DOI:
10.1039/D4TC02806F
(Paper)
J. Mater. Chem. C, 2024,
12, 17338-17346
Near-infrared emitting single- and mixed-ligand MOFs that can be excited in the visible region: synthesis, crystal structures and sensitization of Nd3+, Er3+ and Yb3+†
Received
2nd July 2024
, Accepted 9th September 2024
First published on 14th September 2024
Abstract
Near-infrared (NIR) luminescent lanthanide(III) (Ln3+) metal–organic frameworks (MOFs) constitute a class of materials possessing unique advantages for a wide range of applications due to their photophysical and structural properties. In this work, we present the synthesis and characterization of two series of Ln3+-based MOFs emitting in the NIR region (Ln-MOFs) constructed using 2,6-naphthalene dicarboxylic acid (H2NDC) and its two amino derivatives, 4-aminonaphthalene-2,6-dicarboxylic acid (H2ANDC) and 4,8-diaminonaphthalene-2,6-dicarboxylic acid (H2DANDC). The first series comprises mixed-ligand and mixed-metal MOFs with the general formula [La1−xLnx(NDC)1−y(ANDC)yCl(DMF)] (Ln = Nd3+, Yb3+, x = 0.005–1, y = 0–0.2), while the second series features MOFs corresponding to the formulae [La1−xLnx((NDC)1−y(ANDC)y)1.5(DMF)2]·DMF (Ln = Nd3+, Er3+, Yb3+, Lu3+, x = 0.1 or 1, y = 0.45 or 1) and [Ln(DANDC)1.5(DMF)2]·DMF (Ln = Nd3+, Er3+, Yb3+). Detailed studies of the photophysical properties of Ln-MOFs have demonstrated that MOF scaffolds provide an efficient sensitization of NIR-emitting Ln3+ ions (ηsens up to 38% for Nd3+ analogues). In addition, Ln-MOFs possess long Ln3+ luminescence lifetimes (up to 12 μs for Yb3+ analogues) and exhibit Ln3+ luminescence quantum yields comparable with the highest values reported today for NIR-emitting Ln-MOFs. We discuss and compare the photophysical processes that lead to Ln3+ sensitization in two series of Ln-MOFs. Most importantly, the studied Ln-MOFs are among the few examples of Ln3+-based NIR-emitting MOFs where Ln3+ emission can be sensitized upon excitation in the visible range (up to 450 nm).
Introduction
Near-infrared (NIR) luminescent materials have been the subject of intense research over the last few decades primarily in the fields of telecommunications, light emitting diodes, lasers, sensing and biological imaging.1–4 Thanks to recent major advances in light detection technology, analytical techniques based on luminescence are reaching sensitivity levels comparable to those attained by methods based on radioactive isotopes.5–8 As a result, optical imaging methods, such as fluorescence microscopy, have gained an increasing importance.9–11 For instance, today, NIR fluorescence imaging offers molecular specificity, real-time detection, and tissue penetration in the centimeter range.12–14 Currently, the field of NIR bioimaging agents primarily involves highly conjugated organic fluorophores, which, despite significant success, suffer from important limitations associated with the nature of their fluorescence due to spin-allowed transitions, including small Stokes shifts and short emission lifetimes (typically in the ns range) comparable to those of the biological background (autofluorescence), thus precluding the use of temporal discrimination for the removal of this unwanted signal.15 Furthermore, organic NIR fluorescent probes very often exhibit strong and rapid photobleaching. They exhibit broad emission bands within the NIR-I imaging window (650–900 nm), while those covering the NIR-II window (1000–1700 nm) are relatively rare.16,17 In contrast, NIR-emitting trivalent lanthanide ions (Ln3+) such as Nd3+, Er3+ and Yb3+ display long-lived emission signals (up to several μs), and sharp, atomic-like emission profiles, the wavelengths of which are largely unaffected by the environment around the Ln3+ centre.18 Additionally, Ln3+ emission bands lie within the NIR-I and NIR-II imaging windows and thus offer an attractive complementary alternative to organic fluorophores.19–22 The forbidden nature of most f–f transitions (typical absorption coefficients < 1–10 M−1 cm−1) implies the generation of a low luminescence intensity through direct Ln3+ excitation, and thus can only be achieved by the use of high-power sources such as lasers. This limitation can be tackled by the indirect population of f–f excited states via energy transfer from highly absorbing organic chromophores which are either in sufficiently close proximity or directly coordinated to Ln3+, an approach known as the “antenna effect” or “Ln3+ sensitisation”.18,23 Despite the existence of a large number of antenna ligands for the efficient sensitisation of visible-emitting Ln3+, the emission quantum yields of NIR-emitting Ln3+ complexes are generally modest (typically ≪ 1%) because of strong non-radiative deactivation of low-lying f–f excited states by the overtones of high-energy oscillators such as O–H, N–H and C–H bonds that are often present in the vicinity of the Ln3+ centre. Approaches to alleviate this limitation include the use of deuterated or fluorinated ligands,24–27 the synthesis of metallacrowns28–30 where the Ln3+ is encapsulated in a d-metal containing scaffold devoid of high energy oscillators, and the study of dendritic systems.31–34
Ln3+ metal–organic frameworks (Ln-MOFs) constitute a highly promising class of NIR-emitting materials as they allow incorporation of a large number of antenna ligands and emitting ions per unit volume within a rigid framework. This densification strategy has been proven to be successful for generating sufficiently strong and easily recordable signals despite the limited emission of each individual Ln3+ centre.35–38 An additional attractive feature of Ln-MOFs is the combination of Ln3+ luminescence properties and the porosity of their structures, enabling them to potentially host a variety of chemical species. Thus, Ln-MOFs are highly promising platforms for numerous applications such as luminescence sensing,39–41 ratiometric thermometry,42–46 anticounterfeiting,47 encoding applications,48–54 bioimaging and biosensing.36,55–59 For the latter applications, it is a major advantage to ensure that the Ln3+ emission is sensitised in the visible/NIR region in order to avoid unwanted effects caused by UV irradiation to biological systems and to maximise the penetration of the excitation light within biological tissues and fluids. It is thus important to gain a deeper understanding of the various processes involved in the sensitization of NIR-emitting Ln3+ for the rational design of luminescent probes.
