DOI:
10.1039/C6RA01717G
(Paper)
RSC Adv., 2016,
6, 28047-28054
A new triazine functionalized luminescent covalent organic framework for nitroaromatic sensing and CO2 storage†
Received
20th January 2016
, Accepted 3rd March 2016
First published on 4th March 2016
Abstract
A new hexagonally ordered covalent organic framework (COF), TRIPTA has been synthesized using Schiff base condensation reaction between 1,3,5-tris-(4-aminophenyl)triazine (TAPT) and 1,3,5-triformylphloroglucinol (TFP). TRIPTA exhibits high crystallinity, a large BET surface area (609 m2 g−1) and pore volume (0.351 cm3 g−1) and possesses high nitrogen content (14.97%). TRIPTA was found to be highly luminescent when suspended in polar solvents upon irradiation of UV light and can detect various nitroaromatic compounds with good sensitivity by fluorescence quenching at concentrations as low as in the range of 10−8 M. The maximum fluorescence quenching was observed for trinitrophenol (61.7% at 5.46 × 10−7 M) with a Stern–Volmer constant of 2.7 × 106 M−1. The COF also showed excellent CO2 uptake capacity of 57.07 wt% at 273 K and 16.02 wt% at 298 K up to 5 bar pressure, with an initial heat of adsorption (Qst) value 56.77 kJ mol−1.
Introduction
Porous polymeric materials formed by strong covalent bonds following a reversible condensation strategy e.g. boronic ester formation,1 polymerization of aromatic nitrile,2 Schiff base condensation of aromatic amine and aromatic aldehyde/anhydride3 etc. are classified as covalent organic frameworks (COFs).4 Since their discovery in 2005 5 these COFs have gained much attention as they are highly crystalline, light weight materials possessing exceptionally high surface area with a definite pore size distribution pattern. These unique properties make them an emerging and promising candidate in various fields like gas storage,6 chemical sensing,7 charge transport carrier8 and for heterogeneous catalysis9 etc. Among these, chemical sensing of hazardous –NO2 containing high energy aromatic compounds such as 2,4,6-trinitrophenol (TNP)-commonly known as picric acid, 2,4,6-trinitrotoluene (TNT), dinitrophenol (DNP), dinitrotoluene (DNT) etc. is of prior importance, as these polynitro aromatic compounds (NACs) are highly explosive and toxic in nature, even present in extremely low concentrations.10 Several fluorescence based chemo-sensors, e.g. metal–organic-frameworks (MOF), polymers and hybrid materials are reported for this purpose, which works upon the simple principle of gradual change in fluorescence intensity of the sensor with the addition of analyte.10 But, surprisingly enough, till date, there are very few reports listed on the fluorescent property and sensing behavior of COFs11 and the ongoing research on covalent organic frameworks is mostly concentrated on improving their porosity and gas storage capacity.12 As these type of materials contain electron rich aromatic building blocks and extended π-conjugation they can be successfully employed as fluorescence based chemosensor.
In our previous report we have presented the first one-step synthesis of 1,3,5-tris-(4-aminophenyl)triazine (TAPT) by trimerization of 4-aminobenzonitrile using trifluoromethanesulfonic acid as catalyst.13 TAPT was successfully employed to design a new covalent organic framework TRITER-1 using Schiff base condensation reaction with terephthaldehyde.13 Banerjee et al. have previously reported a series of COFs, (TpPa),14 where the –OH groups underwent keto–enol tautomerization so that the H-bonded keto–enamine form of the material gives highly crystalline and stable framework. Using this concept, herein we report the synthesis of a new triazine functionalized covalent organic framework TRIPTA via the Schiff base condensation pathway between 1,3,5-tris-(4-aminophenyl)triazine (TAPT) and 1,3,5-triformylphloroglucinol (TFP) which is followed by an irreversible keto–enol tautomerization step, where the reversible condensation reaction helps to produce highly ordered framework and the final H-bonded keto–enamine form introduces high thermal and chemical stability. The fluorescence property of TRIPTA with various nitroaromatic analytes i.e. TNP, DNP, DNT and NP as well as its CO2 storage capacity has been examined thoroughly.
Experimental section
Chemicals
For the synthesis of 1,3,5-triformyl phloroglucinol (TFP), phloroglucinol (MW = 126.11) was obtained from Sigma Aldrich, India whereas hexamine (MW = 140.19), trifluoroacetic acid (MW = 114.02) and hydrochloric acid were produced by E-Merk, India. For the synthesis of 1,3,5-tris-(4-aminophenyl)triazine (TAPT), 4-aminobenzonitrile (MW = 118.14) and trifluoromethanesulfonic acid were purchased from Sigma Aldrich, India. Anhydrous N,N-dimethylformamide was obtained from Spectrochem, India and used without further purification. The nitroaromatic compounds, 2,4,6-trinitrophenol (MW = 229.1), 2,4-dinitrophenol (MW = 184.106), 2,4-dinitrotoluene (MW = 182.134) and 4-nitrophenol (MW = 139.11), for the sensing experiment were purchased from Sigma Aldrich, India. All other solvents used were of analytical grade produced by E-Merk and were distilled prior use.
