DOI:
10.1039/C8NA00209F
(Paper)
Nanoscale Adv., 2019,
1, 799-806
Synthesis of nanoporous Mo:BiVO4 thin film photoanodes using the ultrasonic spray technique for visible-light water splitting†
Received
12th September 2018
, Accepted 7th November 2018
First published on 7th November 2018
Abstract
The use of bismuth vanadate (BiVO4) scheelite structures for converting solar energy into fuels and chemicals for fast growth in lab to industrial scale for large-area modules is a key challenge for further development. Herein, we demonstrate a new ultrasonic spray technique as a scalable and versatile coating technique for coating pristine and doped nanoporous BiVO4 thin film photoanodes directly on FTO-coated glass substrates for water splitting under visible irradiation. The successful Mo doping in BiVO4 lattice was confirmed by various characterization techniques such as XRD, Raman, EDS and XPS. The Mo:BiVO4 photoelectrode showed excellent performance with higher stability as compared to pristine BiVO4 samples.
1. Introduction
Splitting water into hydrogen and oxygen through photoelectrochemical (PEC) solar energy conversion is one of the best approaches for solar fuel applications.1–4 The complete photoelectrochemical water splitting process includes two half reactions, namely, water reduction and oxidation. However, due to the multistep proton-coupled electron transfer process, water oxidation is more challenging in terms of thermodynamics and kinetics. This process can determine the water splitting efficiency. To date, numerous metal oxides, such as TiO2,5 α-Fe2O3,6 WO3 (ref. 7) and BiVO4,8–10 have been used as photoanodes for enhancing the oxygen evolution reaction (OER) from water oxidation. From the above-mentioned photoelectrode materials, BiVO4 is one of the most promising scheelite-type photoanode materials with an appropriate valence band position for OER. So far, BiVO4 thin films have been deposited by chemical as well as physical techniques such as spin-coating,11 dip-coating,12 electrostatic spray/ultrasonic spray pyrolysis,13–15 reactive magnetron co-sputtering16 and facile successive ionic layer adsorption and reaction (SILAR).17 On the other hand, the physical technique of ultrasonic spraying is the most popular and promising technique because of its simplicity, large area production capability and absence of a non-vacuum process.
So far, the ultrasonic spray technique has been used for the synthesis of various nanomaterials including perovskite solar cells (PSCs). To find commercial applications for solar fuels, it is highly important to develop large-area deposition techniques to fabricate uniform photoelectrodes. Furthermore, it is well-known that nanoarchitectures provide unique properties to improve the performance. Therefore, the synthesis of a uniform nanoporous layer of BiVO4 is a key parameter in water splitting. Moreover, improving the short diffusion length of BiVO4 by appropriate dopants, heterojunction formation or metal ion treatment is also important.18,19 Therefore, an effective deposition technique with positive dopants can help develop onsite applications of solar fuel. Cost saving by using less materials, low maintenance cost, highly uniform deposition for large-area application and simple assembly of equipment are the four main features of the ultrasonic spray technique. Herein, we report the synthesis of nanoporous BiVO4 thin films by using the ultrasonic spray technique. However, it is well-known that the photoelectrochemical performance of pristine BiVO4 is limited due to its short carrier diffusion length, which restricts the thickness of the photoelectrodes.20 Therefore, the synthesized nanoporous BiVO4 photoelectrodes have been used for water splitting under visible-light irradiation.
2. Experimental details
2.1 Chemicals and materials
FTO-coated glass substrates were purchased from TEC15, Pilkington with sheet resistance of ∼8 Ω cm−1. Precursors such as Bi(NO3)3·5H2O, NH4VO3, (NH4)MoO4 and HNO3 were purchased from Sigma Aldrich. Double distilled water was used for the preparation of precursor solutions. All these chemicals were used without further purification.
2.2 Preparation of BiVO4 thin film electrodes
The FTO-coated glass substrates were cleaned with detergent, water and ethanol. A feeding solution containing 0.2–3 mmol of Bi(NO3)3·5H2O (Sigma Aldrich; 99.9%) and NH4VO3 (Sigma Aldrich; 99.9%) in an equal molar ratio was dissolved in 10 mL of aqueous nitric acid (HNO3) solution (2 mol L−1) to form a transparent yellow precursor solution. To incorporate Mo doping, we prepared 50 mM ammonium molybdate ((NH4)2MoO4) stock solution. The desired amount of Mo stock solution was added to the above precursor to obtain Mo:BiVO4 sample. We selected 3% Mo-doping according to previous reports.20 Spray coating was performed with an ultrasonic spray system (SonoZap, Ultrasonic Atomizer Corp.). The ultrasonic nozzle (Model: WS130K50S304, Wide Spray Atomizer Nozzle) was fixed to a 130 kHz digital ultrasonic generator and the feeding rate was controlled by a syringe pump (KD Scientific). The nozzle to substrate distance was 10 cm and the precursor solution was sprayed at the rate of 0.5 mL h−1 on the FTO substrate kept at 150 °C on a hot plate in an open atmosphere, as shown in Fig. 1. The prepared light yellow films were further annealed at 450 °C for 1 h and used for further characterizations.
