DOI:
10.1039/C6RA23947A
(Communication)
RSC Adv., 2016,
6, 102968-102971
Template mediated and solvent-free route to a variety of UiO-66 metal–organic frameworks†
Received
26th September 2016
, Accepted 24th October 2016
First published on 25th October 2016
Abstract
A facile and efficient solvent-free, template oriented route has been applied for the synthesis of UiO-66 and UiO-66 analogue metal–organic frameworks (MOFs). The crystal growth was observed via time dependent powder X-ray diffraction (PXRD) and the obtained product was fully characterized by PXRD and ATR-IR. Different substances were screened as possible templates and the water content was found to be of vital importance for morphology control.
Introduction
Metal–organic frameworks (MOFs) are an emerging class of materials with useful applications in various fields, such as gas storage and separation, luminescence, catalysis, biomedicine and so on.1–5 The MOF syntheses are of great concern, as their application prospects are significantly affected by the cost and efficiency of MOF preparation, especially in the industrial field.6
Hydrothermal and solvothermal syntheses present the predominating pathways in MOF syntheses.7 In order to obtain MOFs in pure phases, larger amounts of organic solvents need to be used not only in the synthetic procedures, but also in the MOF activation processes. In the traditional synthetic procedures, solvents with high boiling points (e.g. DMF, DME) are preferred. Thus, it is either time or resources consuming to gain the final activated MOF materials by solvent exchange or supercritical CO2 extraction processes.8
Aiming to efficient and low cost syntheses of MOFs, ultrasonic,9 mechanochemical10 and microwave assisted routes11 have been applied up till now, especially for the large scale syntheses. James et al. have successively reported the first mechanochemical synthesis of a 3D microporous MOF ([Cu(INA)2], INA = isonicontinc acid) in 2006 and recently the syntheses for MOFs HKUST-1 (Cu3(BTC)2), ZIF-8 (Zn(2-methylimidazolate)2) and Al(fumarate)(OH) in large scales by extrusion, which showed quite promising prospect for the industrial application.12,13
As another important classic porous material, zeolites have recently been demonstrated to be synthesized in solvent-free synthetic routes efficiently.14–17 It is regarded as a green and less polluting way with quantitative yields. However, sustainable solvent-free routes in MOF syntheses are rare.18,19 Emmerling group first synthesized the HKUST-1 in a mechanochemical way.18 Recently, Užarević and Friščić et al. have also in situ monitored and studied the mechanochemical process of MOF-74 formation.19 Thus there's a need to develop and investigate both, methodology and mechanism. Inspired by recent publications about the solvent-free synthetic procedure of zeolites presented by Xiao et al.,14–17 we tried to introduce a template mediated solvent-free approach into the MOF preparation procedure.
Well-known for their thermal, chemical (water) and mechanical stabilities,20 Zr–MOFs are constructed with Zr–O secondary building units (SBUs), which is one of the most stable MOF species found ever. As one promising candidate, UiO-66 (UiO = Universitetet i Oslo) came into our focus as a typical object. Large scale synthesis of UiO-66 in convenient and low cost ways is rather important for the industrial applications of the UiO materials. Researchers have taken great efforts in developing the efficient and low cost syntheses of UiOs in large scales.21,22 Zhao et al. have reported a green and scalable modulated hydrothermal synthesis of UiO-66 in quite mild conditions.21 Užarević and Friščić et al. recently have developed first mechanochemical and solvent-free routes for UiO-66 and UiO-66-NH2 on the gramme scale also.22
Herein, we report on the successful and efficient solvent-free, ammonium salt templated synthesis of UiO-66 and a row of UiO-66 derivatives after crystallization at 180 °C for 24 hours. The synthetic route can also be extended to gramme scale. We also evaluated extensively the effects of templates, Zr sources and water content on the formation of the UiO-66.
Results and discussion
Crystalline UiO-66 can be obtained following the general procedure as shown in Scheme 1 (see ESI† for details). Optimized synthetic procedure is as following: the mixture of BDC (1,4-benzenedicarboxylic acid) (1 mmol), ZrOCl2·8H2O (1 mmol) and TEABr (tetraethylammonium bromide) (1.42 mmol) was grinded in the agate mortar for 5 min, then transferred to the ACE pressure tube before standing at 180 °C for 24 h crystallization.
