DOI:
10.1039/C6RA05302E
(Paper)
RSC Adv., 2016,
6, 39955-39961
Fabrication of a heated electrode modified with a thiol-functionalized ionic liquid for electrochemical/electrochemiluminescence sensors†
Received
29th February 2016
, Accepted 9th April 2016
First published on 11th April 2016
Abstract
A new heated thiol-functionalized ionic liquid (TFIL)/Au composite electrode, which possessed the advantages of a heated electrode technique and ionic liquids (ILs), was designed and fabricated in this study. The electrode temperature (Te) could be accurately controlled by indirect electrical heating. Attributed to the stable Au–S bone and the very low vapor pressure of IL, the immobilized TFIL was stable on the electrode at high Te. The heated TFIL/Au electrode could be expediently used to study the electrochemical behavior of thermally unstable material at high temperature. The analytical performances of electrochemical (EC)/electrochemiluminescence (ECL) detection systems based on the heated TFIL/Au electrodes were evaluated by HSO3− and phenol, respectively. Higher electron-transfer rates, enhanced sensitivities, and improved reproducibilities were obtained compared with the bare Au electrode. The heated TFIL/Au composite electrode presented a promising platform for EC or ECL sensors.
1. Introduction
In the past few years, room temperature ionic liquids (RTILs or ILs), also called room temperature molten salts, have emerged as a frontier and novel area of research because of their excellent chemical and physical properties, such as high chemical and thermal stability, almost negligible vapor pressure, high conductivity and strong solubility.1 Being composed entirely of ions and possessing wide electrochemical windows, the ILs are regarded by electrochemists as attractive potential solvents. In electroanalytical chemistry, ILs have been used to prepare different kinds of composite electrodes, since they provide a remarkable increase in the electron transfer rate at interfaces, a significant decrease in the overpotential, and thus enhance the electrochemical responses in detection.2 The immobilizations of ILs on surface of electrode were via mechanical entrapment, electrostatic interaction, and chemical bone.3 The thiol-functionalized ionic liquid (TFIL), which has a strong bonding force to gold by Au–S, has been aroused much attention in immobilization of ILs on Au.4 Chi5 successfully synthesized a TFIL and developed a TFIL/Au electrode. The results showed that the sensitive and stable ECL response of luminol–O2 system was gained with constructed stable Au–IL|water interface, which was mainly attributed to the hydrophobic and conductive properties of the IL.
During the last two decades, the thermoelectrochemistry, which could be considered as one of the most important technologies, has received increasing research interest, with potential application in sensors, electrochemical determination and catalysts.6 Many methods of heating, such as laser beam7 and focused microwave radiation,8 could be used to elevate the electrode temperature (Te). But these methods required complex experimental setups, which limited their wide use in some analytical practices. Recently, the heated electrode has been developed,6 in which the electrode body itself could be subjected to direct or indirect electrical heating. By this technology, the temperature could be increased in a thin solution layer near electrode surface, while the temperature of the bulk solution was almost unchanged. Heated electrodes have been proven to provide many advantages, such as the simple equipment, enhanced sensitivity and minimized fouling effects, which offer new opportunities for electrochemical (EC)9 and electrochemiluminescent (ECL)10 detection. In previous works, the heated electrodes for sensors were often prepared with metal wire,11 graphite cylinder,12 indium tin oxide (ITO)13 and carbon paste.14 In our previous work, a heated IL/multi-wall carbon nanotube (MWCNT) composite electrode was developed for the ECL detection of ascorbic acid.15 However, it is difficult to modify ILs on the heated metal electrode because high temperature may cause the modified compounds to break off from the surface of the electrode. Fortunately, TFIL could solve this problem, which could be steadily fixed on the Au electrode.
In the present work, a heated TFIL/Au electrode was developed, which combined the heated electrode technology and IL modified electrode. TFIL, 1-methyl-3-(2′-mercaptoacetoxyethyl)imidazolium hexafluorophosphate ([MMIM]PF6−), was immobilized directly on the Au electrode by Au–S bond. And the proposed electrode could be indirectly heated by direct current (DC) to avoid interference from heating current on detecting current. The analytical performances of electrochemical/electrochemiluminescence detection systems based on heated TFIL/Au electrode were evaluated by HSO3− and phenol, respectively.
