DOI:
10.1039/D4RA05628K
(Review Article)
RSC Adv., 2024,
14, 35128-35162
Bisphenol A (BPA) toxicity assessment and insights into current remediation strategies
Received
2nd August 2024
, Accepted 16th October 2024
First published on 11th November 2024
Abstract
Bisphenol A (BPA) raises concerns among the scientific community as it is one of the most widely used compounds in industrial processes and a component of polycarbonate plastics and epoxy resins. In this review, we discuss the mechanism of BPA toxicity in food-grade plastics. Owing to its proliferation in the aqueous environment, we delved into the performance of various biological, physical, and chemical techniques for its remediation. Detailed mechanistic insights into these removal processes are provided. The toxic effects of BPA unravel as changes at the cellular level in the brain, which can result in learning difficulties, increased aggressiveness, hyperactivity, endocrine disorders, reduced fertility, and increased risk of dependence on illicit substances. Bacterial decomposition of BPA leads to new intermediates and products with lower toxicity. Processes such as membrane filtration, adsorption, coagulation, ozonation, and photocatalysis have also been shown to be efficient in aqueous-phase degradation. The breakdown mechanism of these processes is also discussed. The review demonstrates that high removal efficiency is usually achieved at the expense of high throughput. For the scalable application of BPA degradation technologies, removal efficiency needs to remain high at high throughput. We propose the need for process intensification using an integrated combination of these processes, which can solve multiple associated performance challenges.
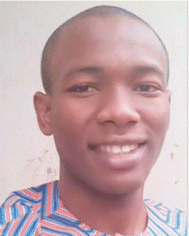 Joshua O. Ighalo | Joshua O. Ighalo is an academic staff in the Department of Chemical Engineering, Nnamdi Azikiwe University, Nigeria. He performs research on nanotechnology for cleaner energy and environmental pollution remediation. He is a registered engineer by the Council for the Regulation of Engineering in Nigeria (COREN) and a member of the American Institute of Chemical Engineers (AIChE). He has co-authored over 200 peer-reviewed papers in Scopus-indexed journals and has published 3 edited books (with Elsevier B. V. and Springer Nature). He currently serves as an academic editor for Advances in Materials Science and Engineering, John Wiley & Sons Ltd. |
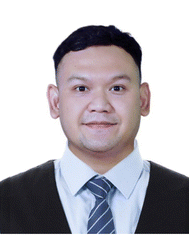 Setyo Budi Kurniawan | Setyo Budi Kurniawan is listed among the World's Top 2% of Scientists by Elsevier (2023–2024). He is interested in the research topics of environmental remediation, wastewater treatment, and circular economy. He is currently a postdoctoral researcher in the Department of Chemical and Process Engineering, National University of Malaysia. He can be contacted via E-mail: setyobk@ukm.my. |
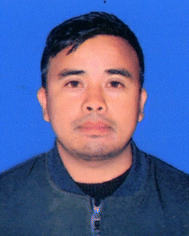 Banlambhabok Khongthaw | Banlambhabok Khongthaw is currently pursuing his PhD in Food Technology at Shoolini University, Solan, Himachal Pradesh. His research focuses on the extraction and encapsulation of phytochemicals for incorporation into edible films. Banlambhabok is a recipient of the prestigious National Fellowship & Scholarship (NF-ST) from the Ministry of Tribal Affairs, Government of India. He has also published a research paper, review paper, and book chapter in both national and international journals. |
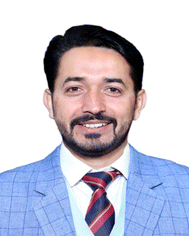 P. K. Chauhan | P. K. Chauhan completed his MSc and PhD at the HNB Central University Garhwal, Srinagar, UK. He also holds a BSc (Medical) from BBN PG College, Hamirpur, HP. Currently, he serves as Professor at the Faculty of Applied Sciences and Biotechnology, Shoolini University, Solan, HP, India. His research is focused on the extraction and purification of bioactive phytonutrients from the medicinal plants of the Himalayan region and their in vitro and in vivo applications against diabetes, hypertension, and cardiovascular diseases. He has published numerous high-quality research articles and book chapters in reputed SCI and Scopus journals/books. |
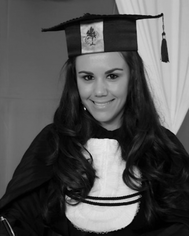 Jordana Georgin | Jordana Georgin graduated in Forest Engineering from UFSM (2013), with experience in environmental and forest licensing. She holds a master's degree in Sanitary and Environmental Engineering (UFSM, 2016), focusing on activated carbon from peanut shells for removing organic pollutants. She completed her PhD in Civil Engineering (UFSM), researching biosorbents and activated carbon for emerging pollutant removal via adsorption. She also earned a Pedagogical Training degree from IFRS (2022). Currently, she is a professor at Universidad de la Costa (CUC), Colombia, teaching Environmental Pollution and Treatment. |
