DOI:
10.1039/D4NR01954G
(Paper)
Nanoscale, 2024,
16, 14302-14309
The interplay of chromophore–spacer length in light-induced gold nanocluster self-assembly†
Received
6th May 2024
, Accepted 3rd July 2024
First published on 4th July 2024
Abstract
The light-induced self-assembly of chromophore-tethered precision nanoclusters (NCs) has recently received significant attention due to their facile control over structure, function, and reversibility under ambient conditions. However, the magnitude of assembly depends on the photoswitching efficiency, chemical structure, and proximity of the chromophore to the NC surface. Herein, using azobenzene alkyl monothiol (AMT)-capped gold NCs with two different spacer lengths (denoted as C3-NC and C9-NC), we show that reversible cis ↔ trans isomerization efficiency can be readily tuned to control the self-assembly kinetics of NCs. Irrespective of the chain length, the time required for trans-to-cis (140 s) and cis-to-trans (260 s) isomerization of individual C3-AMT and C9-AMT is identical in dichloromethane solution. When a similar experiment was performed using a solution of C3-NCs and C9-NCs, it resulted in self-assembled disc-like superstructures. Notably, the trans-to-cis photoswitching in C3-NC could reach only 65% even after 460 seconds of irradiation. On the other hand, C9-NC completed this process within 160 seconds of irradiation. The low photoswitching efficiency of the C3-NC analog is due to the short and rigid spacer length of C3-AMT ligands, which are in close proximity to the NC surface, resulting in steric hindrance experienced at the NC–chromophore interface. Importantly, the slow photoswitching in C3-NCs helps isolate and investigate the intermediates of assembly. Using high-resolution electron microscopy, atomic force microscopy, and 3D reconstruction, we show that the discs are made up of densely packed arrays of NCs. The prolonged illumination of C9-NCs results in a chain-like assembly due to the dipolar attraction between the previously assembled superstructures. The efficient photoisomerization of chromophores located away from the nanocluster surface has been identified as the key element to speed up the light-induced assembly in chromophore-tethered nanoclusters. Such information will be useful while developing nanoscale photoswitches for electrochemistry, biosensors, and electronic devices.
Introduction
Precision nanoclusters (NCs) offer atomic-scale control over the metal core and exhibit remarkable photophysical and electrochemical properties.1–10 Even minor modifications to a single metal atom can have a major influence on the behavior and functionality of NCs.11–16 This characteristic makes NCs ideal building blocks for constructing two-dimensional and three-dimensional self-assembled superstructures with collective and enhanced properties.17–20 The delicate balance between non-covalent interactions (van der Waals interactions, hydrogen bonding, C–H⋯π/π⋯π interactions, electrostatic interactions, aurophilic interactions, amphiphilic interactions, or light-induced dipole–dipole attractions) and thermodynamics controls the generation of self-assembled superstructures, emphasizing the complexity of the assembly process.21–30 Such assembled materials find applications in device fabrication,31–34 green catalysis,35–39 toxin/metal ion screening,40 gas sensing,41,42 bioimaging, and therapeutics.43–47 Among the different non-covalent interactions, light-induced dipolar interaction is promising as it allows reversible assembly by changing the wavelength of light. Studies on the photoswitching properties of azobenzene functionalized gold nanoparticles are well-documented in the literature for over two decades.48–51 Recently, twenty-five atom gold (Au25) NCs functionalized with thiolated-spiropyran or -azobenzene ligands demonstrated the feasibility of light-induced reversible self-assembly for hierarchical structure formation across length scales.29,52,53 These studies have shown that when azobenzene molecules are attached to the surface of NCs, their dipole moments play a significant role in the assembly process. Light irradiation triggers the trans-to-cis isomerization, resulting in an induced dipole moment. This leads to attractive interactions between neighboring NCs, and forms assembled superstructures. Similar photochemistry has been utilized in systems containing other metal clusters and inorganic nanocages.54–56 However, the extent of assembly largely depends on the photoswitching efficiency of the chromophores attached to the NC surface. This efficiency is influenced by the chemical structure of the chromophore and its proximity to the NC surface. Thus, NCs functionalized with photoswitchable ligands offer facile control over self-assembly kinetics and structural diversity of self-assembled structures. Here, we show the interplay between the spacer length and photoswitching ability of azobenzene alkyl monothiol (AMT) capped Au NCs. We used AMT-protected NCs (C3-NCs and C9-NCs) with two different spacer lengths (C3-AMT and C9-AMT). The assembly process of both NCs in dichloromethane (DCM) solution at ambient temperature under 345 nm light was investigated using different spectroscopic studies and microscopic imaging. The C9-NCs showed quicker self-assembly compared to the C3 analog due to negligible steric hindrance experienced at the NC–chromophore interface resulting from their safer separation. The fewer nonbonding steric interactions allow more favorable orientation and arrangement to the surface chromophores, forming self-assembled superstructures via attractive dipoles. Prolonged illumination of C9-NCs showed a chain-like assembly due to the dipolar attraction between nearby superstructures. These findings highlight the importance of rational design and control over the spatial arrangement of chromophores in precision NCs. By carefully tuning the spacer length and considering the steric effects, it becomes feasible to optimize the self-assembly process and achieve desired supramolecular structures with enhanced photophysical and electrochemical properties.