2. Experimental
2.1. Chemicals and materials
1-Methylimidazole, mercaptoacetic acid, and phenol were purchased from Sigma (St. Louis, MO, USA). Chloroacetic acid was obtained from Nanxiang Reagent Factory (Shanghai, China). Fluorescein was purchased from Fluka (Buchs, Switzerland). Other reagents and chemicals were all commercially available and of analytical reagent grade. The stock standard solution was stored in the refrigerator, and the working standard solutions were freshly prepared before analysis. All the water used was de-ionized water prepared with a Milli-Q water purification system (MA, USA).
2.2. Apparatus
A laboratory-built EC/ECL detection system was used in the experiment. A CHI 660D electrochemical system (CH Instruments, USA) was used for controlling waveforms and potentials. The ECL intensity was detected by a BPCL Ultra-Weak Luminescence Analyzer (Institute of Biophysics, Chinese Academy of Science, Beijing, China). The photomultiplier tube (PMT) was setup at −600 mV. The signals were collected and treated by a personal computer. The experiments were carried out using a three-electrode system with the bare Au electrode (1 mm in diameter) or heated TFIL/Au electrode (1 mm in diameter) as the working electrode, a platinum wire (99.99%) as counter electrode, and Ag/AgCl electrode in saturated KCl as the reference electrode. The ECL cell was placed directly at the top of the PMT and was fixed in a dark detection chamber. A DC power supply RXN-303A (Shengzhen Zhao Xin Electronic Equipments and Instruments Producer, Shengzhen, China) was connected to the Cu enameled wire to provide a steady current for heating electrode. The water contact angle (WCA) of bare Au electrode and TFIL/Au electrode was measured utilizing an OCA30 video optical contact angle meter (Dataphysics Instruments GmbH, DE).
2.3. Fabrication of the heated TFIL/Au electrode
The construction of heated Au-disk electrode is shown schematically in Fig. S1 (see ESI†). The “twin-wire-wound coil” was used as a winding coil method, which could cancel the magnetic field produced by the current passing through the Cu enameled wire.12
The heated Au-disk electrode was polished carefully with a 4000# silicon carbide abrasive paper and chamois leather to obtain a mirror surface and then was sonicated in ethanol and rinsed with Milli-Q water for 5 min, respectively. Before modifying, the electrode was subjected to repeated cycling in the potential range of −0.4 to 1.6 V (vs. Ag/AgCl) in 0.5 mol L−1 H2SO4 until a reproducible cyclic voltammogram as obtained. Finally, the electrode was dried in air after it was thoroughly rinsed with Milli-Q water.
The [MMIM]PF6− was prepared as Scheme S1 (see ESI†). Then, the treated electrode was immersed in a 1.0 × 10−3 mol L−1 [MMIM]PF6− acetonitrile solution for a certain time, such as 2 h for EC detection of HSO3− and 1 h for ECL detection of phenol, under 4 °C and darkness to form IL monolayer. Then, the modified electrode was dried by nitrogen stream to remove acetonitrile, and rinsed thoroughly with acetonitrile and Milli-Q water in turn to remove the physically adsorbed IL. The prepared electrode was kept in PBS (0.1 mol L−1, pH 7.4) at 4 °C until used.
2.4. Electrochemical impedance spectra (EIS) measurement
EIS were recorded by alternating current impedance measurements at amplitude of 5 mV in the frequency range from 100k to 0.1 Hz. The Nyquist plots for EIS measurements were obtained in phosphate buffer solution (Na2HPO4 + NaH2PO4, abbreviated to PBS, pH 7.0), containing 5.0 × 10−3 mol L−1 K3[Fe(CN)6]/K4[Fe(CN)6] and 0.1 mol L−1 KCl as the redox probe.