In this work, we describe the design, synthesis and photophysical properties of two series (series 1 and 2) of NIR-emitting MOFs incorporating three Ln3+ of different natures, Nd3+, Er3+ and Yb3+ as emitting centres, and the ligands 2,6-naphthalene dicarboxylate (NDC2−), 4-aminonaphthalene-2,6-dicarboxylate (ANDC2−) and 4,8-diaminonaphthalene-2,6-dicarboxylate (DANDC2−) as Ln3+ sensitizers. Series 1 and 2 differ by their structures. In addition to MOFs containing only one type of ligand and metal ion, we have also synthesized mixed-ligand and mixed-metal Ln-MOFs for both series to perform a more detailed systematic investigation of the photophysical processes leading to Ln3+ sensitization and of the quenching pathways due to intermetallic Ln–Ln energy transfer. The Ln-MOFs of series 1 can be described by the general formula [La1−xLnx(NDC)1−y(ANDC)yCl(DMF)] (shorthand 1-LnxANDCy, Ln = Nd3+, Yb3+, x = 0.005–1, y = 0–0.2; DMF = dimethylformamide) and those of series 2 can be defined by the general formulae [La1−xLnx((NDC)1−y(ANDC)y)1.5(DMF)2]·DMF (shorthand 2-LnxANDCy, Ln = Nd3+, Er3+, Yb3+, Lu3+, x = 0.1 or 1, y = 0.45 or 1) and [Ln(DANDC)1.5(DMF)2]·DMF (shorthand 2-LnDANDC, Ln = Nd3+, Er3+, Yb3+) (Table 1). We demonstrate that the NIR Ln3+ emission can be sensitized upon excitation of the ligands at wavelengths up to 450 nm. We discuss how the structural characteristics of these Ln-MOFs impact the energy transfer and Ln3+ sensitization processes. Overall, the Ln-MOFs synthesized in this work show promising potential for the design of NIR optical imaging agents for biological applications.
Table 1 List of synthesized Ln-MOFs discussed in the main texta
Series |
Metal (mol%) |
Ligand (mol%) |
Code |
Series |
Metal (mol%) |
Ligand (mol%) |
Code |
General formulae of the Ln-MOFs: [La1−xLnx(NDC)1−y(ANDC)yCl(DMF)] (shorthand 1-LnxANDCy, Ln = Nd3+, Yb3+, x = 0.005–1, y = 0–0.2; DMF = dimethylformamide), [La1−xLnx((NDC)1−y(ANDC)y)1.5(DMF)2]·DMF (shorthand 2-LnxANDCy, Ln = Nd3+, Er3+, Yb3+, Lu3+, x = 0.1 or 1, y = 0.45 or 1) and [Ln(DANDC)1.5(DMF)2]·DMF (shorthand 2-LnDANDC, Ln = Nd3+, Er3+, Yb3+).
|
1 |
Yb |
H2ANDC |
|
1 |
Nd |
H2ANDC |
|
0.5 |
20 |
1-Yb0.005ANDC0.2 |
100 |
0 |
1-Nd1NDC |
1 |
1-Yb0.01ANDC0.2 |
100 |
1 |
1-Nd1ANDC0.01 |
2 |
1-Yb0.02ANDC0.2 |
100 |
2 |
1-Nd1ANDC0.02 |
5 |
1-Yb0.05ANDC0.2 |
100 |
5 |
1-Nd1ANDC0.05 |
10 |
1-Yb0.1ANDC0.2 |
100 |
10 |
1-Nd1ANDC0.1 |
15 |
1-Yb0.15ANDC0.2 |
100 |
20 |
1-Nd1ANDC0.2 |
20 |
1-Yb0.2ANDC0.2 |
2 |
Nd |
100 |
2-Nd1ANDC1 |
30 |
1-Yb0.3ANDC0.2 |
Nd |
45 |
2-Nd1ANDC0.45 |
50 |
1-Yb0.5ANDC0.2 |
Er |
100 |
2-Er1ANDC1 |
2 |
100 |
100 |
2-Yb1ANDC1 |
Yb |
100 |
2-Yb1ANDC1 |
10 |
2-Yb0.1ANDC1 |
|
H2DANDC |
|
Lu |
100 |
2-LuANDC |
Er |
100 |
2-ErDANDC |
|
|
|
Nd |
100 |
2-NdDANDC |
|
|
|
Yb |
100 |
2-YbDANDC |
Results and discussion
Synthesis
We synthesized two series of Ln-MOFs (Ln = La3+, Nd3+, Er3+, Yb3+) assembled using the carboxylic acids H2NDC, H2ANDC and H2DANDC (the doubly deprotonated forms of the organic ligands are referred in the main text as NDC, ANDC and DANDC). Ln-MOFs of both series were prepared using modified methods previously described by our group.60 The synthesis of series 1 Ln-MOFs was performed using Ln3+ chlorides as metal sources in combination with the mixtures of H2NDC/H2ANDC; the molar ratio of H2ANDC
:
H2NDC was varied from 0 to 0.25 while the total ligand to metal molar ratio was kept at 1
:
1. The reaction was conducted at 110 °C during 24 h in DMF. In addition, we synthesized Yb3+-doped MOFs by adding specified amounts of a standard solution of the dopant in DMF to the reaction mixture. The Ln-MOFs of series 1 can be described by the following formula [La1−xLnx(NDC)1−y(ANDC)yCl(DMF)] (Ln = Nd3+, Yb3+, x = 0.005–1, y = 0–0.2). Series 2 features Nd3+, Er3+ and Yb3+ Ln-MOFs of the general formulae ([La1−xLnx((NDC)1−y(ANDC)y)1.5(DMF)2]·DMF (x = 0.1 or 1, y = 0.45 or 1) and [Ln(DANDC)1.5(DMF)2]·DMF). They were synthesized by the reaction between the Ln3+ nitrates, as the metal sources, with the ligands H2ANDC and H2DANDC in a 1
:
1.5 molar ratio in a DMF/H2O/EtOH 10
:
1
:
1 solvent mixture at 70 °C for 72 h. A detailed list of the synthesized Ln-MOFs is provided in Table 1.
Crystal structures from X-ray diffraction on single crystals
Ln-MOFs of series 1 crystalize in the orthorhombic space group Pnma. We have previously reported the crystal structure of the visible light-emitting 1-LaNDC MOF,60 and here we will only briefly describe the one of 1-NdNDC. The crystal structures of series 1 and 2 Ln-MOFs are summarized in Fig. 1. The Nd3+ ion is coordinated to nine atoms, six carboxylate oxygen atoms from the NDC ligand, one oxygen atom from a terminal DMF molecule and two chloride anions. The coordination polyhedron is best described as a tricapped trigonal prism. The metal centres are bridged by the chloride ions and the carboxylate oxygens to form infinite zig–zag chains along the a axis. Neighbouring chains are connected by bridging NDC ligands to form a 3D framework with rhombic channels along the a axis, which are filled with terminally coordinated DMF molecules.