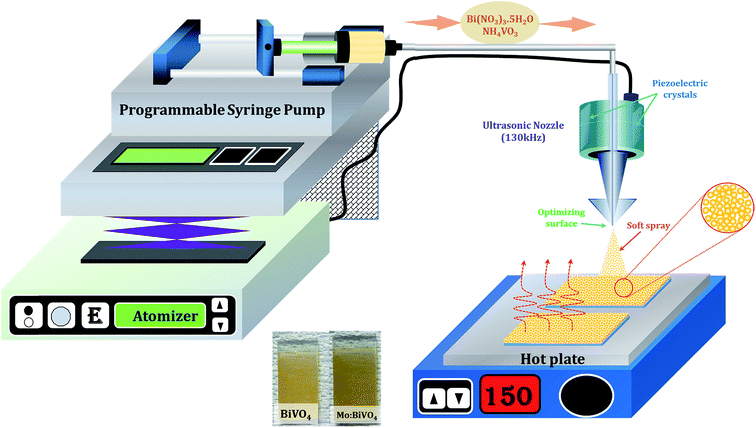 |
| Fig. 1 Schematic of concurrently pumped ultrasonic spray coating for pristine and Mo-doped BiVO4 thin film deposition. | |
2.3 Characterizations
The top-surface and cross-sectional images of un-doped and Mo-doped BiVO4 thin films were recorded by a scanning electron microscope (SEM; S-4700, Hitachi). Transmission electron microscopy (TEM) micrographs, selected area electron diffraction (SAED) patterns and high-resolution transmission electron microscopy (HRTEM) images were obtained by TECNAI F20 Philips operated at 200 kV. X-ray diffraction (XRD) measurements were carried out using a D/MAX Ultima III XRD diffractometer (Rigaku, Japan) with Cu-Kα line at 1.5410 Å. The elemental analysis of the deposited samples was performed using an X-ray photoelectron spectrometer (XPS) (VG Multilab 2000-Thermo Scientific, USA, K-Alpha) with a multi-channel detector, which can endure high photonic energies from 0.1 to 3 keV. The micro-Raman spectra of the BiVO4 samples were recorded in the spectral range of 100–1000 cm−1 using a micro-Raman spectrometer (JASCO, NRS-5100 Raman microscope) that employed a He–Ne laser source with an excitation wavelength of 633 nm and resolution of 1 cm−1 at 15 mW laser power.
2.4 Photoelectrochemical measurements
The photoelectrochemical (PEC) properties of un-doped and Mo-doped BiVO4 photoanodes were evaluated using Iviumstat (Ivium Technologies B.V., Eindhoven, the Netherlands) with a three-electrode H-type cell divided into working and counter electrode compartments by Nafion 117 (DuPont) (Fig. 6). Pristine or Mo-doped BiVO4 thin film electrode, a Pt electrode, and a saturated Ag/AgCl electrode were used as the working, counter, and reference electrodes, respectively. Also, 0.5 mol L−1 of aqueous Na2SO4 solution in potassium buffer solution (K-Pi, pH 7) was used as the electrolyte. An aqueous phosphate buffer solution [0.1 mol L−1 KH2PO3 (Sigma Aldrich; 99.6%), 0.1 mol L−1 K2HPO4 (Sigma Aldrich; 99.5%)] was employed to adjust the pH to 7 [E(RHE) = E(Ag/AgCl) + 0.1976 V + 0.0591 pH]. Nitrogen was initially purged through the solution to remove trace oxygen. Simulated solar illumination was obtained by passing light from a 300 W Xe arc lamp through an AM 1.5 G filter. The power density of the incident light was calibrated to 100 mW cm−2 by using a thermopile detector and a National Renewable Energy Laboratory – certified reference cell. J–V curves were measured by sweeping the potential in the positive direction at a scan rate of 10 mV s−1 in dark and under illumination. Electrochemical impedance spectroscopy (EIS) was conducted using Iviumstat (Ivium Technologies B.V., Eindhoven, the Netherlands) at an open-circuit potential at frequencies ranging from 10−1 to 105 Hz with AC amplitude of 10 mV.