Results and discussion
To investigate the role of chromophore–spacer length in the light-induced assembly of NCs, we first covalently linked the azobenzene core with the C3-carbon chain (short; C3-AMT) and C9-carbon chain (long; C9-AMT) thiols (Fig. 1a). The synthesis and characterization of the ligands are presented in the ESI (Fig. S1–S15†). The time-dependent absorption spectra obtained from C3-AMT and C9-AMT dispersed in DCM and irradiated separately using UV light (345 nm) are shown in Fig. 1b and c, respectively. The systematic decrease in optical density (OD) at 345 nm and simultaneous gradual increase in OD around 435 nm indicate the trans-to-cis isomerization of azobenzene moieties. The absorption spectra of C3-AMT and C9-AMT show the cis-to-trans isomerization when irradiated at 435 nm (Fig. S16a and b†). The energy-minimized structures of cis/trans geometrical isomers of C3 and C9-AMT obtained through density functional theory (DFT) are illustrated in the inset of Fig. 1b and Fig. S17,† respectively. From the combined plot of OD@345 nm vs. irradiation time of C3-AMT and C9-AMT recorded under 345 nm and 435 nm light, it is evident that the time required for trans-to-cis (∼140 s) and cis-to-trans (∼260 s) isomerization in AMT is nearly identical, irrespective of the spacer length (inset in Fig. 1c). However, the cis-to-trans conversion was not complete due to the overlap of the n–π* transition of cis and trans isomers.57
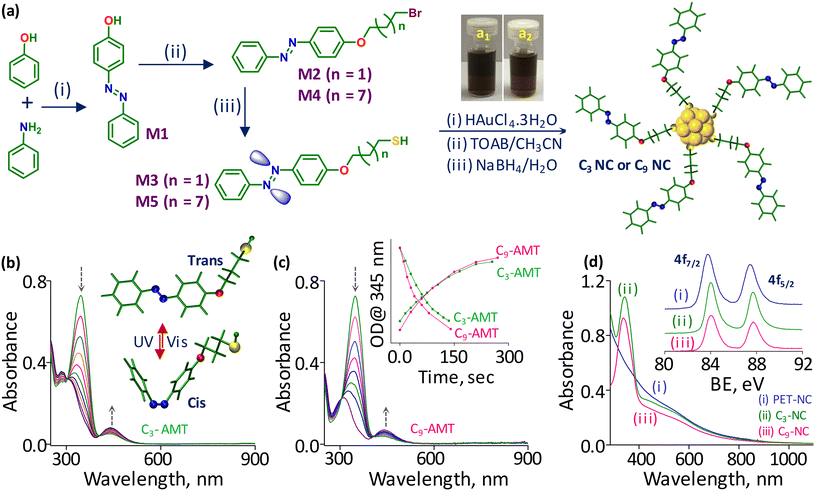 |
| Fig. 1 (a) Synthesis schemes of C3-AMT and C9-AMT (M1–M5), C3-NC, and C9-NC; (i) conc. HCl, NaNO2, 0 °C, (ii) 1,3-dibromopropane or 1,9-dibromononane, K2CO3, KI, acetone, 80 °C, and (iii) HMDST and TBAF, distilled THF, −10 °C. The photographs of C3 and C9 NC solutions are shown in a1 and a2. (b and c) The temporal optical absorption spectra of (b) C3-AMT and (c) C9-AMT under 345 nm illumination. Energy-minimized structures of trans and cis isomers of C3-AMT are shown in the inset of b. Combined plots of OD (ca. 345 nm) vs. illumination time recorded from C3-AMT and C9-AMT under 345 nm and 435 nm are given in the inset of c. (d) UV-Vis absorption spectra of (i) PET-NC, (ii) C3-NC and (iii) C9-NC recorded in DCM. The XPS spectra (Au 4f levels) of all three NCs are shown in the inset of d. | |
To evaluate the role of chromophore–spacer length in the light-induced assembly of NCs, photoswitchable gold NCs with two different spacer lengths (C3-NC and C9-NC) were synthesized and purified using the procedure reported for [Au144(PET)60] NCs (abbreviated as PET-NC; Fig. 1a, see the ESI† for details; here PET represents 2-phenylethanethiol). The optical absorption features of C3 and C9 NCs were compared with those of the PET analog (PET-NC; Fig. 1d). The presence of two weak humps at ca. 520 nm and 700 nm, similar to that of PET-NC, and an overlapping absorption maximum at 345 nm equivalent to the optical absorption characteristics of AMT confirms the formation of C3 and C9 NCs. Since electrospray ionization mass spectrometry (ESI-MS) and matrix-assisted laser desorption ionization (MALDI) mass spectrometry trials were not successful, we compared the X-ray photoelectron spectra (XPS) of C3-NC and C9-NC with that of PET-NC. The well-matching binding energy (BE) values of gold 4f electron orbitals in C3-NC and C9-NC compared to the reported values of PET-NC (inset in Fig. 1d) suggest a similar metal core for all the three NCs (C3-NC, C9-NC, and PET-NC). A small shift in the BE of the NCs is presumably due to the difference in the electron densities of the organic counterparts. The BE values of C 1s, N 1s, O 1s, and S 2p levels of all three NCs are shown in Fig. S18a–d.† The core size of C3-NC and C9-NC was examined using TEM (transmission electron microscopy) analysis. TEM images of C3- and C9-NCs (inset in Fig. 2a and Fig. S19,† respectively) show nearly uniform size distributions (1.9 ± 0.14 nm and 1.87 ± 0.12 nm). The core sizes of C3- and C9-NCs are in agreement with that of PET-NC.58 Next, we evaluated the photoisomerization efficiency of C3 and C9 NC solutions in DCM under two different wavelengths of light. The absorption spectra of C3 and C9 NCs illuminated under 345 nm are shown in Fig. 2a and b, respectively. The time-dependent absorption spectra of C3 and C9 NCs recorded separately during the reverse (cis-to-trans) photoswitching (435 nm) are shown in Fig. S20a and b.† The combined plots of OD@345 nm vs. irradiation time recorded during the photoisomerization (trans-to-cis and cis-to-trans) of C3 and C9 NCs are shown in the inset of Fig. 2b. Notably, the time required for trans-to-cis photoswitching in C3 and C9 NCs (same concentration) under a similar intensity of light (0.2 mW cm−2) was found to be different. More precisely, the trans-to-cis photoswitching in C3-NC could reach only 65% even after 460 seconds of illumination. Beyond this point, there was no change in their absorbance.