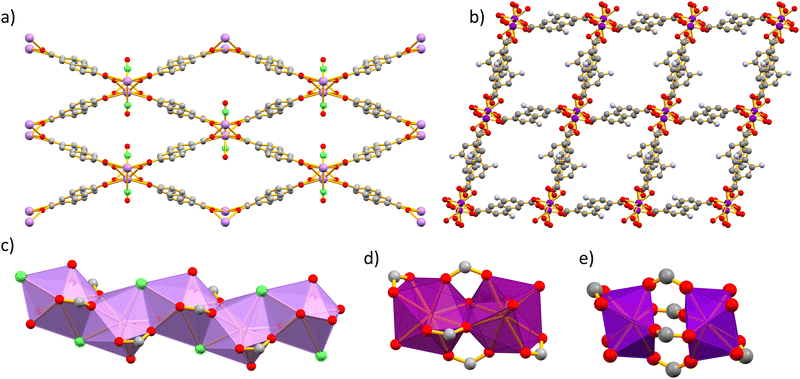 |
| Fig. 1 Crystal structures obtained from X-ray diffraction on single crystals of (a) series 1 Ln-MOFs viewed along the a axis and (b) series 2 viewed along the a axis (the DMF molecules are omitted for clarity). (c) The zig–zag chain of the [NdO8Cl] polyhedra of series 1 Ln-MOFs viewed along the c axis. (d)–(e) Secondary building units (SBUs) of series 2 Ln-MOFs. Colour code: O: red, C: grey, N: blue, Cl: green, Nd: lilac, Er: magenta, and Yb: purple. | |
Single-crystal X-ray analysis of Ln-MOFs from series 2 revealed two closely related structures, associated with the early (e.g. Nd3+) and the late (e.g. Yb3+) lanthanide(III) ions. The 2-Nd1ANDC1 MOF crystalizes in the triclinic space group P
. The Nd3+ centre is coordinated with nine oxygen atoms, namely seven from the carboxylate groups of the ANDC ligand and two from the terminally coordinated DMF molecules. The coordination [NdO9] polyhedron is best described as a distorted capped square antiprism. Two adjacent Nd3+ polyhedra form an edge-sharing [Nd2O16] dimer (Fig. 1d). Neighbouring dimers are bridged by ANDC ligands with hapticities μ2: η1–η2 and μ2: η1–η1 forming a three-dimensional framework with parallelepiped channels along the a axis, which are occupied by terminally coordinated and guest DMF molecules.
The Ln-MOFs 2-Yb1ANDC1 and 2-LuANDC share the general structural characteristics with 2-Nd1ANDC1, with a few subtle differences attributed to the lanthanide(III) contraction.61 First, the Yb3+ and Lu3+ centres are coordinated to a total of eight oxygen atoms, six from carboxylate groups of ANDC and two from the terminally coordinated DMF molecules, with the [LnO8] coordination polyhedra (Ln = Yb3+, Lu3+) being best described as distorted square antiprisms. Two adjacent polyhedra are bridged by four μ2: η1–η1 carboxylate groups from ANDC ligands to form the dimeric SBU which, in this case, comprises two distinct [LnO8] coordination polyhedra (Fig. 1e).
Powder X-ray diffraction
The phase purity of the Ln-MOFs was analyzed by powder X-ray diffraction (PXRD) measurements (Fig. S4 and S5, ESI†). It was demonstrated that 1-LnxANDCy MOFs retained their phase purity up to doping concentrations of x ≤ 0.2 and y ≤ 0.5 (Fig. S4, ESI†). Furthermore, when the value of y is more than 0.3, formation of the P
phase is preferred. The PXRD patterns of 2-LnxANDCy and 2-LnDANDC are in a good agreement with their respective simulated diagrams. In addition, there is a shift of the diffraction peak appearing at the lowest angle for 2-LnANDC and 2-LnDANDC MOFs that depends on the nature of Ln3+ ion. More specifically, the observed peak positions range from 2-theta values equal to 6.85° for Ln = Nd3+ to 7.00° for Ln = Er3+ and 7.03° for Ln = Yb3+. This observation reflects a slight shrinkage of the MOF, which can be attributed to lanthanide(III) contraction (Fig. S5, ESI†).61
Photophysical studies
In order to assess the ability of our new MOFs to sensitize Nd3+, Yb3+ and Er3+, steady-state and time-resolved luminescence spectra were collected on microcrystalline samples of Ln-MOFs at room temperature. The absorption spectra of the studied bridging ligands in MeOH solution are given in Fig. 2. H2NDC displays two structured π–π* absorption bands in the UV range centred at 280 and 340 nm with the absorption tailing off at ca. 360 nm. H2ANDC and H2DANDC show similar absorption features in the UV range in addition to two substantially red-shifted absorption bands centred at 385 and 412 nm, respectively. The latter features, that tail off at ca. 450 (H2ANDC) and 460 nm (H2DANDC), can be assigned to charge transfer transitions involving an electron from an orbital localized mainly on the amino group to a vacant π* orbital of the aromatic core.62
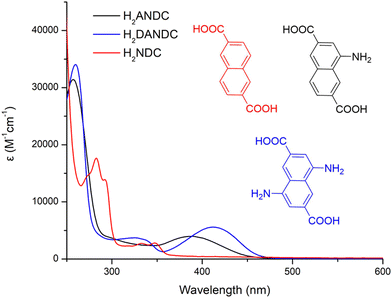 |
| Fig. 2 Absorption spectra of the ligands H2NDC, H2ANDC and H2DANDC in MeOH (10−5 M). | |
Representative optical spectra of Ln-MOFs are collected in Fig. 3. Excitation spectra of 2-Nd1ANDC1, 2-Er1ANDC1 and 2-Yb1ANDC1 MOFs upon monitoring of the emission of the respective Ln3+ (Nd3+: 4F3/2 → 4I11/2 at 1064 nm, Er3+: 4I13/2 → 4I15/2 at 1525 nm and Yb3+: 2F5/2 → 2F7/2 at 980 nm) exhibit similar broad bands in the range of 250–470 nm (Fig. 3f). This observation confirms that for all three 2-LnANDC MOFs the sensitization of different NIR-emitting Ln3+ ions is occurring through the electronic states of the chromophoric ligands. The excitation spectra of 1-LnxANDCy MOFs in the range up to 380 nm are dominated by the bands associated with the 2,6-naphthalene dicarboxylate chromophoric group. The ANDC contribution appears as a shoulder located around 400 nm, which becomes more pronounced as the ANDC content increases (Fig. 3c, d and Fig. S6, ESI†). Additionally, we observed narrow bands that correspond to direct excitation occurring through the f–f transitions of the respective Ln3+. For example, 2-Nd1ANDC1 exhibits these narrow excitation bands at 524, 582, 683, 746, 803 and 867 nm that can be attributed to the Nd3+ 4I9/2 → 4G7/2, 4G5/2, 2H11/2,4F9/2, 4F7/2 + 4S3/2 and 4F5/2 + 2H9/2 transitions, respectively. 2-ErANDC shows the Er3+-based narrow excitation bands at 520 and 656 nm arising from the 4I15/2 →2H11/2, 4F9/2 transitions, respectively. On the other hand, no such sharp features are observed in the excitation spectrum of 2-YbANDC since Yb3+ does not possess any electronic levels in the UV-visible range.