3. Results and discussion
The surface morphology of the as-deposited samples was studied by scanning electron microscopy (SEM) (Fig. S1†). The SEM micrograph of as-deposited pristine BiVO4 sample exhibited irregular deposition and agglomeration of nanoparticles (Fig. S1a†), whereas as-deposited Mo:BiVO4 showed uniform coating with porous nanostructures (Fig. S1b†). To crystallize and remove the remaining solvent, we annealed these samples at 450 °C for 2 h. Fig. 2 shows the top view and cross sectional SEM images of pristine and Mo:BiVO4 thin films at different magnifications. The pristine BiVO4 sample showed agglomerated nanoparticle morphology. In contrast, the Mo:BiVO4 sample showed more nanoporous networks compared to pristine sample. These networks along with porous nanostructures were obtained due to volatilization of H2O and HNO3 and decomposition of by-products. To check the thickness of fabricated Mo:BiVO4 photoelectrode, scanning electron microscopy (SEM) images were acquired for both samples. The SEM micrographs revealed that the surface of FTO substrate was uniformly coated with BiVO4 films with 1.1–1.2 μm thickness. This nanoporous architecture facilitated better penetration of aqueous Na2SO4 electrolyte solution into either BiVO4 or Mo:BiVO4 films.
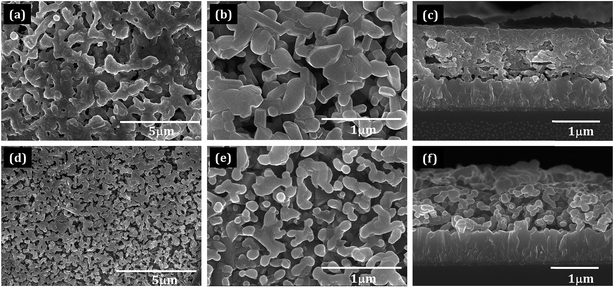 |
| Fig. 2 Scanning electron microscopy images of pristine BiVO4 (a and b) and Mo:BiVO4 (d and e) thin films deposited by the ultrasonic technique. (c) Cross-section of BiVO4 thin film electrodes. (f) Cross-section of Mo:BiVO4 thin film electrodes (both samples were annealed at 450 °C for 2 h). | |
Fig. 3 shows the XRD patterns of un-doped BiVO4 and Mo:BiVO4 thin film samples. The XRD patterns revealed the formation of single phase BiVO4 crystals with scheelite structures and monoclinic phase.21 The lattice constants were calculated to be a = 5.0195 Å, b = 11.701 Å, and c = 5.092 Å, which matched well with literature data (JCPDS no. 014-0688). On the other hand, Mo-doped BiVO4 did not show any significant peak for Mo doping, which might be due to weak diffraction intensities of Mo compared to those of pristine BiVO4 or due to very less amount of Mo (2%) employed in this synthesis. However, the characteristic peak of Mo:BiVO4 sample at 2θ = 28.822° corresponding to the (hkl) plane (−121) slightly shifted and appeared at 2θ = 28.889° due to compressive lattice strain.22 Furthermore, the peak for (−130) at 2θ = 28.586° disappeared. Raman spectra showed the same features for pristine BiVO4 and Mo:BiVO4 thin films (Fig. 4). The prominent peak at 827.53 cm−1 was assigned to stretching mode νs (V–O) vibration. Careful observation revealed a weak shoulder at 710 cm−1 corresponding to νas (V–O). The δas (VO)43− and δa (VO)43− modes were around 344.12 and 367.45 cm−1. For Mo:BiVO4, the V–O stretching mode shifted to a lower wavenumber (823.42 cm−1) as compared to 827.53 cm−1 for BiVO4, which revealed that the short-range symmetry of VO4 tetrahedron increased. This Raman shift indicated that longer V–O bond length was observed because V was replaced with Mo in VO4 tetrahedron.23–25 The Raman spectrum also showed a satellite peak at 872.02 cm−1 corresponding to the Mo–O–Mo stretching mode.
