DOI:
10.1039/D1RA05021D
(Review Article)
RSC Adv., 2021,
11, 27406-27419
Recent progress of biomimetic motions—from microscopic micro/nanomotors to macroscopic actuators and soft robotics
Received
29th June 2021
, Accepted 5th August 2021
First published on 11th August 2021
Abstract
Motion is a basic behavioral attribute of organisms, and it is a behavioral response of organisms to the external environment and internal state changes. Materials with switchable mechanical properties are widespread in living organisms and play crucial roles in the motion of organisms. Therefore, significant efforts have been made toward mimicking such architectures and motion behaviors by making full use of the properties of stimulus-responsive materials to design smart materials/machines with specific functions. In recent years, the biomimetic motions based on micro/nanomotors, actuators and soft robots constructed from smart response materials have been developed gradually. However, a comprehensive discussion on various categories of biomimetic motions in this field is still missing. This review aims to provide such a panoramic overview. From nano-to macroscales, we summarize various biomimetic motions based on micro/nanomotors, actuators and soft robotics. For each biomimetic motion, we discuss the driving modes and the key functions. The challenges and opportunities of biomimetic motions are also discussed. With rapidly increasing innovation, advanced, intelligent and multifunctional biomimetic motions based on micro/nanomotors, actuators and soft robotics will certainly bring profound impacts and changes for human life in the near future.
1. Introduction
Nature has evolved high-performance objects using commonly found materials from the nanoscale to the macroscale. Biologically inspired design or adaptation or derivation from nature is referred to as ‘biomimetics’. Biomimetic motions are derived from the many different functional materials and/or intricate and highly organized structure of the biological material from the molecular to the nanoscale, microscale and macroscale. For example, the early developments of the wing design of airplanes was inspired by birds' several consecutive rows of covering flexible feathers on the wings, which develop the lift by movable flaps. The sandfish found in the Sahara desert moves over the sand. The scales on its body and biomaterials used in the body provide wear resistance. In addition, there are a large number of objects, including bacteria, plants, land and aquatic animals from nature and their selected functions will sever as the inspiration for various biomimetic motions.
An important characteristic of living matter is the intelligent behavioral response to external stimuli. In nature, the communication between living organisms is well-established and is essential for the ecosystems, which gives us a lot of inspirations.1–4 For example, geckos are able to crawl by the adhesion of tens of thousands of extremely fine bristle fibrils on the soles of their feet with the surface structure.5–8 Scyphomedusae ephyra, the juvenile of the most widely distributed jellyfish, can smartly control the fluidic flow around their body to realize diverse functionalities.9–13 Plants exhibit hydration-trigged changes in their morphology due to differences in local swelling behaviour that arise from the directional orientation of stiff cellulose fibrils within plant cell walls.14–17 Inspired by plants and animals in nature, a variety of stimuli-responsive materials and machines are fabricated to realize various functions and motion behaviors by mimicking the mechanisms and motion behaviors of various biological systems.18–21 The biomimetic behaviors could help or even replace the human body to perform a variety of functions, which will have a significant influence on society.22,23 For example, Whitesides et al.22 constructed the multi-legged robots that mimic some of the important musculoskeletal features of arthropods, which is well suited for safe robot–human interaction. In addition, R. V. Martinez et al.23 reported soft actuators and robots that can display remarkable resistance to mechanical damage. These soft robotic structures have the potential to be used in congested and hazardous spaces, which will protect humans from harm.
Materials with switchable mechanical properties are widespread in living organisms and endow many species with traits that are essential for their survival. Therefore, materials with stimuli-responsive properties are the foundation of achieving biomimetic motion. When stimuli-responsive materials are exposed to a highly specific or predefined stimulus, the materials are able to change their mechanical properties on command or stimulation. In addition to the stimuli-responsive materials, the design of the structure and the mechanism of movement are the key factors that determine the motion. Bioinspired design is a general approach of applying biological principles to develop new solutions.24–27 By fully understanding the motion mechanism of organisms, researchers design and realize biomimetic motion by taking advantage of the switchable mechanical properties of materials. It is instructive that most of the mechanically adaptive materials found in nature rely on simple mechanisms and building blocks. The structures of building elements and modulation of interactions are the key factors to achieve different and complex functions.28,29 Many examples of artificial stimuli-responsive materials being designed to mimic the motion behaviors of living things have been reported, with applications ranging from micro/nanomotors to soft robotics.30–42
This review is aimed to provide such a panoramic discussion of existing various biomimetic motions. Based on the motion mechanism of organisms, the intelligent materials/machines with specific functions are designed by making full use of the properties of stimulus-responsive materials. In this review, the motion behaviors from macroscopic to microscopic are divided into the following three categories (Fig. 1): (1) biomimetic motions based on micro/nanomotors. In this section, we mainly introduce various structures and driven modes of micro/nanomotors and the applications in biomedicine, environmental remediation and so on. What's more, the swarm behavior of micro/nano motors imitating organisms is also discussed. (2) Biomimetic motions based on actuators. In this section, the compositions and biomimetic behaviors (jumping, locomotion, walking, capturing, etc.) of actuators based on smart materials such as hydrogels, shape memory materials and liquid crystal elastomers are reviewed. (3) Biomimetic motions based on soft robotics. A variety of biomimetic motions, such as snake-like, tendril-like and underwater jellyfish-like have been designed, and the motion mechanisms and motion behaviors have been summarized in this section. We finally briefly review the opportunities and challenges of biomimetic motions.
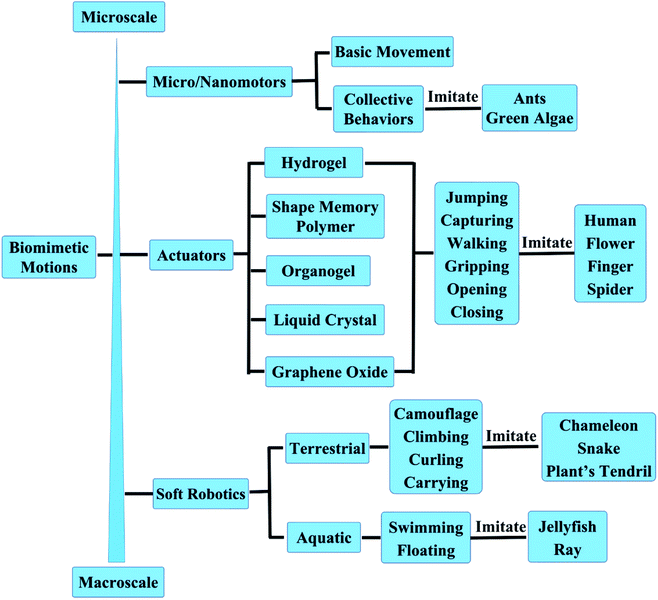 |
| Fig. 1 Overview of various biomimetic motions based on micro/nanomotors, actuators and soft robotics. | |
2. Biomimetic motions based on micro/nanomotors
2.1 Biomimetic behaviors of single micro/nanomotor
Micro/nanomotors are micro/nanoscale machines capable of converting different energies into mechanical energy that drives machinery movement. The energy sources can be chemical energy, derived from chemical reactions,43–47 or various sources of external stimuli (such as, light, magnetic, ultrasonic or electric field).48–52 Micro/nanomotors have been applied in a wide range of fields to perform specific tasks, including biomedical, environmental remediation, energy generation, and so on.53–71 Zhang et al.72 at the University of California San Diego developed the platelet-mimicking nanoparticles, which can deliver drugs to target sites of the body (Fig. 2A). Platelet membranes preferentially bind to damaged blood vessel walls, allowing nanoparticles to specifically deliver and release drugs to target sites of the body to treat cardiovascular disease and bacterial infections. This work addresses a major challenge in the field of nanomedicine. Considering the targeting ability, platelet-mimicking nanoparticles can deliver higher doses of drugs specifically to the affected area without flooding the whole body with drugs. This study also has wide implications for targeted therapies for other diseases, such as cancer and neurodegenerative diseases. This work is published in the journal Nature. Later Liangfang Zhang & Joseph Wang et al.73 present the first in vivo therapeutic micromotors application for active drug delivery to treat gastric bacterial infection in a mouse model using clarithromycin as a model antibiotic and Helicobacter pylori infection as a model disease, as shown in Fig. 2B. This work opens the door to the use of synthetic motors as an active-delivery platform for in vivo treatment of diseases and will likely trigger intensive research interests in this area.
