DOI:
10.1039/D3NR02561F
(Paper)
Nanoscale, 2023,
15, 11927-11934
Secondary ligand-induced orthogonal self-assembly of silver nanoclusters into superstructures with enhanced NIR emission†
Received
31st May 2023
, Accepted 14th June 2023
First published on 23rd June 2023
Abstract
Orthogonal self-assembly is one of the crucial strategies for forming complex and hierarchical structures in biological systems. However, creating such ordered complex structures using synthetic nanoparticles is a challenging task and requires a high degree of control over structure and multiple non-covalent interactions. In this context, nanoarchitectonics serves as an emerging tool to fabricate complex functional materials. Here, we present a secondary ligand-induced orthogonal self-assembly of atomically precise silver nanoclusters into complex superstructures. Specifically, we use Ag14NCs protected with naphthalene thiol and 1,6-bis(diphenylphosphino)hexane ligands. Controlled addition of 1,6-bis(diphenylphosphino)hexane, the secondary ligand resulted in a self-assembled supracolloidal structure including helical fibers, spheres, and nanosheets. The self-assembly process is tunable by controlling the molar ratio of the ligand. The resulting superstructures exhibit enhanced NIR emission due to restricted intramolecular motion. This demonstrates that by tuning supramolecular interactions, hierarchical nanostructures with desired properties similar to biomolecules can be obtained from atomically precise building blocks.
Introduction
Self-assembly of molecular building units into complex functional and structural biological superstructures is omnipresent in nature.1–3 Orthogonal self-assembly is one of the important features of biological systems.4–8 In orthogonal self-assembly, multiple but non-interfering non-covalent interactions play a crucial role in the formation of complex structures. Examples include the double helical structure of DNA, protein folding, and cell membranes.2,3,9 Extensive research has been conducted to synthesize/mimic such biological architectures using molecular-level building blocks, supramolecular polymers, and metal–ligand complexes using orthogonal self-assembly.6,7,10–13 Hierarchical structures of metallacycles and metallacages are fabricated by exploiting the orthogonal metal–ligand coordination and other noncovalent interactions.9 Sun et al. demonstrated that alanine-based chiral metallacycles could self-assemble into nanospheres at low concentrations and chiral metallogels at high concentrations utilizing the noncovalent interactions.12 Recently, nano architectures from nanomaterials are fabricated using the nanoarchitectonics concept.14–16
Despite numerous efforts using molecular and macromolecular building blocks, orthogonal self-assembly of synthetic metal nanoparticles has been a challenge. This is attributed to non-uniform size, uncontrolled ligand density, and aggregation tendencies. In recent years, atomically precise metal nanoclusters (NCs)17,18 have gained attention as functional materials due to their small size, well-defined structure, and unique physical properties.19 They are considered as potential luminophores due to their unique optical properties.20,21 They are also known to self-assemble into hierarchical structures reminiscent of biological systems.22,23 Several strategies are employed to assemble NCs into dimers and higher hierarchical structures, which show self-assembly-induced luminescence, mechanical performance, and biological activities.22,24–27 The most common strategies involve the functionalization of protecting ligands and introducing new linkers such as organic molecules, ions, counterions, solvents, and phase transfer agents that can induce self-assembly in NCs.28,29 Well-defined cluster assembled solids (CASs) have been assembled by exploiting the noncovalent interactions-mediated inter-cluster assembly.30 Apart from their ease of handling, hierarchical/supramolecular architectures of atomically precise NCs retain the intrinsic properties of individual NCs and at the same time display emerging or collective properties.31,32
In this context, a facile and novel route is demonstrated here to self-assemble NCs into supramolecular architectures reminiscent of biomolecules. We have synthesized Ag14 nanoclusters co-protected by naphthalene thiolate and 1,6-bis(diphenylphosphino)hexane (DPPH). These NCs exhibited distinct optical properties compared to analogous Ag14 NCs protected with different ligands.33–36 By controlled addition of DPPH as a bidentate secondary ligand, the Ag14 NCs initially assemble into thin sheets. The sheets further assemble into spherical superstructures and rope-like wraps. Mass spectrometric investigations of supramolecular structures reveal the formation of NC-DPPH adducts. Such supramolecular architectures of NCs show enhanced near-infrared (NIR) emission due to the restricted intramolecular motion (RIM). We believe that such assemblies are directed by the supramolecular interactions between bidentate DPPH ligands and naphthalene thiol (NT) ligands, also the long chain of DPPH acts as a bridge between two NCs resulting in an orthogonal assembly. This method could pave the way to create hierarchical NC architectures with desired properties, which can further offer new avenues to programmable self-assembly of NCs with controllable properties.
Experimental section
Materials
Silver nitrate (AgNO3) was purchased from RANKEM. 1-Napthalenethiol (NT), 1,6-bis(diphenylphosphino)hexane (DPPH), tetraphenylphosphonium chloride (PPh4Cl), triethylamine (TEA), and sodium borohydride (NaBH4) were purchased from Sigma Aldrich. All other solvents such as methanol, hexane, dichloromethane (DCM), trichloromethane (TCM), and N,N-dimethylformamide (DMF) were of analytical grade.