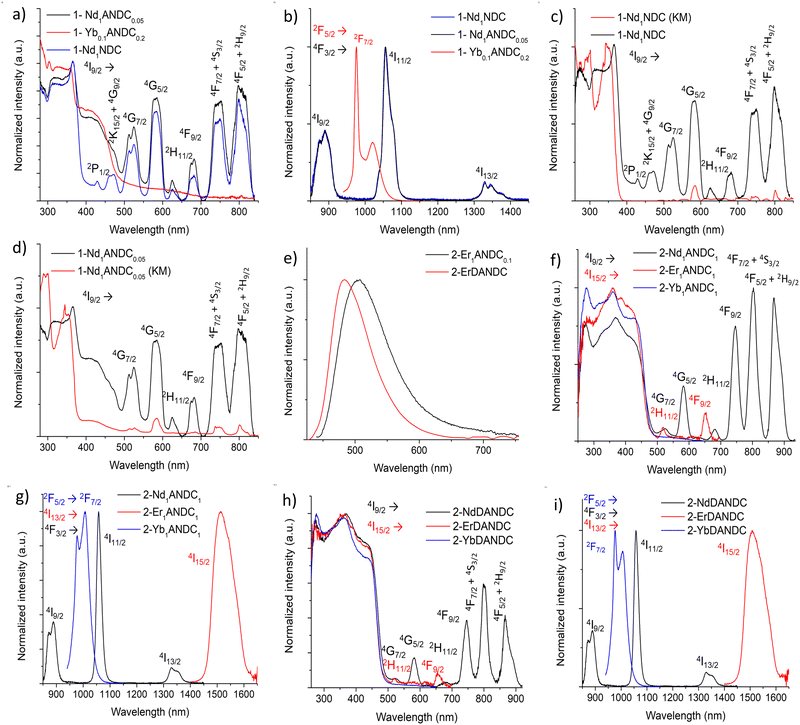 |
| Fig. 3 (a) Excitation spectra of 1-Nd1ANDC0.05, 1-Yb0.1ANDC0.2 and 1-Nd1NDC. (b) Emission spectra of 1-Nd1NDC, 1-Nd1ANDC0.05 and 1-Yb0.1ANDC0.2 (λexc = 350 nm). Overlay of excitation and diffuse reflectance spectra, after conversion to Kubelka–Munk (KM) function, for (c) 1-Nd1NDC and (d) 1-Nd1ANDC0.05. (e) Representative ligand-centred emission spectra of 2-Er1ANDC0.1 and 2-ErDANDC (λexc = 400 nm). (f) Excitation spectra of 2-Nd1ANDC1, 2-Er1ANDC1 and 2-Yb1ANDC1 and (g) emission spectra of 2-Nd1ANDC1, 2-Er1ANDC1 and 2-Yb1ANDC1 (λexc = 400 nm). (h) Excitation spectra of 2-NdDANDC, 2-ErDANDC and 2-YbDANDC and (i) emission spectra of 2-NdDANDC, 2-ErDANDC and 2-YbDANDC (λexc = 400 nm). The excitation spectra were recorded upon monitoring the emission maximum of the corresponding Ln3+. All spectra were corrected for detector response and normalized. | |
Upon excitation of the chromophoric ligands at 400 nm, 2-LnANDC MOFs (Ln = Nd, Er, Yb) exhibit characteristic Ln3+ luminescence signals indicating that the ligand sensitizes all three NIR-emitting Ln3+ ions via the antenna effect (Fig. 3g). In particular, the emission spectrum of 2-NdANDC revealed the presence of the Nd3+ bands centred at 873, 1056 and 1330 nm, attributed to the 4F3/2 → 4I9/2, 4I11/2, and 4F3/2 → 4I13/2 electronic transitions, while those of 2-ErANDC and 2-YbANDC exhibit the Er3+ (centered at 1514 nm) and Yb3+ (centered at 978 nm) emission bands attributed to the 4I13/2 →4I15/2 and 2F5/2 → 2F7/2 transitions, respectively. The 2-LnDANDC MOFs show similar behaviour (Fig. 3h–i) with respect to the sensitization of NIR-emitting Ln3+. In addition, both series of Ln-MOFs containing DANDC or ANDC ligands exhibit residual broad-band ligand-centred emission in the visible range centred at 480 and 500 nm, respectively (Fig. 3e), indicating that the energy transfer from the MOF sensitizers to the Ln3+ is incomplete for all three Ln3+ cations investigated in this work.
We further investigated the contribution of the NDC ligand on the sensitization of NIR-emitting Ln3+ by analyzing the photophysical properties of the mixed-ligand Ln-MOFs, i.e. 1-Nd1ANDCy (y = 0–0.2) and 1-YbxANDC0.2 (x = 0.005–0.5). We observed that, in both cases, upon excitation either into the NDC-centred bands at 350 nm or into the ANDC-centred bands at 415 nm, the characteristic emissions of Nd3+ and Yb3+ in the NIR range are generated (Fig. 3a and b and Fig. S6, S7, ESI†). This observation indicates that NDC contributes to the sensitization of the NIR-emitting Ln3+ either directly or indirectly via an NDC-to-ANDC energy transfer.60 Excitation spectra of 1-LnxANDCy MOFs (Fig. 3c and d) exhibit similar ligand-centred spectral features as the ones observed in the diffuse reflectance spectra further confirming Ln3+ sensitization through the Ln-MOF ligands. Moreover, in the case of 1-NdxANDCy MOFs, sharp features corresponding to the Nd3+ transitions are observed. It should be noted that the band located in the range 400–450 nm, attributed to the presence of the ANDC ligand, becomes more pronounced upon increasing the ANDC content in the 1-LnxANDCy MOFs (Fig. S6 and S7, ESI†). The absolute quantum yields of Ln3+ emission under excitation into the electronic levels of the chromophoric ANDC and DANDC ligands (ΦLLn) were collected for all relevant Ln-MOFs (Table 2). It was found that for 1-NdANDCy (y = 0.05–0.2) MOFs the ANDC loading level has no effect on the Nd3+ emission quantum yields even when the excitation takes place selectively on the ANDC chromophore. This observation potentially reflects the presence of a mechanism in which initial ANDC excitation is followed by the thermal equilibration of the NDC and ANDC triplet (T1) levels (ΔE ≈ 1600 cm−1, vide infra), with both acting as sensitizers for Nd3+. In contrast to 1-NdANDCy MOFs, the quantum yields of the Yb3+-based emission in mixed-metal Yb/La-MOFs with x = 0.1 show significant dependence on the ANDC loading. Notably, there is a ten-fold increase of the Yb3+ emission quantum yield, upon excitation at 410 nm, between 1-Yb0.1ANDC0.2 and 2-Yb0.1ANDC1 where the ANDC content increases from 20 to 100 mol%. This can be mainly attributed to the more efficient ANDC-Yb3+ interaction in 2-Yb0.1ANDC1 since effectively all Yb3+ ions are directly connected to an ANDC sensitizer. On the other hand, the Yb3+ emission quantum yields and lifetimes are comparable for 2-Yb1ANDC1 and 2-Yb0.1ANDC1 MOFs (Table 2) indicating that there is no significant Yb–Yb quenching pathway.