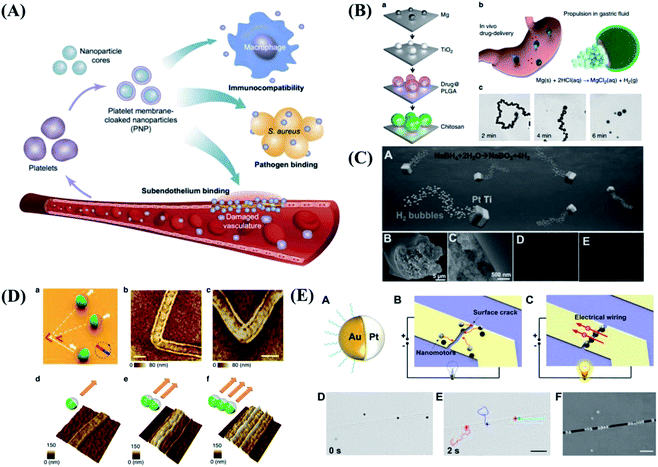 |
| Fig. 2 The biomimetic behaviors of micro/nanomotors. (A) Poly(lactic-co-glycolicacid) (PLGA) nanoparticles are enclosed entirely in plasma membrane derived from human platelets. Reproduced with permission from ref. 72; copyright 2015 Nature Publishing Group. (B) Schematic of in vivo propulsion and drug delivery of the Mg-based micromotors in a mouse stomach. Reproduced with permission from ref. 73; copyright 2017 Nature Publishing Group. (C) Self-propelled catalytic micromotors for energy generation. Reproduced with permission from ref. 74; copyright 2015 Wiley-VCH. (D) Controlled and parallel patterning by Janus sphere motors. Reproduced with permission from ref. 75; copyright 2014 Nature Publishing Group. (E) The artificial nanomotors autonomously seek and repair microscopic mechanical cracks. Reproduced with permission from ref. 76; copyright 2015 American Chemical Society. | |
The self-propulsion of micro/nanomotors facilitates fluid mixing and further accelerates chemical reactions. Therefore Wang et al.74 studied a new self-propelled catalytic micromotor-based strategy for rapid energy production from liquid fuels and demonstrate its capability for powering external devices, as shown in Fig. 2C. The new micromotor approach paves the way for the development of efficient on-site energy generation for powering external devices or meeting growing demands on the energy grid. The rapid miniaturization of devices and machines has fuelled the evolution of advanced fabrication techniques. However, the complexity and high cost of the state-of-the-art high-resolution lithographic systems are prompting unconventional routes for nanoscale patterning. Joseph Wang's group reported a new nano-patterning approach—nanomotor lithography.75 The motion trajectories of the nanomotors are transformed into controllable surface features. This nanomotor-fabrication strategy combines controlled movement with unique light focusing or blocking abilities for direct surface writing and provides researchers with considerable freedom for creating diverse features with different shapes and sizes through modular nanomotor design (Fig. 2D). This method provides a unique nanofabrication platform for creating diverse nanodevices. Inspired by biological self-healing processes, Wang et al.76 design and characterize a synthetic repair system where self-propelled nanomotors autonomously seek and localize at microscopic cracks and repair microscopic mechanical defects to restore the electrical conductivity of broken electronic pathways (Fig. 2E). Such a nanomotor-based repair system represents an important step toward the realization of biomimetic nanosystems that can autonomously sense and respond to environmental changes, a development that potentially can be expanded to a wide range of applications.
2.2 Collective behaviors of micro/nanomotors
In addition to the motion and application of a single micro/nanomotor, another important goal of micro/nanomotors is to mimicking those of live organisms. Organisms in nature can create highly complex collective behaviors through local interactions. The collective behaviors of flocking and swarming make organisms perform different tasks. The ability of micro/nanomotors to be aligned and move in a controlled manner will be important to study swarm behavior in nature, which allows us to explore complex assembly behavior in vivo. Micro/nanomotors are not only capable of responding to their environment but also adapting to its changes. As shown in Fig. 3A, researchers present a general strategy to reconfigure active particles into various collective states by introducing imbalanced interactions. The swarms, chains, clusters and isotropic gases from the same precursor particle were realized by changing the electric-field frequency.77 By learning from the natural world, He et al.78 present a reconfigurable, dynamic self-organization system based on acoustically actuated eutectic gal-lium–indium alloy liquid metal colloidal motors, inspired by the unique dynamic structure of dandelions. The liquid metal colloidal motors could dynamically self-organize to form striped, quasi-round and then flower-like clusters under subtle modulation of the acoustic frequency (Fig. 3B).
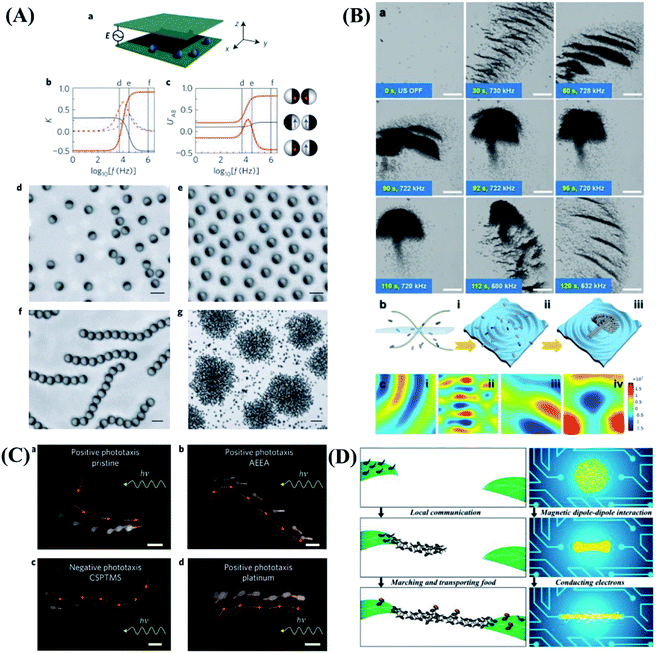 |
| Fig. 3 The collective behaviors of micro/nanomotors. (A) The collective active states formed by spheres under an electric field. Reproduced with permission from ref. 77; copyright 2016 Nature Publishing Group. (B) Acoustically triggered dandelion-like assembly of gal-lium–indium alloy colloidal motors. Reproduced with permission from ref. 78; copyright 2020 Wiley-VCH. (C) Light-driven nanotree-shaped micromotors simulate the group phototaxis behavior of green algae. Reproduced with permission from ref. 79; copyright 2016 Nature Publishing Group. (D) The generation and reversible elongation process of an ant bridge-mimicked microswarm under an oscillating magnetic field. Reproduced with permission from ref. 80; copyright 2019 American Chemical Society. | |
What's more, Tang et al.79 from the University of Hong Kong are inspired by the algae phototaxis, developed Janus nanotree-shaped micromotors. The photoelectric anode and photocathode of the micromotors release the cation and anion respectively under the illumination of UV light. The local electric field generated by the ion gradient can achieved the self-driven of micromotors. In addition, through chemical modification, the micromotors have the ability to sense the irradiation direction of external light source and realize positive and negative phototaxis, which successfully simulates the group phototaxis behavior of green algae, as shown in Fig. 3C. In addition to mimicking collective behavior, some specific functionality can be also implemented. For example, Zhang et al.80 realized a microswarm system that mimics the structure and function of an ant bridge by employing functionalized magnetite nanoparticles (Fig. 3D). Through the application of a programmed oscillating magnetic field, the magnetite nanoparticles are reconfigured into a ribbon-like microswarm, which can perform reversible elongation and then be capable of constructing a conductive pathway for electrons between two disconnected electrodes with the bodies of functionalized nanoparticles. Furthermore, the microswarm can also serve as a microswitch, repair broken microcircuits, and constitute flexible circuits, exhibiting a promising future for the practical applications in the electronics field.
3. Biomimetic motions based on actuators
3.1 Biomimetic behaviors based on hydrogel actuators
Responsive hydrogels have broad application prospects in soft actuators, artificial muscles, medical instruments and other fields. Hydrogel actuators are generally prepared by responsive hydrogels with asymmetric structure. Under the stimulation of electricity, heat, light and pH, the volume or shape of hydrogel actuators will change.81–87 Through the precise design of the structure, simple behaviors such as grasping, releasing, walking can be realized, as shown in Fig. 4. Muscles are typical flexible biological actuators, which produce powerful explosive force through contraction and rapid elongation to realize actions such as jumping. Inspired by this, Fu and his team accumulate the elastic energy by taking advantage of the constraint of substrate on the hydrogel deformation. Then the explosive release of energy can be achieved by using the interface instability, which further motivate controllable jumping of hydrogel bilayers (Fig. 4A).88 Origami-inspired folding techniques are widely used for realizing hydrogel microgrippers due to the convenience of planar microfabrication techniques. Sakar et al.89 introduced a universal soft robotic microgripper by utilizing programmable chemomechanical properties of double network hydrogels. The device was manufactured from a combination of natural and synthetic hydrogels. The gripping processes are illustrated in Fig. 4B. The soft robotic microgripper has the capability for tethered and untethered micromanipulation of a wide range of different objects including biological samples. In order to expand the utility and functionality of hydrogel actuators, it is advantageous to develop hydrogels that can respond to multiple stimuli and mimic the living systems for yielding new applications. Inspired by the biological color-changing capacity, considerable progress has been achieved in fabricating polymeric hydrogels with color-tunable behavior upon the external trigger. Chen et al.90 developed a convenient and general applicable strategy to fabricate an anisotropic hydrogel actuator with on–off switchable and color-tunable fluorescence behaviors (Fig. 4C). The shape of the actuator could be deformed via thermostimulus, which could control the on–off fluorescence behavior of the actuator. Moreover, the fluorescence color could be tuned through adjusting the pH. Therefore, shape deformation and fluorescence color-changing functions are integrated and optimized in one system. This method may provide some new strategies to explore smarter biomimetic systems coupling synergistic visually detecting and complexly actuating functions.