Characterization
PerkinElmer Lambda 25 spectrometer was used to record the UV-Vis absorption spectrum of the nanocluster samples. Photoluminescence measurements were carried out using a Horiba Jobin Yvon NanoLog instrument. Mass spectrometric measurements were performed using a Waters Synapt G2 Si instrument (details in ESI†). The X-ray photoelectron spectroscopy (XPS) measurements of nanocluster samples were carried out with an Omicron ESCA Probe Spectrometer. Nuclear magnetic resonance (NMR) spectra (31P) of the nanocluster samples were recorded using a 500 MHz Bruker Avance III spectrometer. Dynamic light scattering (DLS) measurements were performed using a Malvern Zetasizer ZSP instrument. High-resolution transmission electron microscopy (HRTEM) was performed with a JEOL 3010. Scanning electron microscope (SEM) images and energy dispersive X-ray analysis (EDAX) were collected using an FEI QUANTA-200 SEM. Scanning transmission electron microscope (STEM) imaging was performed using JEOL JEM-2800 high throughput electron microscope equipped with a Schottky type field emission gun operated at 200 kV with simultaneous bright field (BF) and dark field (DF) STEM imaging. For elemental mapping energy dispersive X-ray mapping and spectra were collected using dual silicon drift detectors. The tilt series for electron tomographic reconstruction of the nanocluster assembled architectures were collected using the JEM 3200FSC microscope operated at 300 keV (see electronic ESI† for details).
Synthesis of Ag14(NT)7(DPPH)2Cl2 nanoclusters
The Ag14(NT)7(DPPH)2Cl2 NCs were synthesized by dissolving 20 mg of silver nitrate in 3 mL of methanol. To this solution, 11 mL of DCM was added. A 10 μL of 1-NT was added and the reaction is carried out at 0 °C. After the addition of NT, the color of the solution changed to turbid yellow indicating the formation of silver thiolates. After 5 minutes, 6.8 mg of DPPH (in 0.5 mL DCM) and 6 mg of PPh4Cl (in 0.5 mL DCM) were added. After 30 minutes, 45 mg of NaBH4 in 1 mL ice-cold (DI) water and 50 μL of TEA were added. The color of the solution changed to dark brown after the addition of NaBH4. After five hours of continuous stirring, the crude cluster was kept in the fridge overnight for size focusing. The resultant material was centrifuged and the precipitate was washed several times with methanol, hexane, and DMF and dispersed in DCM or TCM for characterization.
Secondary ligand-induced nanoarchitectures of Ag14 nanoclusters
Approximately 10 mg of Ag14(NT)7(DPPH)2Cl2 NCs were dissolved in 10 mL of TCM. To this NC solution, the DPPH ligand was added with the molar ratio of DPPH/NC ranging from 0.5 to 10.
Results and discussion
Characterization of Ag14 nanoclusters
Naphthalene thiol-protected Ag14NCs were synthesized and characterized according to the methods described in the experimental section. The quantized electronic structure of the NCs is evident from the optical absorption spectral peaks centered at 340, 490, and 690 nm (Fig. 1A). They also display intense NIR emission at 1035 nm when excited at 340 nm (inset of Fig. 1A). The ESI MS in the positive mode resulted in a peak centered at m/z 3605, which was assigned as [Ag14(NT)7(DPPH)2Cl2] (Fig. 1B). Experimental and theoretical isotopic distribution matched well and revealed that the Ag14 NCs are monovalent (Fig. 1B1).
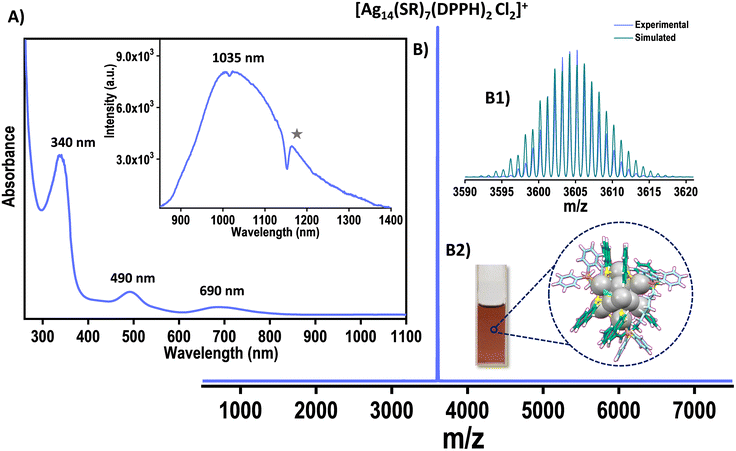 |
| Fig. 1 (A) UV-Vis spectrum of Ag14 NCs. Inset of A shows the emission at 340 nm excitation of Ag14 NCs (star symbol (*) denotes the solvent-induced structuration).37 (B) The ESI MS of Ag14 NCs. (B1) The experimental and simulated spectra match well. (B2) Photograph of the Ag14 NC solution. Color codes: grey, Ag; blue/sea green, C; yellow, S; orange, P; green, Cl; white, H. | |
Collision-induced dissociation (CID) study of [Ag14(NT)7(DPPH)2Cl2]+ further reaffirms the assigned molecular formula (Fig. S1†). A systematic loss of DPPH and NT ligand (L) was observed with an increase in collision energy (CE). Initially, one DPPH was lost, and with further increase in the CE, the second DPPH unit was lost, followed by other primary ligands. The composition of Ag14 NC was further confirmed by elemental analysis which confirms the presence of Ag, S, Cl, and P. Their atomic percentages matched well with the assigned formula (Fig. S2†). Besides, elemental characterization was also performed using X-ray photoelectron spectroscopy (XPS). Peaks corresponding to Ag 3d5/2, S 2p3/2, P 2p3/2, and Cl 2p3/2 appeared at ∼368.5, ∼162.9, ∼131.8, and ∼198.3 eV, respectively (Fig. S3†).