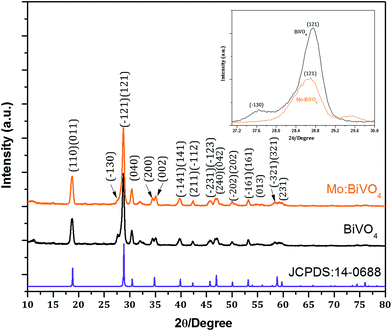 |
| Fig. 3 XRD patterns of bare BiVO4 and Mo:BiVO4 thin films synthesized by the ultrasonic spray technique. For comparison, standard diffraction pattern of JCPDS no. 14-0688 is given. The inset shows characteristic peak shift to higher angle 2θ position. | |
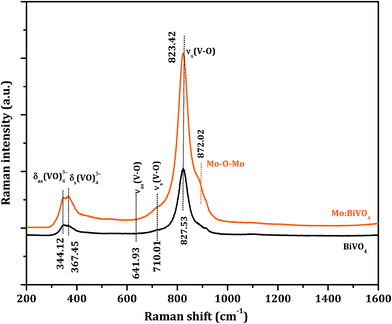 |
| Fig. 4 Raman spectra of BiVO4 and Mo:BiVO4 nanoporous thin films. | |
For in-depth analysis, we have recorded TEM/HRTEM of Mo:BiVO4 sample. Fig. 5 shows the TEM images of Mo:BiVO4 sample at different magnifications. The HRTEM images and SAED pattern were recorded to analyse the crystal structures of the Mo:BiVO4 nanoporous sample. The SAED intense ring pattern (Fig. 5b inset) revealed that synthesized Mo:BiVO4 is highly crystalline in nature. The HRTEM analysis of Mo:BiVO4 was confirmed from the continuous bright long lattice fringes with interplanar lattice spacing of d130 = 0.312 nm between the adjacent lattice fringes, as shown in Fig. 5d. The highly polycrystalline nature of BiVO4 was confirmed from the continuous bright long lattice fringes. The elemental analysis was confirmed by recording STEM/EDS elemental mapping (Fig. 5e–j). The elemental mapping confirmed the uniform distribution of bismuth, vanadium, oxygen and molybdenum throughout the surface. The calculated Mo doping percentage was ∼1.85%.26,27 Optical properties of these samples were confirmed by UV-Vis spectroscopy, as shown in Fig. S2.† Both electrodes showed typical light absorption in the UV and visible wavelength ranges. The band edge for BiVO4 thin film sample was observed at nearly 515 nm, which further increased up to 537 nm. From the Tauc plots, it was observed that the initial band-gap of 2.41 eV reduced to 2.31 eV due to Mo doping.
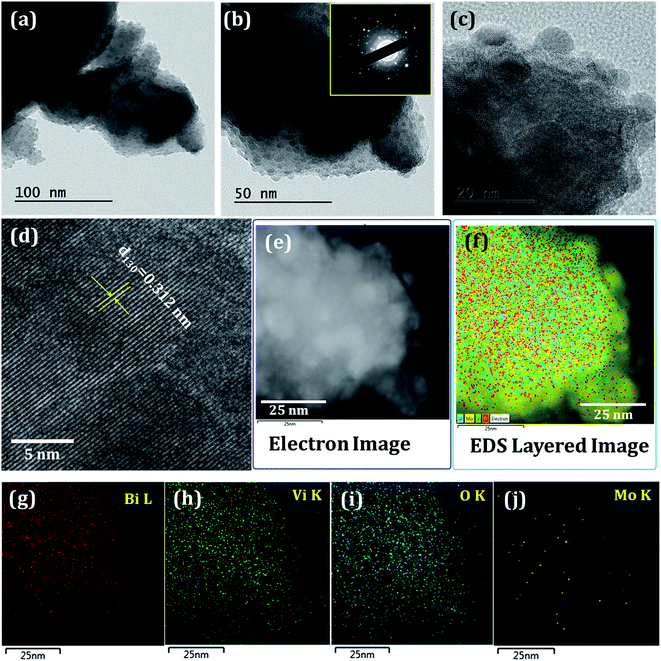 |
| Fig. 5 (a–c) TEM images of Mo:BiVO4 sample at different magnifications (the inset in b shows the SAED pattern); (d) HRTEM image; (e–j) the corresponding STEM and EDS element mapping images for Bi, V, O and Mo. | |
Chemical compositions of un-doped and Mo:BiVO4 samples were analysed by XPS (Fig. 6). The binding energies of pristine BiVO4 sample for Bi(4f5/2) and Bi(4f7/2) core levels were located at 163.77 and 158.47 eV, respectively; however, for the Mo:BiVO4 sample, these core levels slightly shifted toward higher energy and appeared at 163.96 and 158.59 eV, respectively. Besides, the binding energies of V 2p showed negligible difference between BiVO4 and Mo:BiVO4 (Fig. 6a). In contrast, the V 2p states at 523.70 and 516.45 eV for BiVO4 slightly shifted to 524.36 and 516.82 eV for Mo:BiVO4 (Fig. 6b). This shift may be caused by different electronegativities of Mo6+ and V5+. The two peaks at 529.5 and 531.3 eV were ascribed to the lattice oxygen (O 1s) of BiVO4 crystal and –OH groups formed on the surface of both samples, respectively (Fig. 6c). The above results support the conclusion that Mo6+ ions can be substituted for Bi3+ ions in BiVO4.28,29 The binding energy of Mo(3d5/2) at 231.47 eV and Mo(3d3/2) at 234.7 eV showed that Mo is present in 6+ oxidation state (Fig. 6d).22,30 No additional phases were observed. Furthermore, the doping concentration of Mo:BiVO4 was determined to be ∼2% via XPS spectra. These results are also consistent with the results of EDS (Fig. S3†) and XPS analyses (Fig. S4†).