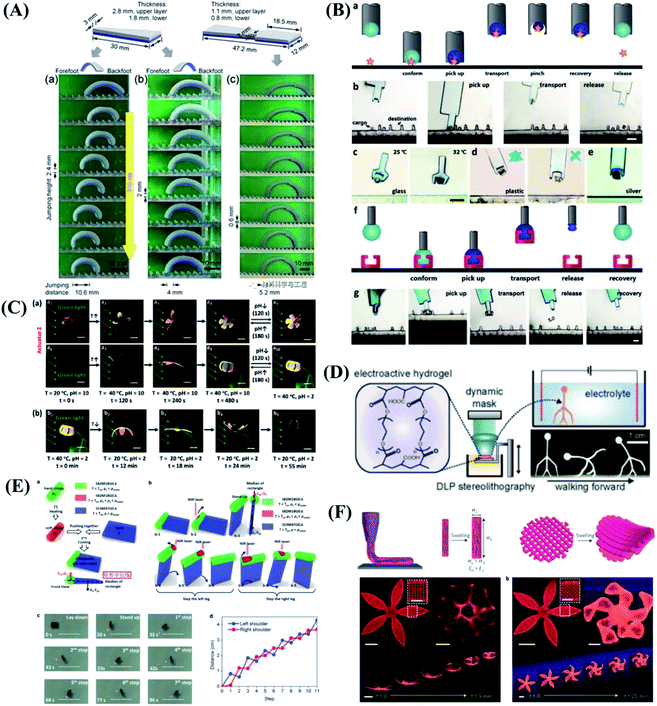 |
| Fig. 4 Biomimetic behaviors based on hydrogels. (A) Controlled jumping of hydrogel bilayer. Reproduced with permission from ref. 88; copyright 2018 American Chemical Society. (B) Transport of a wide range of microscopic objects by hydrogel microgripper. Reproduced with permission from ref. 89; copyright 2019 Wiley-VCH. (C) Thermoresponsive complex shape deformation and fluorescent color displaying of hydrogel actuator. Reproduced with permission from ref. 90; copyright 2018 Wiley-VCH. (D) The bi-directional locomotion of a human-like electroactive hydrogel structure. Reproduced with permission from ref. 93; copyright 2018 American Chemical Society. (E) The light-controlled “bipedal-like” walking motion in water of composite gel mini-robot. Reproduced with permission from ref. 91; copyright 2015 Wiley-VCH. (F) 4D print functional biomimetic hydrogel folding flower. Reproduced with permission from ref. 94; copyright 2016 Nature Publishing Group. | |
In addition to the above gripping, jumping, walking, etc. The “swimming” motions based on hydrogel in aqueous solutions have also been studied. Inspired by swimming bladders of fish, a real-time depth-controllable swimming hydrogel have been successfully prepared, as shown in Fig. 4E. Based on the swimming motion, rolling, somersaulting, and bipedal-like walking motions have been further realized by modulating and combining hydrogel shapes, different hydrogel compositions, and the location of NIR laser.91 In addition, W. Lu and T. Chen et al.92 construct artificial soft actuators with octopus-like synergistic shape/color change and directional locomotion behaviors. The artificial soft swimming robots can directional swimming motion. Other motions such as pick up and move objects by “finger” gripper and transporter in water can also be achieved by Lee et al.93 They presented soft robotic manipulation and locomotion with 3D printed electroactive hydrogels micro-structures. Through 3D design and precise dimensional control enabled by a digital light processing based micro 3D printing technique, bi-directional locomotion of a human-like electroactive hydrogel structure are achieved, as shown in Fig. 4D. The 3D printed electroactive hydrogels have a lot of water and are very soft. They can also be used in many different types of underwater devices to simulate aquatic creatures like octopuses.
Recent many efforts to create organism-inspired, shape-changing structures have employed differential swelling in isotropic or composite bilayers and hinges. However, none of these approaches enable shape change using a single material patterned in a one-step process, nor do they utilize a predictive model capable of tackling both the forward and inverse design problems. Lewis et al.94 developed a biomimetic hydrogel composite that can be 4D printed into programmable bilayer architectures patterned in space and time, which are encoded with localized swelling anisotropy that induces complex shape changes on immersion in water. Inspired by flower opening/closing, Lewis group printed petals in a floral form comprised of a bilayer lattice with a 90°/0° configuration, and see that the structure closes as it swells. When the petals are printed with the ink filaments oriented at −45°/45°, the resulting structure yields a twisted configuration, as shown in Fig. 4F. This study opens new avenues for creating designer shape-shifting architectures for tissue engineering, biomedical devices, soft robotics and beyond.
3.2 Biomimetic behaviors based on other actuators
Nature inspires scientists to realize artificial systems that mimic their natural counterparts in function, flexibility, and adaptation. The stimuli-responsive “smart” materials such as shape memory polymers, liquid crystal network, carbon nanotubes or reduced graphene oxide, and so on, that reversibly change their shape or property in response to external triggers, pave way for soft robotics and self-regulating devices.95–99 This depicts that for realization of manmade devices capable of mimicking the complex functionalities of natural species, the use of multi-responsive materials is one of the key design principles. Despite the significant research effort dedicated to hydrogel actuators, other stimuli-responsive materials and structures that can be triggered by light, magnetism and moisture have been studied.100 As shown in Fig. 5A, inspired by the human's flick finger behavior which can release large force output, a soft jumping robot mimicking the gymnast's somersault is designed based on the rolled carbon nanotube/polymer bilayer composite actuator.101 This new type of rolled bilayer actuator with tubular shape shows electrically and sunlight-induced actuation with versatile complex biomimetic motions, including ultralarge deformation from tubular to flat, light-induced tumbler with cyclic wobbling, electrically/light-induced crawling-type walking robots and gripper, and so on. These results open the way for using one simple type of actuator structure for the construction of various soft robots and devices toward practical biomimetic applications. Magnetic soft materials composed of magnetic particles in a soft polymer matrix have drawn great interest recently due to their untethered control for shape change, motion, and tunable mechanical properties.102–106 A novel magnetic shape memory polymer composite with integrated reprogrammable, untethered, fast, and reversible actuation and shape locking is reported.107 The integrated multifunctional shape manipulations offered by magnetic shape memory polymer can be exploited for a wide range of novel applications, including soft grippers for heavy loads, reconfigurable morphing antennas, and sequential logic circuits for digital computing, as shown in Fig. 5B. In analogy to animals, a biomimetic motor system inevitably relies on numerous actuators to be precisely assembled with man-made skeletons and judicious coordination. To solve the integration problem, a holistic artificial muscle with integrated light-addressable nodes, using one-step laser printing from a bilayer structure of poly(methyl methacrylate) and graphene oxide compounded with gold nanorods, is reported.108 Utilizing the synergistic effect of the gold nanorods with high plasmonic property and wavelength-selectivity as well as graphene with good flexibility and thermal conductivity, the artificial muscle can implement full-function motility including a light-assisted hand, light-driven Venus flytrap and light manipulation of the Venus flytrap robot, as shown in Fig. 5C.