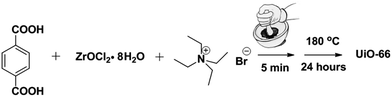 |
| Scheme 1 Templated synthesis of UiO-66 by solvent-free route. | |
The PXRD peaks of the UiO-66 prepared fit well with the simulated one derived from the UiO-66 single crystal patterns (Fig. 1), which confirms the UiO-66 framework was achieved. However, the solvent-free process without TEABr can not afford the corresponding crystalline UiO-66 materials. To our knowledge, this is the first example for a template mediated UiO-66 synthesis and excels the crystallinity obtained without templates.23 Moreover, our new solvent-free route represents a highly efficient (nearly 100% yield) and economically beneficial strategy for constructions of Zr–MOFs. The BET method showed a comparable surface area value (1113 m2 g−1) with the literature (1100 m2 g−1) for UiO-66 obtained via solvothermal process (Fig. 2).23 The thermal gravimetric analysis results also confirm the template mediated UiO-66 framework synthesized can maintain thermal stability as high as conventional synthesized one (Fig. S1†).
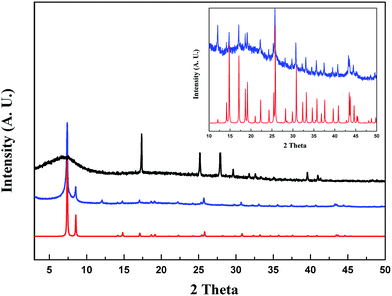 |
| Fig. 1 PXRD pattern of solvent-free synthesized UiO-66 with TEABr as the template (blue), without template (black) and the simulated one derived from single crystal (red). | |
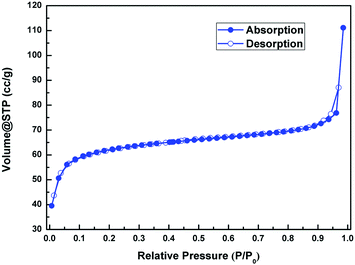 |
| Fig. 2 N2 adsorption and desorption isotherms of solvent-free synthesized UiO-66 at 77 K (adsorption branch: filled symbols, desorption branch: open symbols). | |
To further investigate this unique solvent-free synthetic procedure, the whole process has been traced by recording PXRD pattern changes (Fig. 3). The featured peaks of BDC ligand diminished, while the UiO-66 pattern developed. After 12 h crystallization, the free BDC ligand pattern disappeared and the featured peaks of UiO-66 showed comparable relative intensities with the simulated one. Moreover, the peak width showed no obvious further changes after 24 h, which means the particle sizes of UiO-66 products are consistent.
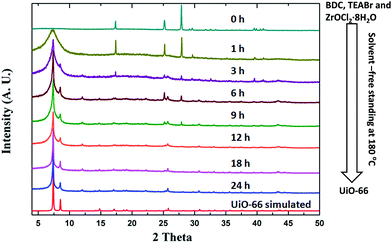 |
| Fig. 3 PXRD traces showing UiO-66 formation with time, with BDC (1 mmol), ZrOCl2·8H2O (1 mmol) and TEABr (1.42 mmol) as the precursors. | |
As discussed in the literature,24 the coordination information of the SBUs with BDC ligands and Zr ions can also be obtained by analysis on the O–C
O coordination modes via ATR-IR spectra (Fig. 4). The harmonic vibration mode for free –COOH locates at 1673 cm−1, which disappeared in the both UiO-66 spectra. In addition, ν(OCO) asymmetric and symmetric stretchings became apparent at 1581 and 1392 cm−1 respectively. Moreover, ν(C
O) at 1658 cm−1 clearly shows the appearance of DMF in the framework material obtained via solvothermal procedure. The vibration mode at 679 cm−1 can be ascribed to μ3-O stretching of the SBU Zr6O4(OH)4(–CO2)12 in the UiO-66 framework, which can be easily identified as a benchmark when tracing the solvent-free synthetic process via ATR-IR spectra (Fig. S2†). With the crystallization time increasing, the vibration peak for free –COOH at 1673 cm−1 diminished. Meanwhile, the peak at 679 cm−1 formed after 1 h, then became more and more obvious and sharper, which fits well with the trends observed in PXRD patterns in course of synthetic process.