2.5. Procedure for EC measurement
The EC determination of HSO3− was performed by differential potential voltammetry (DPV) in PBS (0.1 mol L−1, pH 7.4). The analytical procedure involved the temperature-controlling process and DPV-obtaining process. Te was elevated to the desired temperature (such as 55 °C) by DC heating. The differential potential voltammogram was recorded with potentials ranging from 0.3 to 0.7 V after 60 s of heating time. After each measurement, a preconditioning step was used to remove the previous deposits. In this step, the working electrode was placed in PBS (0.1 mol L−1, pH 7.0). Then, Te was regulated to 70 °C, and the electrode was cleaned by cyclic voltammetry (CV) in the potential range between +0.7 and −0.5 V (vs. Ag/AgCl) for 20 successive cycles with 100 mV s−1. For wiping off the dissolved oxygen in the PBS solution, the high pure nitrogen was bubbled into the solution for 10 min before the addition of HSO3−, and nitrogen flow was maintained during the experiment. The room temperature was stabilized in 22 °C by air-conditioning.
2.6. Procedure for ECL measurement
Before each experiment, the heated IL/Au electrode was subjected to repeated cycling in the potential range of −1.3 to 0.5 V (vs. Ag/AgCl) in 0.2 mol L−1 PBS (pH 9.5) until a reproducible cyclic voltammogram was obtained. Similar to the EC detection procedure, the procedure also involved the temperature-controlling process and ECL-obtaining process. In ECL measurement of phenol, the working electrode was electrochemically heated and Te was elevated to 65 °C, while the temperature of bulk solution was kept at 22 °C. Then, the CV was preformed in the potential range of −1.2 to 0.5 V (vs. Ag/AgCl) with a scan rate of 100 mV s−1 to get the ECL signal. The determination of phenol was carried out in PBS (0.2 mol L−1, pH 9.5) containing 1 × 10−5 mol L−1 fluoresceinfluorescenin and 1 × 10−3 mol L−1 K2S2O8. The quantitative analysis was performed based on the net ECL intensity changes (ΔECL), ΔECL = ECL0 − ECLs, where ECL0 was the background signal (ECL intensity) of the fluorescein/K2S2O8 system without adding of sample, and ECLs was the signal after the addition of sample. The ΔECL was plotted against the corresponding concentration of the phenol and the calibration curve was constructed by quintuplicate analysis.
3. Results and discussion
3.1. Electrochemical impedance spectra (EIS) of the TFIL/Au electrode
The thiol-functionalized ionic liquid could be immobilized on the surface of Au electrode because of the strong bonding force between sulfhydryl group with Au, which could construct a stable Au–IL|water interface. The TFIL/Au electrode was further characterized by EIS and the semicircle portion observed at high frequencies in the Nyquist diagrams corresponded to the electron transfer limiting process. Fig. 1 showed the EIS of the bare Au electrode and TFIL/Au electrode in a solution of 5.0 × 10−3 mol L−1 [Fe(CN)6]3−/[Fe(CN)6]4− containing 0.1 mol L−1 KCl with the frequencies ranging from 100k to 0.1 Hz. Conspicuous semicircle portion was observed at bare Au electrodes. But a smaller semicircle was exhibited at the TFIL/Au electrode, indicating a higher conductivity of the electrode interface on the TFIL/Au electrode due to the presence of the highly conductive IL. It demonstrated that the immobilized IL on the Au electrode surface can greatly increase the electron-transfer rate.
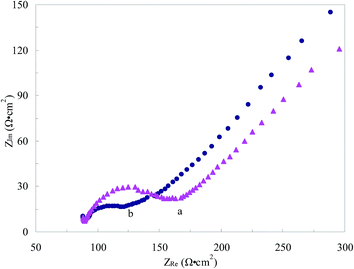 |
| Fig. 1 The EIS of 5 mM K3[Fe(CN)6]/K4[Fe(CN)6] and 0.1 mol L−1 KCl in PBS (pH 7.0) at bare Au electrode (a) and TFIL/Au electrode (b). The frequency ranges from 100k to 0.1 Hz. | |
3.2. Control and measurement of Te
Te of proposed electrode could be adjusted by heating DC, therefore, the relationship between the heating current and Te should be examined before experiments. The procedure of electrode temperature calibration was similar to the previous literatures.11 As shown in Fig. 2A, Te nearly increases linearly with the increasing square of the heating current (I2). This linear dependence clearly indicates that a thermal diffusion layer of constant thickness exists. Based on this relationship, Te could be changed easily by changing the heating current on the electrode. It should be mentioned that the thermal conductivity for different electrode is possibly inhomogeneous, so the temperature calibration should be performed for every electrode.