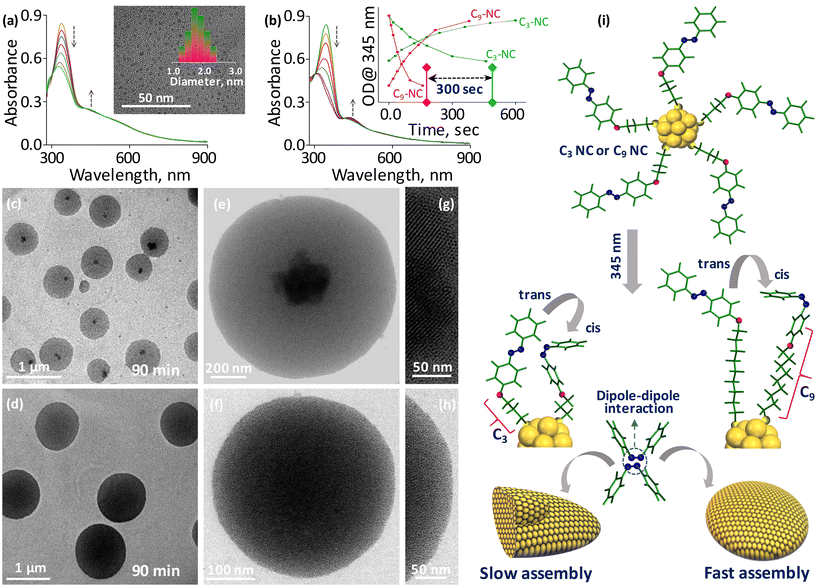 |
| Fig. 2 (a and b) Temporal optical absorption spectra recorded from (a) C3-NC and (b) C9-NC under 345 nm illumination. The TEM micrograph of C3-NC is shown in the inset of a. The combined plots of OD (ca. 345 nm) vs. irradiation time, under 345 nm and 435 nm light recorded from C3-NC and C9-NC are shown in the inset of b. (c and d) Large area TEM images captured from (c) C3-NC and (d) C9-NC assembly after 90 min of illumination under 345 nm light. (e and f) Magnified and tightly focused TEM micrographs of a single superstructure derived from (e) C3-NC and (f) C9-NC. (g and h) An enlarged image captured from (g) the center dark spot of C3-NC and (h) the edge of C9-NC assemblies. (i) The schematic shows the distance-dependent self-assembly in C3-NC and C9-NC and the possible mechanism for their slow and quick assembly. | |
On the other hand, C9-NC completed the isomerization process within 160 seconds of illumination. Importantly, the trans-to-cis isomerization in C9-NC is comparable to that of free AMT molecules (inset in Fig. 1c; 140 s), while the isomerization is three times slower in the case of C3-NCs. This suggests the effect of chromophore proximity on photoswitching. In C9-NCs, the chromophore is located far from the metal surface. Furthermore, the spacer is flexible, allowing less steric crowding. However, in C3-NCs the short spacer is rigid, and the chromophore is in close proximity to the NC surface, resulting in steric crowding of surface ligands. Therefore, the steric hindrance experienced at the NC–chromophore interface finally restricts the photoswitching process. To understand the role of chromophore–spacer length in the self-assembly of NCs and investigate the resulting superstructures, we used TEM imaging. For this, ∼100 μM solutions of C3 and C9 NCs prepared in DCM separately were irradiated at 345 nm for 90 min in a dark room. Constant stirring of the NC solutions in a temperature-controlled bath helped to reduce thermal aggregation. The TEM images of the illuminated C3 and C9 NCs are shown in Fig. 2c and d, respectively. Though C3 and C9 NCs underwent light-induced self-assembly and formed disc-like superstructures, there are some morphological differences in the superstructures formed from both NCs. TEM images of individual superstructures from C3 and C9 NCs are shown in Fig. 2e and f, respectively. The C3-NCs are self-assembled into disc-like superstructures with a crystallized array of NCs encapsulated in each superstructure (Fig. 2g). This is presumably due to the slow assembly and the rigid nature of the ligands, which promote a well-ordered array of NCs. Furthermore, an incomplete and slow isomerization may also affect the assembly kinetics, resulting in heterogeneous structures. On the other hand, the C9-NC superstructure (Fig. 2h) showed a nearly uniform assembly of densely packed structures. From the above spectroscopic and microscopic studies, it is obvious that the timescale of light-induced self-assembly largely depends on the suitable distance between the chromophores and NCs. The uniform assembly in C9-NC compared to that in the C3 analog is attributed to the negligible steric hindrance experienced at the NC–chromophore interface. The schematic shows the distance-dependent self-assembly in C3 and C9 NCs and the possible mechanism for their slow and quick assembly is shown in Fig. 2i. The structural details of self-assembled superstructures were further explored using dark-field scanning transmission electron microscopy (STEM) imaging and elemental mapping. The STEM images of C3- and C9-NC assemblies are shown in Fig. S21a and b,† respectively. High contrast in the STEM image of the C3-NC assembly at the center shows the existence of crystallized aggregates encapsulated within the disc-like structures (Fig. S21c†). The STEM micrograph of the C9-NC assembly shows uniform contrast all over the surface (Fig. S21d†). The chemical composition assessment of both self-assembled superstructures using energy-dispersive X-ray analysis (EDS) verifies the existence of gold, sulfur, nitrogen, oxygen, and carbon. The gold (yellow) and sulfur (green) mappings of single superstructures derived from C3- and C9-NCs are shown in Fig. S21e–h.