The 31P NMR spectrum of DPPH alone shows major signals at 32.7 and −17.1 ppm (Fig. S4†), which can be attributed to oxidized species such as DPPHO2 and uncoordinated DPPH ligands.38 The 31P NMR spectrum of Ag14 NCs shows two doublets due to the coupling of 31P with 109Ag and 107Ag, which implies the coordination of the DPPH ligand to silver.39,40
Ligand engineering induced tailoring of the optical properties
The UV-vis spectrum of the NT-protected Ag14 NCs is compared with that of the other two Ag14 NCs reported in the literature.33,34 A drastic change in optical properties was observed for NT-protected Ag14NCs (Fig. 2). The UV-vis spectrum of Ag14(3,4-DFBT)12TPP8 (3,4-DFBT = 3,4 diflourobenzenethiol, TPP = triphenylphosphine) displays two major absorption bands at 368 and 530 nm whereas, Ag14(1,2-BDT)6TPP8 (1,2-BDT = 1,2 benzenedithiol, TPP = triphenylphosphine) shows peaks at 425, 600, and 860 nm. The [Ag14(NT)7(DPPH)2Cl2] NC shows molecular peaks at 340, 490, and 690 nm. The Ag14(3,4-DFBT)12TPP8 NCs display dual emission peaks around 536 nm and 420 nm. Such dual emission was also observed in the case of Ag14(1,2-BDT)6TPP8 NCs. The NT-protected Ag14 NCs, in contrast, show NIR emission at 1035 nm. This observation signifies the pivotal role of ligands in tailoring the optoelectronic properties of NCs.
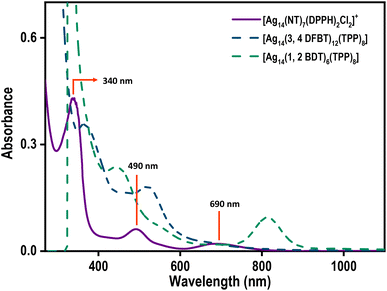 |
| Fig. 2 The UV-vis spectra of [Ag14(NT)7(DPPH)2Cl2]+ (violet trace), Ag14(3,4 DFBT)12TPP8 (dark blue trace), and Ag14(1,2 BDT)6TPP8 (sea green trace). | |
Secondary ligand-induced assembly of Ag14 NCs
NCs are assembled into hierarchical structures using strategies that involve introducing organic molecules, ions, counterions, solvents, and phase transfer agents. The secondary ligand induced transformation and self-assembly of nanoclusters are studied in detail.41–43 Considering the long-chain length of the bidentate DPPH ligand, we were motivated to introduce different ratios of DPPH ligands to induce self-assembly in Ag14 NCs.
Different concentrations of DPPH were introduced into the Ag14 NCs, and their corresponding UV-vis spectra were monitored (Fig. 3). The resulting NC architectures (NCA) are labelled as NCA-0.5, NCA-1.0, NCA-3.0, NCA-5.0, and NCA-10.0 with 0.5, 1.0, 3.0, 5.0, and 10.0 mole ratio of DPPH (with respect to the cluster), respectively. Up to a 1.0 mole ratio, the molecular features of Ag14 NCs were intact, with further increasing the concentration of DPPH, the NC solution becomes colorless. The corresponding UV-vis spectrum reveals the disappearance of major molecular features centered at 490 and 690 nm of the NCs. The peak at 340 nm is red shifted to 390 nm. We assume that this could be due to the degradation of NCs. The disappearance of NC features with increased DPPH ligands concentration illustrates that the NCs decompose or transform in this operation. The TEM images of Ag14 NCs with different concentrations of DPPH revealed the formation of supramolecular hierarchical structures (Fig. S5†). The NCA-0.5 shows a sheet-like assembly, and NCA-1.0 forms right-handed (P-type) super-helical bundles (Fig. S6 and S7†) or coiled coil-like structures44,45 alongside spherical structures of ∼200–300 nm (Fig. S8†). The EDS mapping of NCA 1.0 shows the presence of Ag, S, Cl, P and C which further suggests the aggregation of NCs (Fig. S9, S10, and S11†). With further increase in the concentration of DPPH, we have observed sheets and aggregates, which could be due to the decomposition or transformation of NCs; as also evident from the 31P NMR data (Fig. S12 and S13†). The NCA-1.0 was analyzed in greater detail. The magnified images of spheres and helical bundles, similar to intermediate filaments such as keratin suggest that they comprise atomically precise Ag14 NCs (Fig. 4). Resolving the three-dimensional (3D) structures using electron tomography (ET) reconstruction reaffirms the spherical nature of superstructures (Fig. 4D and E). The cross-sectional view of the sphere reveals a solid interior due to the Ag14 NC assembly (Fig. 4F). Such NC assemblies could be formed by the intercluster supramolecular interactions between the NT ligands and secondary DPPH ligands.