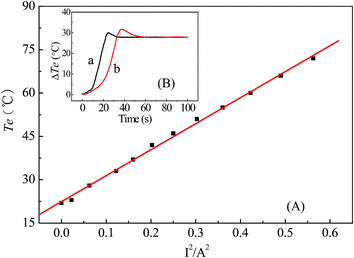 |
| Fig. 2 Relationship between the square of the I2 and Te (A); and Te change at the heated ITO electrode during heating and after heating (B) with the presence (a) and absence (b) of thermal conductive adhesion in electrode. | |
When the IL/Au electrode was heated, Te could be changed easily and rapidly by changing the heating current on the electrode. As illustrated in Fig. 2B, Te could rise rapidly after initiation of heating, and reached a constant value in approximately 25 s. The constant value of Te depends on the output of the DC power supply. In brief, Te could be changed rapidly by changing the heating current. In the directly heating mode, the thermal response of the IL/Au electrode was slightly lower than that of the heated metal wire microelectrodes with directly heating.16 It showed that thermal response was also sensitive by indirectly heating mode. Additionally, the thermal conductive adhesion adding into the electrode is beneficial to expediting the temperature response with the indirectly heating mode.
3.3. The stability and reproducibility of the heated IL/Au electrode
The CV of 1 × 10−3 mol L−1 K3[Fe(CN)6]/K4[Fe(CN)6] was scanned for 50 cycles every day. Then, the electrode was heated to 70 °C for 3 min. This procedure was repeated in the next fifteen days. The results showed that the redox peak currents decreased less than 5% in the first 12 days, and the peak currents gradually decreased in the following three days. It indicated that the stability of electrode could be maintained for 12 days when the electrode was continuously used. The results could be attributed to the existence of the stable Au–S bond and the use of the heated electrode technology. The surface fouling effects could be minimized by moderately heating electrode, which could be attributed to the increasing of mass transport at elevated temperature, and led to fast removal of reaction products, thus preventing the formation of blocking polymeric films.17 In contrast, TFIL could be stably immobilized on the surface of Au electrode in high Te. So, the propose electrode can be used for a long time if avoiding heating to an extremely high temperature, which results from the combination of heating electrode with IL-modified electrode.
After the IL/Au electrode was exposed in air for two months, it was found that the oxidation–reduction potential of K3[Fe(CN)6]/K4[Fe(CN)6] were essentially unchanged, and the redox peak currents decreased less than 5% compared with the initial steady-state value. It showed that the preservation period of electrode could last for at least two months, which is attributed to the stable Au–S bone and the very low vapor pressure in ambient conditions of ILs on electrode.