† The large area STEM images, elemental maps (gold, sulfur, and nitrogen), and EDS spectra recorded from C3- and C9-NC assemblies are shown in the ESI (Fig. S22†).
Furthermore, field emission scanning electron microscopy (FESEM) and atomic force microscopy (AFM) images were utilized to investigate the structural details of assembled superstructures. Fig. 3a and b show the FESEM micrographs captured from C3- and C9-NC assemblies. The disc-like superstructures with a few layers of subsequent assemblies on the top surface are seen in the case of the C3-NC assembly. The C9-NC assembly shows densely packed disc-like superstructures similar to those observed in the TEM image shown in Fig. 2d. The morphology of the assembled superstructures was further examined using AFM imaging. Fig. S23a and b† show the large-area AFM images captured from C3- and C9-NC assemblies, in which the subsequent assemblies are visible in the case of C3-NC. The AFM images of single superstructures derived from C3- and C9-NCs are shown in Fig. S24.† The AFM data are consistent with TEM, STEM, and FESEM results. The high-resolution AFM images captured from a single superstructure derived from C3-NC (Fig. 3c–g) show the periodic assembly of NCs. The zig-zag patterns represent the NC directions in the assembly (Fig. 3d and e).
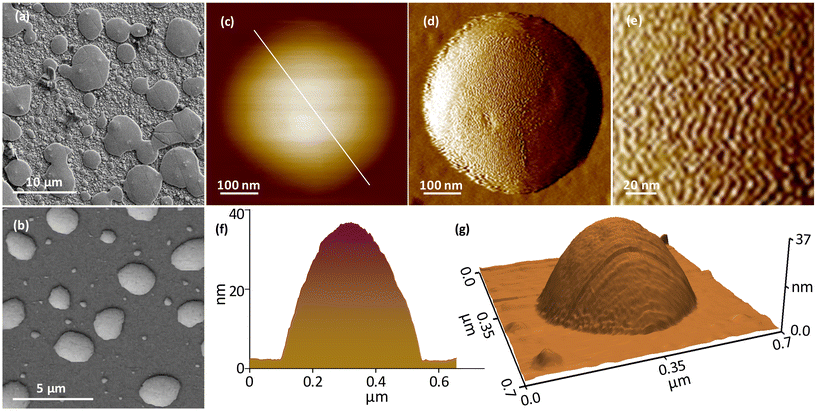 |
| Fig. 3 (a and b) FESEM images of (a) C3-NC and (b) C9-NC assemblies. (c and d) High-resolution tapping mode AFM (c: topography and d: amplitude) images of the single superstructure derived from C3-NC. (e) A zoomed and focused AFM image captured from the top surface of the C3-NC assembly showing the NC direction in the assembly. (f and g) The height profile and three-dimensional (3D) AFM image of the same assembly. | |
To further gain insights into the 3D internal structures of self-assembled structures, we used electron tomography (ET).59 Details are provided in the ESI.† Accordingly, the 2D projections were collected over a range of ±70° with an increment angle of 2° (Fig. S25†). The corresponding TEM images (Fig. 4a and b) and the 3D reconstruction images of C3- and C9-NC assemblies (Fig. 4c–g, Videos S1 and S2†) revealed the disc-like morphology of the superstructures. The cross-sectional view suggests that the superstructures are composed of densely packed NC networks. However, there is a significant difference in the overall disc thickness. As observed in 2D projections, the C3 NCs contain crystalline aggregates within the discs that protrude outside the discs (Fig. 4c, e, and f). On the other hand, a uniform array of NCs was observed in the case of C9 NC-based assemblies (Fig. 4d and g). It is important to note that deformed shapes and flattening are commonly observed in such assemblies due to solvent evaporation and drying artifacts during specimen preparation. However, we observed a clear difference in the 3D reconstructed structures obtained from C3 NCs and C9 NCs. Superstructures obtained from C3 NCs display uniform thickness across the particle. This suggests that the superstructures are disc-like assemblies. On the other hand, in the case of C9 NCs, the deformation and flattening of the structure are evident due to the non-uniform thickness between the center and the periphery.