Table 2 Ln3+-centred luminescence quantum yields (ΦLLn) and observed luminescence lifetimes (τobs) of the studied Ln-MOFs in the solid statea
Ln-MOF |
Φ
LLn(10−2%)b |
τ
obs (μs)c |
Data obtained at room temperature. Standard deviation (2σ) is between parentheses; estimated relative errors: τobs, ±2%; ΦLLn, ±10%.
Under excitation at 400–410 nm.
Under excitation at 355 nm.
|
1-NdNDC |
13.03(6)c |
1.035(4) |
1-Nd1ANDC0.01 |
12.2(4)c |
1.032(3) |
1-Nd1ANDC0.02 |
10.5(1)c |
1.06(1) |
1-Nd1ANDC0.05 |
9.52(7) |
1.08(1) |
1-Nd1ANDC0.1 |
10(7) |
1.072(8) |
1-Nd1ANDC0.2 |
10.8(1) |
1.03(1) |
2-Nd1ANDC |
11.5(2) |
0.96(1) |
2-Nd1ANDC0.45 |
17.3(2) |
0.957(6) |
2-Nd1ANDC0.1 |
8.1(2) |
1.018(5) |
2-Nd1DANDC |
7.2(5) |
0.81(4) |
1-Yb0.005ANDC0.2 |
0.10(1) |
N/A |
1-Yb0.01ANDC0.2 |
0.116(9) |
1.349(8) |
1-Yb0.02ANDC0.2 |
0.24(1) |
14.9(1) |
1-Yb0.05ANDC0.2 |
0.58(2) |
14.3(1) |
1-Yb0.1ANDC0.2 |
1.56(2) |
14.8(4) |
1-Yb0.15ANDC0.2 |
2.46(5) |
15.63(6) |
1-Yb0.2ANDC0.2 |
5.41(5) |
15.09(8) |
1-Yb0.3ANDC0.2 |
0.880(1) |
14.4(1) |
1-Yb0.5ANDC0.2 |
1.93(2) |
12.1(4) |
2-Yb0.1ANDC1 |
13.6(3) |
11.35(2) |
2-Yb1ANDC1 |
14.7(3) |
9.68(2) |
2-YbDANDC |
21.4(5) |
8.11(4) |
2-ErANDC |
0.73(4) |
1.68(2) |
2-ErDANDC |
0.48(5) |
1.27(1) |
In addition, for 2-Nd1ANDC1, 2-Nd1ANDC0.1 and 2-NdDANDC MOFs, the intrinsic quantum yields of the Nd3+ emission under direct excitation into its f–f transition at 750 nm (ΦLnLn) could be acquired and were found to be 0.307(7) %, 0.293(1) % and 0.201(1) %, respectively. Using the values of ΦLnLn and ΦLLn, we calculated the sensitization efficiencies of Nd3+ (ηsens) according to the formula:
We found that the values of ηsens are similar for 2-Nd1ANDC1 and 2-NdDANDC (38 ± 8% vs. 35 ± 5%) and slightly lower for 2-NdANDC0.1 (27 ± 1%).
Experimental luminescence decay curves of Ln-MOFs (Ln = Yb3+, Nd3+, Er3+) measured under excitation of the chromophoric sensitizers (λex = 355 nm) and upon monitoring the NIR emission signals arising from the main transitions of the corresponding Ln3+ (Nd3+: 4F3/2 → 4I11/2 at 1064 nm, Er3+: 4I13/2 → 4I15/2 at 1525 nm and Yb3+: 2F5/2 → 2F7/2 at 980 nm) are best fitted with a monoexponential function. Such behaviour indicates that Ln3+ ions are located in a single well-defined coordination environment within the MOF scaffold. The measured values of the observed luminescence lifetimes (τobs) are in the order of 1 μs for Nd3+ MOFs and range between 10 and 15 μs for the MOFs incorporating Yb3+ (Table 2). The similarity of the values of τobs for a particular Ln3+ within the studied series of MOFs suggests that they are insignificantly affected by the nature of the ligands and their content in Ln-MOFs. The values of τobs are comparable with the ones reported for similar NIR-emitting Ln-MOFs.63,64
Ligand-centred photophysical properties and the Ln3+ sensitization mechanism
To further investigate the energy transfer processes that take place between NDC, its amino- derivatives, and the Ln3+ ions within the Ln-MOF matrices, we conducted steady-state and time-gated luminescence analysis at a low temperature (77 K) of the 1-Nd1NDC, 1-LaANDC0.02 and 1-LaDANDC0.02 MOFs. The energy of an excited state is defined as the energy gap between the lowest vibrational level of the excited state and that of the corresponding ground state (the so-called 0–0 energy gap). Spectroscopically, this energy is quantified by identifying the 0–0 transition at low temperature as the highest-energy vibrational feature of the corresponding emission signal. Consequently, the energy of the lowest spin-allowed (S1) excited state is determined by deconvoluting the fluorescence spectrum measured at 77 K. Similarly, the energy of the long-lived lowest spin-forbidden state (T1) can be quantified from the corresponding phosphorescence spectrum which is measured in a time-gating mode by applying a delay of ca. 1 μs to remove short-lived fluorescence signals. Using the described method, we determined the energies of the ligands’ excited states by performing Gaussian deconvolution of the emission spectra of the mixed-ligand Ln-MOFs acquired at 77 K in the steady-state mode for the S1 excited states and in the time-gated mode for the T1 excited states (Fig. S11, ESI†).65 The energy diagram of Fig. 4 shows that the excited levels, including the long-lived T1 states of NDC, ANDC and DANDC, lie above the main emissive levels of Nd3+ (4F3/2), Er3+ (4I13/2) and Yb3+ (2F5/2), therefore, are in favourable energy positions to populate them.66
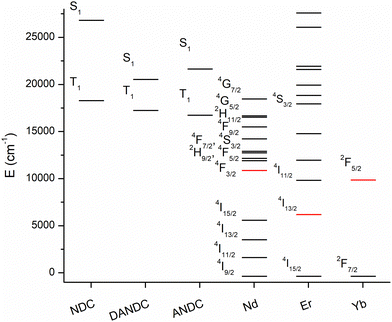 |
| Fig. 4 Energy diagram of the singlet (S1) and triplet (T1) excited states of the NDC, ANDC and DANC ligands and the electronic levels of the Ln3+ ions (red: emissive levels). | |