3.4. Electrochemical detection of HSO3− by the presented heated TFIL/Au electrode
To further prove the performance of the presented heated TFIL/Au electrode for practical application, DPV behavior of HSO3− was investigated and used to establish a method of measuring HSO3−. As curve (b) and (c) shown in Fig. 3, when TFIL was modified on the Au electrode, the oxidation potential shifted in the negative direction with the visibly enhancing peak current. The result indicated that the presence of the ionic liquid on the Au electrode showed great promotion to increase the electrochemical response, which was attributed to the decrease of the impedance of the double-layer by the highly conductive IL and the catalytic effect of the IL modified electrode towards the HSO3− oxidation.5
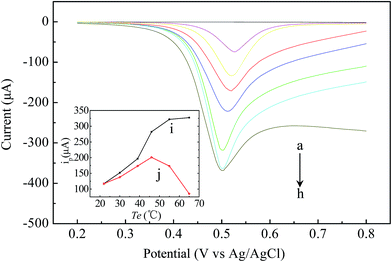 |
| Fig. 3 DPV curves of HSO3− at different Te (from top to bottom: blank solution (a); HSO3− on bare Au electrode at 22 °C (b); HSO3− on the heated TFIL/Au electrode at 22, 30, 39, 46, 55 and 65 °C (c–h), respectively). Insets: plots of the peak currents vs. temperatures of heating electrode (i), heating the entire solution by thermostatic soil bath (j). Detection buffer, PBS (0.1 mol L−1, pH 7.4); scan rate, 100 mV s−1; [HSO3−] = 1 × 10−5 mol L−1. | |
The increased temperature of the electrode not only affects the thermodynamic quality but also dramatically changes all the kinetic phenomena, including transport processes.13 Therefore, the DPV of the heated electrode was further investigated. As shown in Fig. 3, with successive increases of the electrode temperature by DC heating, the peak currents were remarkably increased, and the peak potentials shifted negatively. By using the heated electrode technology, the temperature in a thin hot solution layer near to the electrode surface could be significantly increased while the bulk solution could stay at room temperature, which has more advantages than the traditional thermostatic water or oil bath. The effect of increasing the electrode temperature on the peak current by heating the entire solution with a thermostatic oil bath is also displayed in the inset of Fig. 3. In contrast to those when heating only the electrode (curve b in the inset), the increases of the peak currents with temperature were less (curve j in the inset) by a thermostatic soil bath, and the decreased responses were observed at temperatures over about 46 °C. The phenomena were possibly due to the denaturation of HSO3− at higher temperature of the whole solution. This is a notable advantage of the heated IL/Au electrode, which could be used to study the electrochemical behavior of thermal unstable materials at higher temperature.
The linear relationships between the peak current and the concentration of HSO3− were observed in PBS (0.1 mol L−1, pH 7.4). On the bare Au electrode (Te = 22 °C), the linear regression equations were ip (μA) = 2.42 × C (μmol L−1) + 144.82 in the range of 10–800 μmol L−1. On the heated TFIL/Au electrode (Te = 55 °C), the linear regression equations were ip (μA) = 19.92 × C (μmol L−1) + 90.82 in the range of 0.6–100 μmol L−1. The detection limits (3σ/K, where σ denotes the standard deviation of arithmetic media of the currents obtained from ten voltammograms of blanks and K is the slope of analytical curves) for HSO3− were found to be 4.8 and 0.2 μmol L−1 on the bare Au electrode (Te = 22 °C) and the TFIL/Au electrode (Te = 55 °C), respectively. Generally, these results indicated that the application of the heated TFIL/Au composite electrode in an EC sensor for HSO3− could provide higher sensitivities, wider linear ranges and lower detection limits. On one hand, the immobilized IL on the Au surface can greatly increase the electron-transfer rate, which was attributed to the specific physicochemical properties of ILs. On the other hand, the increased Te by heating the electrode not only affects the electrochemical reaction kinetic but also dramatically enhances the mass transport through diffusion and convection on the electrode surface,18 which was beneficial to the improving of EC signals.
3.5. ECL detection of phenol on the heated TFIL/Au electrode
As electrochemical reaction is the key course for ECL, the application of the heated TFIL/Au electrode in ECL detection also could offer many advantages. Simultaneously, the hydrophilic and hydrophobic properties on the electrode surface could be tuned by the cation and/or the anion of the ionic liquid moiety. Considering that hydrophobicity of [MMIM]PF6− used in proposed electrode, the phenol was used as detection target for evaluating the application performance of heated TFIL/Au electrode on ECL sensors.
Fluorescein has been widely used as the fluorescent label and the chemiluminescence (CL) reagent because of its high-quantum efficiency and high sensitivity. It was also reported that the ECL from fluorescein could be initiated by various oxygen-containing species electrogenerated.19 Based on the quenching effect on ECL fluorescein/K2S2O8 by phenol in an alkaline condition, the ECL detection of phenol was developed to characterize the performance of the heated TFIL/Au electrode.
The amphiphilic nature of the electrode was demonstrated using contact-angle measurements. As shown in Fig. 4, the contact angle of water on bare Au electrode is about 64.1°, which is much less than that of water on TFIL/Au electrode (85.3°). The high contact angle indicates that the hydrophobic substance, such as phenol, easily wets on TFIL/Au electrode.