Synthesis of covalent organic framework TRIPTA
1,3,5-Tris-(4-aminophenyl)triazine (TAPT) was synthesized by the trimerization reaction of 4-aminobenzonitrile using trifluoromethanesulfonic acid as catalyst following the procedure described in our previous report13 and the purified product was characterized by 1H and 13C NMR (ESI† section S1). The yield of TAPT was found to be 90.6 mol%. 1,3,5-Triformyl phloroglucinol (TFP) was synthesized via Duff formylation procedure using phloroglucinol and hexamine.15 The polymer TRIPTA was synthesized by Schiff base condensation reaction between TAPT and TFP (Scheme 1). For the synthesis 709 mg (2 mmol) of TAPT and 420 mg (2 mmol) TFP were taken in a flame-dried two-neck round bottom flask fitted with a reflux condenser under inert atmosphere. To the reaction flask 15 mL anhydrous DMF was added and the mixture was refluxed for 12 h. The final solid product obtained was filtered, washed several times with anhydrous DMF and ethanol to remove any starting material present. The material thus obtained was further purified by Soxhlet extraction using methanol as solvent for 48 h. The yield of the final COF was calculated to be 85.41 wt%.
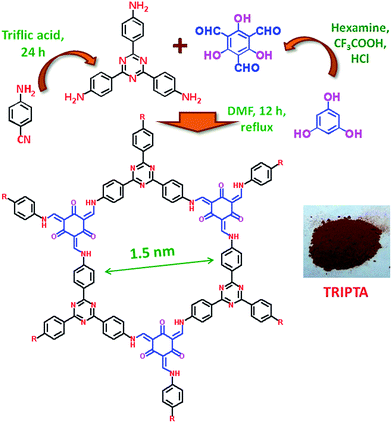 |
| Scheme 1 Schematic representation of synthesis of TRIPTA. | |
Sensing experiment
1 mg TRIPTA was suspended in 2 mL acetonitrile and sonicated in an ultrasonic bath for 10 min to get uniform dispersion of 0.5 mg mL−1. To the suspension increasing concentration [5.2 × 10−8 (M) to 5.46 × 10−7 (M)] of analytes (TNP, DNP, DNT and NP) were added and the fluorescence spectra were studied upon excitation at 365 nm.
Characterization technique
Powder X-ray diffraction pattern of the COF TRIPTA was obtained in a Bruker AXS D-8 Advanced SWAX diffractometer using Cu-Kα (0.15406 nm) radiation. N2 adsorption/desorption isotherm of the material was recorded using Quantachrome Autosorb 1C at 77 K. CO2 sorption isotherms of the material were recorded using a Bel Japan Inc. Belsorp-HP at 273 K and 298 K. The isosteric heat of adsorption was obtained using the software BELMaster™ version 5.3.3.0. Prior to all the adsorption study, the sample was degassed at 393 K for 12 h under high vacuum conditions. NLDFT pore-size distribution was obtained using N2 at 77 K carbon on the slit pore model of the Autosorb-1 software. 13C CP MAS NMR spectrum of TRIPTA was recorded using Bruker Advance 500 MHz NMR spectrometer using a 4 nm MAS probe under static condition (spinning rate 5000 Hz, with sideband suppression). High resolution transmission electron microscopic (HR-TEM) image of the material was obtained using a JEOL JEM 2010 transmission electron microscope operated at 200 kV. Sample was prepared by dropping a sonicated ethanol solution of the TRIPTA over a carbon-coated copper grid. Scanning electron microscopic image was obtained with a JEOL JEM 6700F field-emission scanning electron microscope (FE-SEM). FT-IR spectrum of TAPT, TFP and TRIPTA were recorded using a Nicolet MAGNA-FT IR 750 Spectrometer Series II. The UV-Visible diffuse reflectance spectrum of the solid sample was recorded in a UV 2401PC with an integrating sphere attachment. BaSO4 was used as the background standard. The 1H and 13C NMR spectra of the amine TAPT and the aldehyde TFP were recorded in a Bruker Advance 500 MHz NMR spectrometer. Mass spectrometric data of TAPT was acquired using the electron spray ionization (ESI) technique at 25–70 eV in a Micromass Q-tof-Micro Quadruple mass spectrophotometer. Thermogravimetric analysis (TGA and DTA) of the COF was carried out between the temperature ranges from 308 to 1073 K with a temperature ramp of 10 °C min−1 in a TGA instrument thermal analyzer TA-SDT Q-600 under N2 flow. Elemental analysis of the material was carried out using a Perkin Elmer 2400 Series II CHN analyzer. The fluorescence spectra were recorded using Horiba Jobin Yvon fluorimeter (slit width 5 nm, excitation wavelength 365 nm). Time-Correlated Single Photon Counting (TCSPC) measurements were performed using a Horiba Jobin Yvon IBH instrument attached with a MCP PMT Hamamatsu R3809 detector. NANO-LED source was used for excitation of samples at 375 nm. All data were fitted using Data Station v2.3 software.