In general, the sensitization of the Ln3+ in the studied series of MOFs involves the excitation of the electronic states of the NDC chromophore, since it is a dominant one in the matrix, thus creating a ligand excited state (exciton). Then, this excited state migrates through the matrix by a series of energy transfer steps (exciton hoping) until it reaches an energy acceptor site (either an amino-substituted ligand, i.e. ANDC or DANDC, or a low-lying excited state of Ln3+), or it eventually decays through radiative or non-radiative (thermal) processes.67 To gain insight into the process of energy transfer from NDC to its amino derivatives, ANDC and DANDC, we measured and analysed the NDC-centred quantum yields (ΦL) of 1-LaANDCy and 1-LaDANDCy with low doping levels of amino-substituted derivatives (y = 0–0.002) under excitation at 330 nm (experimental details in the ESI† and results in Table S3). This process allowed the achievement of partial NDC-to-(D)ANDC energy transfer, which is readily quantifiable with our experimental setup, instead of the almost complete quenching of NDC-centred emission which is observed in Ln-MOFs with higher acceptor loadings. We plotted the ratios of the quantum yield values obtained for the 1-LaNDC MOF (ΦL0) over the ones acquired for 1-LaANDCy and 1-LaDANDCy (ΦL) MOFs against the molar fraction of ANDC and DANDC within the MOF in order to construct a Stern–Volmer type plot (Fig. 5).67 In both cases, the quantum yield of the NDC-centred emission decreases progressively upon addition of a quencher (ANDC or DANDC) due to the incorporation of more low-energy acceptor sites into the MOF. We found that the slopes observed in the Stern–Volmer plots for 1-LaANDCy and 1-LaDANDCy are in the same order of magnitude. The steeper slope for the latter possibly indicates that the energy transfer efficiency from the NDC ligand is more efficient for the DANDC ligands than for the ANDC ones.68,69
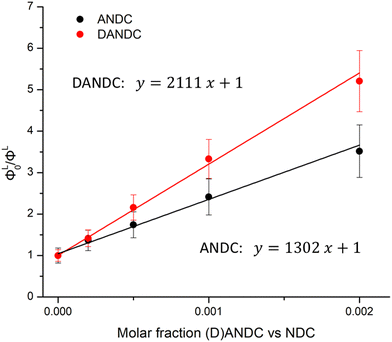 |
| Fig. 5 Stern–Volmer-type plots of ΦL0/ΦL for LaANDCy and LaDANDCy over the molar fractions of ANDC (red) and DANDC (black) (y = 0–0.002) in the MOF (R2 > 0.996). The values of the corresponding quantum yields are given in Table S3 (ESI†). | |
Conclusions
In summary, we have synthesized two new series of Ln-MOFs (Ln = La3+, Nd3+, Er3+, Yb3+, Lu3+) featuring H2NDC and its amino derivatives, H2ANDC and H2DANDC, with the general formulae [La1−xLnx(NDC)1−y(ANDC)yCl(DMF)] (Ln = Nd3+, Yb3+, x = 0.005–1, y = 0–0.2) (series 1) and [La1−xLnx((NDC)1−y(ANDC)y)1.5(DMF)2]·DMF (Ln = Nd3+, Er3+, Yb3+, Lu3+, x = 0.1 or 1, y = 0.45 or 1), [Ln(DANDC)1.5(DMF)2]·DMF (Ln = Nd3+, Er3+, Yb3+) (series 2). Series 1 comprises Ln-MOFs with an orthorhombic structure, while the Ln-MOFs of series 2 adopt a triclinic structure. We have demonstrated that series 1 retains its structural integrity upon incorporation of amino-substituted ligands for y ≤ 0.3, while for higher loadings formation of the triclinic phase is preferred. In addition, Ln-MOFs constructed using 100 mol% H2ANDC and H2DANDC ligands crystallize in the triclinic phase with small structural variations pertinent to the lanthanide(III) contraction.61 In particular, Nd3+ and Er3+ in the aforementioned Ln-MOFs are nine-coordinated while Yb3+ and Lu3+ are eight-coordinated. Thus, the secondary building units of the former Ln3+ consist of edge sharing [Ln2O16] dimers while Yb3+ and Lu3+ form two discrete carboxy-bridged coordination polyhedra. The photophysical analysis of the Ln-MOFs of series 1 revealed that each of the three chromophoric ligands studied in this work is efficient for the sensitization of three different lanthanide(III) cations emitting in the NIR domain, Nd3+, Yb3+ and Er3+. This is a major result as the chromophoric ligands that combine absorption in the visible region with the ability to sensitize NIR-emitting Ln3+ within a MOF scaffold remain scarce. In mixed-ligand Ln-MOFs, both NDC and ANDC contribute to the sensitization of NIR-emitting Ln3+. An increase of the ANDC content in these Ln-MOFs has a beneficial effect on the Nd3+ and Yb3+ emission quantum yields. The Nd3+ sensitization efficiencies of the series 2 Ln-MOFs were found to be similar for 2-Nd1ANDC1 and 2-NdDANDC, ranging within 35–38%. Overall, this work demonstrates that the use of amino-derivatives of 2,6-naphthalene dicarboxylic acid (H2NDC) in the construction of Ln-MOFs results in shifting the absorption wavelength to the visible region while achieving efficient sensitization of NIR-emitting Ln3+ ions.
Experimental
All experimental information (synthesis, characterization data, X-ray crystallography, instrumentation and software used) are provided in the ESI.†
Conflicts of interest
There are no conflicts to declare.
Acknowledgements
The research work in Greece was supported by the Hellenic Foundation for Research and Innovation (HFRI) under the 3rd Call for HFRI PhD Fellowships (Fellowship Number: 6322). The work in France was partially supported by the ‘Ligue Regionale Contre le Cancer’ (comités du Loiret, d’Eure-et-Loir, du Loir-et-Cher and de la Sarthe), the network ‘Molecules marines, metabolisme et cancer’ from the Canceropôle Grand Ouest. S. P. acknowledges support from the Institut National de la Santé et de la Recherche Medicale (Inserm).
Notes and references
- J.-C. G. Bünzli and C. Piguet, Chem. Soc. Rev., 2005, 34, 1048–1077 RSC.
- S. V. Eliseeva and J.-C. G. Bünzli, Chem. Soc. Rev., 2010, 39, 189–227 RSC.
- A. Mech, A. Monguzzi, F. Meinardi, J. Mezyk, G. MacChi and R. Tubino, J. Am. Chem. Soc., 2010, 132, 4574–4576 CrossRef CAS PubMed.
- M. D. Ward, J. Solid State Electrochem., 2005, 9, 778–787 CrossRef CAS.
- G. T. Dempsey, J. C. Vaughan, K. H. Chen, M. Bates and X. Zhuang, Nat. Methods, 2011, 8, 1027–1036 CrossRef CAS PubMed.
- V. Ntziachristos, C. Bremer and R. Weissleder, Eur. Radiol., 2003, 13, 195–208 CrossRef PubMed.