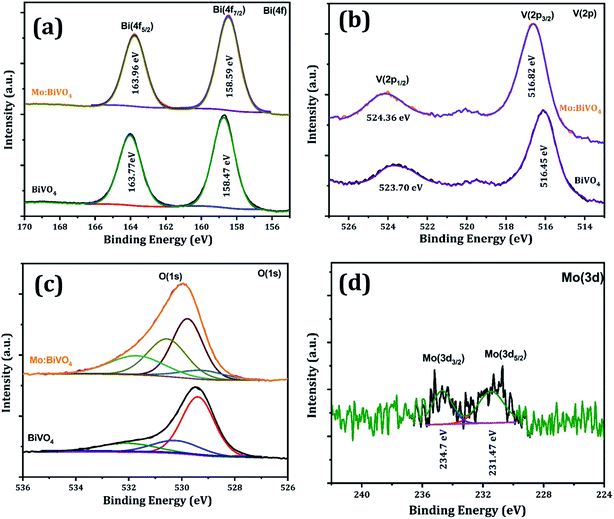 |
| Fig. 6 XPS spectra of pristine BiVO4 and Mo:BiVO4 thin films; high-resolution core level XPS spectrum of (a) Bi 4f (b), V 2p (c), O 1s (d) and Mo 3d. | |
The photoelectrochemical properties of undoped and Mo doped BiVO4 thin films were tested in an H-cell two-compartment cell composed of FTO/BiVO4 as the photoanode, Ag/AgCl as the reference electrode and Pt mesh as the counter electrode. The experimental arrangement for photocurrent measurements is shown in Fig. 7a. Here, Nafion 117 membrane was used to separate the cathode and anode compartments. A three-electrode cell configuration was used for the measurements. FTO/BiVO4, Ag/AgCl and Pt mesh were used as the working electrode, reference electrode and counter electrode, respectively. Fig. 7b shows the processes in which the holes react with OH- in the electrolyte to produce oxygen (4OH− + 4h+ → 2H2O + O2).16 At the same time, the electrons can transfer to the counter electrode (Pt electrode) to react with water to produce hydrogen (2H2O + 2e− → H2 + 2OH−). This results in a photocurrent, and its intensity mainly depends on the charge carrier separation rate and thus can reflect true water splitting. The photocurrent generation activity of both type of samples was studied under 100 mW cm−2 (AM 1.5 G) illumination in 0.5 M Na2SO4 electrolyte. The measured photocurrent–density curves versus applied potential for both type of electrodes are shown in Fig. 7c. During measurement of current in the dark, a very negligible current of ∼0.05 mA cm−2 was observed at ∼1.3 V (vs. Ag/AgCl), which may be due to the non-faradic reaction. To measure the rate of hydrogen and oxygen production by water splitting, current–voltage measurements are the best option. Fig. 7c shows the J–V plots of pristine BiVO4 and Mo:BiVO4 samples measured under illumination. It is clear that the photo-generated current density for Mo:BiVO4 is much higher than that of pristine BiVO4. This enhanced photoelectrochemical water oxidation for Mo:BiVO4 can be ascribed to the enhanced hole diffusion length and increased charge separation, which reduces the charge transfer resistance for water oxidation. Furthermore, it is noted that Mo doping facilitates improved electron mobility. It is also noted that the nanoporous network facilitates enhanced electronic transport due to Mo doping. Interestingly, these photoelectrodes are quite stable and show good photocurrent stability as well as switching behaviour (Fig. 7d).30 Electrochemical impedance spectroscopy (EIS) measurements of pristine sample and Mo:BiVO4 sample were further obtained to investigate the charge transfer resistance and separation efficiency. As shown in Fig. 8, the arc radius of Mo:BiVO4 under light is smaller than that of pristine BiVO4, thus indicating that Mo:BiVO4 has lower resistance than pristine BiVO4 and accelerated interfacial charge-transfer process. To check the long-term stability under chopped light, we recorded the transient properties of BiVO4 (Fig. S5a and S5b†) and Mo:BiVO4 (Fig. S5c and S5d†) electrodes in Na2SO4 electrolyte (Fig. S5†). The pristine BiVO4 sample initially shows 0.241 mA cm−2 current density, which further decreases up to 0.185 mA cm−2 after 500 s; furthermore, decrease is observed up to 0.140 mA cm−2 after 3600 s. However, in the case of Mo:BiVO4, the initial current density of 0.618 mA cm−2 decreases slightly up to 0.594 mA cm−2 at 200 s, and there is no observable decrease up to 3600 s. From stability analysis, it is clear that the Mo:BiVO4 sample shows much higher stability and performance than the pristine BiVO4 electrode.