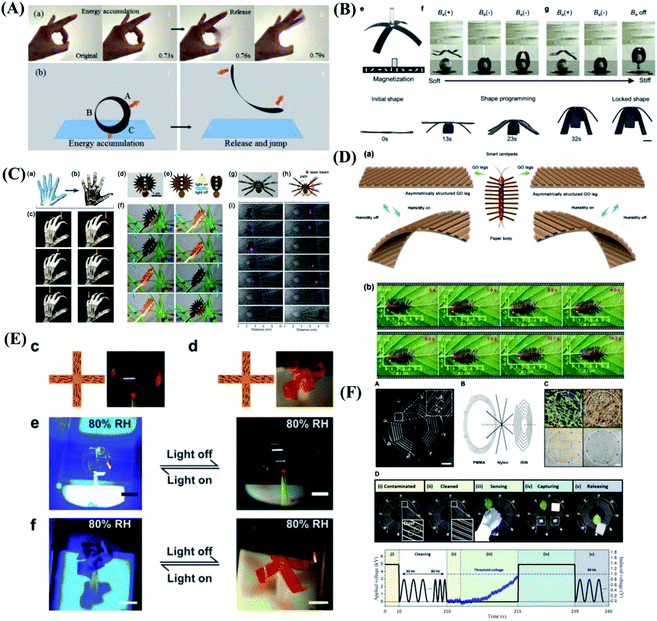 |
| Fig. 5 Biomimetic behaviors based on other stimuli-responsive materials. (A) The jumping of the rolled carbon nanotube/polymer bilayer composite actuator mimicking the flicking finger behavior. Reproduced with permission from ref. 101; copyright 2017 Wiley-VCH. (B) Sequential shape transforming and shape locking of magnetic shape memory polymer gripper. Reproduced with permission from ref. 107; copyright 2019 Wiley-VCH. (C) Plasmonic-assisted graphene oxide artificial mini robots. Reproduced with permission from ref. 108; copyright 2018 Wiley-VCH. (D) A centipede-like soft mini-robot based on the smart graphene oxide paper. Reproduced with permission from ref. 109; copyright 2019 Wiley-VCH. (E) The artificial liquid-crystal-network “flower” opens and closes mimicking some nocturnal flowers such as moon plant. Reproduced with permission from ref. 110; copyright 2018 Wiley-VCH. (F) The ionic spiderwebs based on ionically conductive and stretchable organogel exhibit the continuous process of contamination, cleaning, sensing, capturing, and releasing. Reproduced with permission from ref. 112; copyright 2020 Science Publishing Group. | |
In addition to thermal conductivity, graphene oxide have been considered extremely promising candidates for developing layered quantum confined superfluidics system due to their tunable interlayer-spacing and nanoporous structures. Therefore, inspired from the natural quantum-tunneling-fluidics-effect, Sun et al.109 reported quantum-confined-superfluidics-enabled moisture actuation of graphene oxide paper by introducing periodic gratings unilaterally. Sun's team designed and prepared two kinds of smart graphene oxide ribbons with chiral twisting behaviors as legs for assembling a centipede mini-robot. As shown in Fig. 5D, under moisture actuation, the smart centipede with chiral bending graphene oxide legs can crawl toward the right side. Additionally, a smart leaf capable of catching a living ladybug have been successfully fabricated. This work reveals great potential for developing graphene-based smart robots. To simplify structure and realize sophisticated control over the directionality of the movements. Liquid crystal networks, which combine the anisotropic properties of liquid crystals with the mechanical rigidity of polymer networks, providing stimuli-responsive anisotropic deformation of the network with movement direction governed by the alignment of the constituent liquid crystal molecules. Therefore gradually attracts researchers' interest.110,111 Schenning et al.110 take inspiration from the nocturnal flower and devise a monolithic liquid crystal network actuator whose movements are controlled by a delicate interdependence between light and humidity. The artificial nocturnal flower is devised that is closed under daylight conditions when the humidity level is low and/or the light level is high, while it opens in the dark when the humidity level is high, as shown in Fig. 5E. Scientists have been devoting to achieve complementary interactions in a single system. Young Kim & Jeong-Yun Sun et al.112 emulate the capturing strategies of a spider with a single pair of ionic threads based on ionically conductive and stretchable organogel. The ionic spiderwebs completed consecutive missions of cleaning contamination on itself, sensing approaching targets, capturing those targets, and releasing them, as shown in Fig. 5F. The ionic spiderwebs demonstrate the importance of learning from nature and push the boundaries of soft robotics in an attempt to combine mutually complementary functions into a single unit with a simple structure.
4. Biomimetic motions of soft robots
Scientists have long worked to develop soft robots made of soft material. Compared with traditional rigid robots, soft robots are not only able to work safer in existing conditions and prevent unnecessary damage, but their flexibility allows them to perform more functions, such as dexterity when manipulating objects, the ability to squeeze into narrow spaces or walk on uneven surfaces. Soft materials capable of transforming between three-dimensional (3D) shapes in response to stimuli such as light, heat, solvent, electric and magnetic fields have applications in diverse areas such as flexible electronics, soft robotics and biomedicine.113–118
4.1 Biomimetic motions of terrestrial soft robots
The soft robots are mainly composed of artificial muscles driven by intelligent soft materials, which have the characteristics of structure-drive integration. Soft robots can generate active body deformation when contacting the external environment and have high flexibility. In recent years, all kinds of new soft robots prototype systems such as soft grasping robot, soft crawling robot, soft camouflage robot, soft growth robot and soft mechanical fish, etc. have appeared in domestic and foreign laboratories.119–123 Inspired by cuttlefish and chameleons, they can change colors in different environments to adapt to their surroundings, forage for food, and send signals to their peers to protect themselves from being injured. To mimic these functions, Whitesides et al.124 designed a soft robot with camouflage and display functions. The system combines microfluidics, pattern, and color to provide both camouflage/display and movement of the soft machines. Illustrated in Fig. 6A are the operation of camouflage in a rock bed and a leaf-covered concrete slab using two different color layers filled with two different sets of colored solutions. In addition to mimicking the functions of color-changing animals, Zhu & Zhao et al.125 developed the first soft climbing robot consisting of dielectric-elastomers artificial muscles and electrostatic adsorption feet. The dielectric-elastomer artificial muscles drive fast periodic deformation of the soft robotic body, electroadhesive feet give spatiotemporally controlled adhesion of different parts of the robot on the wall, and the stable and fast climbing movement of the robot is realized by the cooperative control of the dielectric-elastomer artificial muscles and the electrostatic adsorption feet. The soft robots have abilities similar to living organisms, including climbing, crawling, turning and agile environmental adaptation, as shown in Fig. 6B. There are lots of potential applications for the soft robots, for example, dangerous environment exploring, disaster rescue as well as wall cleaning of vertical and narrow space. The skin of the vast majority of current soft robots consists of an unstructured flexible membrane that lacks directional frictional properties. As a result, multiple actuators activated independently are typically required to achieve locomotion. Inspired by the friction-assisted locomotion of snakes and by the recent advances in engineered surfaces with programmable tribological behavior. Bertoldi et al.126 designed highly stretchable kirigami surfaces in which mechanical instabilities induce a transformation from flat sheets to 3D-textured surfaces akin to the scaled skin of snakes, which enables a single soft actuator to propel itself. Bertoldi's group built a fully untethered kirigami-skinned soft crawler by integrating on-board control, sensing, actuation, and power supply (Fig. 6C).
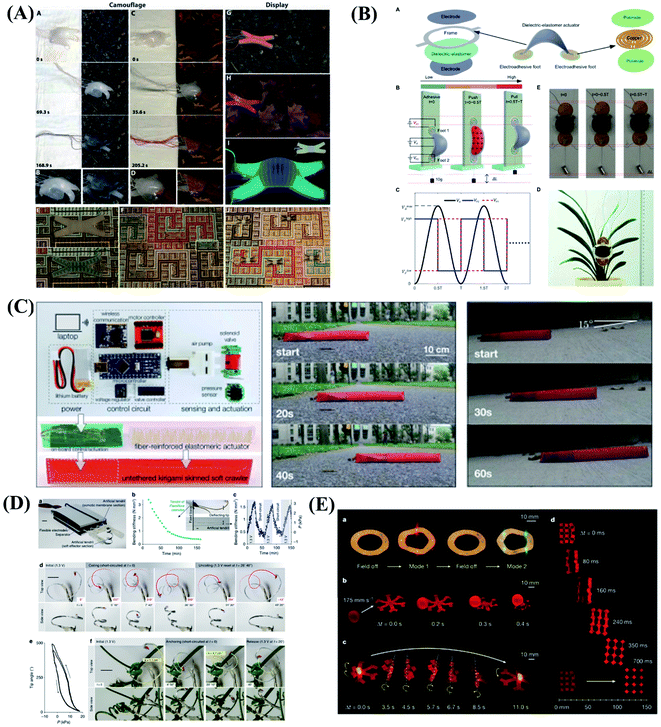 |
| Fig. 6 Biomimetic motions of soft robots. (A) Camouflage and display coloration of soft machines. Reproduced with permission from ref. 124; copyright 2012 Science Publishing Group. (B) Design and climbing of the soft wall-climbing robot. Reproduced with permission from ref. 125; copyright 2018 Science Publishing Group. (C) Untethered kirigami-skinned soft crawlers inspired by snakes. Reproduced with permission from ref. 126; copyright 2018 Science Publishing Group. (D) Reversible stiffness modulation and actuation of a tendril-like soft robot. Reproduced with permission from ref. 127 copyright 2019 Nature Publishing Group. (E) Functional demonstrations of 3D-printed soft materials and soft machines with programmed ferromagnetic domains. Reproduced with permission from ref. 128; copyright 2018 Nature Publishing Group. | |
In addition to imitating animals, plants also give us a lot of inspirations. Plants use a coordinated and reversible modulation of intracellular turgor (pressure) to tune their stiffness and achieve macroscopic movements. Mazzolai et al.127 exhibited the first soft robot that mimics plant tendrils, which show a reversible osmotic actuation strategy based on the electrosorption of ions on flexible porous carbon electrodes driven at low input voltage. The driving design was inspired entirely by the transport of water through plants. This soft robot mimics the principle of water transport in plants, and is able to curl and climb simply like a plant (Fig. 6D). This approach highlights the potential of plant-inspired technologies for developing soft robots based on biocompatible materials and safe voltages making them appealing for prospective applications. Although extensive efforts have been made in the design and fabrication of shape-programmable soft materials, fast and fully reversible actuation between targeted 3D shapes has remained a challenge. To address these limitations and challenges, Zhao's team at the Massachusetts Institute of Technology (MIT) first proposed printing programmable magnetic domain soft materials and soft machines that enable fast transformations between complex 3D shapes via magnetic actuation.128 This approach is based on direct ink writing of an elastomer composite containing ferromagnetic microparticles. By applying a magnetic field to the dispensing nozzle while printing, researchers reorient particles along the applied field to impart patterned magnetic polarity to printed filaments. 3D-printed soft materials demonstrate the capability to achieve diverse functions, such as interacting with an object based on the complex shape changes of the hexapedal structure, carrying and releasing an object on demand, as shown in Fig. 6E. This work also provides a good paradigm that combines effectively the material design and the structure design to achieve programmable shape transformations and even functional reconfiguration.