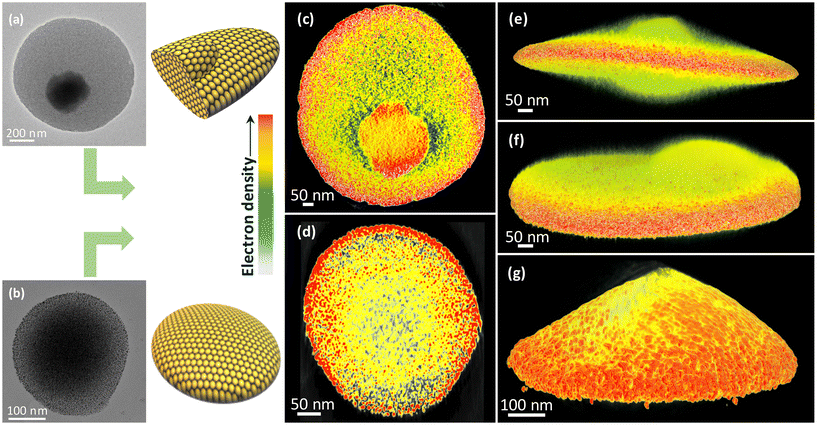 |
| Fig. 4 (a and b) TEM images and (c and d) corresponding cross-sectional views from the ET reconstructed images of (a and c) C3-NC and (b and d) C9-NC superstructures. (e–g) The 3D-reconstructed tomograms of (e and f) C3-NC and (g) C9-NC superstructures in different orientations. | |
NCs were also assembled into dimer and trimer superstructures during the light-induced self-assembly. However, the percentage of dimers and trimers formed was significantly lower compared to that of the monomer assembly. The early stages of monomer, dimer, and trimer assemblies derived from C9-NCs are shown in Fig. S26,† in which the dimer and the trimer show well-connected interfaces with periodic assembly. Eventually, they transformed into densely packed superstructures with constant illumination under 345 nm light (Fig. S27†). The early stages of dimer and trimer assemblies fabricated from C3-NCs with a few layers of incomplete assemblies similar to the monomer are shown in Fig. S28.† Subsequently, we investigated the long-term stability of the assembled superstructures using TEM imaging of the same grid stored at low temperature. Notably, the morphology of the assembly was intact even after one year (Fig. S29†). To understand the formation of disc-like assemblies, TEM imaging was performed on samples prepared using a solution of C9-NC in DCM under 345 nm illumination at different time intervals. The TEM images revealed the formation of disc-like superstructures that evolved from the short circular assembly (Fig. S30†).
We finally investigated the impact of prolonged illumination on the structural transformation of the assembled superstructures. For this, a solution of C9-NC in DCM was UV irradiated at 345 nm for 5 h. The sustained illumination resulted in the solidification of NCs due to the formation of bulky superstructures. To understand the structural rearrangements, the solid material was further examined by redissolving it in DCM. The FESEM image (Fig. 5b) shows a chain-like assembly formed by fusing the individual superstructures due to dipole–dipole interactions between them. A similar chain-like framework seen using the dark-field scattering microscope (Fig. 5c) is in good agreement with the FESEM data. The TEM micrographs captured from the surface and edge of the chain-like structures (Fig. 5d and e) showed a highly ordered multilayer assembly throughout. A scheme for the formation of a chain-like assembly is shown in Fig. 5a, in which the individual NCs initially self-assembled into disc-like superstructures and then into chain-like assemblies due to the extended dipolar interactions between them during the course of illumination. The above-demonstrated idea will be useful in fulfilling industrial needs, especially in the development of molecular photoswitches.
