and funding. Yoon-Seok Chang: supervision and editing.
Conflicts of interest
There are no conflicts to declare.
Acknowledgements
This work was supported by the National Research Foundation of Korea (NRF) grant funded by Korea government (2022R1C1C1003475 and 2023R1A2C2004069), the ‘Land/Sea-based input and fate of microplastics in the marine environment’ of Korea Institute of Marine Science & Technology Promotion (KIMST) funded by the Ministry of Oceans and Fisheries, Republic of Korea (no. 20220357) and the National Research Council of Science & Technology (NST) grant by the Korea government (MSIT) (no. CAP23022-200).
References
-
T. Phenrat and G. V. Lowry, Nanoscale zerovalent iron particles for environmental restoration, Springer, 2019 Search PubMed
.
- G. N. Glavee, K. J. Klabunde, C. M. Sorensen and G. C. Hadjipanayis, Chemistry of borohydride reduction of iron(II) and iron(III) ions in aqueous and nonaqueous media. Formation of nanoscale Fe, FeB, and Fe2B powders, Inorg. Chem., 1995, 34, 28–35 CrossRef CAS
.
- C. Wang and W. Zhang, Synthesizing nanoscale iron particles for rapid and complete dechlorination of TCE and PCB, Environ. Sci. Technol., 1997, 31, 2154–2156 CrossRef CAS
.
- M. Liu, G. Chen, L. Xu, Z. He and Y. Ye, Environmental remediation approaches by nanoscale zero valent iron (nZVI) based on its reductivity: a review, RSC Adv., 2024, 14, 21118–21138 RSC
.
- N. Saleh, K. Sirk, Y. Liu, T. Phenrat, B. Dufour, K. Matyjaszewski, R. D. Tilton and G. V. Lowry, Surface Modifications enhance nanoiron transport and NAPL targeting in saturated porous media, Environ. Eng. Sci., 2007, 24, 45–57 CrossRef CAS
.
- W. X. Zhang, C. B. Wang and H. L. Lien, Treatment of chlorinated organic contaminants with nanoscale bimetallic particles, Catal. Today, 1998, 40, 387–395 CrossRef CAS
.
- Y. Xu and W. Zhang, Subcolloidal Fe/Ag particles for reductive dehalogenation of chlorinated benzenes, Environ. Sci. Technol., 2000, 39, 2238–2244 CAS
.
- N. Zhu, H. Luan, S. Yuan, J. Chen, X. Wu and L. Wang, Effective dechlorination of HCB by nanoscale Cu/Fe particles, J. Hazard. Mater., 2010, 176, 1101–1105 CrossRef CAS PubMed
.
- E. J. Kim, J. H. Kim, A. M. Azad and Y. S. Chang, Facile synthesis and characterization of Fe/FeS nanoparticles for environmental applications, ACS Appl. Mater. Interfaces, 2011, 3, 1457–1462 CrossRef CAS PubMed
.
- D. Turcio-Ortega, D. Fan, P. G. Tratnyek, E. J. Kim and Y. S. Chang, Reactivity of Fe/FeS nanoparticles: electrolyte composition effects on corrosion electrochemistry, Environ. Sci. Technol., 2012, 46, 12484–12492 CrossRef CAS PubMed
.
- D. Fan, R. P. Anitori, B. M. Tebo, P. G. Tratnyek, J. S. L. Pacheco, R. K. Kukkadapu, M. H. Engelhard, M. E. Bowden, L. Kovarik and B. W. Arey, Reductive sequestration of pertechnetate (99TcO4−) by nano zerovalent iron (nZVI) transformed by abiotic sulfide, Environ. Sci. Technol., 2013, 47, 5302–5310 CrossRef CAS PubMed
.
- Y. Han and W. Yan, Reductive dechlorination of trichloroethene by zero-valent iron nanoparticles: reactivity enhancement through sulfidation treatment, Environ. Sci. Technol., 2016, 50, 12992–13001 CrossRef CAS PubMed
.
