DOI:
10.1039/D0SC01936D
(Minireview)
Chem. Sci., 2020,
11, 5404-5409
Novel ultraviolet (UV) nonlinear optical (NLO) materials discovered by chemical substitution-oriented design
Received
5th April 2020
, Accepted 29th April 2020
First published on 29th April 2020
Abstract
Exploring novel functional materials via chemical substitution-oriented design is an emerging strategy. The method can be expanded to the discovery of high performance ultraviolet (UV) nonlinear optical (NLO) solid state materials by a careful tuning of the substituted atoms. This minireview presents a brief introduction to chemical substitution-oriented design including single-site substitution, dual-site substitution, and multisite substitution. Several state-of-the-art UV NLO materials such as K3VO(O2)2CO3-type, KBe2BO3F2 (KBBF)-type, Ca5(PO4)3(OH)-type, and KTiOPO4 (KTP)-type phases successfully discovered by the chemical substitution method are discussed.
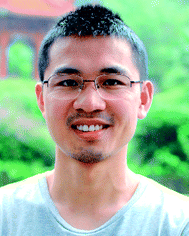 Guohong Zou | Professor Guohong Zou earned his BS degree from Central South University in 2008, and got his PhD degree in 2013 from Fujian Institute of Research on the Structure of Matter, Chinese Academy of Sciences, China, under the direction of Professor Ning Ye on discovering new nonlinear optical materials. He performed postdoctoral research in the group of Prof. Kang Min Ok at Chung-Ang University, Korea, on UV nonlinear optical crystals. Since joining the faculty of Sichuan University, China in 2017, he has performed research on discovering novel inorganic optical materials. |
 Kang Min Ok | Professor Kang Min Ok attended Sogang University, Korea, earning his BS and MS degrees in Chemistry, followed by a PhD from the University of Houston, USA, working for Professor P. Shiv Halasyamani on discovering novel second-harmonic generating solid state materials. He performed postdoctoral research in the group of Professor Dermot O'Hare at the University of Oxford, UK, on the kinetics of solid state reactions. Professor Ok worked as a distinguished scholar at Chung-Ang University (CAU), Korea. Currently, he is a Professor at Sogang University. His research includes discovering novel functional solid state materials with an asymmetric coordination environment. |
Introduction
Coherent light sources in the ultraviolet (UV) (<400 nm) region producing lasers with better beam quality, higher energy, and finer processing accuracy play a unique and crucial role in many newly developed scientific instruments that have opened up unprecedented opportunities for the exploration of novel phenomena in chemical science.1 There is an endless competition on making higher energy wavelengths accessible as we go down the Moore's law road. Thus, it is a huge challenge exploring new superior performing UV nonlinear optical (NLO) materials, the most important components for solid state UV lasers. Tremendous efforts by a number of scientists over the past few decades to discover excellent NLO materials via elucidating the structure–property relationship resulted in several successful classical and semiclassical theoretical models.2 Among them, the anionic group theory3 proposed by Chen has guided the exploration of borate UV NLO materials. A series of classical borate crystals such as β-BaB2O4 (BBO),4 LiB3O5 (LBO),5 KBe2BO3F2 (KBBF),6 and SrBe2BO7 (SBBO)7 have been successfully discovered. Subsequently, the system was further expanded to carbonates,8 nitrates,9 phosphates,10 and sulfates.11 A high performance UV NLO material should meet comprehensive requirements, which include crystallographic noncentrosymmetry (NCS), wide transparency, large second-order NLO coefficient (dij > 0.39 pm V−1), appropriate birefringence (Δn ∼ 0.07–0.10 at 1064 nm), good physicochemical stability, and “easy” growth of large high-quality single crystals. The rational combination of different asymmetric polar units including anionic groups with a π-conjugate system, d0 transition metal cations, stereochemically active lone pair (SCALP) units, and polarizable d10 metal cations is a general strategy that is beneficial for producing NCS NLO materials.12 Although combining the asymmetric units during the synthesis steps significantly improved the possibility of macroscopic NCS, the major reaction products still turned out to be centrosymmetric (CS), attributable to the preference of antiparallel alignment of the asymmetric units. Since the inefficient synthetic approaches impeded the development of UV laser technology, other effective strategies toward excellent UV NLO materials are urgently required. An emerging highly effective method to discover high-performance UV NLO materials is chemical substitution-oriented design,13 in which one or more fundamental building units (FBUs), cations, and/or anions in a prototype phase are replaced to produce better properties. In general, to discover new UV NLO materials via the chemical substitution method, the following procedures are followed: (1) choice of model phases with adjustable backbones; (2) design of novel materials through chemical substitution; (3) uncovering new UV NLO compounds through the appropriate synthesis, structure determination, and NLO property measurement; (4) growing high quality large single crystals.14 In this minireview, several recently reported UV NLO materials discovered by chemical substitution-oriented design including single-site substitution, dual-site substitution, and multisite substitution are presented. Closer structural examinations for substitution of suitable functional building blocks will successfully guide the discovery of new UV NLO materials.