- G. T. Dempsey, J. C. Vaughan, K. H. Chen, M. Bates and X. Zhuang, Nat. Methods, 2011, 8, 1027–1036 CrossRef CAS PubMed.
-
R. Rigler, M. Orrit and T. Basché, Single molecule spectroscopy: Nobel conference lectures, Springer Science & Business Media, 2012, vol. 67 Search PubMed.
- Z. Yang, A. Sharma, J. Qi, X. Peng, D. Y. Lee, R. Hu, D. Lin, J. Qu and J. S. Kim, Chem. Soc. Rev., 2016, 45, 4651–4667 RSC.
- B. Hötzer, I. L. Medintz and N. Hildebrandt, Small, 2012, 8, 2297–2326 CrossRef PubMed.
- G. D. Luker and K. E. Luker, J. Nucl. Med., 2008, 49, 1–4 CrossRef PubMed.
- C. Ding and T. Ren, Coord. Chem. Rev., 2023, 482, 215080 CrossRef CAS.
- R. Weissleder and V. Ntziachristos, Nat. Med., 2003, 9, 123–128 CrossRef CAS PubMed.
- X. Dang, N. M. Bardhan, J. Qi, L. Gu, N. A. Eze, C.-W. Lin, S. Kataria, P. T. Hammond and A. M. Belcher, Sci. Rep., 2019, 9, 3873 CrossRef PubMed.
-
J. R. Lakowicz, Principles of Fluorescence Spectroscopy, Springer, US, 2007 Search PubMed.
- J. Mu, M. Xiao, Y. Shi, X. Geng, H. Li, Y. Yin and X. Chen, Angew. Chem., Int. Ed., 2022, 61, e202114722 CrossRef CAS PubMed.
- A. L. Antaris, H. Chen, K. Cheng, Y. Sun, G. Hong, C. Qu, S. Diao, Z. Deng, X. Hu, B. Zhang, X. Zhang, O. K. Yaghi, Z. R. Alamparambil, X. Hong, Z. Cheng and H. Dai, Nat. Mater., 2016, 15, 235–242 CrossRef CAS PubMed.
- J.-C. G. Bünzli, Coord. Chem. Rev., 2015, 293–294, 19–47 CrossRef.
- E. Baggaley, J. A. Weinstein and J. A. G. Williams, Coord. Chem. Rev., 2012, 256, 1762–1785 CrossRef CAS.
- A. D’Aléo, A. Bourdolle, S. Brustlein, T. Fauquier, A. Grichine, A. Duperray, P. L. Baldeck, C. Andraud, S. Brasselet and O. Maury, Angew. Chem., Int. Ed., 2012, 51, 6622–6625 CrossRef PubMed.
- G.-Q. Jin, Y. Ning, J.-X. Geng, Z.-F. Jiang, Y. Wang and J.-L. Zhang, Inorg. Chem. Front., 2020, 7, 289–299 RSC.
- C. Li, Y. Pang, Y. Xu, M. Lu, L. Tu, Q. Li, A. Sharma, Z. Guo, X. Li and Y. Sun, Chem. Soc. Rev., 2023, 52, 4392–4442 RSC.
- M. D. Ward, Coord. Chem. Rev., 2010, 254, 2634–2642 CrossRef CAS.
- A. S. Hyre and L. H. Doerrer, Coord. Chem. Rev., 2020, 404, 213098 CrossRef CAS.
- T. M. George, S. Varughese and M. L. P. Reddy, RSC Adv., 2016, 6, 69509–69520 RSC.
- G. A. Hebbink, D. N. Reinhoudt and F. C. J. M. van Veggel, Eur. J. Org. Chem., 2001, 4101–4106 CrossRef CAS.
- G.-Q. Jin, D. Sun, X. Xia, Z.-F. Jiang, B. Cheng, Y. Ning, F. Wang, Y. Zhao, X. Chen and J.-L. Zhang, Angew. Chem., Int. Ed., 2022, 61, e202208707 CrossRef CAS PubMed.
- S. V. Eliseeva, T. N. Nguyen, J. W. Kampf, E. R. Trivedi, V. L. Pecoraro and S. Petoud, Chem. Sci., 2022, 13, 2919–2931 RSC.
- I. Martinić, S. V. Eliseeva, T. N. Nguyen, V. L. Pecoraro and S. Petoud, J. Am. Chem. Soc., 2017, 139, 8388–8391 CrossRef PubMed.
- T. N. Nguyen, S. V. Eliseeva, I. Martinić, P. L. Carver, T. Lathion, S. Petoud and V. L. Pecoraro, Chem. – Eur. J., 2023, 29, e202300226 CrossRef CAS PubMed.
- C. M. Andolina, P. J. Klemm, W. C. I. I. I. Floyd, J. M. J. Fréchet and K. N. Raymond, Macromolecules, 2012, 45, 8982–8990 CrossRef CAS PubMed.
- S. Plunkett, M. El Khatib, İ. Şencan, J. E. Porter, A. T. N. Kumar, J. E. Collins, S. Sakadžić and S. A. Vinogradov, Nanoscale, 2020, 12, 2657–2672 RSC.
- D. Kovacs, X. Lu, L. S. Mészáros, M. Ott, J. Andres and K. E. Borbas, J. Am. Chem. Soc., 2017, 139, 5756–5767 CrossRef CAS PubMed.
- A.-M. Caminade, A. Hameau, C.-O. Turrin, R. Laurent and J.-P. Majoral, Coord. Chem. Rev., 2021, 430, 213739 CrossRef CAS.
- M. D. Allendorf, C. A. Bauer, R. K. Bhakta and R. J. T. Houk, Chem. Soc. Rev., 2009, 38, 1330–1352 RSC.
- A. Foucault-Collet, K. A. Gogick, K. A. White, S. Villette, A. Pallier, G. Collet, C. Kieda, T. Li, S. J. Geib, N. L. Rosi and S. Petoud, Proc. Natl. Acad. Sci. U. S. A., 2013, 110, 17199–17204 CrossRef CAS PubMed.
- J. Rocha, L. D. Carlos, F. A. A. Paz and D. Ananias, Chem. Soc. Rev., 2011, 40, 926–940 RSC.
- H. Furukawa, K. E. Cordova, M. O’Keeffe and O. M. Yaghi, Science, 2013, 341, 1230444 CrossRef PubMed.
- H. Xu, M. Fang, C.-S. Cao, W.-Z. Qiao and B. Zhao, Inorg. Chem., 2016, 55, 4790–4794 CrossRef CAS PubMed.
- J. A. Smith, M. A. Singh-Wilmot, K. P. Carter, C. L. Cahill and J. A. Ridenour, Cryst. Growth Des., 2019, 19, 305–319 CrossRef CAS.
- G. Ji, J. Liu, X. Gao, W. Sun, J. Wang, S. Zhao and Z. Liu, J. Mater. Chem. A, 2017, 5, 10200–10205 RSC.