Results and discussion
Ordered hexagonal phase and nanostructure
The PXRD spectrum (Fig. 1) of TRIPTA reveals highly crystalline nature of the COF material with periodic hexagonal framework possessing the first sharp peak at a 2θ value 5.8, which can be attributed to the 100 reflection plane. This peak is accompanied with two minor peaks at 2θ values 10.0 and 15.1 corresponding to the 110 and 210 reflection planes respectively. The broad peak at higher 2θ value (26.5) could be originated via π–π stalking between the 2D sheets and was designated as the 001 reflection plane.14a Using Bragg's equation (nλ = 2d
sin
θ, where n = 1 for the 100 plane and λ = 0.15406 nm) the average d spacing between the 100 planes (d100) was obtained to be 1.52 nm, whereas the inter-layer distance between the 2D sheets (001 plane) was calculated to be 3.36 Å. The experimental PXRD values were further matched with the theoretical data obtained using REFLEX software (unit cell parameters a = b = 17.55 Å, c = 3.36 Å, α = β = 90°, γ = 120°) and the result is summarized in Table 1. The energy minimized hexagonal structural unit of TRIPTA has been analyzed in section S2 of the ESI.†
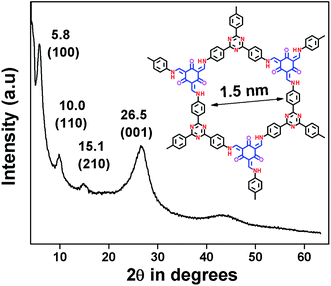 |
| Fig. 1 PXRD pattern of TRIPTA. | |
Table 1 Unit cell parameters and indexing of hexagonal planes of TRIPTAa
The experimental small angle PXRD has been matched with the theoretical PXRD pattern using REFLEX software. |
Symmetry |
Hexagonal |
Lattice parameters |
a = b = 17.55, c = 3.36, α = β = 90°, γ = 120° |
Wavelength (Å) |
1.54056 |
Range of data collection |
0.4 ≤ 2θ ≤ 40 |
h |
k |
l |
Theta (°) |
2 theta (°) |
d |
1 |
0 |
0 |
2.90502 |
5.81005 |
15.199 |
1 |
1 |
0 |
5.03597 |
10.0719 |
8.775 |
2 |
1 |
0 |
7.70589 |
15.4118 |
5.745 |
0 |
0 |
1 |
13.2529 |
26.5058 |
3.360 |
Porosity and BET surface area
N2 adsorption/desorption study at 77 K were performed to investigate the surface area and porosity of the material. The N2 adsorption/desorption isotherm of TRIPTA is shown in Fig. 2 which exhibits mainly the type I pattern associated with some features of type IV pattern. Large amount of N2 uptake in the initial pressure range (0–0.1 bar) suggests micropores being present in the framework. From the isotherm the Brunauer–Emmett–Teller (BET) surface area and pore volume of TRIPTA were found to be 609 m2 g−1 and 0.351 cm3 g−1. Nonlocal density functional theory (NLDFT) using N2 at 77 K, carbon on the slit pore model was employed on the N2 adsorption/desorption isotherm to investigate the materials pore size distribution pattern and the result suggests presence of uniform supermicropores of dimensions ca. 1.68 nm (inset of Fig. 2). The HR-TEM image (ESI, Fig. S1†) also shows micropores arranged throughout the matrix. The external morphology of TRIPTA was studied employing FE-SEM analysis which showed aggregation of small crystalline particles (ESI, Fig. S2†).
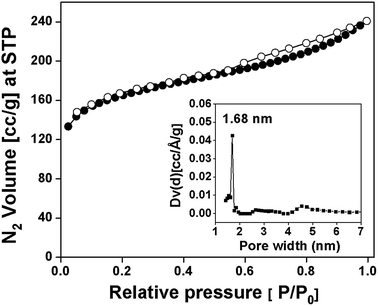 |
| Fig. 2 N2 adsorption/desorption isotherms of TRIPTA at 77 K. Pore size distribution is shown in the inset. | |
Framework and bonding
The FT-IR spectra (Fig. 3) for TRIPTA shows a distinct peak at 1620 cm−1 for carbonyl group (–C
O) associated with a sharp peak at 1576 cm−1 arising due to the –C
C– stretching. The absorption peak at 1255 cm−1 originates from the –C–N– stretching vibration. These peaks indicates that the –OH group of the phloroglucinol entity is present in the keto form and the imine bond formed via Schiff base condensation reaction is tautomerized to give enamine group. This phenomenon occurs as the keto form is stabilized by the strong hydrogen bonding between the –NH and the carbonyl carbon. Also the absence of the absorption band for C
N stretching at 1700 cm−1 and 1202 cm−1 supports the existence of keto–enamine form.14 Two strong absorption peak at 1503 cm−1 and 1360 cm−1 (aromatic –C
N–) indicates the presence of the triazine ring in both the spectra of TAPT and TRIPTA. Further the aromatic –C
C– stretching frequency of the benzene ring appears at 1450 cm−1. The absorption peak for carbonyl stretching of TFP comes at 1641 cm−1.