In biological systems, large-scale behavior can be achieved through swarm coupling and coordination of randomly moving small-scale components. Inspired by the biological mechanisms, Li et al.129 reported a collective robotic system in which deterministic locomotion is a result of the stochastic movement of many loosely coupled, disc-shaped components. The diameter of each component varies from 15.5 to 23.5 centimetres during the oscillations. Li and colleagues show that the system can achieve robust locomotion and object transport, as well as light-directed movement and obstacle avoidance. The results show that stochasticity offers a promising approach to developing large-scale, collective robotic systems that exhibit robust deterministic behaviour.
4.2 Biomimetic motions of aquatic soft robots
Except for the terrestrial environment, underwater biomimetic intelligence has been gradually developed in artificial integrated systems. Nature has provided us with inspiration for building intelligent underwater biomimetic systems. The soft robots built with some actuation methods such as biological muscle cells, shape memory alloys, hydraulic actuators, dielectric elastomers, hydrogels, and liquid crystal elastomers.130–133 Parker et al.134 reported the construction of a freely swimming jellyfish from chemically dissociated rat tissue and silicone polymer. The constructs, termed ‘medusoids’, were designed with computer simulations and experiments to match key determinants of jellyfish propulsion and feeding performance by quantitatively mimicking structural design, stroke kinematics and animal–fluid interactions. As shown in Fig. 7A, jellyfish swimming, a well-defined animal behavior, has been successfully implemented.
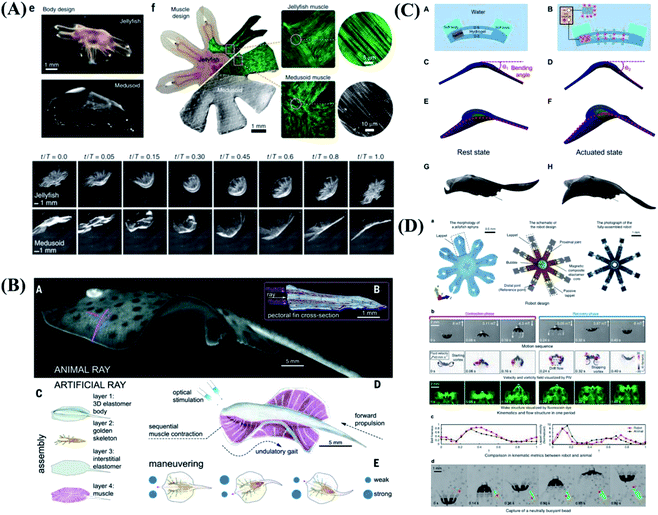 |
| Fig. 7 Biomimetic motions of underwater soft robots. (A) The design and free-swimming of jellyfish. Reproduced with permission from ref. 134; copyright 2012 Nature Publishing Group. (B) Fast-moving soft electronic fish. Reproduced with permission from ref. 136; copyright 2017 Science Publishing Group. (C) Bioinspired tissue-engineered ray driven by cardiomyocytes. Reproduced with permission from ref. 135; copyright 2016 Science Publishing Group. (D) Design and swimming behavior of the jellyfish-inspired swimming soft millirobot. Reproduced with permission from ref. 138; copyright 2019 Nature Publishing Group. | |
At above stage, swimming behavior is limited to exactly one stereotypic mode of execution because local muscle contraction is difficult to achieve. Faced with the problems of complex driving device, low driving efficiency and lack of controllable motion control, Parker et al.135 was inspired by the relatively simple morphological blueprint provided by batoid fish such as stingrays and skates, created a biohybrid system that enables an artificial animal-a tissue-engineered ray-to swim and phototactically follow a light cue. The light-sensitive cardiomyocytes are integrated into the artificial ray body as driving components, and the contraction of cardiomyocytes is controlled by light to achieve the controlled movement of the bionic ray (Fig. 7C). The grand challenge lies in achieving self-powered soft robots with high mobility, environmental tolerance, and long endurance. Tiefeng Li & Zhilong Huang et al.136,137 developed a soft electronic fish with a fully integrated onboard system for power and remote control. Without any motor, the fish is driven solely by a soft electroactive structure made of dielectric elastomer and ionically conductive hydrogel, as shown in Fig. 7B. The fish shows consistent performance in a wide temperature range and permits stealth sailing due to its nearly transparent nature. The functionalities of the untethered miniature swimming robots significantly decrease as the robot size becomes smaller, due to limitations of feasible miniaturized on-board components. Sitti et al.138 proposed an untethered jellyfish-inspired soft millirobot that could realize multiple functionalities in moderate Reynolds number by producing diverse controlled fluidic flows around its body using its magnetic composite elastomer lappets, which are actuated by an external oscillating magnetic field. The soft millirobot can achieve diverse physical functions and robotic tasks by manipulating the fluidic flow around the robot's lappets, as shown in Fig. 7D. The proposed lappet kinematics can inspire other existing jellyfish-like robots to achieve similar functionalities at the same length and time scale.
5. Conclusions and outlook
In conclusion, an important characteristic of living organisms is the intelligent response to external stimuli. Building stimuli-responsive materials and machines could help or even replace the human body to perform a variety of functions, which will have a significant influence on society. The appearance of bionic technology provides a new idea for our research and provides a reference for designing excellent micro/nanomotors, actuators and soft robotics. Up to date there are a large number of methods developed to use smart response materials to prepare various micro/nanomotors, actuators and soft robotics driven by chemical/biochemical reactions and external fields, which then are used to mimic the motion behaviors of organisms (Table 1). Remarkable progresses have been made in both understanding and controlling the motion behaviors based on micro/nanomotors, actuators and soft robotics. The extensive research into biomimetic motions from nano-to macroscales has achieved many key functions of organisms, such as capturing, jumping, swimming, collective behavior and so on. However, there are still many challenges for biomimetic motions, including the limitation of the practical application environment, the same active deformation ability as the creatures in nature, and the difficulty of high maneuvering and flexible motion. Therefore, extensive efforts will still be made to biomimetic motions based on micro/nanomotors, actuators and soft robotics in innovative ways.
Table 1 A brief summary of biomimetic motions based on micro/nanomotors, actuators and soft robotics
Categories |
Typical examples |
Power sources |
Motion behaviors |
Limitations |
Micro/nanomotors |
Micro/nanorods, micro/nanoparticles, micro/nanotubes |
Chemical reaction, light, magnetic, ultrasonic, electric |
Movement, collective behaviors |
Precise regulation of motions and performance of specific tasks in special environments cannot be achieved. |
Actuators |
Bilayer structures, gripper, flower, etc. |
Light, electronic, magnetic, temperature |
Flicking, jumping, walking, etc. |
Soft robotics |
Soft grasping robot, soft crawling robot, soft camouflage robot, soft growth robot and soft mechanical fish |
Biological muscle cells, osmotic actuation, friction, light, electronic, magnetic, etc. |
Swimming, grasping, crawling, releasing, curling |
In the future, with the further development of science and technology, with the more research of perception, special functional structure, motion mechanics and motion control, we believe it is possible to realize truly perception and movement in the real environment, and which will be applied to industrial and agricultural production and benefit mankind.
Conflicts of interest
There are no conflicts to declare.
Acknowledgements
This work was supported by National Natural Science Foundation of China (No. 51905526), Jiaxing Science and Technology Project (No. 2020AY10018), Open Project Program of Key Laboratory of Yarn Materials Forming and Composite Processing Technology of Zhejiang Province (No. MTC 2020-04), National College Students Innovation and Entrepreneurship Training Program (No. 202110354002).