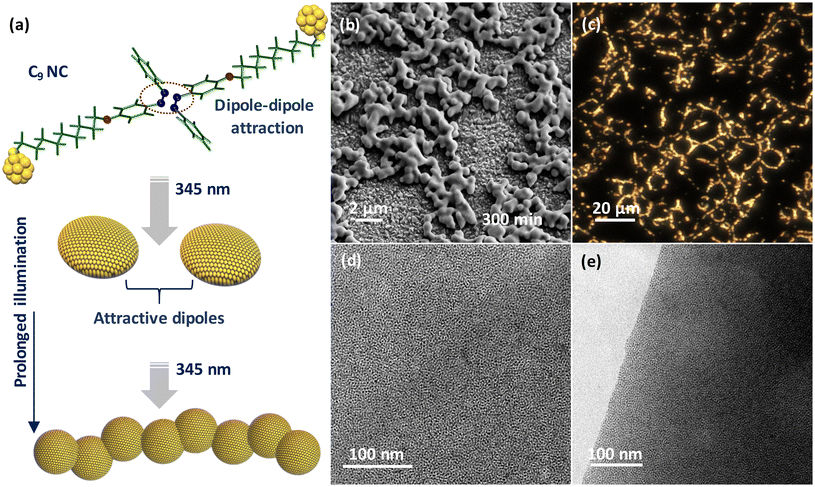 |
| Fig. 5 (a) The schematic depicts the formation of a chain-like assembly. (b) FESEM and (c) dark-field scattering images of the chain-like assembly. (d and e) TEM micrographs from the surface and edge of the chain-like assembly. | |
Conclusions
The reversible self-assembly of NCs using external stimuli such as light, heat, and magnetic fields is a tremendously evolving research area. In the recent past, light has been identified as an efficient probe to demonstrate the supramolecular self-assembly of chromophore-tethered NCs. However, the magnitude of assembly in chromophore-tethered NCs largely depends on their photoswitching efficiency. We have successfully demonstrated this concept by introducing two photoswitchable NCs fabricated using thiolated-azobenzene molecules with different spacer lengths. The core and molecular information of both NCs were meticulously explored using various spectroscopic and microscopic techniques. Photoactivation of both NC solutions at ambient temperature using 345 nm light resulted in the formation of self-assembled superstructures. FESEM, AFM, TEM, ET reconstruction, STEM, and dark field scattering microscopic studies showed the presence of densely packed NCs in self-assembled superstructures. By introducing the idea of distance dependency, we successfully demonstrated the prompt self-assembly in NCs. This was evident from the fast assembly observed in C9-NCs due to their improved photoswitching efficiency resulting from the negligible steric hindrance experienced at the NC–chromophore interface. Such molecular control over the self-assembly of NCs by placing the chromophores suitably away from the nanosurface is useful for developing next-generation switchable sensors, especially in biological, electrochemical, and electronic devices.
Author contributions
The project was designed and supervised by E. S. S. The chromophores and nanoclusters were synthesized by J. V. R. All the experiments were performed by E. S. S. and J. V. R. The electron tomography data acquisition and reconstruction were performed by N. The manuscript was written through the contributions of all authors. All authors have given approval to the final version of the manuscript.
Data availability
The data supporting this article have been included as part of the ESI.†
Conflicts of interest
There are no conflicts to declare.
Acknowledgements
E. S. S. thanks the Science and Engineering Research Board (SERB) for the Ramanujan Fellowship (SB/S2/RJN-005/2017) and Start-up Research grant (SRG/2022/586). E. S. S. thanks the Department of Science and Technology (DST), India, for the Promotion of University Research and Scientific Excellence grant (PURSE; SR/PURSE/2023/191). E. S. S. thanks the University of Calicut for the seed money grant. The authors thank the DST Unit of Nanoscience (DST-UNS) and Thematic Unit of Excellence (TUE), IIT Madras, for HRTEM measurements and DFT calculations. The authors thank the Central Instrumentation Facility (CIF), CSIR-CECRI, for the instrumentation support. J. V. R. thanks the SERB for supporting the research fellowship. The authors thank the Academy of Finland for project funding (No. 352900) and the Photonic Research and Innovation (PREIN) flagship. This work made use of the Tampere Microscopy Centre facilities at Tampere University, Finland.
References
- R. L. Whetten, J. T. Khoury, M. M. Alvarez, S. Murthy, I. Vezmar, Z. L. Wang, P. W. Stephens, C. L. Cleveland, W. D. Luedtke and U. Landman, Adv. Mater., 1996, 8, 428–433 CrossRef CAS.
- G. Schmid, M. Bäumle, M. Geerkens, I. Heim, C. Osemann and T. Sawitowski, Chem. Soc. Rev., 1999, 28, 179–185 RSC.
- Y. Negishi, K. Nobusada and T. Tsukuda, J. Am. Chem. Soc., 2005, 127, 5261–5270 CrossRef CAS.
- H. Häkkinen, Chem. Soc. Rev., 2008, 37, 1847–1859 RSC.
- R. Jin, C. Zeng, M. Zhou and Y. Chen, Chem. Rev., 2016, 116, 10346–10413 CrossRef CAS PubMed.
- W. Kurashige, Y. Niihori, S. Sharma and Y. Negishi, Coord. Chem. Rev., 2016, 320–321, 238–250 CrossRef CAS.
- I. Chakraborty and T. Pradeep, Chem. Rev., 2017, 117, 8208–8271 CrossRef CAS PubMed.
- K. Kwak and D. Lee, Acc. Chem. Res., 2019, 52, 12–22 CrossRef CAS PubMed.
- M. Hesari and Z. Ding, Acc. Chem. Res., 2017, 50, 218–230 CrossRef CAS PubMed.
- Y.-M. Su, Z. Wang, C.-H. Tung, D. Sun and S. Schein, J. Am. Chem. Soc., 2021, 143, 13235–13244 CrossRef CAS.
- X. Kang and M. Zhu, Chem. Soc. Rev., 2019, 48, 2422–2457 RSC.
- X. Kang, S. Chen, S. Jin, Y. Song, Y. Xu, H. Yu and H. Sheng, ChemElectroChem, 2016, 25, 1–6 Search PubMed.
- W. Fei, S. Antonello, T. Dainese, A. Dolmella, M. Lahtinen, K. Rissanen, A. Venzo and F. Maran, J. Am. Chem. Soc., 2019, 141, 16033–16045 CrossRef CAS.