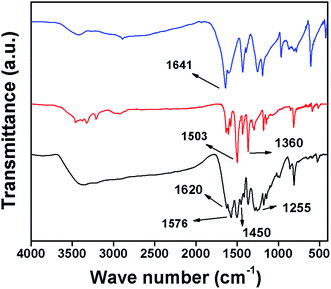 |
| Fig. 3 FT-IR spectra of TFP (blue), TAPT (red) and TRIPTA (black). | |
Solid state NMR and chemical environment
13C cross polarization magic angle spinning NMR spectroscopy was used to find out the chemical environment of each type of carbon atom present in the framework. As seen in Fig. 4 the 13C CP MAS NMR spectra of TRIPTA displays resonance signals at 185, 172, 151, 145, 134, 124, 118 and 110 ppm corresponding to different types of carbon atoms indicated in the structure in inset of Fig. 4. The signal at 185 ppm originates from the carbonyl carbon whereas the peak at 172 ppm confirms the formation of the triazine ring. The signal at 145 ppm can be assigned as the enamine carbon atom. The benzene ring carbon atom adjacent to the –NH group appears at 151 ppm and that closer to the triazine ring comes at 124 ppm. The other two types of aromatic carbon atoms of the benzene rings resonance at 134 and 118 ppm and the remaining sp2 carbons of the phloroglucinol moiety appears at 110 ppm. Also the absence of peak at 192 ppm indicates complete consumption of the starting aldehyde. Thus the 13C CP MAS NMR spectra of TRIPTA confirm the existence of keto–enamine form.
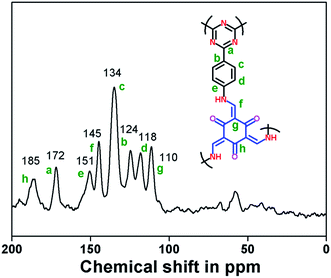 |
| Fig. 4 13C CP MAS NMR spectra of the polymer TRIPTA. | |
Thermogravimetric and elemental analysis
The TGA and DTA profile of TRIPTA is shown in ESI, Fig. S3,† indicating high thermal stability of the material. The TGA plot indicates that the material starts decomposing after 350 °C giving the major weight loss at around 393 °C as seen from the DTA profile. The elemental analysis of the COF provides the C, H and N content in the framework as C = 63.93%, H = 4.05% and N = 14.97%. These values closely matches with the theoretically calculated values obtained from the molar ratio of the starting reactant monomers (ESI, Table S2†).
Photophysical property
Fig. 5 represented the UV-Vis diffuse reflectance spectrum of TRIPTA, which showed absorbance maxima at 408 nm arising due to the π → π* transition of the heteroatom substituted conjugated system. The band gap energy obtained from the spectrum was 2.0 eV as shown in the inset of Fig. 5. Interestingly TRIPTA showed highly fluorescent green color when suspended in different polar aprotic solvents (ESI, Fig. S4†), which can be attributed to the extended π-conjugation and acceptor–donor charge transfer in the framework.16 ESI, Fig. S5† represented the fluorescence spectra of TRIPTA upon excitation at 365 nm in solvents of varying polarity. The spectra suggested that the fluorescence intensity increases with increasing polarity of aprotic solvents, whereas in case of polar protic solvents (water, methanol and ethanol) and non-polar solvents (cyclohexane and toluene) the COF shows no significant fluorescence property. The solid state fluorescence spectrum of the COF (λex = 325 nm) is shown in ESI, Fig. S6,† where a broad emission maxima at 608 nm is observed. The fluorescence spectrum (Fig. 6, black line) of the acetonitrile suspension of TRIPTA exhibited a strong emission band with emission maxima at 513 nm upon excitation at 365 nm corresponding to the chromophoric triphenyl triazine moiety. It was observed that the fluorescence intensity is effectively quenched upon addition of various nitroaromatic analytes. The fluorescence quenching profile of TRIPTA upon the addition of increasing concentration of 2,4,6-trinitrophenol (TNP) from 5.2 × 10−8 M to 5.46 × 10−7 M is shown in Fig. 6. The UV-Vis diffuse reflectance spectrum of acetonitrile suspension of TRIPTA in presence of TNP is shown in ESI, Fig. S7.