- B. Schrick, B. W. Hydutsky, J. L. Blough and T. E. Mallouk, Delivery vehicles for zerovalent metal nanoparticles in soil and groundwater, Chem. Mater., 2004, 16, 2187–2193 CrossRef CAS
.
- F. He and D. Zhao, Preparation and characterization of a new class of starch-stabilized bimetallic nanoparticles for degradation of chlorinated hydrocarbons in water, Environ. Sci. Technol., 2005, 39, 3314–3320 CrossRef CAS PubMed
.
- N. Saleh, T. Phenrat, K. Sirk, B. Dufour, J. Ok, T. Sarbu, K. Matyjaszewski, R. D. Tilton and G. V. Lowry, Adsorbed triblock copolymers deliver reactive iron nanoparticles to the oil/water interface, Nano Lett., 2005, 5, 2489–2494 CrossRef CAS PubMed
.
- J. Quinn, C. Geiger, C. Clausen, K. Brooks, C. Coon, S. O'Hara, T. Krug, D. Major, W. S. Yoon, A. Gavaskar and T. Holdsworth, Field demonstration of DNAPL dehalogenation using emulsified zero-valent iron, Environ. Sci. Technol., 2005, 39, 1309–1318 CrossRef CAS PubMed
.
- Y. P. Sun, X. Q. Li, W. X. Zhang and H. P. Wang, A method for the preparation of stable dispersion
of zero-valent iron nanoparticles, Colloids Surf., A, 2007, 308, 60–66 CrossRef CAS
.
- M. R. Wiesner, G. V. Lowry, P. Alvarez, D. Dionysiou and P. Biswas, Assessing the risk of manufactured nanomaterials, Environ. Sci. Technol., 2006, 40, 4326–4528 CrossRef PubMed
.
- R. A. Crane and T. B. Scott, Nanoscale zero-valent iron : future prospects for an emerging water treatment technology, J. Hazard. Mater., 2012, 211–212, 112–125 CrossRef CAS PubMed
.
-
J. Filip, T. Cajthaml, P. Najmanová, M. Černík and R. Zbořil, Advanced nano-bio technologies for water and soil treatment, Springer, 2020 Search PubMed
.
- C. Lee, J. Y. Kim, W. I. Lee, K. L. Nelson, J. Yoon and D. L. Sedlak, Bactericidal effect of zero-valent iron nanoparticles on Escherichia coli, Environ. Sci. Technol., 2008, 42, 4927–4933 CrossRef CAS PubMed
.
- K. Murugesan, V. Bokare, J. R. Jeon, E. J. Kim, J. H. Kim and Y. S. Chang, Effect of Fe-Pd bimetallic nanoparticles on Sphingomonas sp. PH-07 and a nano-bio hybrid process for triclosan degradation, Bioresour. Technol., 2011, 102, 6019–6025 CrossRef CAS PubMed
.
- Z. Li, K. Greden, P. J. J. Alvarez, K. B. Gregory and G. V. Lowry, Adsorbed polymer and NOM limits adhesion and toxicity of nano scale zerovalent iron to E. coli, Environ. Sci. Technol., 2010, 44, 3462–3467 CrossRef CAS PubMed
.
- E. J. Kim, T. L. Thanh, J. H. Kim and Y. S. Chang, Synthesis of metal sulfide-coated iron nanoparticles with enhanced surface reactivity and biocompatibility, RSC Adv., 2013, 3, 5338–5340 RSC
.
- E. J. Kim, T. L. Thanh and Y. S. Chang, Comparative toxicity of bimetallic Fe nanoparticles toward Escherichia coli: mechanism and environmental implications, Environ. Sci.: Nano, 2014, 1, 223–237 RSC
.
- E. Lefevre, N. Bossa, M. R. Wiesner and C. K. Gunsch, A review of the environmental implications of in situ remediation by nanoscale zero valent iron (nZVI): behavior, transport and impacts on microbial communities, Sci. Total Environ., 2016, 565, 889–901 CrossRef CAS PubMed
.