Single-site substitution
Single-site substitution is a general and facile strategy during the synthesis, in which one cation or anion in the parent structure is replaced by another ion in the same group of the periodic table to obtain better UV NLO properties. In general, the cations include alkali and alkaline-earth metal cations, while the anions include halide ions. Once one of the ions is substituted, the stoichiometrically similar materials often exhibit different performances due to the ion size difference.
Two novel alkali metal carbonatoperoxovanadates, A3VO(O2)2CO3 (A = Rb and Cs),15 have been developed via single-site substitution after the discovery of K3VO(O2)2CO3 with the localized (O2)2− π-orbital NLO-active unit.16 The three stoichiometrically equivalent materials share a common molecular structure, VO(O2)2CO3 polyhedra. The backbones of A3VO(O2)2CO3 (A = K, Rb, and Cs) reveal better thermal stability as the radius of the alkali metal cations increases (Table 1). As seen in Fig. 1, since the size of K+ in K3VO(O2)2CO3 is smaller, substantial interactions between K+ cations and oxide ligands result in peroxo ligands with shorter O–O distances (1.456 Å) and more strained VO3(O2)2 pentagonal bipyramids (pbps). However, the large Cs+ cations in Cs3VO(O2)2CO3 with a larger coordination environment reveal peroxo ligands with longer O–O distances (1.503 Å) and less strained, more stable VO3(O2)2 pbps. Therefore, the thermal stability of A3VO(O2)2CO3 (A = K, Rb, and Cs) increases in the order K < Rb < Cs owing to the structural stability of the corresponding pbps. In addition, while K3VO(O2)2CO3 with the highly distorted VO(O2)2CO3 pbps exhibits a smaller band gap, those with less strained pbps reveal larger optical gaps. Moreover, the SHG responses of A3VO(O2)2CO3 increase as the alkali metal cation size increases, which should be mainly attributable to the increased polarizability of larger cations along with their stronger interatomic interactions with adjacent O atoms.
Table 1 Thermal and optical characteristics of A3VO(O2)2CO3 (A = K, Rb, and Cs)
Compound |
Dec. T (°C) |
E
g (eV) |
SHG (×KDP) |
K3VO(O2)2CO3 |
230 |
2.57 |
20 |
Rb3VO(O2)2CO3 |
250 |
2.68 |
21 |
Cs3VO(O2)2CO3 |
300 |
2.81 |
23 |
 |
| Fig. 1 Influence of the size of alkali metal cations on the stability of VO3(O2)2 polyhedra in A3VO(O2)2CO3 (A = K, Rb, and Cs). The shorter O–O distances in peroxo ligands owing to the smaller coordination environment of K+ result in more strained VO3(O2)2 pbps, while the longer O–O distances in peroxo ligands due to the large Cs+ result in less strained stable VO3(O2)2 pbps. | |
The replaceable cations in single-site substitution may be bivalent cations in the emerging UV NLO metal fluoride carbonates KMCO3F (M = Mg, Ca, Sr, Zn, and Cd).17 Also, the replaceable cations could be trivalent cations in Cd4REO(BO3)3 (RE = Y, La, Gd, Yb, and Lu).18 Here, the SHG efficiencies increase as the polarizability of cations increases. The replaceable ions in single-site substitution could also be anions, especially halogen anions. Pb2BO3Cl with a trans-KBBF structure has been proved to be a promising UV NLO crystal.19 The SHG coefficient gradually increases as the Cl− anion is replaced by larger and more polarizable Br− and I− in Pb2BO3X (X = Br and I).20
Dual-site substitution
As the name implies, dual-site substitution is a simultaneous replacement of two cations, anions, complex anions, or fundamental building units. Although the substitution has to keep the sum of the oxidation states constant, each component is not necessarily equivalent during the replacement procedure. Dual-site substitution is an aliovalent substitution that is utilized commonly to design new materials with better performance. In order to keep the framework structure of a prototype phase, the interchangeable cations or anions should present similar sizes and structures. Proposed mechanisms for the dual-site substitution to design new UV NLO materials may include the following: | M+ + AOx− = N2+ + ZOy2− = R3+ + EOz3− | (3) |