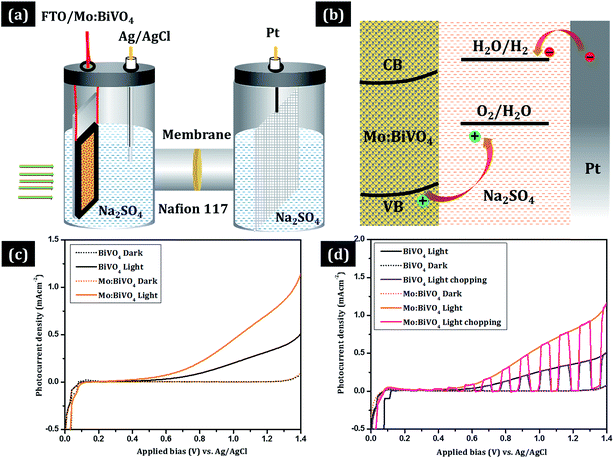 |
| Fig. 7 (a) Experimental set-up of an H-shaped photoelectrochemical cell with two separate compartments for photocatalytic oxidation of water to O2; (b) mechanism of photoelectrochemical water splitting; (c) photocurrent density–potential curves recorded under dark (dotted line) and light (solid line) (scan rate = 10 mV s−1); (d) chopping performance. | |
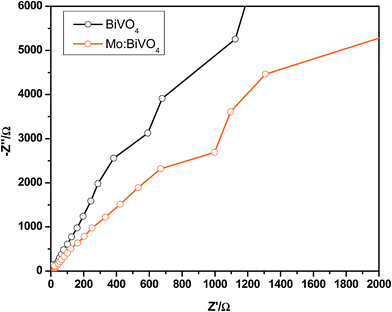 |
| Fig. 8 Electrochemical impedance spectroscopy (EIS) of pristine and Mo:BiVO4 samples under illumination. | |
4. Conclusions
In conclusion, we have successfully developed a new method to synthesize pristine and doped BiVO4 nanoporous thin films using the ultrasonic spray technique for efficient water splitting under visible-light irradiation. The pristine and Mo-doped BiVO4 nanoporous thin films were characterized by XRD, HRTEM and XPS. Our water splitting results revealed enhanced photocurrent density for Mo:BiVO4 against that for pristine BiVO4 due to the nanoporous structure and Mo doping. The present ultrasonic spray technique opens a new approach toward large-area deposition of nanoporous BiVO4 photoelectrodes for onsite applications.
Conflicts of interest
There are no conflicts to declare.
Acknowledgements
This research was supported by the National Research Foundation of Korea (NRF) (NRF-2017R1A2B4008117). This work was also supported by the Korea Research Fellowship Program through the National Research Foundation of Korea (NRF) funded by the Ministry of Science, ICT and Future Planning (2016H1D3A1909289) for an outstanding overseas young researcher. This work was supported by Priority Research Centers Program through the National Research Foundation of Korea (NRF) funded by the Ministry of Education, Science and Technology (2018R1A6A1A03024334).
References
-
A. Fujishima and K. HondaElectrochemical Photolysis of Water at a Semiconductor Electrode Nature, 1972, vol. 238, pp. 37–38 Search PubMed
.
- K. Ohashi, J. Mccann and J. O. Bockris, Stable Photoelectrochemical Cells for Splitting of Water, Nature, 1977, 266, 610–611 CrossRef CAS
.
- M. G. Walter, E. L. Warren, J. R. McKone, S. W. Boettcher, Q. Mi, E. A. Santori and N. S. Lewis, Solar Water Splitting Cells, Chem. Rev., 2010, 110, 6446–6473 CrossRef CAS PubMed
.