- V. Braun and K. Hantke, Recent insights into iron import by bacteria, Curr. Opin. Chem. Biol., 2011, 15, 328–334 CrossRef CAS PubMed
.
- C. R. Keenan and D. L. Sedlak, Factors affecting the yield of oxidants from the reaction of nanoparticulate zero-valent iron and oxygen, Environ. Sci. Technol., 2008, 42, 1262–1267 CrossRef CAS PubMed
.
- M. Auffan, W. Achouak, J. Rose, M. A. Roncato, C. Chanéac, D. T. Waite, A. Masion, J. C. Woicik, M. R. Wiesner and J. Y. Bottero, Relation between the redox state of iron-based nanoparticles and their cytotoxicity toward Escherichia coli, Environ. Sci. Technol., 2008, 42, 6730–6735 CrossRef CAS PubMed
.
- Y. Lv, Z. Niu, Y. Chen and Y. Hu, Bacterial effects and interfacial inactivation mechanism of nZVI/Pd on Pseudomonas putida strain, Water Res., 2017, 115, 297–308 CrossRef CAS PubMed
.
- L. Han, S. Patil, D. Boehm, V. Milosavljević, P. J. Cullen and P. Bourke, Mechanisms of inactivation by high-voltage atmospheric cold plasma differ for Escherichia coli and Staphylococcus aureus, Appl. Environ. Microbiol., 2016, 82, 450–458 CrossRef CAS PubMed
.
- J. Zhang, P. Su, H. Chen, M. Qiao, B. Yang and X. Zhao, Impact of reactive oxygen species on cell activity and structural integrity of Gram-positive and Gram-negative bacteria in electrochemical disinfection system, Chem. Eng. J., 2023, 451, 138879 CrossRef CAS
.
- M. Xie, E. H. W. Koch, C. A. Walree, A. Sobota, A. F. P. Sonnen, J. A. Killian, E. Breukink and J. H. Lorent, Synergistic effects of oxidative and acid stress on bacterial membranes of Escherichia coli and Staphylococcus simulans, Commun. Biol., 2024, 7, 1161 CrossRef CAS PubMed
.
- J. Chen, Z. Xiu, G. V. Lowry and P. J. J. Alvarez, Effect of natural organic matter on toxicity and reactivity of nano-scale zero-valent iron, Water Res., 2011, 45, 1995–2001 CrossRef CAS PubMed
.
- O. V. Bakina, E. A. Glazkova, N. V. Svarovskaya, N. G. Rodkevich and M. I. Lerner, «Janus»-like Cu-Fe bimetallic nanoparticles with high antibacterial activity, Mater. Lett., 2019, 242, 187–190 CrossRef CAS
.
- Q. Chen, J. Li, Y. Wu, F. Shen and M. Yao, Biological responses of Gram-positive and Gram-negative bacteria to nZVI (Fe0), Fe2+ and Fe3+, RSC Adv., 2013, 3, 13835–13842 RSC
.
- A. H. Sadek, M. S. Asker and S. A. Abdelhamid, Bacteriostatic impact of nanoscale zero-valent iron against pathogenic bacteria in the municipal wastewater, Biologia, 2021, 76, 2785–2809 CrossRef CAS PubMed
.
- Q. Liu, C. Liu, S. Wang, L. Zhang, H. Sun and X. Liao, Differing envelope composition of Gram-negative and Gram-positive bacteria controls the adhesion and bactericidal performance of nanoscale zero-valent iron, J. Hazard. Mater., 2025, 137663 CrossRef CAS PubMed
.
- S. Jyung, J. W. Kang and D. H. Kang, L. monocytogens exhibited less cell membrane damage, lipid peroxidation, and intracellular reactive oxygen species accumulation after plasma-activated water treatment compared to E. coli O157:H7 and S. Typhimurium, Food Microbiol., 2022, 108, 104098 CrossRef CAS PubMed
.
- Y. H. Hsueh, P. H. Tsai, K. S. Lin, W. J. Ke and C. L. Chiang, Antimicrobial effects of zero-valent iron nanoparticles on gram-positive Bacillus strains and gram-negative Escherichia coli strains, J. Nanotechnol., 2017, 15, 77 Search PubMed
.