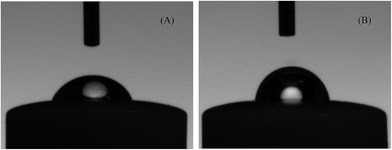 |
| Fig. 4 Images of water droplet on a bare Au electrode (A) and on a TFIL/Au electrode (B). Volume of water droplet, 3 μL; flow rate, 1 μL s−1. | |
As illustrated in Fig. 5A and B, more stable ECL signals can be observed on the TFIL/Au electrode. And the ECL signals of fluorescein/K2S2O8 were enhanced slightly by the immobilization of TFIL on the Au electrode. Conversely, when phenol was present, the ECL signals on the TFIL/Au electrode were significantly lower than that on the bare Au electrode. Hence, the significant enhancements of quenching signals (ΔECL) were obtained by the application of TFIL. Besides the increase of the electron-transfer rate, higher quenching signals on the TFIL/Au electrode might be attributed to the hydrophobic properties of [MMIM]PF6−, which resulted in the enrichment of phenol at the hydrophobic IL layer on the electrode. As illustrated in Fig. 5D, when the electrode was heated, ECL signals were enhanced to different degrees. The highest ECL of fluorescein/K2S2O8 with presence and absence of phenol were gained at 65 and 55 °C, respectively. It resulted in the highest ΔECL was obtained at 65 °C. The ECL signals at 65 °C were showed in Fig. 5C. Compared with on TFIL/Au (Fig. 5B) or bare Au electrode (Fig. 5A) at room temperature, the ΔECL at 65 °C was obviously enhanced, which resulted in higher sensitivities of ECL determination for phenol.
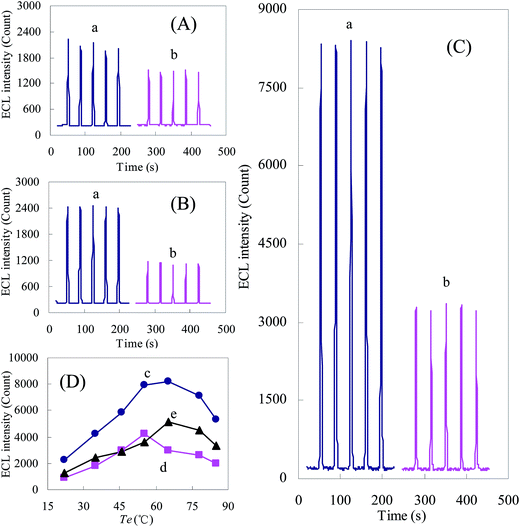 |
| Fig. 5 ECL signals fluorescein/K2S2O8 (a) and phenol/fluorescein/K2S2O8 (b) of on bare Au electrode (A) at 22 °C, heated TFIL/Au electrode at 22 °C (B) and 65 °C (C). Insets (D), plots of the ECL intensity of fluorescein/K2S2O8 (c), fluorescein/K2S2O8/phenol (d) and ΔECL (e) vs. temperatures of TFIL/Au electrode. Detection buffer, PBS (0.2 mol L−1, pH 9.5) containing 1 × 10−5 mol L−1 fluorescein and 1 × 10−3 mol L−1 K2S2O8; the range of potential, −1.2 to 0.5 V (vs. Ag/AgCl); scan rate, 100 mV s−1; [phenol] = 1 × 10−5 mol L−1. | |
The performances for phenol measurements on different electrodes were determined. On the TFIL/Au electrode, the linear regression equations were ΔECL (count) = 444.48 × C (μmol L−1) + 198.25 at 65 °C and ΔECL (count) = 108.84 × C (μmol L−1) + 146.12 at 22 °C, respectively. The linear ranges were 0.2–80 μmol L−1 at 65 °C and 3.0–200 μmol L−1 at 22 °C, with detection limit of 0.05 and 0.6 μmol L−1, respectively. In contrast to that, the detection limit for phenol was 1.5 × 10−6 mol L−1 on the bare Au electrode. The RSDs (n = 5) of ΔECL for 5 μmol L−1 and 0.5 μmol L−1 phenol were 3.7% and 4.3% at room temperature on the bare Au electrode, respectively. And those were 2.9% and 3.3% at 65 °C on the TFIL/Au electrode, respectively. The stable signals were due to the modification of ILs and the moderately heated electrode, which could reduce the surface fouling effects of Au electrodes. It could probably be attributed to desorption of reaction products by the increase of mass transport rate at elevated Te,13 and the decrease of adsorption by ILs on electrode.5 The reduction of surface fouling effects resulted in higher reproducibility on the heated TFIL/Au electrode.