| M+ + ZOy2− = N2+ + EOz3− = R3+ + FOm4− | (4) |
| M+ + EOz3− = N2+ + FOm4− | (5) |
The evolution of the KBe2BO3F2 (KBBF)6 family provides a perfect example of dual-site substitution (Fig. 2). It is well known that KBBF is an important deep-UV NLO material due to a large NLO coefficient, a short UV absorption cutoff edge, and appropriate birefringence. However, its strong layered habit limits its practical application. In order to overcome the problem of layered habit during the crystal growth process and maintain all of the KBBF's excellent optical properties, Sr2Be2B2O7 (SBBO)7 was designed via dual-site substitution of Sr2+ + O2− = K+ + F−. As expected, SBBO presents a perfect plane infinite [Be3B3O6]∞ network which provides a large NLO coefficient and appropriate birefringence. Furthermore, the strong Be–O bonds between [Be3B3O6] layers are beneficial to solve the problem of layered habit in crystal growth. However, high quality large single crystals of SBBO have not been grown due to the structural polymorphism problem. Therefore, in order to stabilize the crystal structure, the proposed substitution of F− + Na+ = O2− + Sr2+ was applied to discover NaCaBe2B2O6F.21 As we know, the constituent Be in KBBF, SBBO, and NaCaBe2B2O6F is highly toxic. Thus, Be-free UV NLO materials have been produced through the replacement of Be with Mg, Zn, or Al. From KBBF, KZn2BO3X (X = Cl or Br) have been obtained by the cosubstitution of Cl/Br− + Zn2+ = F− + Be2+. In addition, the substitution of Be with Al can also be a method to design new Be-free KBBF family materials. K2Al2B2O7 (ref. 22) and BaAlBO3F2 (ref. 23) have been derived from KBBF through the dual-site substitution of O2− + Al3+ = F− + Be2+ and Ba2+ + Al3+ = K+ + 2Be2+, respectively. Simultaneously, BaAl2B2O7 (ref. 24) has been designed from SBBO by substituting Ba2+ + 2Al3+ = 2Sr2+ + 2Be2+. Recently, Pan's group predicted a promising Be-free UV NLO fluorooxoborate, SrB2O3F2 (ref. 25), derived from SBBO by the substitution of 4F− + 2B3+ = O2−+ 2Be2+. Hence, dual-site substitution is a green and effective strategy to design Be-free deep-UV NLO materials with high performance compared to the traditional trial-and-error method.
 |
| Fig. 2 Structural evolution of several KBBF-type UV NLO materials developed via dual-site substitutions. | |
In addition to the KBBF family, dual-site substitution can also have an effect on improving the NLO properties of other systems. For example, Pb2TiVOF(SeO3)2Cl26 exhibited a much larger SHG coefficient than its prototype compound Pb2NbVO2(SeO3)2Cl26 through the cosubstitution of Ti4+ + F− = Nb5+ + O2−. Also, the first bismuth selenite fluoride, BiFSeO3,27 reported by Mao's group revealed an enlarged SHG response, in which the novel material was designed from the parent compound iodate, BiOIO3 (ref. 28), by the cosubstitution of F− + Se2− = O2−+ I−.
Multisite substitution
Multisite substitution, as the name suggests, is the replacement of three or more cations, anions, complex anions, fundamental building units, or vacancies in prototype compounds. By far, most of the substitutions applied to design new UV NLO materials involved only two or less atomic sites with one charge difference for cations or anions, which substantially limited the diversity of structural change. If multisite substitution could be realized, the family of UV NLO materials can be greatly expanded. Firstly, we should select an appropriate prototype phase containing three or more substitutable atomic sites, in which the framework should be remarkably tolerant to structural distortion and chemical substitution. As we know, a large portion of our modern scientific database consists of a variety of minerals. Especially, a number of minerals such as apatite and perovskite presenting adaptable framework structures have significantly influenced the discovery of many novel inorganic materials with tunable physical properties in the laboratory.