- L. Zhou, T. L. Thanh, J. Gong, J. H. Kim, E. J. Kim and Y. S. Chang, Carboxymethyl cellulose coating decreases toxicity and oxidizing capacity of nanoscale zerovalent iron, Chemosphere, 2014, 104, 155–161 CrossRef CAS PubMed
.
- H. H. Kim, M. S. Kim, H. E. Kim, H. J. Lee, M. H. Jang, J. Choi, Y. Hwang and C. Lee, Nanoparticulate zero-valent iron coupled with polyphosphate: the sequential redox treatment of organic compounds and its stability and bacterial toxicity, Environ. Sci.: Nano, 2017, 4, 396–405 RSC
.
- M. Diao and M. Yao, Use of zero-valent iron nanoparticles in inactivating microbes, Water Res., 2009, 43, 5243–5251 CrossRef CAS PubMed
.
- L. Li, H. Dong, Y. Lu, H. Zhang, Y. Li, J. Xiao, S. Xiao and Z. Jin, In-depth exploration of toxicity mechanism of nanoscale zero-valent iron and its aging products toward Escherichia coli under aerobic and anaerobic conditions, Environ. Pollut., 2022, 313, 120118 CrossRef CAS PubMed
.
- Q. Xie, L. Li, H. Dong, R. Li, R. Tian and J. Chen, Influence of several crucial groundwater components on the toxicity of nanoscale zero-valent iron towards Escherichia coli under aerobic and anaerobic conditions, Chemosphere, 2021, 285, 131453 CrossRef CAS PubMed
.
- J. Wang, C. Liu, H. Sun, S. Wang, X. Liao and L. Zhang, Membrane disruption boosts iron overload and endogenous oxidative stress to inactivate Escherichia coli by nanoscale zero-valent iron, J. Hazard. Mater., 2022, 435, 128951 CrossRef CAS PubMed
.
- Y. Xie, H. Dong, G. Zeng, L. Tang, Z. Jiang, C. Zhang, J. Deng, L. Zhang and Y. Zhang, The interactions between nanoscale zero-valent iron and microbes in the subsurface environment: A review, J. Hazard. Mater., 2017, 321, 390–407 CrossRef CAS PubMed
.
- J. Filip, F. Karlický, Z. Marušák, P. Lazar, M. Černík, M. Otyepka and R. Zbořil, Anaerobic reaction of nanoscale zerovalent iron with water: Mechanism and kinetics, J. Phys. Chem., 2014, 118, 13817–13825 CAS
.
- N. A. Beauchene, E. L. Mettert, L. J. Moore, S. Keleş, E. R. Willey and P. J. Kiley, O2 availability impacts iron homeostasis in Escherichia coli, Proc. Natl. Acad. Sci. U. S. A., 2017, 114, 12261–12266 CrossRef CAS PubMed
.
- B. D. Bennett and J. A. Gralnick, Mechanisms of toxicity by and resistance to ferrous iron in anaerobic systems, Free Radical Biol. Med., 2019, 140, 167–171 CrossRef CAS PubMed
.
- J. P. Kehrer, The Haber–Weiss reaction and mechanisms of toxicity, Toxicology, 2000, 149, 43–50 CrossRef CAS PubMed
.
- G. Zeng, Y. He, F. Wang, H. Luo, D. Liang, J. Wang, J. Huang, C. Yu, L. Jin and D. Sun, Toxicity of Nanoscale Zero-Valent Iron to Soil Microorganisms and Related Defense Mechanisms: A Review, Toxics, 2023, 11, 514 CrossRef CAS PubMed
.
- M. L. Saccà, C. Fajardo, M. Martinez-Gomariz, G. Costa, M. Nande and M. Martin, Molecular Stress Responses to Nano-Sized Zero-Valent Iron (nZVI) Particles in the Soil Bacterium Pseudomonas stutzeri, PLoS One, 2014, 9, e89677 CrossRef PubMed
.