- T. Hisatomi, J. Kubota and K. Domen, Recent Advances in Semiconductors for Photocatalytic and Photoelectrochemical Water Splitting, Chem. Soc. Rev., 2014, 43, 7520–7535 RSC
.
- L. Li, L. Yu, Z. Lin and G. Yang, Reduced TiO2-Graphene Oxide Heterostructure as Broad Spectrum-Driven Efficient Water-Splitting Photocatalysts, ACS Appl. Mater. Interfaces, 2016, 8, 8536–8545 CrossRef CAS PubMed
.
- H. Dotan, K. Sivula, M. Gratzel, A. Rothschild and S. C. Warren, Probing the Photoelectrochemical Properties of Hematite (α-Fe2O3) Electrodes Using Hydrogen Peroxide as a Hole Scavenger, Energy Environ. Sci., 2011, 4, 958–964 RSC
.
- P. Dias, T. Lopes, L. Meda, L. Andradea and A. Mendes, Photoelectrochemical Water Splitting Using WO3 Photoanodes: The Substrate and Temperature Roles, Phys. Chem. Chem. Phys., 2016, 18, 5232–5243 RSC
.
- R. Li, F. Zhang, D. Wang, J. Yang, M. Li, J. Zhu, X. Zhou, H. Han and C. Li, Spatial Separation of Photogenerated Electrons and Holes Among {010} and {110} Crystal Facets of BiVO4, Nat. Commun., 2013, 4, 1432 CrossRef PubMed
.
- L. Chen, F. M. Toma, J. K. Cooper, A. Lyon, Y. Lin, I. D. Sharp and J. W. Ager, Mo-doped BiVO4 Photoanodes Synthesized by Reactive Sputtering, ChemSusChem, 2015, 8, 1066–1071 CrossRef CAS PubMed
.
- S. K. Pilli, T. E. Furtak, L. D. Brown, T. G. Deutsch, J. A. Turner and A. M. Herring, Cobaltphosphate (Co-Pi) Catalyst Modified Mo-doped BiVO4 Photoelectrodes for Solar Water Oxidation, Energy Environ. Sci., 2011, 4, 5028–5034 RSC
.
- Q. Jia, K. Iwashina and A. Kudo, Facile Fabrication of an Efficient BiVO4 Thin Film Electrode for Water Splitting Under Visible Light Irradiation, Proc. Natl. Acad. Sci. U. S. A., 2012, 109, 11564–11569 CrossRef CAS PubMed
.
- S. Hilliard, D. Friedrich, S. Kressman, H. Strub, V. Artero and C. Laberty–Rober, Solar-Water-Splitting BiVO4 Thin-Film Photoanodes Prepared By Using a Sol–Gel Dip-Coating Technique, ChemPhotoChem, 2017, 1, 273–280 CrossRef CAS
.
- X. Liu, Y. Liu, J. Su, M. Li and L. Guo, Facile Preparation of BiVO4 Nanoparticle Film by Electrostatic Spray Pyrolysis for Photoelectrochemical Water Splitting, Int. J. Hydrogen Energy, 2015, 40, 12964–12972 CrossRef CAS
.
- M. Li, Z. Liang and L. Guo, Preparation and Photoelectrochemical Study of BiVO4 Thin Films Deposited by Ultrasonic Spray Pyrolysis, Int. J. Hydrogen Energy, 2010, 35, 7127–7133 CrossRef CAS
.
- S. S. Dunkle, R. J. Helmich and K. S. Suslick, BiVO4 as a Visible-Light Photocatalyst Prepared by Ultrasonic Spray Pyrolysis, J. Phys. Chem. C, 2009, 113, 11980–11983 CrossRef CAS
.
- H. Gong, N. Freudenberg, M. Nie, R. van de Krol and K. Ellmer, BiVO4 Photoanodes for Water Splitting with High Injection Efficiency, Deposited by Reactive Magnetron Co-sputtering, AIP Adv., 2016, 6, 045108 CrossRef
.
- Y. Li, J. Zhu, H. Chu, J. Wei, F. Liu, M. Lv, J. Tang, B. Zhang, J. Yao, Z. Huo, L. Hu and S. Dai, BiVO4 semiconductor sensitized solar cells, Sci. China: Chem., 2015, 58, 148 Search PubMed
.
- Y. Pihosh, I. Turkevych, K. Mawatari, J. Uemura, Y. Kazoe, S. Kosar, K. Makita, T. Sugaya, T. Matsui, D. Fujita, M. Tosa, M. Kondo and T. Kitamori, Photocatalytic generation of hydrogen by core-shell WO3/BiVO4 nanorods with ultimate water splitting efficiency, Sci. Rep., 2015, 5, 11141, DOI:10.1038/srep11141
.