References
- D. T. Hughes and V. Sperandio, Nat. Rev. Microbiol., 2008, 6, 111–120 CrossRef CAS PubMed
. - C. M. Waters and B. L. Bassler, Annu. Rev. Cell Dev. Biol., 2005, 21, 319–346 CrossRef CAS PubMed
. - S. A. West, A. S. Griffin, A. Gardner and S. P. Diggle, Nat. Rev. Microbiol., 2006, 4, 597–607 CrossRef CAS PubMed
. - L. B. Buck, Cell, 2000, 100, 611–618 CrossRef CAS PubMed
. - L. F. Boesel, C. Greiner, E. Arzt and A. del Campo, Adv. Mater., 2010, 22, 2125–2137 CrossRef CAS PubMed
. - C. Zhang, D. A. Mcadams and J. C. Grunlan, Adv. Mater., 2016, 28, 6292–6321 CrossRef CAS PubMed
. - M. T. Northen, C. Greiner, E. Arzt and K. L. Turner, Adv. Mater., 2008, 20, 3905–3909 CrossRef CAS
. - D.-M. Drotlef, M. Amjadi, M. Yunusa and M. Sitti, Adv. Mater., 2017, 29, 1701353 CrossRef PubMed
. - M. J. McHenry and J. Jed, Exp. Biol., 2003, 206, 4125–4137 CrossRef PubMed
. - T. Blough, S. P. Colin, J. H. Costello and A. C. Marques, Biol. Bull., 2011, 220, 6–14 CrossRef PubMed
. - R. M. Nagata, A. C. Morandini, S. P. Colin, A. E. Migotto and J. H. Costello, Mar. Ecol.: Prog. Ser., 2016, 557, 145–159 CrossRef
. - K. E. Feitl, A. F. Millett, S. P. Colin, J. O. Dabiri and J. H. Costello, Biol. Bull., 2009, 217, 283–291 CrossRef CAS PubMed
. - J. C. Nawroth and J. O. Dabiri, Phys. Fluids, 2014, 26, 091108 CrossRef
. - Y. Forterre, J. Skotheim, J. Dumais and L. Mahadevan, Nature, 2005, 433, 421–425 CrossRef CAS PubMed
. - E. Reyssat and L. Mahadevan, J. R. Soc., Interface, 2009, 6, 951–957 CrossRef CAS PubMed
. - S. Armon, E. Efrati, R. Kupferman and E. Sharon, Science, 2011, 333, 1726–1730 CrossRef CAS PubMed
. - P. Fratzl and I. Burgert, Philos. Trans. R. Soc., A, 2009, 6, 1541–1557 Search PubMed
. - Y. Forterre, J. M. Skotheim, J. Dumais and L. Mahadevan, Nature, 2005, 433, 421–425 CrossRef CAS PubMed
. - J. R. Capadona, K. Shanmuganathan, D. J. Tyler, S. J. Rowan and C. Weder, Science, 2008, 319, 1370–1374 CrossRef CAS PubMed
. - A. W. Feinberg, A. Feigel, S. S. Shevkoplyas, S. Sheehy, G. M. Whitesides and K. K. Parker, Science, 2007, 317, 1366–1370 CrossRef CAS PubMed
. - S. Iamsaard, S. J. Aßhoff, B. Matt, T. Kudernac, J. J. L. M. Cornelissen, S. P. Fletcher and N. Katsonis, Nat. Chem., 2014, 6, 229–235 CrossRef CAS PubMed
. - A. Nemiroski, Y. Y. Shevchenko, A. A. Stokes, B. Unal, A. Ainla, S. Albert, G. Compton, E. MacDonald, Y. Schwab, C. Zellhofer and G. M. Whitesides, Soft Robot., 2017, 4, 1–8 CrossRef PubMed
. - R. V. Martinez, A. C. Glavan, C. Keplinger, A. I. Oyetibo and G. M. Whitesides, Adv. Funct. Mater., 2014, 24, 3003–3010 CrossRef CAS
. - N. F. Lepora, P. Verschure and T. J. Prescott, Bioinspiration Biomimetics, 2013, 8, 013001 CrossRef PubMed
. - S. Bauer, S. Bauer-Gogonea, I. Graz, M. Kaltenbrunner, C. Keplinger and R. Schwödiauer, Adv. Mater., 2014, 26, 149–162 CrossRef CAS PubMed
. - R. Schirhagl, C. Weder, J. Lei, C. Werner and H. M. Textor, Chem. Soc. Rev., 2016, 45, 234–236 RSC
. - C. Heinzmann, C. Weder and L. M. de Espinosa, Chem. Soc. Rev., 2016, 45, 342–358 RSC
. - N. D. Wanasekara and L. T. J. Korley, J. Polym. Sci., Part B: Polym. Phys., 2013, 51, 463–467 CrossRef CAS
. - A. R. Studart, Adv. Mater., 2012, 24, 5024–5044 CrossRef CAS PubMed
. - A. R. Studart, Angew. Chem., Int. Ed., 2015, 54, 3400–3416 CrossRef CAS PubMed
. - R. Dong, Q. Zhang, W. Gao, A. Pei and B. Ren, ACS Nano, 2015, 10, 839–844 CrossRef PubMed
. - Z. Wu, T. Si, W. Gao, X. Lin, J. Wang and Q. He, Small, 2016, 12, 577–582 CrossRef CAS PubMed
. - C. Chen, F. Mou, L. Xu, S. Wang, J. Guan, Z. Feng, Q. Wang, L. Kong, W. Li and J. Wang, Adv. Mater., 2017, 29, 1603374 CrossRef PubMed
. - S. Sánchez, L. Soler and J. Katuri, Angew. Chem., Int. Ed., 2015, 54, 1414–1444 CrossRef PubMed
. - J. Palacci, S. Sacanna, A. P. Steinberg, D. J. Pine and P. M. Chaikin, Science, 2013, 339, 936–940 CrossRef CAS PubMed
. - D. Kagan, P. Calvo-Marzal, S. Balasubramanian, S. Sattayasamitsathit, K. M. Manesh, G.-U. Flechsig and J. Wang, J. Am. Chem. Soc., 2009, 131, 12082–12083 CrossRef CAS PubMed
. - F. Peng, Y. Tu, J. C. M. van Hest and D. A. Wilson, Angew. Chem., Int. Ed., 2015, 54, 11662–11665 CrossRef CAS PubMed
. - J. Shintake, S. Rosset, B. Schubert, D. Floreano and H. Shea, Adv. Mater., 2016, 28, 231–238 CrossRef CAS PubMed
. - J. H. Pikul, S. Li, H. Bai, R. T. Hanlon, I. Cohen and R. F. Shepherd, Science, 2017, 358, 210–214 CrossRef CAS PubMed
. - E. W. Hawkes, L. H. Blumenschein, J. D. Greer and A. M. Okamura, Sci. Robot., 2017, 2, eaan3028 CrossRef PubMed
. - M. S. Verma, A. Ainla, D. Yang, D. Harburg and G. M. Whitesides, Soft Robot., 2018, 5, 133–137 CrossRef PubMed
. - C. Christianson, N. N. Goldberg, D. D. Deheyn, S. Cai and M. T. Tolley, Sci. Robot., 2018, 3, eaat1893 CrossRef PubMed
. - X. Ma, A. Jannasch, U.-R. Albrecht, K. Hahn, A. MiguelLópez, E. Schäffer and S. Sánchez, Nano Lett., 2015, 15, 7043–7050 CrossRef PubMed
. - M. Uygun, V. V. Singh, K. Kaufmann, D. A. Uygun, S. D. DeOliveira and J. Wang, Angew. Chem., Int. Ed., 2015, 54, 12900–12904 CrossRef CAS PubMed
. - V. V. Singh, F. Soto, K. Kaufmann and J. Wang, Angew. Chem., Int. Ed., 2015, 54, 6896–6899 CrossRef CAS PubMed
. - Z. Wu, J. Li, B. E.-F. Ávila, T. Li, W. Gao, Q. He, L. Zhang and J. Wang, Adv. Funct. Mater., 2015, 26, 7497–7501 CrossRef
. - S. Sanchez, L. Soler and J. Katuri, Angew. Chem., Int. Ed., 2015, 54, 1414–1444 CrossRef CAS PubMed
. - T. Xu, W. Gao, L.-P. Xu, X. Zhang and S. Wang, Adv. Mater., 2017, 29, 1603250 CrossRef PubMed
. - L. Xu, F. Mou, H. Gong, M. Luo and J. Guan, Chem. Soc. Rev., 2017, 46, 6905–6926 RSC
. - T. Xu, L. Xu and X. Zhang, Appl. Mater. Today, 2017, 9, 493–503 CrossRef
. - Y. Tu, F. Peng and D. A. Wilson, Adv. Mater., 2017, 29, 1701970 CrossRef PubMed
. - H. Chen, Q. Zhao and X. Du, Micromachines, 2018, 9, 41 CrossRef PubMed