† The effect of addition of other nitroaromatic compound i.e. 2,4-dinitrophenol (DNP), 2,4-dinitrotoluene (DNT) and 4-nitrophenol (NP) on the fluorescence intensity of TRIPTA and the corresponding S–V plots are shown in Fig. 7 and 8. The quenching constant of the sensor was evaluated analyzing the Stern–Volmer (S–V) plots following the equation I0/I = 1 + KSV[M]. From the plots the highest quenching constant (KSV) value was obtained for TNP to be 2.7 × 106 M−1. The KSV values follow the order TNP > DNP > DNT > NP. Fig. 9 showed a comparative bar diagram of the quenching efficiencies [I0 − I]/I0 of TRIPTA for different nitroaromatic analytes at a concentration 5.46 × 10−7 M, where the quenching efficiency value for TNP is 61.7%. Further the Time Correlated Single Photon Counting (TCSPC) studies of acetonitrile suspension of TRIPTA in presence of various nitroaromatic analytes were performed to measure the change in average fluorescence lifetime of the COF on addition of the analyte solutions. TRIPTA exhibits a triexponential decay profile with an average lifetime value 1.49 ns upon excitation at 375 nm and emission at 513 nm (Fig. 10). The average lifetime remains almost unchanged (1.59 ns) after addition of TNP (5.46 × 10−7 M), which indicate static quenching via the formation of a ground state complex. On the other hand, the average lifetime value of TRIPTA on addition of DNP, DNT and NP decreases to 0.49, 0.36 and 0.35 ns respectively, indicating that dynamic quenching mechanism is involved in these cases. ESI, Table S3† represents the details of TCSPC measurements.7b
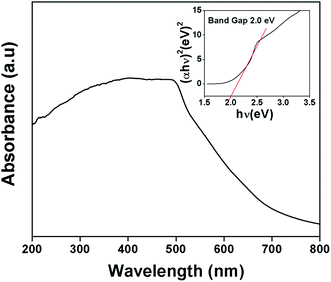 |
| Fig. 5 Solid state UV-Vis absorbance spectrum of TRIPTA (bandgap of the material is shown in inset). | |
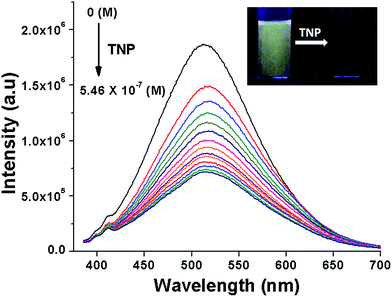 |
| Fig. 6 Photoluminescence spectra of acetonitrile suspension of TRIPTA upon addition of increasing concentration [5.2 × 10−8 (M) to 5.46 × 10−7 (M)] of 2,4,6-trinitrophenol (λex = 365 nm). Photograph of TNP sensing under UV light is shown in inset. | |
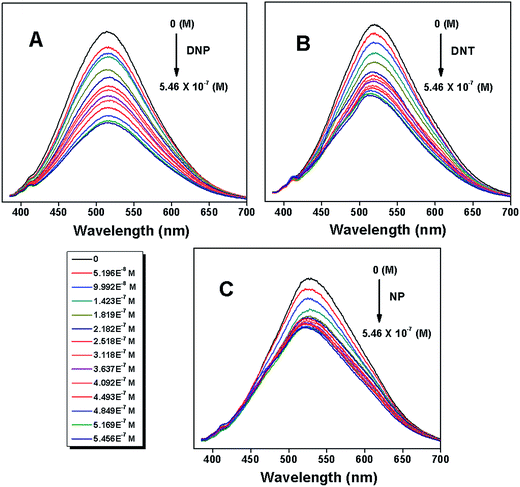 |
| Fig. 7 Photoluminescence spectra of acetonitrile suspension of TRIPTA upon addition of increasing concentration [5.2 × 10−8 (M) to 5.46 × 10−7 (M)] of (A) 2,4-dinitrophenol, (B) 2,4-dinitrotoluene and (C) 4-nitrophenol (λex = 365 nm). | |
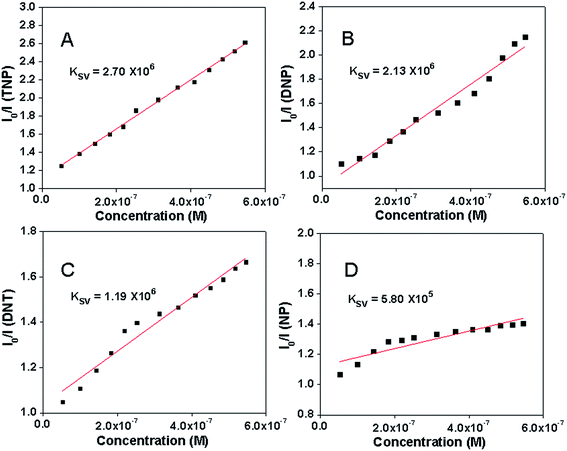 |
| Fig. 8 Stern–Volmer plots for fluorescence quenching of TRIPTA by (A) 2,4,6-trinitrophenol, (B) 2,4-dinitrophenol, (C) 2,4-dinitrotoluene and (D) 4-nitrophenol. | |
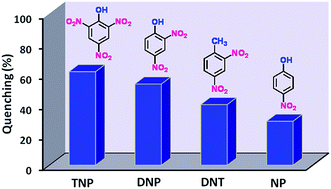 |
| Fig. 9 Fluorescence quenching efficiency of TRIPTA towards various nitroaromatic compounds at concentration 5.46 × 10−7 (M). | |
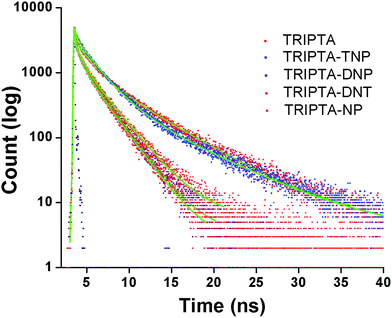 |
| Fig. 10 TCSPC average fluorescence lifetime decay profile of acetonitrile suspension of TRIPTA in presence of different nitroaromatic compounds (λex = 375 nm and λem = 513 nm). | |