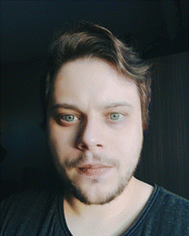 Dison Stracke Pfingsten Franco | Dison Stracke Pfingsten Franco is Chemical Engineer from the Federal University of Pampa (2014), with a master's degree (2016) and PhD (2021) in Chemical Engineering from UFSM, focusing on adsorbents for removing contaminants and heavy metals. He holds a pedagogical degree (IFRS, 2022) with a project on educational trends. He studies transport phenomena, unit operations, effluent treatment, and separation processes. He is experienced in adsorption for pollutant removal using biosorbents, carbon materials, and biopolymers, as well as modeling, process optimization, and surface chemistry. Currently, he is a professor at Universidad de la Costa (CUC), Colombia, teaching Environmental and Hydraulic Modeling for Environmental and Civil Engineering courses. |
1. Introduction
Society's current consumption model stands out for the growing consumption of industrial compounds in processes that use epoxy resins and polycarbonate plastics, which contain bisphenol A (BPA).1,2 Among the products consumed around the world are beverage and food packaging, toys, and medical devices.3,4 These products are consumed in most cultures and social classes encompassing millions of people, resulting in an exponential increase in the release of BPA into the environment, raising concerns about its toxic effects throughout the food chain.5 Its chemical and physical characteristics are similar to those of hormones and can easily exhibit effects close to estrogen.6 Owing to this behavior, BPA can generate disturbances in the endocrine system, affecting the regulation and production of hormones.7
Other studies also relate its toxic effects to the formation of cancer cells, increased obesity rates, decreased fertility, and propensity for diabetes.8 These issues have raised interest in eliminating or reducing BPA exposure in various products consumed worldwide. The major concern is mainly linked to its presence in beverage and food packaging, which facilitates leaching into soil and water resources.9 Transport is favored when products are exposed to higher temperatures or even acidic compounds; in addition to this, the risk of contact is intensified with prolonged and continuous use of packaging.10 Owing to the damage caused by BPA, the search for new alternatives that aim to replace the product in terms of production has been enhanced and encouraged.11 The concern also involves the environment, considering its contamination in water and soil, which is intensified by inadequate disposal of various packaging, corroborating its leaching in landfills.12,13
Because of its characteristics such as transparency, robustness, and heat resistance, BPA is preferably used in the production of various food-grade plastic containers.14 The insecurity in using this product is its ability to move into products for human consumption, which leads to constant exposure of living organisms.15,16 The application of BPA in food-grade plastic packaging has been widely studied and discussed around the world.17 It is worth noting that although some studies affirm unlikely toxicity at low concentration levels, others state that continued exposure over the years causes serious damage, making it highly necessary to substitute new, more environmentally friendly alternatives in place of BPA in food-grade plastics.18–21
It was in the 1950s that the commercialization of BPA began in the United States (USA) and then spread throughout Europe.22 The increase in its production is currently being led by China with a growth rate of approximately 5%, while in Asia it increased by 13% in the early 2000s until 2006.23 In the USA alone, in the period from 2004 to 2006, around 1 million tons were generated, a number close to that generated in Western Europe.24 In the USA, BPA is called a toxic chemical with a large volume of use.25,26 It is worth noting that in poorer countries such as Africa and Latin America, these compounds do not have defined and established guidelines, even though it has been observed in studies that in countries such as Nigeria (Africa) the same was detected in several sources, highlighting the high risk of exposure in humans.27 In 2011, the use of 5.5 million tons of BPA was recorded worldwide.28–31 Inefficient treatments in treatment plants show that domestic sewage, together with manufacturing industries, are the main routes for BPA to enter the environment.32
Various technologies are used to remedy its presence in water and soil, and the treatments can be grouped into biological, chemical, and physical techniques. It is worth noting that all technologies have their limitations; therefore, other studies are analyzing the application of hybrid processes as a way to increase the process efficiency and reduce the operating costs. In particular, for BPA reported in the literature, one can observe studies on biological degradation (native microbes and enzymes),33,34 biochemical and photo-oxidation,35–37 membrane separation processes,38,39 thermal degradation40–42 and adsorption (graphene, zeolites, and modified carbon).43–45 These are among the most applicable technologies for decontaminating solutions containing BPA. It is essential to have a critical understanding of the efficiency of these processes across the varied ecospheres of the environment.