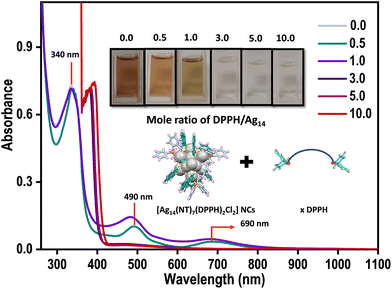 |
| Fig. 3 Variation of UV-vis spectra trend of [Ag14(NT)7(DPPH)2Cl2]+ NCs with the addition of different mole ratios of DPPH ligand. Inset shows the corresponding photographs of the NCs solutions. | |
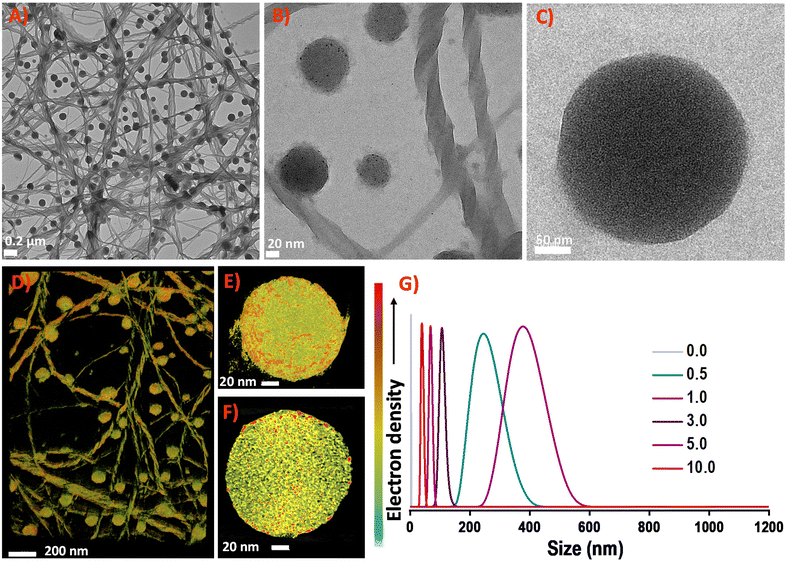 |
| Fig. 4 TEM images showing the self-assembled superhelices and spheres NCA-1.0. Magnified TEM images are shown in A–C. (D) Electron tomography (ET) reconstruction of NCA-1.0. (E) and (F) are the 3D reconstructed structure of the Ag14 NC assembled sphere and its cross-sectional view. (G) DLS measurements show variation in size of [Ag14(NT)7(DPPH)2Cl2]+ NCs with the addition of different molar ratios of DPPH ligands. | |
Dynamic light scattering (DLS) studies unveil the size evolution of the nanostructures formed during the controlled addition of DPPH ligands (Fig. 4G). The average size of Ag14 NCs was found to be 3.2 ± 0.36 nm; NCA-0.5 shows an average size of 245 ± 2 nm. The NCA-1.0 superstructures show an average size of 375 ± 5 nm. With further increasing the concentration of DPPH, the size reduces, suggesting small aggregates and dissolved species.
To gain more insight into the NC assembled superstructures, we have performed ESI MS. Along with the parent NC ion (X+), several new peaks were observed in the ESI MS of NCA-1.0 in the m/z range of 3900–4600 (Fig. 5). Detailed analysis of the peaks reveals that they are NC-DPPH adducts, the composition of the adducts are [X3Y3]3+ (Y = DPPH), (m/z 4059), [X2Y3]2+ (m/z 4287) along with other fragmentation peaks (Fig. 5). Higher NC-DPPH adducts were not observed, which could be due to their lower ionization efficiency. The compositions and the charge states of the adducts were determined by analyzing the m/z values and isotopic patterns of the peaks. In the isotopic distribution of [X3Y3]3+, the difference between the peaks is m/z 0.33, which confirmed a charge state of 3+ (Fig. 5A2). The experimental isotopic distribution matches well with the theoretical isotopic distribution of [X3Y3]3+, which further reaffirms the assigned composition. The difference between peaks in the isotopic distribution of [X2Y3]2+ is m/z 0.5, which suggests a charge state of 2+ (Fig. 5A3). Along with these adducts there are several other low intensity peaks which could be due to weak gas phase adducts of X and Y. Due to low intensity, we could not perform CID measurements on the DPPH-mediated trimers and dimers of Ag14 NCs. To understand the role of DPPH in directing the hierarchical structures, NCA-1.0 was redispersed in DMF as DPPH is insoluble in DMF and analysed using UV-vis spectroscopy and ESI MS (Fig. S14†). The ESI MS in DMF didn't show any NC adducts which further confirms the role of DPPH in the NC assembly (Fig. S14†). We have also performed TEM measurements of the DPPH–NT mixture, which revealed that they also form thin sheets (Fig. S15†). The formation of sheet-like structures further reaffirms the supramolecular interactions between the primary NT ligands and secondary DPPH ligands.