CO2 adsorption study
The CO2 uptake experiment for the COF TRIPTA was conducted at two different temperatures (273 K and 298 K) up to 5 bar pressure of the adsorbate and the adsorption/desorption isotherms at these temperatures are presented in Fig. 11. A gradually increasing uptake was observed in the amount of CO2 adsorbed with increasing pressure, the value reaching a maximum of 12.97 mmol g−1 (57.07 wt%) and 3.64 mmol g−1 (16.02 wt%) at 273 K and 298 K respectively, at 5 bar pressure. Although the rate of CO2 adsorption is relatively slower in the high pressure region but the isotherms does not reach any saturation value, indicating that the material can adsorb some more CO2 if the pressure is further increased. Moreover, the desorption plot followed a fully reversible pathway, which signifies that the adsorbed gas can be completely recovered from the adsorbent solid. Fig. 12 represents the isosteric heat of adsorption (Qst) for TRIPTA as a function of volume of adsorbed CO2, as obtained using Clausius–Clapeyron equation (ln
P1/P2 = ΔH/R [1/T2 − 1/T1], at constant V) on the adsorption isotherms at 273 K and 298 K. The Qst value for the 1st point was found to be 56.77 kJ mol−1, which then decreases and becomes almost stable at ∼30–35 kJ mol−1. Such high initial Qst value reflects strong interaction between the adsorbate gas molecules and the material surface, although the value is considerably lesser than the energy of chemical bond so that reversible physisorption is feasible. The CO2 adsorption experiment was repeated four times at both 273 K and 298 K to check the reusability of the material and the results are summarized in the histogram of ESI, Fig. S8.† The CO2 uptake value of TRIPTA was reduced only by 1.34 wt% at 273 K and 1.14 wt% at 298 K after fourth cycle. The presence of N-rich functionality (N-content 14.97%) in the framework of the material leads to stronger interaction with the Lewis acidic CO2 molecules.17 Moreover high BET surface area (609 m2 g−1), pore volume (0.351 cm3 g−1) and sufficient micropore content of the material also contribute for such high CO2 uptake behavior.
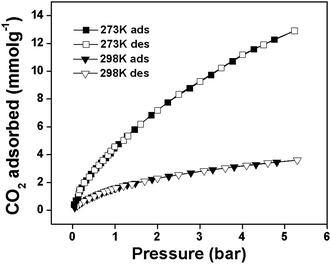 |
| Fig. 11 CO2 adsorption/desorption isotherm of TRIPTA at 273 K and 298 K. | |
 |
| Fig. 12 Isosteric heat of adsorption (Qst) of TRIPTA as a function of CO2 adsorbed. | |
Conclusions
In conclusion, we have successfully synthesized a new crystalline, high surface area covalent organic framework TRIPTA via Schiff base polycondensation of 1,3,5-tris-(4-aminophenyl)triazine (TAPT) and 1,3,5-triformylphloroglucinol (TFP). TRIPTA showed good luminescent property in various polar solvents when irradiated with UV light. The material was successfully employed for chemical sensing of nitro aromatic compounds at concentration as low as 5.196 × 10−8 M. A maximum fluorescence quenching was observed for TNP (61.7% at 5.46 × 10−7 M) with a Stern–Volmer constant of 2.7 × 106 M−1. TRIPTA also showed high CO2 uptake capacity of 57.07 wt% at 273 K and 16.02 wt% at 298 K up to 5 bar pressure with an initial Qst value 56.77 kJ mol−1 suggesting its future potential in sensing and environmental research.