- W. Kurashige, R. Hayashi, K. Wakamatsu, Y. Kataoka, S. Hossain, A. Iwase, A. Kudo, S. Yamazoe and Y. Negishi, ACS Appl. Energy Mater., 2019, 2, 4175–4187 CrossRef CAS.
- S. Wang, Q. Li, X. Kang and M. Zhu, Acc. Chem. Res., 2018, 51, 2784–2792 CrossRef CAS PubMed.
- A. Ghosh, O. F. Mohammed and O. M. Bakr, Acc. Chem. Res., 2018, 51, 3094–3103 CrossRef CAS PubMed.
- P. Chakraborty, A. Nag, A. Chakraborty and T. Pradeep, Acc. Chem. Res., 2019, 52, 2–11 CrossRef CAS.
- Z. Wu, Q. Yao, S. Zang and J. Xie, ACS Mater. Lett., 2019, 1, 237–248 CrossRef CAS.
- J. V. Rival, P. Mymoona, K. M. Lakshmi, Nonappa, T. Pradeep and E. S. Shibu, Small, 2021, 17, 2005718 CrossRef CAS PubMed.
- Nonappa, Chem. Commun., 2023, 59, 13800–13819 RSC.
- Z. Wu, J. Liu, Y. Li, Z. Cheng, T. Li, H. Zhang, Z. Lu and B. Yang, ACS Nano, 2015, 9, 6315–6323 CrossRef CAS.
- Nonappa, T. Lahtinen, J. S. Haataja, T.-R. Tero, H. Häkkinen and O. Ikkala, Angew. Chem., Int. Ed., 2016, 55, 16035–16038 CrossRef CAS PubMed.
- B. Yoon, W. D. Luedtke, R. N. Barnett, J. Gao, A. Desireddy, B. E. Conn, T. Bigioni and U. Landman, Nat. Mater., 2014, 13, 807–811 CrossRef CAS.
- L. Shi, L. Zhu, J. Guo, L. Zhang, Y. Shi, Y. Zhang, K. Hou, Y. Zheng, Y. Zhu, J. Lv, S. Liu and Z. Tang, Angew. Chem., Int. Ed., 2017, 56, 15397–15401 CrossRef CAS.
- T. Higaki, C. Liu, M. Zhou, T.-Y. Luo, N. L. Rosi and R. Jin, J. Am. Chem. Soc., 2017, 139, 9994–10001 CrossRef CAS.
- L. He, Z. Gan, N. Xia, L. Liao and Z. Wu, Angew. Chem., Int. Ed., 2019, 58, 9897–9901 CrossRef CAS PubMed.
- Z. Wu, Y. Du, J. Liu, Q. Yao, T. Chen, Y. Cao, H. Zhang and J. Xie, Angew. Chem., Int. Ed., 2019, 58, 8139–8144 CrossRef CAS PubMed.
- Q. Yao, X. Yuan, Y. Yu, Y. Yu, J. Xie and J. Y. Lee, J. Am. Chem. Soc., 2015, 137, 2128–2136 CrossRef CAS.
- J. V. Rival, Nonappa and E. S. Shibu, ACS Appl. Mater. Interfaces, 2020, 12, 14569–14577 CrossRef CAS PubMed.
- P.-P. Sun, B.-L. Han, H.-G. Li, C.-K. Zhang, X. Xin, J.-M. Dou, Z.-Y. Gao and D. Sun, Angew. Chem., Int. Ed., 2022, 61, e202200180 CrossRef CAS.
- Z. Wu, J. Liu, Y. Gao, H. Liu, T. Li, H. Zou, Z. Wang, K. Zhang, Y. Wang, H. Zhang and B. Yang, J. Am. Chem. Soc., 2015, 137, 12906–12913 CrossRef CAS.
- Y. Liu, D. Yao and H. Zhang, ACS Appl. Mater. Interfaces, 2018, 10, 12071–12080 CrossRef CAS.
- H.-Y. Huang, K.-B. Cai, M. J. Talite, W.-C. Chou, P.-W. Chen and C.-T. Yuan, Sci. Rep., 2019, 9, 4053 CrossRef.
- P. Sun, Z. Wang, Y. Bi, D. Sun, T. Zhao, F. Zhao, W. Wang and X. Xin, ACS Appl. Nano Mater., 2020, 3, 2038–2046 CrossRef CAS.
- Z. Wu, Y. Li, J. Liu, Z. Lu, H. Zhang and B. Yang, Angew. Chem., Int. Ed., 2014, 53, 12196–12200 CrossRef CAS PubMed.
- Z. Wu, H. Zou, T. Li, Z. Cheng, H. Liu, Y. Liu, H. Zhang and B. Yang, Chem. Commun., 2017, 53, 416–419 RSC.
- Y. Zhu, X. Qiu, S. Zhao, J. Guo, X. Zhang, W. Zhao, Y. Shi and Z. Tang, Nano Res., 2020, 13, 1928–1932 CrossRef CAS.
- M. Cao, R. Pang, Q.-Y. Wang, Z. Han, Z.-Y. Wang, X.-Y. Dong, S.-F. Li, S.-Q. Zang and T. C. W. Mak, J. Am. Chem. Soc., 2019, 141, 14505–14509 CrossRef CAS PubMed.