For the exploration of UV NLO materials, hydroxyapatite, Ca5(PO4)3(OH),29 is an ideal prototype structure with four substitutable atomic sites, i.e., two different Ca2+ sites, one PO43− site, and one OH− site. Proposed substitution mechanisms in Ca5(PO4)3(OH) include the following:
| M+ + ZO42− = Ca2+ + PO43− | (8) |
| M3+ + ZO44− = Ca2+ + PO43− | (10) |
| 2M3+ + ZO45− = 2Ca2+ + PO43− | (11) |
| ZO42− + ZO44− = 2PO43− | (13) |
| X2− + M3+ = OH− + Ca2+ | (15) |
According to the above mechanisms, a series of excellent UV NLO borates, Ca4REO(BO3)3 (RE = rare earth metals),30 were designed and synthesized via the multisite substitution of RE3+ + O2− + BO33− = Ca2+ + OH− + PO43−. Then, in order to enhance the SHG coefficient, the introduction of a d10 cation, Cd2+, with polar displacement properties to replace Ca2+, and Bi3+ with the stereochemically active lone pair to replace RE3+ produced an excellent NLO material, Cd4BiO(BO3)3.31 In fact, Cd4BiO(BO3)3 exhibited the largest NLO coefficient among borates when it was discovered owing to the three NCS chromophores. Ye's group has extended the apatite-type UV NLO materials to carbonates. The multisite substitutions of hydroxyapatite successfully produced Na8Lu2(CO3)6F2 (ref. 32) and Na3Ca2(CO3)3F33 revealing large SHG responses and appropriate birefringence.
Recently, KTiOPO4 (KTP)34 was selected as an ideal candidate for multisite substitution to explore new UV NLO materials. KTP is a classical commercial NLO material presenting large NLO coefficients, high thermal stability, and wide acceptance angles. However, the narrow energy bandgap (3.52 eV) hinders its practical application in the UV region. Energy band engineering has been proved to be an effective strategy to enlarge the bandgap in some materials systems. In KTP, a detailed strategy involving substituting an early transition metal, Ti4+, with a main group metal to eliminate the d–p electronic transition, and substituting partial O2− with F− would induce the enlargement of the bandgap. Based on the strategy, our group developed a series of sulfates, ASbX2SO4 (A = NH4, Rb, or Cs; X = F or Cl),35 with a sharply enlarged bandgap (>4.7 eV) by introducing the main group Sb3+ cations with stereochemically active lone pairs (Fig. 3). The multisite substitution in CsSbF2SO4 is in accordance with the following scheme:
K+ + Ti4+ + O2− + PO43− (KTP) → Cs+ + Sb3+ + 2F− + SO42− (CsSbF2SO4) |
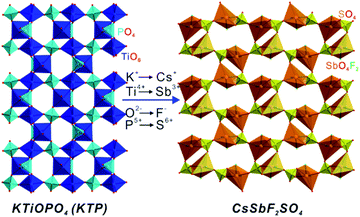 |
| Fig. 3 Polyhedral representations showing a structural comparison between KTiOPO4 and CsSbF2SO4 synthesized by multisite substitution. | |
From the viewpoint of structural evolution, CsSbF2SO4 can be regarded as a derivative of KTP. The 1-D [SbF2O2SO4]5− chains produced by the interconnection of [SbF2O4]7− distorted octahedra and [SO4]2− anions in CsSbF2SO4 are similar to those of [TiO4PO4]5− chains composed of [TiO6]8− and [PO4]3− anions in KTP. The main difference between the two structures occurs due to the introduction of fluorine atoms into the terminal sites of [SbO4F2]7− octahedra in CsSbF2SO4. While 3- and 6-membered rings (MRs) composed of bridging oxygen atoms in [TiO6]8− octahedra and [PO4]3− tetrahedra are observed in KTP, 4- and 8-MRs are formed in CsSbF2SO4 containing terminal fluorides (Fig. 3). Thus, KTP-type compounds have been extended to sulfate systems as novel UV NLO materials. Subsequently, RbSnFSO4 (ref. 36) and A2Bi2(SO4)2Cl4 (A = NH4, K, or Rb)37 with large bandgaps were successfully synthesized via the replacement of Ti4+ with Sn2+ and Bi3+ cations, respectively. Hence, the successful substitution of sulfates for phosphates may open a door to efficiently explore excellent sulfate NLO materials for UV application. Also, multisite substitution can have an effect on improving the bandgap of other systems. For example, α- and β-Ba2[GaF4(IO3)2](IO3) derived from α- and β-Ba2[VO2F2(IO3)2](IO3) via multisite substitution involving one cationic and two anionic sites exhibit sharply enlarged bandgaps.38