Acknowledgements
RG wishes to thank CSIR, New Delhi for senior research fellowship. AB wishes to thank DST, New Delhi for instrumental facilities through DST Unit on Nanoscience and DST-SERB project grants.
References and Notes
-
(a) D. D. Medina, J. M. Rotter, Y. Hu, M. Dogru, V. Werner, F. Auras, J. T. Markiewicz, P. Knochel and T. Bein, J. Am. Chem. Soc., 2015, 137, 1016–1019 CrossRef CAS PubMed
;
(b) J. Plas, O. Ivasenko, N. Martsinovich, M. Lackinger and S. D. Feyter, Chem. Commun., 2016, 52, 68–71 RSC
;
(c) N. Huang, X. Ding, J. Kim, H. Ihee and D. Jiang, Angew. Chem., Int. Ed., 2015, 54, 8704–8707 CrossRef CAS PubMed
;
(d) A. P. Cote, H. M. El-Kaderi, H. Furukawa, J. R. Hunt and O. M. Yaghi, J. Am. Chem. Soc., 2007, 129, 12914–12915 CrossRef CAS PubMed
. -
(a) S. Hug, M. B. Mesch, H. Oh, N. Popp, M. Hirscher, J. Senkerd and B. V. Lotsch, J. Mater. Chem. A, 2014, 2, 5928–5936 RSC
;
(b) A. Bhunia, V. Vasylyeva and C. Janiak, Chem. Commun., 2013, 49, 3961–3963 RSC
;
(c) P. Kuhn, A. Thomas and M. Antonietti, Macromolecules, 2009, 42, 319–326 CrossRef CAS
. -
(a) F. J. Uribe-Romo, J. R. Hunt, H. Furukawa, C. Klock, M. O'Keeffe and O. M. Yaghi, J. Am. Chem. Soc., 2009, 131, 4570–4571 CrossRef CAS PubMed
;
(b) M. K. Bhunia, S. K. Das, P. Pachfule, R. Banerjee and A. Bhaumik, Dalton Trans., 2012, 41, 1304–1311 RSC
;
(c) G. Das, D. Balaji Shinde, S. Kandambeth, B. P. Biswal and R. Banerjee, Chem. Commun., 2014, 50, 12615–12618 RSC
. -
(a) P. J. Waller, F. Gandara and O. M. Yaghi, Acc. Chem. Res., 2015, 48, 3053–3063 CrossRef CAS PubMed
;
(b) J. Gao and D. Jiang, Chem. Commun., 2016, 52, 1498–1500 RSC
. - A. P. Cote, A. I. Benin, N. W. Ockwig, M. O'Keeffe, A. J. Matzger and O. M. Yaghi, Science, 2005, 310, 1166–1170 CrossRef CAS PubMed
. -
(a) H. Furukawa and O. M. Yaghi, J. Am. Chem. Soc., 2009, 131, 8875–8883 CrossRef CAS PubMed
;
(b) Z. Li, Y. Zhi, X. Feng, X. Ding, Y. Zou, X. Liu and Y. Mu, Chem.–Eur. J., 2015, 21, 12079–12084 CrossRef CAS PubMed
;
(c) S. Wu, S. Gu, A. Zhang, G. Yu, Z. Wang, J. Jianc and C. Pan, J. Mater. Chem. A, 2015, 3, 878–885 RSC
. -
(a) G. Das, B. P. Biswal, S. Kandambeth, V. Venkatesh, G. Kaur, M. Addicoat, T. Heine, S. Verma and R. Banerjee, Chem. Sci., 2015, 6, 3931–3939 RSC
;
(b) D. Kaleeswaran, P. Vishnoi and R. Murugavel, J. Mater. Chem. C, 2015, 3, 7159 RSC
. -
(a) Y. Chen, H. Cui, J. Zhang, K. Zhao, D. Ding, J. Guo, L. Li, Z. Tian and Z. Tang, RSC Adv., 2015, 5, 92573–92576 RSC
;
(b) S. L. Cai, Y. B. Zhang, A. B. Pun, B. He, J. Yang, F. M. Toma, I. D. Sharp, O. M. Yaghi, J. Fan, S. R. Zheng, W. G. Zhang and Y. Liu, Chem. Sci., 2014, 5, 4693–4700 RSC
;
(c) C. R. DeBlase, K. H. Burgos, K. E. Silberstein, G. G. R. Calero, R. P. Bisbey, H. D. Abruna and W. R. Dichtel, ACS Nano, 2015, 9, 3178–3183 CrossRef CAS PubMed
. -
(a) Y. Wu, H. Xu, X. Chen, J. Gao and D. Jiang, Chem. Commun., 2015, 51, 10096–10098 RSC
;