To validate the presented ECL method by the heated TFIL/Au electrode, phenol contents in wastewater samples were analyzed. The experiment results obtained from our method were compared with those obtained from the UV-vis detection method,20 in which phenol reacts with 4-aminoantipyrine and then is detected spectrophotometrically at 510 nm. There were no significant differences for the two methods, with relative error less than ±5%, which indicates that the results obtained by ECL detection on the heated TFIL/Au electrode are reliable.
In short, the presented electrode has many incomparable advantages over the bare Au electrode in ECL detection. Higher sensitivities and reproducibilities of ECL detection for phenol were gained by the heated TFIL/Au electrode. It is showed that this electrode has great potential for the ECL determination.
4. Conclusions
In this paper, an electrically heated TFIL/Au composite electrode was successfully fabricated. Here, Te could be controlled accurately while the temperature of the bulk solution kept constant. The application of the thermal conductive adhesion in electrode is beneficial to expediting the temperature response of the indirectly heated electrode. The performance of the fabricated electrode was evaluated for EC detection of HSO3− and ECL detection of phenol with fluorescein/K2S2O8. The results showed several advantages by the combination of the TFIL modified Au electrode with the heated electrode technique.
Firstly, the heated TFIL/Au electrode can be used to study the electrochemical behavior of thermal unstable materials at higher temperature, such as HSO3−. Secondly, compared with the poor response on the bare Au electrode at room temperature, great improvements for the EC and ECL responses were gained on the presented electrode, which showed good stabilities and high sensitivities for the EC detection of HSO3− and ECL detection of phenol. Thirdly, a long preservation period was gained by this modified electrode, which could be attributed to the stable Au–S bone and the very low vapor pressure in ambient conditions of ILs. These results indicate that the combination of the thiol-functionalized ionic liquid with the heated electrode provides a promising electrode in EC/ECL sensing applications. It is also quite promising to couple with separation techniques, such as HPLC or CE. Furthermore, the hydrophilic and hydrophobic properties on the electrode surface could be tuned by the cation or anion of the ionic liquid moiety. And different functional ionic liquids can be used to tailor the surfaces of the heated electrode to be negatively or positively charged, towards the selective attachment of different materials, such as DNA, protein, semiconductor nanocrystals and magnetic nanoparticles.
Acknowledgements
This project was financially supported by the National Nature Science Foundation of China (51402050, 21405075, 20905034), Industrial Technology Key Project of Fujian Province (2014H0040), Young Teachers' Teaching and Scientific Research Foundation of Fujian Province (JA14248, JA14257), the Science and Technology Foundation of Minjiang University (MYK15001), and the Foster Plan for Youth Scientific Research Talent of Fujian Province.