Conclusions and outlook
Chemical substitution-oriented design is a very effective strategy to explore high-performance UV NLO materials compared to the time-consuming conventional trial-and-error method. The method involves single-site, dual-site, and multisite substitutions. The prototype phases from naturally occurring minerals or synthetic materials should present remarkable tolerance toward structural distortion and chemical substitution in order to accommodate a large number of elements or structural units. Examples of chemical substitution-oriented design to explore novel UV NLO materials include K3VO(O2)2CO3, KBBF, Ca5(PO4)3(OH), and KTP-type phases. The chemical substitution approach can be easily applied to tune and extend the desired properties of solid state materials. Chemical substitution-oriented design is an emerging strategy for the exploration of novel UV NLO materials. Although a number of novel materials have been successfully discovered by virtue of the method, it should be emphasized that elucidating the intrinsic substitution mechanism of the thermodynamically stable crystalline phases, understanding the detailed crystal structures and compositions, and explaining the origin of experimentally observed physical properties on the basis of a firm theoretical background are indispensable.
Conflicts of interest
There are no conflicts to declare.
Acknowledgements
This research was supported by the National Research Foundation of Korea (NRF) funded by the Ministry of Science and ICT (grant no. 2018R1A5A1025208 and 2019R1A2C3005530). This work was also supported by the National Natural Science Foundation of China (no. 21875146) and the Fundamental Research Funds for the Central Universities (no. YJ201921).
Notes and references
-
(a) N. Savage, Nat. Photonics, 2007, 1, 83 CrossRef CAS;
(b) K. M. Ok, E. O. Chi and P. S. Halasyamani, Chem. Soc. Rev., 2006, 35, 710 RSC;
(c) H. Lee and K. M. Ok, Bull. Korean Chem. Soc., 2020, 41, 139 CrossRef CAS.
-
(a) B. F. Levine, Phys. Rev. Lett., 1970, 25, 440 CrossRef CAS;
(b)
C. Chen, T. Sasaki, R. Li, Y. Wu, Z. Lin, Y. Mori, Z. Hu, J. Wang, S. Uda, M. Yoshimura and Y. Kaneda, Nonlinear Optical Borate Crystals, Principles and Applications, Wiley-VCH, Weinheim, Germany, 2012, pp. 15–116 CrossRef;
(c) J. Jeon, J. Lee and T.-S. You, Bull. Korean Chem. Soc., 2018, 39, 1066 CrossRef CAS.
-
(a) C. Chen, Sci. Sin., 1979, 22, 756 CAS;
(b) C. Chen and G. Liu, Annu. Rev. Mater. Sci., 1986, 16, 203 CrossRef CAS;
(c) C. T. Chen, Y. C. Wu and R. K. Li, Int. Rev. Phys. Chem., 1989, 8, 65 Search PubMed.
- C. T. Chen, B. C. Wu, A. D. Jiang and G. M. You, Sci. Sin. Ser. B, 1985, 28, 23 Search PubMed.
- C. T. Chen, Y. C. Wu, A. D. Jiang, B. C. Wu, G. M. You, R. K. Li and S. J. Lin, J. Opt. Soc. Am. B, 1989, 6, 616 CrossRef CAS.
- L. Mei, Y. Wang, C. Chen and B. Wu, J. Appl. Phys., 1993, 74, 7014 CrossRef CAS.
- C. T. Chen, Y. B. Wang, B. C. Wu, K. C. Wu, W. L. Zeng and L. H. Yu, Nature, 1995, 373, 322 CrossRef CAS.
-
(a) Y. T. Zhang, Y. Long, X. H. Dong, L. Wang, L. Huang, H. M. Zeng, Z. E. Lin, X. Wang and G. H. Zou, Chem. Commun., 2019, 55, 4538 RSC;
(b) Q. F. Li, G. H. Zou, C. S. Lin and N. Ye, New J. Chem., 2016, 40, 2243 RSC;
(c) Q. Wang, F. F. He, L. Huang, D. J. Gao, J. Bi and G. H. Zou, Cryst. Growth Des., 2018, 18, 3644 CrossRef CAS.