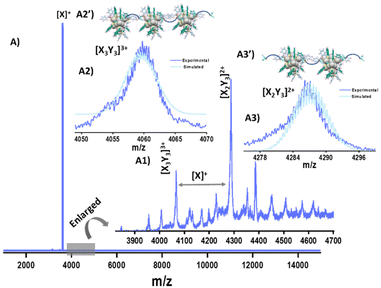 |
| Fig. 5 (A) ESI MS showing the supramolecular adducts in NCA-1.0. (A1) Enlarged view of the same. (A2) The experimental and theoretical isotopic patterns of [X3Y3]3+. (A2′) Schematic representation of [X3Y3]3+. (A3) The experimental and theoretical isotopic patterns of [X2Y3]2+. (A3′) Schematic representation of [X3Y3]2+. (X = [Ag14(NT)7(DPPH)2Cl2]; Y = DPPH). Color codes: grey, Ag; blue/sea green, C; yellow, S; orange, P; green, Cl; white, H. | |
Mechanism for the formation of supramolecular architectures
We speculate that the optimum concentration of DPPH directs orthogonal self-assembly, which leads to the formation of two different supramolecular architectures in the same system. Specific non-covalent intermolecular interactions could direct the formation of such interpenetrating networks. Orthogonal self-assembly6,11,13 is an essential strategy in biological systems, where complex structures are generated by highly specific non-covalent interactions between constituents such as proteins, amphiphiles, and biopolymers.10 In supramolecular chemistry, specific non-covalent interactions are exploited to achieve such orthogonal self-assembly.46 The naphthalene diimide chromophores47 are known to organize into supramolecular helices.48 Substitutions on naphthalene had resulted in helical tubes,49 twisted ribbons or helices with preferred handedness.50
Based on microscopic and spectroscopic investigations, we propose that the orthogonal self-assembly process of Ag14 NCs relies on (i) bidentate nature of DPPH ligand, (ii) π⋯π stacking of the NT ligands in Ag14 NCs, (iii) CH⋯π interactions between NT and DPPH ligands (Fig. S16†), and (iv) H⋯H interactions between NT ligands (Scheme 1). Such covalent and non-covalent interactions together lead to the formation of the microspheres and sheets/films of Ag14 NCs (Scheme 1A1) whereas, a lower degree of self-assembly in the z-axis results in the formation of superhelices which tends to bundle to form rope-like NC assemblies (Scheme 1A2). Compared to other ligand-protected Ag14 nanoclusters such as Ag14(3,4-DFBT)12TPP8,34 Ag14(1,2-BDT)6TPP8
33 and Ag14(pntp)10(dpph)4Cl2,51 [Ag14(NT)7(DPPH)2Cl2]+ NCs are ligand deficient. Therefore, their surface structure can afford a few more ligands without stripping the thiolates and phosphines. The NT and DPPH ligands and their π⋯π and CH⋯π interactions play a crucial role in directing such orthogonal self-assembled nanostructures.
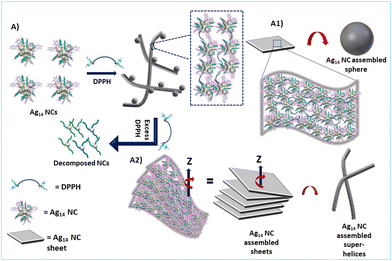 |
| Scheme 1 Schematic illustration showing the mechanism of DPPH-induced orthogonal self-assembly of Ag14 NCs into superhelices and spheres. | |
Enhanced emission
The optical features of Ag14 NCs were retained up to NCA-1.0 which suggests that the NC property is retained in hierarchical structures. This prompted us to investigate their emission properties. A significant enhancement in the emission of Ag14 NCs assembled superstructures was observed (Fig. 6).