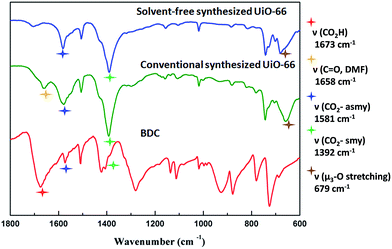 |
| Fig. 4 ATR-IR spectra of UiO-66 via different procedure and comparison with BDC ligand. | |
The templates, water contents and temperature etc. may have great impacts on the formation of the porous materials, as demonstrated in the solvent-free synthetic procedures for zeolites.14–17 Thus, we have also explored the possible influence factors in the solvent-free UiO-66 formation procedure.
Templates effect
As illustrated in the SEM images (Fig. 5), the morphology of the UiO-66 gained with TEABr as the template for solvent-free synthetic route, is nearly the same as the conventional solvo-thermal products. However, the change of template resulted in a change of morphology from agglomerated cube-shaped particles to rectangular rods, with the template TMABr (tetramethylammonium bromide) or TPABr (tetrapropylammonium bromide) used instead, which defined the different preferred growth directions with various templates. The PXRD patterns also showed the evidence that crystalline UiO-66 gained with TMABr or TPABr as the templates preferentially grow along the (2, 0, 0) crystal plane rather than (1, 1, 1) (Fig. S3†). As demonstrated in Fig. S4,† with less amount of TEABr template (0.47 mmol for 1 mmol BDC), there remains unreacted BDC ligand and the prior growth direction of crystals changes from (1, 1, 1) to (2, 0, 0) in the final product. Meanwhile, too much template (2.37 mmol for 1 mmol BDC) leads to the low crystallinity (broader PXRD peaks). In other words, the morphologies of the UiO-66 obtained can be controlled by using different templates and the amount of the template also affect the crystallinity of the final product.
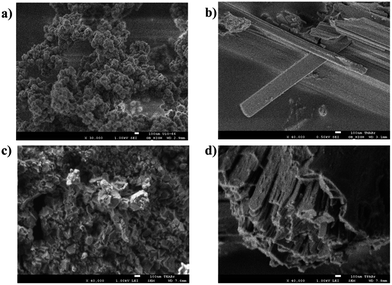 |
| Fig. 5 SEM images of UiO-66 synthesized via (a) conventional solvothermal synthesis and solvent-free synthetic route with (b) TMABr, (c) TEABr and (d) TPABr as the template respectively. | |
Water content effect
As demonstrated in the solvent-free processes for zeolite framework constructions, water is rather important for improving ion movements, which affects the synthetic procedures and final morphologies of the materials.14 Hence, the precursors were totally dried under vacuum before crystallization proceeded as a contrast in our case. As shown in Fig. S5,† the conversion of BDC ligand into UiO-66 is not quantitative, if fully-dried precursors are used for the synthesis. Thus, although the amount of water from the precursors is rather scarce, it did play an important role in ion movements, affected the reaction efficiency, just as its function in the zeolite syntheses in the literature.16 Furthermore, preferable crystal growth direction (2, 2, 0) (PXRD pattern in Fig. S5†) was obtained under such totally dried conditions.
Zr precursor effect
It's shown in literatures that the metal precursors play a vital role in the formation of the final UiO-66.20–22 Thus, we tried to use ZrCl4 instead of ZrOCl2·8H2O in our solvent-free process, following the general procedure with other synthetic conditions unchanged. And the PXRD patterns gained afterwards are quite similar with the ones taking TMABr or TPABr as the template (Fig. S6†), which means the (2, 0, 0) growth direction is favored. It may be due to the different mobilities of the metal ions (Zr4+) using different metal precursors, which affects the oriented crystal growth.