. - W. Gao and J. Wang, ACS Nano, 2014, 8, 3170–3180 CrossRef CAS PubMed
. - M. Guix, J. Orozco, M. Garcia, W. Gao, S. Sattayasamitsathit, A. Merkoci, A. Escarpa and J. Wang, ACS Nano, 2012, 6, 4445–4451 CrossRef CAS PubMed
. - L. Soler and S. Sanchez, Nanoscale, 2014, 6, 7175–7182 RSC
. - L. Soler, V. Magdanz, V. M. Fomin, S. Sanchez and O. G. Schmidt, ACS Nano, 2013, 7, 9611–9620 CrossRef CAS PubMed
. - J. G. Moo and M. Pumera, Chem.–Eur. J., 2015, 21, 58–72 CrossRef CAS PubMed
. - J. Orozco, D. Vilela, G. Valdes-Ramirez, Y. Fedorak, A. Escarpa, R. Vazquez-Duhalt and J. Wang, Chem. - Eur. J., 2014, 20, 2866–2871 CrossRef CAS PubMed
. - D. Kagan, R. Laocharoensuk, M. Zimmerman, C. Clawson, S. Balasubramanian, D. Kang, D. Bishop, S. Sattayasamitsathit, L. Zhang and J. Wang, Small, 2010, 6, 2741–2747 CrossRef CAS PubMed
. - W. Gao, D. Kagan, O. S. Pak, C. Clawson, S. Campuzano, E. Chuluun-Erdene, E. Shipton, E. E. Fullerton, L. Zhang and E. Lauga, Small, 2012, 8, 460–467 CrossRef CAS PubMed
. - Z. Wu, Y. Wu, W. He, X. Lin, J. Sun and Q. He, Angew. Chem., Int. Ed., 2013, 52, 7000–7003 CrossRef CAS PubMed
. - W. Gao and J. Wang, Nanoscale, 2014, 6, 10486–10494 RSC
. - R. Mhanna, F. Qiu, L. Zhang, Y. Ding, K. Sugihara, M. Zenobi-Wong and B. J. Nelson, Small, 2014, 10, 1953–1957 CrossRef CAS PubMed
. - D. Patra, S. Sengupta, W. Duan, H. Zhang, R. Pavlick and A. Sen, Nanoscale, 2013, 5, 1273–1283 RSC
. - T. Laurell, F. Petersson and A. Nilsson, Chem. Soc. Rev., 2007, 36, 492–506 RSC
. - F. Kuralay, S. Sattayasamitsathit, W. Gao, A. Uygun, A. Katzenberg and J. Wang, J. Am. Chem. Soc., 2012, 134, 15217–15220 CrossRef CAS PubMed
. - S. Kim, F. Qiu, S. Kim, A. Ghanbari, C. Moon, L. Zhang, B. J. Nelson and H. Choi, Adv. Mater., 2013, 25, 5863–5868 CrossRef CAS PubMed
. - V. Marx, Nat. Methods, 2014, 12, 41–44 CrossRef PubMed
. - F. Guo, P. Li, J. B. French, Z. Mao, H. Zhao, S. Li, N. Nama, J. R. Fick, S. J. Benkovic and T. L. Huang, Proc. Natl. Acad. Sci. U. S. A., 2015, 112, 43–48 CrossRef CAS PubMed
. - S. Balasubramanian, D. Kagan, C. M. Hu, S. Campuzano, M. J. Lobo-Castanon, N. Lim, D. Y. Kang, M. Zimmerman, L. Zhang and J. Wang, Angew. Chem., Int. Ed., 2011, 50, 4161–4164 CrossRef CAS PubMed
. - J. Wang and W. Gao, ACS Nano, 2012, 6, 5745–5751 CrossRef CAS PubMed
. - C.-M. J. Hu, R. H. Fang, K.-C. Wang, B. T. Luk, S. Thamphiwatana, D. Dehaini, P. Nguyen, P. Angsantikul, C. H. Wen, A. V. Kroll, C. Carpenter, M. Ramesh, V. Qu, S. H. Patel, J. Zhu, W. Shi, F. M. Hofman, T. C. Chen, W. Gao, K. Zhang, S. Chien and L. Zhang, Nature, 2015, 526, 118–121 CrossRef CAS PubMed
. - B. E.-F. de Ávila, P. Angsantikul, J. Li, M. A. Lopez-Ramirez, D. E. Ramírez-Herrera, S. Thamphiwatana, C. Chen, J. Delezuk, R. Samakapiruk, V. Ramez, L. Zhang and J. Wang, Nat. Commun., 2017, 8, 272 CrossRef PubMed
. - V. V. VSingh, F. Soto, K. Kaufmann and J. Wang, Angew. Chem., Int. Ed., 2015, 127, 1–5 CrossRef
. - J. Li, W. Gao, R. Dong, A. Pei, S. Sattayasamitsathit and J. Wang, Nat. Commun., 2014, 5, 5026 CrossRef CAS PubMed
. - J. Li, O. E. Shklyaev, T. Li, W. Liu, H. Shum, I. Rozen, A. C. Balazs and J. Wang, Nano Lett., 2015, 15, 7077–7085 CrossRef CAS PubMed
. - J. Yan, M. Han, J. Zhang, C. Xu, E. Luijten and S. Granick, Nat. Mater., 2016, 15, 1095–1099 CrossRef CAS PubMed
. - Z. Li, H. Zhang, D. Wang, C. Gao, M. Sun, Z. Wu and Q. He, Angew. Chem., Int. Ed., 2020, 132, 20056–20060 CrossRef
. - B. Dai, J. Wang, Z. Xiong, X. Zhan, W. Dai, C.-C. Li, S.-P. Feng and J. Tang, Nat. Nanotechnol., 2016, 11, 1087–1092 CrossRef CAS PubMed
. - D. Jin, J. Yu, K. Yuan and L. Zhang, ACS Nano, 2019, 13, 5999–6007 CrossRef CAS PubMed
. - P. Calvert, Adv. Mater., 2009, 21, 743–756 CrossRef CAS
. - A. Shastri, L. M. McGregor, Y. Liu, V. Harris, H. Nan, M. Mujica, Y. Vasquez, A. Bhattacharya, Y. Ma, M. Aizenberg, O. Kuksenok, A. C. Balazs, J. Aizenberg and X. He, Nat. Chem., 2015, 7, 447–454 CrossRef CAS PubMed
. - S. Y. Chin, Y. C. Poh, A.-C. Kohler, J. T. Compton, L. L. Hsu, K. M. Lau, S. Kim, B. W. Lee, F. Y. Lee and S. K. Sia, Sci. Rob., 2017, 2, eaah6451 CrossRef PubMed
. - C. Keplinger, J.-Y. Sun, C. C. Foo, P. Rothemund, G. M. Whitesides and Z. Suo, Science, 2013, 341, 984–987 CrossRef CAS PubMed
. - Y. Kim, H. Yuk, R. Zhao, S. A. Chester and X. Zhao, Nature, 2018, 558, 274–279 CrossRef CAS PubMed
. - E. Palleau, D. Morales, M. D. Dickey and O. D. Velev, Nat. Commun., 2013, 4, 2257 CrossRef PubMed
. - L. Ionov, Adv. Funct. Mater., 2013, 23, 4555–4570 CrossRef CAS
. - G. Gao, Z. Wang, D. Xu, L. Wang, T. Xu, H. Zhang, J. Chen and J. Fu, ACS Appl. Mater. Interfaces, 2018, 10, 41724–41731 CrossRef CAS PubMed
. - H. Jia, E. Mailand, J. Zhou, Z. Huang, G. Dietler, J. M. Kolinski, X. Wang and M. S. Sakar, Small, 2019, 15, 1803870 CrossRef PubMed
. - C. Ma, W. Lu, X. Yang, J. He, X. Le, L. Wang, J. Zhang, M. J. Serpe, Y. Huang and T. Chen, Adv. Funct. Mater., 2018, 28, 1704568 CrossRef
. - L. Wang, Y. Liu, Y. Cheng, X. Cui, H. Lian, H. Liang, F. Chen, H. Wang, W. Guo, H. Li, M. Zhu and H. Ihara, Adv. Sci., 2015, 2, 1500084 CrossRef PubMed
. - S. Wu, H. Shi, W. Lu, S. Wei, H. Shang, H. Liu, M. Si, S. Le, G. Yin, P. Theato and T. Chen, Angew. Chem., Int. Ed., 2021, 60 DOI:10.1002/anie.202107281
. - D. Han, C. Farino, C. Yang, T. Scott, D. Browe, W. Choi, J. W. Freeman and H. Lee, ACS Appl. Mater. Interfaces, 2018, 10, 17512–17518 CrossRef CAS PubMed
. - A. S. Gladman, E. A. Matsumoto, R. G. Nuzzo, L. Mahadevan and J. A. Lewis, Nat. Mater., 2016, 15, 413–418 CrossRef PubMed
. - S. I. Rich, R. J. Wood and C. Majidi, Nat. Electron., 2018, 1, 102–112 CrossRef
. - L. Hines, K. Petersen, G. Z. Lum and M. Sitti, Adv. Mater., 2017, 29, 1603483 CrossRef PubMed
. - S. Maeda, Y. Hara, T. Sakai, R. Yoshida and S. Hashimoto, Adv. Mater., 2007, 19, 3480–3484 CrossRef CAS