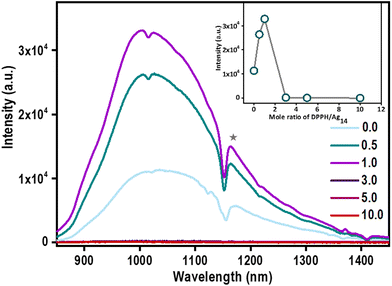 |
| Fig. 6 Variation of PL trend of [Ag14(NT)7(DPPH)2Cl2]+ NCs with the addition of different mole ratios of DPPH ligand (the star denotes the solvent-induced structuration). Inset shows the variation of PL intensity at 1035 nm with a different mole ratio of DPPH ligand. | |
A three-fold enhancement in PL emission was observed in the case of NCA-1.0 than its parent Ag14 NCs. With increasing the concentration of DPPH (3.0, 5.0, and 10.0), the PL intensity reduces significantly. Increment in the emission of Ag14 NCs in solution by the addition of DPPH is attributed to the restricted motion of the ligands through ordered self-assembly. Atomically precise NCs are known to display aggregation-induced emission (AIE) due to restrictions in the intermolecular rotation (RIR).52 The ordered assembly of surface motifs in aggregation-induced nanostructures reduces vibrational relaxation enhancing emission.53 The inter-nanocluster CH⋯π interactions are also known to restrict the intramolecular rotations/vibrations and amplify the PL emission of NCs.54 We hypothesize that inter-nanocluster CH⋯π and π⋯π interactions that direct the ordered assembly of the NCs together restrict the molecular rotations and enhances the emission.
Conclusions
In this work, we demonstrate a novel facile route for creating supramolecular architectures of atomically precise NCs. The controlled addition of bidentate secondary ligand DPPH directs the orthogonal self-assembly of NCs reminiscent of biological systems. The mass spectrometric investigations reveal that the supramolecular architectures are formed by the assembly of NC–DPPH adducts. The distinct non-covalent interactions of surface motifs with excess secondary bidentate ligands play a pivotal role in such orthogonal self-assembly of NCs. The self-assembled structures show an enhanced NIR emission due to restricted intramolecular motion (RIM). To the best of our knowledge, this is the first example of secondary-ligand induced orthogonal self-assembly of atomically precise NCs. We envision the application of orthogonal self-assembly approaches to create self-assembled structures from atomically precise NCs that offer complex hierarchical architectures of NCs.
Author contributions
K.S.S and A.P.S performed synthesis, and other experimental studies. Nonappa performed electron tomography, HRTEM and STEM studies. D.G and J.R performed mass spectrometric studies. J.S.M performed the TEM measurements. M.P.K performed XPS studies. B.S.S performed NMR studies. P.S performed SEM measurements. W.A.D was involved in the discussion of experimental data. K.S.S wrote the first draft of the manuscript and all co-authors have given approval to the final version of the manuscript. T.P conceptualized and finalized the manuscript.
Conflicts of interest
There are no conflicts to declare.
Acknowledgements
We thank the Department of Science and Technology, Government of India, for constantly supporting our research program on nanomaterials. K. S. S thanks the University Grants Commission (UGC), Govt. of India, for her research fellowship. P. S., J. R., and D. G. thanks IIT Madras for their research fellowship. W. A. D thanks SERB-DST for the award of a National Postdoctoral Fellowship (NPDF). B. S. S thanks CSIR for his research fellowship. We thank Dr Ganesan Paramasivam for his help and suggestions in this work. We acknowledge the Academy of Finland for project funding (No. 352900), Photonics Research and Innovation (PREIN) flagship, Nanomicroscopy Center at Aalto University and Tampere Microscopy Centre at Tampere University for instrumentation facilities.
References
- J. D. Watson and F. H. C. Crick, Nature, 1953, 171, 737–738 CrossRef CAS PubMed
.
-
T. Xiao, X.-Q. Sun and L. Wang, in Handbook of Macrocyclic Supramolecular Assembly, ed. Y. Liu, Y. Chen and H.-Y. Zhang, Springer Singapore, Singapore, 2019, pp. 1–28, DOI:10.1007/978-981-13-1744-6_54-1
.
- T. Leigh and P. Fernandez-Trillo, Nat. Rev. Chem., 2020, 4, 291–310 CrossRef CAS PubMed
.
- C. K. McLaughlin, G. D. Hamblin and H. F. Sleiman, Chem. Soc. Rev., 2011, 40, 5647–5656 RSC
.
- P. Yakovchuk, E. Protozanova and M. D. Frank-Kamenetskii, Nucleic Acids Res., 2006, 34, 564–574 CrossRef CAS PubMed
.
- M. L. Saha, S. De, S. Pramanik and M. Schmittel, Chem. Soc. Rev., 2013, 42, 6860–6909 RSC
.
- X.-Y. Hu, T. Xiao, C. Lin, F. Huang and L. Wang, Acc. Chem. Res., 2014, 47, 2041–2051 CrossRef CAS PubMed
.
-
T. Xiao, X.-Q. Sun and L. Wang, Handbook of Macrocyclic Supramolecular Assembly, 2020, pp. 1317–1344 Search PubMed
.
- S. Datta, M. L. Saha and P. J. Stang, Acc. Chem. Res., 2018, 51, 2047–2063 CrossRef CAS PubMed
.