Temperature effect
Once using lower crystallization temperatures (120, 150 °C), quite much BDC ligand still remained in the final synthesized products (Fig. S7†), which also showed lower crystallinities. In addition, at temperature higher than 210 °C, the target product with undefined impurities formed, which may be due to the pyrolysis of the ammonium salts during the crystallization process.
BDC/Zr ratio effect
The BDC/Zr ratio can also affect the UiO-66 crystallization process, as illustrated in Fig. S8.† The ideal ratio is meant to be 1
:
1, as it is the ratio in the perfect UiO-66 framework.20 In our case, the BDC ligand remains unreacted with higher BDC/Zr ratio, while broader PXRD reflexes due to lower crystallinity of the product are found for lower ratio. That's also the indirect evidence for our solvent-free reaction with quantitative yield.
Ligands with various substituent groups
After the synthesis of UiO-66, the solvent-free route has also been extended to the UiO-66 analogies with substituent groups (–NH2, –NO2 and –OH) on the terephthalic acid ligands, so called UiO-66-NH2, UiO-66-NO2 and UiO-66-OH. The PXRD patterns are identical with the simulated ones respectively (Fig. S9†), except for the broader peaks. However, for the ligands with the substituent group –Br and –CH3, we cannot gain the desired framework UiO-66-Br and UiO-66-CH3. How the substituent groups affect the framework construction process in our synthetic procedure is still unknown, which we will take efforts in the future.
Conclusions
We have demonstrated for the first time that the UiO-66 and its analogues can be synthesized in a template (quaternary ammonium salt) mediated solvent-free way. The process benefits from higher atom efficiency and quantitative yield as well as lower amount of organic waste. We have investigated the crystallization process and demonstrated the influence of templates, water content, Zr sources, temperature and ligand/metal ratio on the properties of UiO-66 gained. It's also believed to be the pioneer work for introducing the quaternary ammonium salt mediated solvent-free synthesis methodology from zeolites field to MOFs. Generalization of the reported methodology for synthesis of other MOF-types would be an interesting trial which might make beneficial various industrial applications on these classes of materials. The work in this direction as well as on understanding the mechanism of solvent-free MOF formation is in progress in our lab.
Acknowledgements
Dr Chao Zou is supported by Lindau Fellowship Programme (GZ 1046) from Sino-German Science Center during the stay at Technische Universität München. SUSTC Presidential Postdoctoral Fellowship and China Postdoctoral Science Foundation (2016M590709) are also acknowledged for Dr Chao Zou's stay at South University of Science and Technology of China. Franz Koschany and Ursula Herrmann helped with the BET measurement. Katia Rodewald is thanked for the assistances of the SEM measurement. Thank Prof. Dr Thomas F. Fässler for using their facilities on PXRD tests.
Notes and references
- J.-R. Li, R. J. Kuppler and H.-C. Zhou, Chem. Soc. Rev., 2009, 38, 1477 RSC.
- Y. Cui, Y. Yue, G. Qian and B. Chen, Chem. Rev., 2012, 112, 1126 CrossRef CAS PubMed.
- A. Corma, H. Garcia and F. X. Llabres i Xamena, Chem. Rev., 2010, 110, 4606 CrossRef CAS PubMed.
- P. Horcajada, R. Gref, T. Baati, P. K. Allan, G. Maurin, P. Couvreur, G. Férey, R. E. Morris and C. Serre, Chem. Rev., 2012, 112, 1232 CrossRef CAS PubMed.
- Y. Cui, B. Li, H. He, W. Zhou, B. Chen and G. Qian, Acc. Chem. Res., 2016, 49, 483 CrossRef CAS PubMed.
- A. U. Czaja, N. Trukhan and U. Müller, Chem. Soc. Rev., 2009, 38, 1284 RSC.
- N. Stock and S. Biswas, Chem. Rev., 2012, 112, 933 CrossRef CAS PubMed.