- C. Fajardo, M. L. Saccà, M. Martinez-Gomariz, G. Costa, M. Nande and M. Martin, Transcriptional and proteomic stress responses of a soil bacterium Bacillus cereus to nanosized zero-valent iron (nZVI) particles, Chemosphere, 2013, 93, 1077–1083 CrossRef CAS PubMed
.
- X. Wang, Y. Che, Y. Xu, Y. Wu, H. Xu and L. Li, Mechanisms of nano zero-valent iron in enhancing dibenzofuran degradation by a Rhodococcus sp.: Trade-offs between ATP production and protection against reactive oxygen species, J. Hazard. Mater., 2025, 481, 136502 CrossRef CAS PubMed
.
- Y. N. Slavin, J. Asnis, U. O. Häfeli and H. Bach, Metal nanoparticles: understanding the mechanisms behind antibacterial activity, J. Nanotechnol., 2017, 15, 65 Search PubMed
.
- Y. Hu, J. Wang, H. Sun, S. Wang, X. Liao, J. Wang and T. An, Roles of extracellular polymeric substances in the bactericidal effect of nanoscale zero-valent iron: trade-offs between physical disruption and oxidative damage, Environ. Sci.:Nano, 2019, 6, 2061–2073 RSC
.
- R. J. Barnes, C. J. van der Gast, O. Riba, L. E. Lehtovirta, J. I. Prosser, P. J. Dobson and I. P. Thompson, The impact of zero-valent iron nanoparticles on a river water bacterial community, J. Hazard. Mater., 2010, 184, 73–80 CrossRef CAS PubMed
.
- M. T. Gómez-Sagasti, L. Epelde, M. Anza, J. Urra, I. Alkorta and C. Garbisu, The impact of nanoscale zero-valent iron particles on soil microbial communities is soil dependent, J. Hazard. Mater., 2019, 364, 591–599 CrossRef PubMed
.
- C. Hui, B. Liu, L. Du, L. Xu, Y. Zhao, D. Shen and Y. Long, Transformation of sulfidized nanoscale zero-valent iron particles and its effects on microbial communities in soil ecosystems, Environ. Pollut., 2022, 306, 119363 CrossRef CAS PubMed
.
- Y. Yang, G. Jialiang and H. Zhiqiang, Impact of nano zero valent iron (NZVI) on methanogenic activity and population dynamics in anaerobic digestion, Water Res., 2013, 47, 6790–6800 CrossRef CAS PubMed
.
- T. L. Kirschling, K. B. Gregory, E. G. Minkley, G. V. Lowry and R. D. Tilton, Impact of nanoscale zero valent iron on geochemistry and microbial populations in trichloroethylene contaminated aquifer materials, Environ. Sci. Technol., 2010, 44, 3474–3480 CrossRef CAS PubMed
.
- E. L. Tilston, C. D. Collins, G. R. Mitchell, J. Princivalle and L. J. Shaw, Nanoscale zerovalent iron alters soil bacterial community structure and inhibits chloroaromatic biodegradation potential in Aroclor 1242-contaminated soil, Environ. Pollut., 2013, 173, 38–46 CrossRef CAS PubMed
.
- C. M. D. Kocur, L. Lomheim, O. Molenda, K. P. Weber, L. M. Austrins, B. E. Sleep, H. K. Boparai, E. A. Edwards and D. M. O'Carroll, Long-term field study of microbial community and dechlorinating activity following carboxymethyl cellulose-stabilized nanoscale zero-valent iron
injection, Environ. Sci. Technol., 2016, 50, 7658–7670 CrossRef CAS PubMed
.
- P. Shao, Y. Chen, D. Gu, J. Zeng, S. Zhang, Y. Wu and X. Lin, Resistance and resilience of soil bacterial community to zero-valent iron disposal of lindane contamination, Chemosphere, 2022, 306, 135612 CrossRef CAS PubMed
.