- K. Sayama, A. Nomura, T. Arai, T. Sugita, R. Abe, M. Yanagida, T. Oi, Y. Iwasaki, Y. Abe and H. Sugihara, Photoelectrochemical Decomposition of Water into H2 and O2 on Porous BiVO4 Thin-Film Electrodes under Visible Light and Significant Effect of Ag Ion Treatment, J. Phys. Chem. B, 2006, 110(23), 11352–11360 CrossRef CAS PubMed
.
- Y. Qiu, W. Liu, W. Chen, W. Chen, G. Zhou, P. C. Hsu, R. Zhang, Z. Liang, S. Fan, Y. Zhang and Y. Cui, Efficient Solar-Driven Water Splitting by Nanocone BiVO4-Perovskite Tandem Cells, Sci. Adv., 2016, 2, e1501764 CrossRef PubMed
.
- L. Zhang, D. Chen and X. Jiao, Monoclinic Structured BiVO4 Nanosheets: Hydrothermal Preparation, Formation Mechanism, and Coloristic and Photocatalytic Properties, J. Phys. Chem., 2006, 110, 2668–2673 CrossRef CAS PubMed
.
- Z. Jiang, Y. Liu, T. Jing, B. Huang, X. Zhang, X. Qin, Y. Dai and M. H. Whangbo, Enhancing the Photocatalytic Activity of BiVO4 for Oxygen Evolution by Ce Doping: Ce3+ Ions as Hole Traps, J. Phys. Chem. C, 2016, 120, 2058–2063 CrossRef CAS
.
- V. I. Merupo, S. Velumani, G. Oza, M. Makowska-Janusik and A. Kassiba, Structural, Electronic and Optical Features of Molybdenum-Doped Bismuth Vanadium Oxide, Mater. Sci. Semicond. Process., 2015, 31, 618–623 CrossRef CAS
.
- M. S. Jang, H. L. Park, J. N. Kim, J. H. Ro and Y. H. Park, Raman Spectrum in Monoclinic BiVO4, Jpn. J. Appl. Phys., 1985, 24, 506–507 CrossRef CAS
.
- R. L. Frost, D. A. Henry, M. L. Weier and W. Martens, Raman Spectroscopy of Three Polymorphs of BiVO4:Clinobisvanite, Dreyerite and Pucherite, With Comparisons to (VO4)3-Bearing Minerals: Namibite, Pottsite and Schumacherite, J. Raman Spectrosc., 2006, 37, 722–732 CrossRef CAS
.
- S. M. Thalluri, C. M. Suarez, M. Hussain, S. Hernandez, A. Virga, G. Saracco and N. Russo, Evaluation of the Parameters Affecting the Visible-Light-Induced Photocatalytic Activity of Monoclinic BiVO4 for Water Oxidation, Ind. Eng. Chem. Res., 2013, 52, 17414 CrossRef CAS
.
- Q. Wang, J. He, Y. Shi, S. Zhang, T. Niu, H. She and Y. Bi, Designing Non-Noble/Semiconductor Bi/BiVO4 Photoelectrode for the Enhanced Photoelectrochemical Performance, Chem. Eng. J., 2017, 326, 411–418 CrossRef CAS
.
- R. P. Antony, P. S. Bassi, F. F. Abdi, S. Y. Chiam, Y. Ren, J. Barber, J. S. C. Loo and L. H. Wong, Electrospun Mo-BiVO4 for Efficient Photoelectrochemical Water Oxidation: Direct Evidence of Improved Hole Diffusion Length and Charge Separation, Electrochim. Acta, 2016, 211, 173–182 CrossRef CAS
.
- M. Xie, Z. Zhang, W. Han, X. Cheng, X. Li and E. Xie, Efficient Hydrogen Evolution Under Visible Light Irradiation Over BiVO4 Quantum Dot Decorated Screw-Like SnO2 Nanostructures, J. Mater. Chem. A, 2017, 5, 10338–10346 RSC
.
- W. Yao, H. Iwai and J. Ye, Effects of Molybdenum Substitution on the Photocatalytic Behavior of BiVO4, Dalton Trans., 2008, 1426–1430 RSC
.
Footnote |
† Electronic supplementary information (ESI) available. See DOI: 10.1039/c8na00209f |
|
This journal is © The Royal Society of Chemistry 2019 |
Click here to see how this site uses Cookies. View our privacy policy here.