- J. Boekhoven, A. M. Brizard, M. C. A. Stuart, L. Florusse, G. Raffy, A. Del Guerzo and J. H. van Esch, Chem. Sci., 2016, 7, 6021–6031 RSC
.
- P. Wei, X. Yan and F. Huang, Chem. Soc. Rev., 2015, 44, 815–832 RSC
.
- Y. Sun, S. Li, Z. Zhou, M. L. Saha, S. Datta, M. Zhang, X. Yan, D. Tian, H. Wang, L. Wang, X. Li, M. Liu, H. Li and P. J. Stang, J. Am. Chem. Soc., 2018, 140, 3257–3263 CrossRef CAS PubMed
.
- S. Datta, S. K. Misra, M. L. Saha, N. Lahiri, J. Louie, D. Pan and P. J. Stang, Proc. Natl. Acad. Sci. U. S. A., 2018, 115, 8087–8092 CrossRef CAS PubMed
.
- K. Ariga, T. Mori, T. Kitao and T. Uemura, Adv. Mater., 2020, 32, 1905657 CrossRef CAS PubMed
.
- V. Karthick, L. Kumar Shrestha, V. G. Kumar, P. Pranjali, D. Kumar, A. Pal and K. Ariga, Nanoscale, 2022, 14(30), 10630–10647 RSC
.
- K. Ariga, Nanoscale, 2022, 14(30), 10610–10629 RSC
.
- R. Jin, C. Zeng, M. Zhou and Y. Chen, Chem. Rev., 2016, 116, 10346–10413 CrossRef CAS PubMed
.
- I. Chakraborty and T. Pradeep, Chem. Rev., 2017, 117, 8208–8271 CrossRef CAS PubMed
.
- J. V. Rival, P. Mymoona, K. M. Lakshmi, Nonappa, T. Pradeep and E. S. Shibu, Small, 2021, 17, 2005718 CrossRef CAS PubMed
.
- H. Zhang, Z. Zhao, P. R. McGonigal, R. Ye, S. Liu, J. W. Y. Lam, R. T. K. Kwok, W. Z. Yuan, J. Xie, A. L. Rogach and B. Z. Tang, Mater. Today, 2020, 32, 275–292 CrossRef CAS
.
- Nonappa, Beilstein J. Nanotechnol., 2020, 11, 533–546 CrossRef CAS PubMed
.
- C. Zeng, Y. Chen, K. Kirschbaum, K. J. Lambright and R. Jin, Science, 2016, 354, 1580–1584 CrossRef CAS PubMed
.
- Y. Li, M. Zhou, Y. Song, T. Higaki, H. Wang and R. Jin, Nature, 2021, 594, 380–384 CrossRef CAS PubMed
.
- Q. Li, J. C. Russell, T.-Y. Luo, X. Roy, N. L. Rosi, Y. Zhu and R. Jin, Nat. Commun., 2018, 9, 3871 CrossRef PubMed
.
- S. Chandra, Nonappa, G. Beaune, A. Som, S. Zhou, J. Lahtinen, H. Jiang, J. V. I. Timonen, O. Ikkala and R. H. A. Ras, Adv. Opt. Mater., 2019, 7, 1900620 CrossRef CAS
.
- Y. Bi, Z. Wang, T. Liu, D. Sun, N. Godbert, H. Li, J. Hao and X. Xin, ACS Nano, 2021, 15, 15910–15919 CrossRef CAS PubMed
.
- J. Shen, Q. Xiao, P. Sun, J. Feng, X. Xin, Y. Yu and W. Qi, ACS Nano, 2021, 15, 4947–4955 CrossRef CAS PubMed
.
- A. Ebina, S. Hossain, H. Horihata, S. Ozaki, S. Kato, T. Kawawaki and Y. Negishi, Nanomaterials, 2020, 10, 1105 CrossRef CAS PubMed
.
- Z. Wu, Q. Yao, S. Zang and J. Xie, ACS Mater. Lett., 2019, 1, 237–248 CrossRef CAS
.
- X. Kang and M. Zhu, Coord. Chem. Rev., 2019, 394, 1–38 CrossRef CAS
.
- A. Som, A. Griffo, I. Chakraborty, H. Hähl, B. Mondal, A. Chakraborty, K. Jacobs, P. Laaksonen, O. Ikkala, T. Pradeep and Nonappa, Small, 2022, 18, 2201707 CrossRef CAS PubMed
.
- P. Sun, Z. Wang, Y. Bi, D. Sun, T. Zhao, F. Zhao, W. Wang and X. Xin, ACS Appl. Nano Mater., 2020, 3, 2038–2046 CrossRef CAS
.
- M. Bodiuzzaman, E. Khatun, K. S. Sugi, G. Paramasivam, W. A. Dar, S. Antharjanam and T. Pradeep, J. Phys. Chem. C, 2020, 124, 23426–23432 CrossRef CAS
.
- H. Yang, J. Lei, B. Wu, Y. Wang, M. Zhou, A. Xia, L. Zheng and N. Zheng, Chem. Commun., 2013, 49, 300–302 RSC
.