- J. E. Mondloch, O. Karagiaridi, O. K. Farha and J. T. Hupp, CrystEngComm, 2013, 15, 9258 RSC.
- V. Safarifard and A. Morsali, Coord. Chem. Rev., 2015, 292, 1 CrossRef CAS.
- T. Friščić, Chem. Soc. Rev., 2012, 41, 3493 RSC.
- J. Klinowski, P. Almeida, A. Filipe, P. Silva and J. Rocha, Dalton Trans., 2011, 40, 321 RSC.
- A. Pichon, A. Lazuen-Garay and S. L. James, CrystEngComm, 2006, 8, 211 RSC.
- D. Crawford, J. Casaban, R. Haydon, N. Giri, T. McNally and S. L. James, Chem. Sci., 2015, 6, 1645 RSC.
- L. Ren, Q. Wu, C. Yang, L. Zhu, C. Li, P. Zhang, H. Zhang, X. Meng and F.-S. Xiao, J. Am. Chem. Soc., 2012, 134, 15173 CrossRef CAS PubMed.
- J. Zhu, Y. Zhu, L. Zhu, M. Rigutto, A. van der Made, C. Yang, S. Pan, L. Wang, L. Zhu, Y. Jin, Q. Sun, Q. Wu, X. Meng, D. Zhang, Y. Han, J. Li, Y. Chu, A. Zheng, S. Qiu, X. Zheng and F.-S. Xiao, J. Am. Chem. Soc., 2014, 136, 2503 CrossRef CAS PubMed.
- Q. Wu, X. Wang, G. Qi, Q. Guo, S. Pan, X. Meng, J. Xu, F. Deng, F. Fan, Z. Feng, C. Li, S. Maurer, U. Müller and F.-S. Xiao, J. Am. Chem. Soc., 2014, 136, 4019 CrossRef CAS PubMed.
- Q. Wu, X. Liu, L. Zhu, L. Ding, P. Gao, X. Wang, S. Pan, C. Bian, X. Meng, J. Xu, F. Deng, S. Maurer, U. Müller and F.-S. Xiao, J. Am. Chem. Soc., 2015, 137, 1052 CrossRef CAS PubMed.
- M. Klimakow, P. Klobes, A. F. Thünemann, K. Rademann and F. Emmerling, Chem. Mater., 2010, 22, 5216 CrossRef CAS.
- P. A. Julien, K. Užarević, A. D. Katsenis, S. A. J. Kimber, T. Wang, O. K. Farha, Y. Zhang, J. Casaban, L. S. Germann, M. Etter, R. E. Dinnebier, S. L. James, I. Halasz and T. Friščić, J. Am. Chem. Soc., 2016, 138, 2929 CrossRef CAS PubMed.
- J. H. Cavka, S. Jakobsen, U. Olsbye, N. Guillou, C. Lamberti, S. Bordiga and K. P. Lillerud, J. Am. Chem. Soc., 2008, 130, 13850 CrossRef PubMed.
- Z. Hu, Y. Peng, Z. Kang, Y. Qian and D. Zhao, Inorg. Chem., 2015, 54, 4862 CrossRef CAS PubMed.
- K. Užarević, T. C. Wang, S.-Y. Moon, A. M. Fidelli, J. T. Hupp, O. K. Farha and T. Friščić, Chem. Commun., 2016, 52, 2133 RSC.
- M. J. Katz, Z. J. Brown, Y. J. Colón, P. W. Siu, K. A. Scheidt, R. Q. Snurr, J. T. Hupp and O. K. Farha, Chem. Commun., 2013, 49, 9449 RSC.
- L. Valenzano, B. Civalleri, S. Chavan, S. Bordiga, M. H. Nilsen, S. Jakobsen, K. P. Lillerud and C. Lamberti, Chem. Mater., 2011, 23, 1700 CrossRef CAS.
Footnote |
† Electronic supplementary information (ESI) available: Experimental details, additional figures. See DOI: 10.1039/c6ra23947a |
|
This journal is © The Royal Society of Chemistry 2016 |
Click here to see how this site uses Cookies. View our privacy policy here.