. - X. He, M. Aizenberg, O. Kuksenok, L. D. Zarzar, A. Shastri, A. C. Balazs and J. Aizenberg, Nature, 2012, 487, 214–218 CrossRef CAS PubMed
. - H. Zeng, O. M. Wani, P. Wasylczyk, R. Kaczmarek and A. Priimagi, Adv. Mater., 2017, 29, 1701814 CrossRef PubMed
. - M. A. C. Stuart, W. T. S. Huck, J. Genzer, M. Müller, C. Ober, M. Stamm, G. B. Sukhorukov, I. Szleifer, V. V. Tsukruk, M. Urban, F. Winnik, S. Zauscher, I. Luzinov and S. Minko, Nat. Mater., 2010, 9, 10–113 CrossRef PubMed
. - Y. Hu, J. Liu, L. Chang, L. Yang, A. Xu, K. Qi, P. Lu, G. Wu, W. Chen and Y. Wu, Adv. Funct. Mater., 2017, 27, 1704388 CrossRef
. - J. Kim, S. E. Chung, S.-E. Choi, H. Lee, J. Kim and S. Kwon, Nat. Mater., 2011, 10, 747–752 CrossRef CAS PubMed
. - V. Q. Nguyen, A. S. Ahmed and R. V. Ramanujan, Adv. Mater., 2012, 24, 4041–4054 CrossRef CAS PubMed
. - W. Hu, G. Z. Lum, M. Mastrangeli and M. Sitti, Nature, 2018, 554, 81–85 CrossRef CAS PubMed
. - J. Yuan, W. Neri, C. Zakri, P. Merzeau, K. Kratz, A. Lendlein and P. PPoulin, Science, 2019, 365, 155–158 CrossRef CAS PubMed
. - Y.-F. Zhang, N. Zhang, H. Hingorani, N. Ding, D. Wang, C. Yuan, B. Zhang, G. Gu and Q. Ge, Adv. Funct. Mater., 2019, 29, 1806698 CrossRef
. - Q. Ze, X. Kuang, S. Wu, J. Wong, S. M. Montgomery, R. Zhang, J. M. Kovitz, F. Yang, H. J. Qi and R. Zhao, Adv. Mater., 2019, 31, 1906657 Search PubMed
. - B. Han, Y.-L. Zhang, L. Zhu, Y. Li, Z.-C. Ma, Y.-Q. Liu, X.-L. Zhang, X.-W. Cao, Q.-D. Chen, C.-W. Qiu and H.-B. Sun, Adv. Mater., 2018, 30, 1806386 CrossRef PubMed
. - Y.-L. Zhang, Y.-Q. Liu, D.-D. Han, J.-N. Ma, D. Wang, X.-B. Li and H.-B. Sun, Adv. Mater., 2019, 31, 1901585 CrossRef PubMed
. - O. M. Wani, R. Verpaalen, H. Zeng, A. Priimagi and A. P. H. J. Schenning, Adv. Mater., 2018, 30, 1805985 Search PubMed
. - R. Lan, Y. Gao, C. Shen, R. Huang, J. Bao, Z. Zhang, Q. Wang, L. Zhang and H. Yang, Adv. Funct. Mater., 2021, 31, 2010578 CrossRef CAS
. - Y. Lee, W. J. Song, Y. Jung, H. Yoo, M.-Y. Kim, H.-Y. Kim and J.-Y. Sun, Sci. Robot., 2020, 5, eaaz5405 CrossRef PubMed
. - M. Ma, L. Guo, D. G. Anderson and R. Langer, Science, 2013, 339, 186–189 CrossRef CAS PubMed
. - M. Zarek, M. Layani, I. Cooperstein, E. Sachyani, D. Cohn and S. Magdassi, Adv. Mater., 2016, 28, 4449–4454 CrossRef CAS PubMed
. - M. Wehner, R. L. Truby, D. J. Fitzgerald, B. Mosadegh, G. M. Whitesides, A. Jennifer, J. A. Lewis and R. J. Wood, Nature, 2016, 536, 451–455 CrossRef CAS PubMed
. - X. Zhao, J. Kimb, C. A. Cezarb, N. Huebsch, K. Lee, K. Bouhadir and D. J. Mooney, Proc. Natl. Acad. Sci. U. S. A., 2011, 108, 67–72 CrossRef CAS PubMed
. - S. Fusco, M. S. Sakar, S. Kennedy, C. Peters, R. Bottani, F. Starsich, A. Mao, G. A. Sotiriou, S. Pané, S. E. Pratsinis, D. Mooney and B. J. Nelson, Adv. Mater., 2014, 26, 952–957 CrossRef CAS PubMed
. - K. A. Davis, K. A. Burke, P. T. Mather and J. H. Henderson, Biomaterials, 2011, 32, 2285–2293 CrossRef CAS PubMed
. - R. F. Shepherd, F. Ilievski, W. Choi, S. A. Morin, A. A. Stokes, A. D. Mazzeo, X. Chen, M. Wang and G. M. Whitesides, Proc. Natl. Acad. Sci. U. S. A., 2011, 108, 20400–20403 CrossRef CAS PubMed
. - S. Palagi, A. G. Mark, S. Y. Reigh, K. Melde, T. Qiu, H. Zeng, C. Parmeggiani, D. Martella, A. Sanchez-Castillo, N. Kapernaum, F. Giesselmann, D. S. Wiersma, E. Lauga and P. Fischer, Nat. Mater., 2016, 15, 647–653 CrossRef CAS PubMed
. - D. Rus and M. T. Tolley, Nature, 2015, 521, 467–475 CrossRef CAS PubMed
. - B. Mosadegh, P. Polygerinos, C. Keplinger, S. Wennstedt, R. F. Shepherd, U. Gupta, J. Shim, K. Bertoldi, C. J. Walsh and G. M. Whitesides, Adv. Funct. Mater., 2014, 24, 2163–2170 CrossRef CAS
. - C. Majidi, Soft Robot., 2014, 1, 5–11 CrossRef
. - S. A. Morin, R. F. Shepherd, S. W. Kwok, A. A. Stokes, A. Nemiroski and G. M. Whitesides, Science, 2012, 337, 828–832 CrossRef CAS PubMed
. - G. Gu, J. Zou, R. Zhao, X. Zhao and X. Zhu, Sci. Robot., 2018, 3, eaat2874 CrossRef PubMed
. - A. Rafsanjani, Y. Zhang, B. Liu, S. M. Rubinstein and K. Bertoldi, Sci. Robot., 2018, 3, eaar7555 CrossRef PubMed
. - I. Must, E. Sinibaldi and B. Mazzolai, Nat. Commun., 2019, 10, 344 CrossRef CAS PubMed
. - Y. Kim, H. Yuk, R. Zhao, S. A. Chester and X. Zhao, Nature, 2018, 558, 274–279 CrossRef CAS PubMed
. - S. Li, Nature, 2019, 567, 314–315 CrossRef PubMed
. - F. Ilievski, A. D. Mazzeo, R. F. Shepherd, X. Chen and G. M. Whitesides, Angew. Chem., 2011, 123, 1890–1895 CrossRef PubMed
. - F. Carpi, S. Bauer and D. De Rossi, Science, 2010, 330, 1759–1761 CrossRef CAS PubMed
. - L. Hines, K. Petersen and M. Sitti, Adv. Mater., 2016, 28, 3690–3696 CrossRef CAS PubMed
. - J. Kim, J. A. Hanna, M. Byun, C. D. Santangelo and R. C. Hayward, Science, 2012, 335, 1201–1205 CrossRef CAS PubMed
. - J. C. Nawroth, H. Lee, A. W. Feinberg, C. M. Ripplinger, M. L. McCain, A. Grosberg, J. O. Dabiri and K. K. Parker, Nat. Biotechnol., 2012, 30, 792–797 CrossRef CAS PubMed
. - S.-J. Park, M. Gazzola, K. S. Park, S. Park, V. Di Santo, E. L. Blevins, J. U. Lind, P. H. Campbell, S. Dauth, A. K. Capulli, F. S. Pasqualini, S. Ahn, A. Cho, H. Yuan, B. M. Maoz, R. Vijaykumar, J.-W. Choi, K. Deisseroth, G. V. Lauder, L. Mahadevan and K. K. Parker, Science, 2016, 353, 158–162 CrossRef CAS PubMed
. - T. Li, G. Li, Y. Liang, T. Cheng, J. Dai, X. Yang, B. Liu, Z. Zeng, Z. Huang, Y. Luo, T. Xie and W. Yang, Sci. Adv., 2017, 3, e1602045 CrossRef PubMed
. - G. Li, X. Chen, F. Zhou, Y. Liang, Y. Xiao, X. Cao, Z. Zhang, M. Zhang, B. Wu, S. Yin, Y. Xu, H. Fan, Z. Chen, W. Song, W. Yang, B. Pan, J. Hou, W. Zou, S. He, X. Yang, G. Mao, Z. Jia, H. Zhou, T. Li, S. Qu, Z. Xu, Z. Huang, Y. Luo, T. Xie, J. Gu, S. Zhu and W. Yang, Nature, 2021, 591, 66–71 CrossRef CAS PubMed
. - Z. Ren, W. Hu, X. Dong and M. Sitti, Nat. Commun., 2019, 10, 2703 CrossRef PubMed
.
|
This journal is © The Royal Society of Chemistry 2021 |
Click here to see how this site uses Cookies. View our privacy policy here.