-
(a) Y. X. Song, M. Luo, C. S. Lin and N. Ye, Chem. Mater., 2017, 29, 896 CrossRef CAS;
(b) L. Wang, F. Yang, X. Y. Zhao, L. Huang, D. J. Gao, J. Bi, X. Wang and G. H. Zou, Dalton Trans., 2019, 48, 15144 RSC;
(c) X. H. Dong, L. Huang, Q. Y. Liu, H. M. Zeng, Z. E. Lin, D. J. G. Xu and G. H. Zou, Chem. Commun., 2018, 54, 5792 RSC.
-
(a) S. G. Zhao, X. Y. Yang, Y. Yang, X. J. Kuang, F. Q. Lu, P. Shan, Z. H. Sun, Z. S. Lin, M. C. Hong and J. H. Luo, J. Am. Chem. Soc., 2018, 140, 1592 CrossRef CAS PubMed;
(b) J. Chen, L. Xiong, L. Chen and L. M. Wu, J. Am. Chem. Soc., 2018, 140, 14082 CrossRef CAS PubMed.
-
(a) F. F. He, L. Wang, C. F. Hu, J. Zhou, Q. Li, L. Huang, D. J. Gao, J. Bi, X. Wang and G. H. Zou, Dalton Trans., 2018, 47, 17486 RSC;
(b) Q. Wang, L. Wang, X. Y. Zhao, L. Huang, D. J. Gao, J. Bi, X. Wang and G. H. Zou, Inorg. Chem. Front., 2019, 6, 3125 RSC;
(c) F. F. He, Y. L. Deng, X. Y. Zhao, L. Huang, D. J. Gao, J. Bi, X. Wang and G. H. Zou, J. Mater. Chem. C, 2019, 7, 5748 RSC.
- K. M. Ok, Acc. Chem. Res., 2016, 49, 2774 CrossRef CAS PubMed.
-
(a) J. Y. Guo, A. Tudi, S. J. Han, Z. H. Yang and S. L. Pan, Angew. Chem., Int. Ed., 2019, 58, 17675 CrossRef CAS PubMed;
(b) F. F. Mao, C. L. Hu, X. Xu, D. Yan, B. P. Yang and J. G. Mao, Angew. Chem., Int. Ed., 2017, 56, 2151 CrossRef CAS PubMed.
- Z. G. Xia and K. R. Poeppelmeier, Acc. Chem. Res., 2017, 50, 1222 CrossRef CAS PubMed.
-
(a) G. H. Zou, H. Jo, S. Lim, T. You and K. M. Ok, Angew. Chem., Int. Ed., 2018, 57, 8619 CrossRef CAS PubMed;
(b) G. H. Zou, Z. Lin, H. M. Zeng, H. Jo, S. Lim, T. You and K. M. Ok, Chem. Sci., 2018, 9, 8957 RSC.
- Y. Song, M. Luo, F. Liang, N. Ye and Z. Lin, Chem. Commun., 2018, 54, 1445 RSC.
-
(a) T. T. Tran, J. Young, J. M. Rondinelli and P. S. Halasyamani, J. Am. Chem. Soc., 2017, 139, 1285 CrossRef CAS PubMed;
(b) G. H. Zou, N. Ye, L. Huang and X. S. Lin, J. Am. Chem. Soc., 2011, 133, 20001 CrossRef CAS PubMed;
(c) G. Yang, G. Peng, N. Ye, J. Wang, M. Luo, T. Yan and Y. Zhou, Chem. Mater., 2015, 27, 7520 CrossRef CAS.
-
(a) G. H. Zou, Z. J. Ma, K. C. Wu and N. Ye, J. Mater. Chem., 2012, 22, 19911 RSC;
(b) G. H. Zou, L. Huang, H. Q. Cai, S. C. Wang and N. Ye, New J. Chem., 2014, 38, 6186 RSC.
- G. Zou, C. Lin, H. Jo, G. Nam, T. S. You and K. M. Ok, Angew. Chem., Int. Ed., 2016, 55, 12078 CrossRef CAS PubMed.
-
(a) M. Luo, Y. Song, F. Liang, N. Ye and Z. Lin, Inorg. Chem. Front., 2018, 5, 916 RSC;
(b) H. Yu, N. Koocher, J. Rondinelli and P. S. Halasyamani, Angew. Chem., Int. Ed., 2018, 57, 6100 CrossRef CAS PubMed.