(b) L. Stegbauer, K. Schwinghammer and B. V. Lotsch, Chem. Sci., 2014, 5, 2789–2793 RSC
;
(c) P. Puthiaraja and K. Pitchumani, Green Chem., 2014, 16, 4223–4233 RSC
;
(d) V. S. Vyas, F. Haase, L. Stegbauer, G. Savasci, F. Podjaski, C. Ochsenfeld and B. V. Lotsch, Nat. Commun., 2015, 6, 8508, DOI:10.1038/ncomms9508
;
(e) W. Zhang, P. Jiang, Y. Wang, J. Zhang, Y. Gaoa and P. Zhang, RSC Adv., 2014, 4, 51544–51547 RSC
;
(f) S. Lin, Y. Hou, X. Deng, H. Wang, S. Sun and X. Zhang, RSC Adv., 2015, 5, 41017–41024 RSC
. -
(a) S. Shanmugaraju and P. S. Mukherjee, Chem. Commun., 2015, 51, 16014 RSC
;
(b) X. Liu, Y. Xu and D. Jiang, J. Am. Chem. Soc., 2012, 134, 8738–8741 CrossRef CAS PubMed
;
(c) C. Gu, N. Huang, Y. Wu, H. Xu and D. Jiang, Angew. Chem., Int. Ed., 2015, 54, 11540–11544 CrossRef CAS PubMed
;
(d) J. Liu, S. Yang, F. Li, L. Dong, J. Liu, X. Wang and Q. Pu, J. Mater. Chem. A, 2015, 3, 10069–10076 RSC
. -
(a) E. Ozdemir, D. Thirion and C. T. Yavuz, RSC Adv., 2015, 5, 69010–69015 RSC
;
(b) Y. F. Xie, S. Y. Ding, J. M. Liu, W. Wang and Q. Y. Zheng, J. Mater. Chem. C, 2015, 3, 10066 RSC
. -
(a) C. Gu, D. Liu, W. Huang, J. Liu and R. Yang, Polym. Chem., 2015, 6, 7410–7417 RSC
;
(b) Z. Li, Y. Zhi, X. Feng, X. Ding, Y. Zou, X. Liu and Y. Mu, Chem.–Eur. J., 2015, 21, 12079–12084 CrossRef CAS PubMed
;
(c) M. G. Rabbani, A. K. Sekizkardes, Z. Kahveci, T. E. Reich, R. Ding and H. M. El-Kaderi, Chem.–Eur. J., 2013, 19, 3324–3328 CrossRef CAS PubMed
. - R. Gomes, P. Bhanja and A. Bhaumik, Chem. Commun., 2015, 51, 10050–10053 RSC
. -
(a) S. Kandambeth, A. Mallick, B. Lukose, M. V. Mane, T. Heine and R. Banerjee, J. Am. Chem. Soc., 2012, 134, 19524–19527 CrossRef CAS PubMed
;
(b) S. Kandambeth, D. B. Shinde, M. K. Panda, B. Lukose, T. Heine and R. Banerjee, Angew. Chem., 2013, 125, 13290–13294 CrossRef
. -
(a) A. Modak, A. Kumar Barui, C. R. Patra and A. Bhaumik, Chem. Commun., 2013, 49, 7644–7646 RSC
;
(b) C. V. Yelamaggad, A. S. Achalkumar, D. S. S. Rao and S. K. Prasad, J. Org. Chem., 2009, 74, 3168 CrossRef CAS PubMed
. -
(a) L. A. Baldwin, J. W. Crowe, M. D. Shannon, C. P. Jaroniec and P. L. McGrier, Chem. Mater., 2015, 27, 6169–6172 CrossRef CAS
;
(b) K. Prasad, R. Haldar and T. K. Maji, RSC Adv., 2015, 5, 74986–74993 RSC
;
(c) D. Chandra, T. Yokoi, T. Tatsumi and A. Bhaumik, Chem. Mater., 2007, 19, 5347–5354 CrossRef CAS
. -
(a) B. Ashourirad, A. K. Sekizkardes, S. Altarawneh and H. M. El-Kaderi, Chem. Mater., 2015, 27, 1349 CrossRef CAS
;
(b) P. Arab, M. G. Rabbani, A. K. Sekizkardes, T. Islamoglu and H. M. El-Kaderi, Chem. Mater., 2014, 26, 1385 CrossRef CAS
;
(c) A. Modak, M. Pramanik, S. Inagaki and A. Bhaumik, J. Mater. Chem. A, 2014, 2, 11642 RSC
.
Footnote |
† Electronic supplementary information (ESI) available. See DOI: 10.1039/c6ra01717g |
|
This journal is © The Royal Society of Chemistry 2016 |
Click here to see how this site uses Cookies. View our privacy policy here.