- A. Jana, M. Jash, A. K. Poonia, G. Paramasivam, M. R. Islam, P. Chakraborty, S. Antharjanam, J. Machacek, S. Ghosh, K. N. V. D. Adarsh, T. Base and T. Pradeep, ACS Nano, 2021, 15, 15781–15793 CrossRef CAS PubMed
.
- Z.-Y. Wang, M.-Q. Wang, Y.-L. Li, P. Luo, T.-T. Jia, R.-W. Huang, S.-Q. Zang and T. C. W. Mak, J. Am. Chem. Soc., 2018, 140, 1069–1076 CrossRef CAS PubMed
.
- Q. Li, D. Zhou, J. Chai, W. Y. So, T. Cai, M. Li, L. A. Peteanu, O. Chen, M. Cotlet, X. Wendy Gu, H. Zhu and R. Jin, Nat. Commun., 2020, 11, 2897 CrossRef CAS PubMed
.
- T. Imamoto, T. Oshiki, T. Onozawa, T. Kusumoto and K. Sato, J. Am. Chem. Soc., 1990, 112, 5244–5252 CrossRef CAS
.
- S. Bestgen, O. Fuhr, B. Breitung, V. S. Kiran Chakravadhanula, G. Guthausen, F. Hennrich, W. Yu, M. M. Kappes, P. W. Roesky and D. Fenske, Chem. Sci., 2017, 8, 2235–2240 RSC
.
- M.-C. Brandys and R. J. Puddephatt, J. Am. Chem. Soc., 2002, 124, 3946–3950 CrossRef CAS PubMed
.
- X. Kang, S. Wang and M. Zhu, Chem. Sci., 2018, 9, 3062–3068 RSC
.
- E. Khatun, A. Ghosh, P. Chakraborty, P. Singh, M. Bodiuzzaman, P. Ganesan, G. Nataranjan, J. Ghosh, S. K. Pal and T. Pradeep, Nanoscale, 2018, 10, 20033–20042 RSC
.
- M. S. Bootharaju, S. Lee, G. Deng, H. Chang, W. Baek and T. Hyeon, J. Chem. Phys., 2021, 155, 014307 CrossRef CAS PubMed
.
- L. Pauling and R. B. Corey, Nature, 1953, 171, 59–61 CrossRef CAS PubMed
.
- F. H. Crick, Nature, 1952, 170, 882–883 CrossRef CAS PubMed
.
- A. J. Savyasachi, O. Kotova, S. Shanmugaraju, S. J. Bradberry, G. M. Ó'Máille and T. Gunnlaugsson, Chem, 2017, 3, 764–811 CAS
.
- M. R. Molla, A. Das and S. Ghosh, Chem. – Eur. J., 2010, 16, 10084–10093 CrossRef CAS
.
- S. M. Wagalgave, S. D. Padghan, M. D. Burud, M. A. Kobaisi, D. D. La, R. S. Bhosale, S. V. Bhosale and S. V. Bhosale, Sci. Rep., 2019, 9, 12825 CrossRef PubMed
.
- G. D. Pantoş, P. Pengo and J. K. M. Sanders, Angew. Chem., Int. Ed., 2007, 46, 194–197 CrossRef PubMed
.
- M. Al Kobaisi, S. V. Bhosale, K. Latham, A. M. Raynor and S. V. Bhosale, Chem. Rev., 2016, 116, 11685–11796 CrossRef PubMed
.
- X. Q. Liang, Y. Z. Li, Z. Wang, S. S. Zhang, Y. C. Liu, Z. Z. Cao, L. Feng, Z. Y. Gao, Q. W. Xue, C. H. Tung and D. Sun, Nat. Commun., 2021, 12, 4966 CrossRef CAS PubMed
.
- X. Kang and M. Zhu, Chem. Soc. Rev., 2019, 48, 2422–2457 RSC
.
- Z. Luo, X. Yuan, Y. Yu, Q. Zhang, D. T. Leong, J. Y. Lee and J. Xie, J. Am. Chem. Soc., 2012, 134, 16662–16670 CrossRef CAS PubMed
.
- T. Chen, S. Yang, J. Chai, Y. Song, J. Fan, B. Rao, H. Sheng, H. Yu and M. Zhu, Sci. Adv., 2017, 3, e1700956 CrossRef PubMed
.
Footnote |
† Electronic supplementary information (ESI) available: Collision-induced dissociation mass spectra, SEM image and EDS mapping, XPS, 31P NMR of Ag14 nanoclusters, TEM images showing the structural evolution of Ag14 nanoclusters with the addition of different molar ratios of DPPH ligands, TEM images of NCA-1.0, STEM images of NCA-1.0, UV-vis spectrum and ESI MS of NCA-1.0 redispersed in DMF, TEM images of the DPPH-NT mixture, and schematic illustration of supramolecular interactions in Ag14 nanoclusters. See DOI: https://doi.org/10.1039/d3nr02561f |
|
This journal is © The Royal Society of Chemistry 2023 |
Click here to see how this site uses Cookies. View our privacy policy here.