- H. Huang, J. Yao, Z. Lin, X. Wang, R. He, W. Yao, N. Zhai and C. Chen, Chem. Mater., 2011, 23, 5457 CrossRef CAS.
- Z. G. Hu, T. Higashiyama, M. Yoshimura, Y. K. Yap, Y. Mori and T. Sasaki, Jpn. J. Appl. Phys., 1998, 37, 1093 CrossRef.
- Z. G. Hu, M. Yoshimura, K. Muramatsu, Y. Mori and T. Sasaki, Jpn. J. Appl. Phys., 2002, 41, L1131 CrossRef CAS.
- N. Ye, W. R. Zeng, B. C. Wu, X. Y. Huang and C. T. Chen, Z. Kristallogr., 1998, 213, 452 CAS.
- Z. H. Yang, B. H. Lei, W. Y. Zhang and S. L. Pan, Chem. Mater., 2019, 31, 2807 CrossRef CAS.
- X. L. Cao, C. L. Hu, X. Xu, F. Kong and J. G. Mao, Chem. Commun., 2013, 49, 9965 RSC.
- M. Liang, C. Hu, F. Kong and J. Mao, J. Am. Chem. Soc., 2016, 138, 9433 CrossRef CAS PubMed.
- S. D. Nguyen, J. Yeon, S. H. Kim and P. S. Halasyamani, J. Am. Chem. Soc., 2011, 133, 12422 CrossRef CAS PubMed.
- J. M. Hughes and J. Rakovan, Rev. Mineral. Geochem., 2002, 45, 1 CrossRef.
-
(a) Y. Fei, B. H. Chai, C. A. Ebbers, Z. M. Liao, K. I. Schaffers and P. Thelin, J. Cryst. Growth, 2006, 290, 301 CrossRef CAS;
(b) H. J. Zhang, H. D. Jiang, J. Y. Wang, X. B. Hu, G. W. Yu, W. T. Yu and M. H. Jiang, Appl. Phys. A, 2004, 78, 889 CrossRef CAS;
(c) G. Aka, A. Kahn-Harari, F. Mougel, D. Vivien, F. Salin, P. Coquelin and J. P. Damelet, J. Opt. Soc. Am. B, 1997, 14, 2238 CrossRef CAS.
- W. L. Zhang, W.-D. Cheng, H. Zhang, L. Geng, C.-S. Lin and Z.-Z. He, J. Am. Chem. Soc., 2010, 132, 1508 CrossRef CAS PubMed.
- M. Luo, G. H. Zou, N. Ye, C. S. Lin and W. D. Cheng, Chem. Mater., 2013, 25, 3147 CrossRef CAS.
- M. Luo, Y. Song, C. Lin, N. Ye, W. Cheng and X. Long, Chem. Mater., 2016, 28, 2301 CrossRef CAS.
- J. D. Bierlein and H. Vanherzeele, J. Opt. Soc. Am. B, 1989, 6, 622 CrossRef CAS.
-
(a) F. F. He, Q. Wang, C. F. Hu, W. He, X. Y. Luo, L. Huang, D. J. Gao, J. Bi, X. Wang and G. H. Zou, Cryst. Growth Des., 2018, 18, 6239 CrossRef CAS;
(b) X. H. Dong, L. Huang, C. F. Hu, H. M. Zeng, Z. E. Lin, X. Wang, K. M. Ok and G. H. Zou, Angew. Chem., Int. Ed., 2019, 58, 6528 CrossRef CAS PubMed.
- F. Yang, L. J. Huang, X. Y. Zhao, L. Huang, D. J. Gao, J. Bi, X. Wang and G. H. Zou, J. Mater. Chem. C, 2019, 7, 8131 RSC.
- K. Chen, Y. Yang, G. Peng, S. Yang, T. Yan, H. Fan, Z. Lin and N. Ye, J. Mater. Chem. C, 2019, 7, 9900 RSC.
- J. Chen, C.-L. Hu, F.-F. Mao, J.-H. Feng and J.-G. Mao, Angew. Chem., Int. Ed., 2019, 58, 2098 CrossRef CAS PubMed.
|
This journal is © The Royal Society of Chemistry 2020 |
Click here to see how this site uses Cookies. View our privacy policy here.