- L. Hainan, L. Peng, L. Qingqing, L. Fang, Z. Dong, H. Shenfa, Y. Jie and L. Zhiheng, Responses of nitrobenzene removal performance and microbial community by modified biochar supported zerovalent iron in anaerobic soil, Sci. Rep., 2024, 14, 17078 CrossRef PubMed
.
- Q. Liu, Y. Sheng, W. Wang and X. Liu, Efficacy and microbial responses of biochar-nanoscale zero-valent during in-situ remediation of Cd-contaminated sediment, J. Cleaner Prod., 2021, 287, 125076 CrossRef CAS
.
- N. Liu, Y. Zhang, C. Zheng, C. Tang, J. Guan and Y. Guo, Sulfidated nanoscale zero valent iron for in situ immobilization of hexavalent chromium in soil and response of indigenous microbes, Chemosphere, 2023, 344, 140343 CrossRef CAS PubMed
.
- Š. Lewandowská, Z. Vaňková, L. Beesley, T. Cajthaml, N. Wickramasinghe, J. Vojar, M. Vítková, D. C. W. Tsang, K. Ndungu and M. Komárek, Nano zerovalent Fe did not reduce metal (loid) leaching and ecotoxicity further than conventional Fe grit in contrasting smelter impacted soils: A 1-year field study, Sci. Total Environ., 2024, 927, 171892 CrossRef PubMed
.
- R. Su, H. Fu, L. Ding, B. Fu, S. He, H. Ma, H. Hu and H. Ren, Long-term impact of nano zero-valent iron on methanogenic activity, microbial community structure, and transcription activity in anaerobic wastewater treatment system, Bioresour. Technol., 2024, 393, 130028 CrossRef CAS PubMed
.
- W. Liu, J. Li, J. Li, M. Zheng and J. Meng, Long-term effect of zero-valent iron on one-stage partial nitritation and anammox, Chem. Eng. J., 2023, 455, 140598 CrossRef CAS
.
- C. Jiang, X. Xu, M. Megharaj, R. Naidu and Z. Chen, Inhibition or promotion of biodegradation of nitrate by Paracoccus sp. in the presence of nanoscale zero-valent iron, Sci. Total Environ., 2015, 530, 241–246 CrossRef PubMed
.
- B. Wang, L. Lu, Y. Zhang, K. Fang, D. An and H. Li, Removal of bisphenol A by waste zero-valent iron regulating microbial community in sequencing batch biofilm reactor, Sci. Total Environ., 2021, 753, 142073 CrossRef CAS PubMed
.
- Y. Zhong, J. He, P. Zhang, X. Zou, X. Pan and J. Zhang, Effects of different particle size of zero-valent iron (ZVI) during anaerobic digestion: performance and mechanism from genetic level, Chem. Eng. J., 2022, 435, 134977 CrossRef CAS
.
- L. Philippot, B. S. Griffiths and S. Langenheder, Microbial Community Resilience across Ecosystems and Multiple Disturbances, Microbiol. Mol. Biol. Rev., 2021, 85, e00026 CrossRef PubMed
.
- S. Wu, T. Cajthaml, J. Semerád, A. Filipová, M. Klementová, R. Skala, M. Vítková, Z. Michálková, M. Teodoro, Z. Wu, D. Martínez-Fernández and M. Komárek, Nano zero-valent iron aging interacts with the soil microbial community: a microcosm study, Environ. Sci.:Nano, 2019, 6, 1189–1206 RSC
.
- S. R. Kanel, R. R. Goswami, T. P. Clement, M. O. Barnett and D. Zhao, Two dimensional transport characteristics of surface stabilized zero-valent iron nanoparticles in porous media, Environ. Sci. Technol., 2008, 42, 896–900 CrossRef CAS PubMed
.
- B. S. Griffiths and L. Philippot, Insights into the resistance and resilience of the soil microbial community, FEMS Microbiol. Rev., 2013, 37, 112–129 CrossRef CAS PubMed
.
Footnote |
† These authors contributed equally. |
|
This journal is © The Royal Society of Chemistry 2025 |
Click here to see how this site uses Cookies. View our privacy policy here.