- C. Deng, C. Sun, Z. Wang, Y. Tao, Y. Chen, J. Lin, G. Luo, B. Lin, D. Sun and L. Zheng, Angew. Chem., Int. Ed., 2020, 59, 12659–12663 CrossRef CAS.
- J. Benavides, I. Quijada-Garrido and O. García, Nanoscale, 2020, 12, 944–955 RSC.
- R. Huang, Y. Wei, X. Dong, X. Wu, C. Du, S.-Q. Zang and T. C. W. Mak, Nat. Chem., 2017, 9, 689–697 CrossRef CAS.
- X.-Y. Dong, Y. Si, J.-S. Yang, C. Zhang, Z. Han, P. Luo, Z.-Y. Wang, S.-Q. Zang and T. C. W. Mak, Nat. Commun., 2020, 11, 3678 CrossRef CAS.
- A. Yahia-Ammar, D. Sierra, F. Mérola, N. Hildebrandt and X. Le Guével, ACS Nano, 2016, 10, 2591–2599 CrossRef CAS PubMed.
- M. Hembury, N. Beztsinna, H. Asadi, J. B. van den Dikkenberg, J. D. Meeldijk, W. E. Hennink and T. Vermonden, Biomacromolecules, 2018, 19, 2841–2848 CrossRef CAS PubMed.
- L. Yang, H. Wang, D. Li, L. Li, X. Lou and H. Liu, Chem. Mater., 2018, 30, 5507–5515 CrossRef CAS.
- M. Wang, Y. Chen, W. Cai, H. Feng, T. Du, W. Liu, H. Jiang, A. Pasquarelli, Y. Weizmann and X. Wang, Proc. Natl. Acad. Sci. U. S. A., 2020, 117, 308–316 CrossRef CAS PubMed.
- S. Biswas, A. K. Das, A. Nath, S. Paul, M. S. Singh and S. Mandal, Nanoscale, 2021, 13, 17325–17330 RSC.
- J. Zhang, J. K. Whitesell and M. A. Fox, Chem. Mater., 2001, 13, 2323–2331 CrossRef CAS.
- S. Bonacchi, A. Cantelli, G. Battistelli, G. Guidetti, M. Calvaresi, J. Manzi, L. Gabrielli, F. Ramadori, A. Gambarin, F. Mancin and M. Montalti, Angew. Chem., Int. Ed., 2016, 55, 11064–11068 CrossRef CAS.
- A. Köhntopp, A. Dabrowski, M. Malicki and F. Temps, Chem. Commun., 2014, 50, 10105 RSC.
- R. Klajn, J. F. Stoddart and B. A. Grzybowski, Chem. Soc. Rev., 2010, 39, 2203 RSC.
- Y. Negishi, U. Kamimura, M. Ide and M. Hirayama, Nanoscale, 2012, 4, 4263–4268 RSC.
- T. Udayabhaskararao, P. K. Kundu, J. Ahrens and R. Klajn, ChemPhysChem, 2016, 17, 1805–1809 CrossRef CAS PubMed.
- L. Ai, Y. Li, Z. Wu, J. Liu, Y. Gao, Y. Liu, Z. Lu, H. Zhang and B. Yang, J. Phys. Chem. C, 2016, 120, 24427–24436 CrossRef CAS.
- Y. Deng, Y. Wu, Z. Li, Z. Jagličić, R. K. Gupta, C. Tung and D. Sun, Chin. J. Chem., 2023, 41, 1667–1672 CrossRef CAS.
- K. Sheng, Y.-N. Liu, R. K. Gupta, M. Kurmoo and D. Sun, Sci. China: Chem., 2021, 64, 419–425 CrossRef CAS.
- Z. Wu, R. Xue, M. Xie, X. Wang, Z. Liu, M. Drechsler, J. Huang and Y. Yan, J. Phys. Chem. Lett., 2018, 9, 163–169 CrossRef CAS PubMed.
- N. Yan, N. Xia, L. Liao, M. Zhu, F. Jin, R. Jin and Z. Wu, Sci. Adv., 2018, 144, 1–8 Search PubMed.
- Nonappa, ACS Mater. Au, 2024, 4, 238–257 CrossRef CAS.
Footnote |
† Electronic supplementary information (ESI) available: Experimental details, 1H, 13C, FT-IR, and LC-MS of molecules; energy-minimized structures and temporal absorption spectra of M3 and M5 molecules; XPS spectra of PET-NC, C3-NC, and C9-NC; HR-TEM images and temporal absorption spectra of C3-NC, and C9-NC; STEM micrographs and elemental maps of C3-NC and C9-NC assemblies; AFM images of C3-NC and C9-NC assemblies; TEM images of C3-NC and C9-NC self-assembled monomers, dimers, and trimers; TEM images of C3-NC and C9-NC assemblies after one year; temporal TEM images of a C9-NC assembly demonstrate the formation of a disc-like assembly; three-dimensional superstructures are composed of densely packed NCs (MOV). See DOI: https://doi.org/10.1039/d4nr01954g |
|
This journal is © The Royal Society of Chemistry 2024 |
Click here to see how this site uses Cookies. View our privacy policy here.