- T. W. Chamberlain, R. V. Perrella, T. M. Oliveira, P. C. de Sousa Filho and R. I. Walton, Chem. – Eur. J., 2022, 28, e202200410 CrossRef CAS PubMed.
- G. E. Gomez, R. Marin, A. N. Carneiro Neto, A. M. P. Botas, J. Ovens, A. A. Kitos, M. C. Bernini, L. D. Carlos, G. J. A. A. Soler-Illia and M. Murugesu, Chem. Mater., 2020, 32, 7458–7468 CrossRef CAS.
- A. Kourtellaris, W. Lafargue-Dit-Hauret, F. Massuyeau, C. Latouche, A. J. Tasiopoulos and H. Serier-Brault, Adv. Opt. Mater., 2022, 10, 2200484 CrossRef CAS.
- D. Andriotou, S. A. Diamantis, A. Zacharia, G. Itskos, N. Panagiotou, A. J. Tasiopoulos and T. Lazarides, Molecules, 2020, 25, 523 CrossRef CAS PubMed.
- T. Feng, Y. Ye, X. Liu, H. Cui, Z. Li, Y. Zhang, B. Liang, H. Li and B. Chen, Angew. Chem., Int. Ed., 2020, 59, 21752–21757 CrossRef CAS PubMed.
- O. Guillou, C. Daiguebonne, G. Calvez and K. Bernot, Acc. Chem. Res., 2016, 49, 844–856 CrossRef CAS PubMed.
- K. Pei, J. Wu, M. Zhao, X. Feng, Y. Li, Y. Ma, H. Li and T. Zhai, Adv. Opt. Mater., 2022, 10, 2102143 CrossRef CAS.
- X. Yu, A. A. Ryadun, D. I. Pavlov, T. Y. Guselnikova, A. S. Potapov and V. P. Fedin, Angew. Chem., Int. Ed., 2023, 62, e202306680 CrossRef CAS PubMed.
- M. Pan, Y.-X. Zhu, K. Wu, L. Chen, Y.-J. Hou, S.-Y. Yin, H.-P. Wang, Y.-N. Fan and C.-Y. Su, Angew. Chem., Int. Ed., 2017, 56, 14582–14586 CrossRef CAS PubMed.
- D. Zhang, W. Zhou, Q. Liu and Z. Xia, ACS Appl. Mater. Interfaces, 2018, 10, 27875–27884 CrossRef CAS PubMed.
- L. L. da Luz, R. Milani, J. F. Felix, I. R. B. Ribeiro, M. Talhavini, B. A. D. Neto, J. Chojnacki, M. O. Rodrigues and S. A. Júnior, ACS Appl. Mater. Interfaces, 2015, 7, 27115–27123 CrossRef CAS PubMed.
- Z. Gao, B. Xu, T. Zhang, Z. Liu, W. Zhang, X. Sun, Y. Liu, X. Wang, Z. Wang, Y. Yan, F. Hu, X. Meng and Y. S. Zhao, Angew. Chem., Int. Ed., 2020, 59, 19060–19064 CrossRef CAS PubMed.
- Y. Wang, H. Li, X. He and Z. Xu, Inorg. Chem., 2021, 60, 15001–15009 CrossRef CAS PubMed.
- R. Cui, W. Sun, M. Liu, J. Shi and Z. Liu, ACS Appl. Mater. Interfaces, 2021, 13, 59164–59173 CrossRef CAS PubMed.
- D. F. Sava Gallis, L. E. S. Rohwer, M. A. Rodriguez, M. C. Barnhart-Dailey, K. S. Butler, T. S. Luk, J. A. Timlin and K. W. Chapman, ACS Appl. Mater. Interfaces, 2017, 9, 22268–22277 CrossRef CAS PubMed.
- X. Liu, R. Li, X. Xu, Y. Jiang, W. Zhu, Y. Yao, F. Li, X. Tao, S. Liu, W. Huang and Q. Zhao, Adv. Mater., 2023, 35, 2206741 CrossRef CAS PubMed.
- W. J. Rieter, K. M. L. Taylor and W. Lin, J. Am. Chem. Soc., 2007, 129, 9852–9853 CrossRef CAS PubMed.
- W. J. Rieter, K. M. L. Taylor, H. An, W. Lin and W. Lin, J. Am. Chem. Soc., 2006, 128, 9024–9025 CrossRef CAS PubMed.
- A. E. Psalti, D. Andriotou, S. A. Diamantis, A. Chatz-Giachia, A. Pournara, M. J. Manos, A. Hatzidimitriou and T. Lazarides, Inorg. Chem., 2022, 61, 11959–11972 CrossRef CAS PubMed.
- M. Seitz, A. G. Oliver and K. N. Raymond, J. Am. Chem. Soc., 2007, 129, 11153–11160 CrossRef CAS PubMed.
- S. A. Diamantis, A. Hatzidimitriou, A. K. Plessas, A. Pournara, M. J. Manos, G. S. Papaefstathiou and T. Lazarides, Dalton Trans., 2020, 49, 16736–16744 RSC.
- T. N. Nguyen, G. Capano, A. Gładysiak, F. M. Ebrahim, S. V. Eliseeva, A. Chidambaram, B. Valizadeh, S. Petoud, B. Smit and K. C. Stylianou, Chem. Commun., 2018, 54, 6816–6819 RSC.
- T. N. Nguyen, S. V. Eliseeva, A. Gładysiak, S. Petoud and K. C. Stylianou, J. Mater. Chem. A, 2020, 8, 10188–10192 RSC.
-
V. Balzani, P. Ceroni and A. Juris, Photochemistry and Photophysics: Concepts, Research, Applications, Wiley, 2014 Search PubMed.
- M. Latva, H. Takalo, V.-M. Mukkala, C. Matachescu, J. C. Rodríguez-Ubis and J. Kankare, J. Lumin., 1997, 75, 149–169 CrossRef CAS.
- S. W. Thomas, G. D. Joly and T. M. Swager, Chem. Rev., 2007, 107, 1339–1386 CrossRef CAS PubMed.
- H.-J. Son, S. Jin, S. Patwardhan, S. J. Wezenberg, N. C. Jeong, M. So, C. E. Wilmer, A. A. Sarjeant, G. C. Schatz, R. Q. Snurr, O. K. Farha, G. P. Wiederrecht and J. T. Hupp, J. Am. Chem. Soc., 2013, 135, 862–869 CrossRef CAS PubMed.
- C. A. Kent, D. Liu, L. Ma, J. M. Papanikolas, T. J. Meyer and W. Lin, J. Am. Chem. Soc., 2011, 133, 12940–12943 CrossRef CAS PubMed.
|
This journal is © The Royal Society of Chemistry 2024 |
Click here to see how this site uses Cookies. View our privacy policy here.