References
- R. D. Rogers and K. R. Seddon, Science, 2003, 302, 792–793 CrossRef CAS PubMed
; P. Wasserscheid, Nature, 2006, 439, 797 CrossRef PubMed
; N. Papaiconomou, N. Glandut, I. Billard and E. Chainet, RSC Adv., 2014, 4, 58910–58915 RSC
. - P. Hapiot and C. Lagrost, Chem. Rev., 2008, 108, 2238–2264 CrossRef CAS PubMed
; W. Sun, M. X. Yang, R. F. Gao and K. Jiao, Electroanalysis, 2007, 19, 1597–1602 CrossRef
. - N. Maleki, A. Safavi and F. Tajabadi, Anal. Chem., 2006, 78, 3820–3826 CrossRef CAS PubMed
; A. Safavi, N. Maleki and F. Tajabadi, Analyst, 2007, 132, 54–58 RSC
; H. J. Chen, Y. L. Wang, Y. Liu, Y. Z. Wang, L. Qi and S. J. Dong, Electrochem. Commun., 2007, 9, 469–474 CrossRef
. - H. Itoh, K. Naka and Y. Chujo, J. Am. Chem. Soc., 2004, 126, 3026–3027 CrossRef CAS PubMed
; K. S. Kim, D. Demberelnyamba and H. Lee, Langmuir, 2004, 20, 556–560 CrossRef PubMed
. - J. F. Rong, Y. W. Chi, Y. J. Zhang, L. C. Chen and G. N. Chen, Electrochem. Commun., 2010, 12, 270–273 CrossRef CAS
. - P. Gründler and G.-U. Flechsig, Microchim. Acta, 2006, 154, 175–189 CrossRef
. - J. H. Ke, H. J. Tseng, C. T. Hsu, J. C. Chen, G. Muthuraman and J. M. Zen, Sens. Actuators, B, 2008, 130, 614–619 CrossRef CAS
. - F. Marken, S. L. Matthews, R. G. Compton and B. A. Coles, Electroanalysis, 2000, 12, 267–273 CrossRef CAS
. - P. Gründler, T. Zerihun, A. Kirbs and H. Grabow, Anal. Chim. Acta, 1995, 305, 232–240 CrossRef
. - Z. Y. Lin, J. J. Sun, J. H. Chen, L. Guo, L. Y. T. Chen and G. N. Chen, Anal. Chem., 2008, 80, 2826–2831 CrossRef CAS PubMed
. - Z. Y. Lin, J. J. Sun, J. H. Chen, L. Guo and G. N. Chen, Anal. Chim. Acta, 2006, 564, 226–230 CrossRef CAS
. - J. J. Sun, L. Guo, D. F. Zhang, W. H. Yin and G. N. Chen, Electrochem. Commun., 2007, 9, 283–288 CrossRef CAS
. - Y. T. Chen, Y. Y. Jiang, Z. Y. Lin, J. J. Sun, L. Zhang and G. N. Chen, Analyst, 2009, 134, 731–737 RSC
; L. C. Chen, Y. W. Chi, X. X. Zheng, Y. J. Zhang and G. N. Chen, Anal. Chem., 2009, 81, 2394–2398 CrossRef CAS PubMed
. - Y. T. Chen, Z. Y. Lin, J. H. Chen, J. J. Sun, L. Zhang and G. N. Chen, J. Chromatogr. A, 2007, 1172, 84–91 CrossRef CAS PubMed
; J. Wang, P. Gründler, G.-U. Flechsig, M. Jasinski, G. Rivas, E. Sahlin and J. L. L. Paz, Anal. Chem., 2000, 72, 3752–3756 CrossRef PubMed
. - Y. T. Chen, X. P. Chen, Z. Y. Lin, H. Dai, B. Qiu, J. J. Sun, L. Zhang and G. N. Chen, Electrochem. Commun., 2009, 11, 1142–1145 CrossRef CAS
. - P. Gründler, Fresenius' J. Anal. Chem., 2000, 367, 324–328 CrossRef
; P. Gründler, A. Kirb and T. Zerihun, Analyst, 1996, 121, 1805–1810 RSC
. - Z. Y. Lin, J. J. Sun, J. H. Chen, L. Guo and G. N. Chen, Electrochem. Commun., 2007, 9, 269–274 CrossRef CAS
. - G. G. Wildgoose, D. Giovanelli, N. S. Lawrence and R. G. Compton, Electroanalysis, 2004, 16, 421–433 CrossRef CAS
. - M. J. Shi and H. Cui, J. Lumin., 2007, 126, 187–195 CrossRef CAS
. - H. L. Qi, J. G. Lv and B. X. Li, Spectrochim. Acta, Part A, 2007, 66, 874–878 CrossRef PubMed
.
Footnote |
† Electronic supplementary information (ESI) available. See DOI: 10.1039/c6ra05302e |
|
This journal is © The Royal Society of Chemistry 2016 |
Click here to see how this site uses Cookies. View our privacy policy here.