This study first analyzes the toxicity of BPA through its detection in environment-grade plastics. As a methodological process, a careful search in the Web of Science, Google Scholar, Scopus, and other databases is performed. A critical and current view is taken to describe the potential for the biodegradation of BPA by different species of bacteria. With this, the potential damage along the food chain is described. An in-depth analysis is described to elucidate the contamination and presence of BPA in food-grade plastic packaging. The influences of various storage conditions, such as pH and temperature parameters, are analyzed. To remediate BPA, an understanding of the entry routes (disposal of packaging and wastewater from industrial activities) and the transformation mechanisms is essential. Therefore, this entire process is also analyzed, corroborating the project to improve remediation technologies. Based on the proliferation of BPA in the environment, it was pertinent to review and discuss the current advances in physical, chemical, and biological processes for BPA remediation. To improve our understanding of these processes, an in-depth analysis of the mechanisms involved in BPA remediation is discussed for each process. This study aims to update the target audience on the problematic presence of BPA in food-grade plastics, to alert them to the extreme urgency of the need to develop safer and more sustainable alternatives.
2. Review methodology
The applied methodology is summarized in Fig. 1. Initially, a literary search was carried out in an extensive database, which includes journals present in the Web of Science, Google Scholar, Scopus, and others. Second, the words used for the search were selected, which include chemical composition and structure, BPA, its concentration in food-grade plastics, the biodegradation of BPA by strains of bacteria, the toxic effects on living organisms, and remediation technologies. The selection of articles that were published over the last 24 years was carried out, seeking to base critical analyses on current studies. For the selected studies, the relevance of the research, the quality of the evidence, and the study design were taken into consideration. The data collected were extracted in a methodical and organized manner to speed up the synthesis and analysis process. All data were carefully examined and collated to generate a comprehensive synopsis regarding the toxicity, concentration, and remediation of food-grade BPA. The results are presented succinctly and lucidly using resources such as figures and tables, explaining the main conclusions drawn. The limitations present in the literature and possible future implications for the area are also provided. Finally, the conclusion provides the main findings of the investigation to describe the main gaps existing in the political, social, and environmental spheres in the study area.
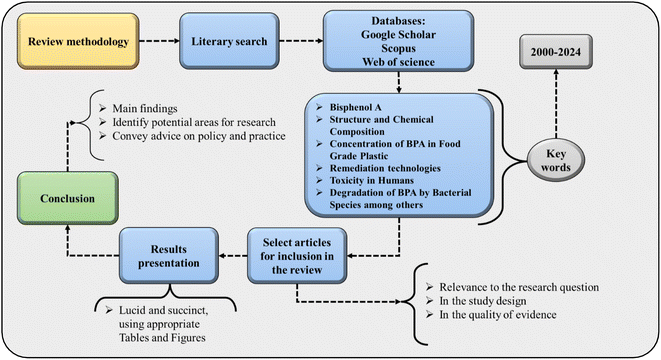 |
| Fig. 1 Steps carried out in the scientific methodology applied in the study. | |
3. Food-grade plastics: bisphenol A concentration and sources
Characteristics such as clarity, resistance to impact and heat, and durability confirm that BPA is the most widely used synthetic compound in environmental quality plastic packaging in the world.46 The major concern is that once present in the environment, BPA is easily leached into groundwater and surface water, especially when exposed to acidic chemical compounds and high temperatures.47 The manufacturing process is one of the main sources of BPA contamination in food-grade plastics.48 In polycarbonate, BPA is applied as a monomer, and this process includes the polymerization of the molecules generating a more durable and robust compound.49 To optimize the durability and resistance, BPA is also applied as an additive in the production of epoxy resins. During these processes, the compound can also be released into the environment. The steps involved in this process are molding and polymerization. The concentration of BPA released from plastics depends on some factors such as acidity, type of plastic, temperature, the manufacturing process used, and duration of exposure time.50 The storage stage and application of food-grade plastic packaging also influence the sources of BPA.51 Since food products are packaged in plastics, depending on storage conditions, BPA can penetrate food, increasing the likelihood of exposure of humans.52 Another point is that many packages until they reach the final consumer are scratched or damaged, which increases the product contamination process.53
The recycling process is also a source of contamination in food-grade plastics, although this also has the positive aspect of reducing waste reduction.54,55 Residual BPA obtained at the manufacturing stage is generally present in the recycled product and can be transported to other products.56 The presence of BPA also occurs in other products (toys, medical and dental devices, and water bottles), which increases the sources of food-grade plastics. The intensity of contamination is high when these products are discarded inappropriately.57 The literature presents several reports that seek to evaluate the concentration of contaminants in food-quality packaging. The results indicate that the quantity varies depending on the pH and temperature, manufacturing process, the substance contained in the container, and the type of plastic. Several studies report its presence in various packaging (water bottles, baby bottles, and food packaging), with the highest concentrations being found in polycarbonate.58,59 Studies have reported the presence of BPA in breast milk samples (Asia).60 A study confirmed that the increase in temperature increases the transfer of BPA from the container to the food, which explains why certain samples contain higher concentrations of BPA.61 Canned products contain a coating that contains epoxy resins. In this investigation, it was observed that the concentration of BPA in foods highly varied, making it possible to detect low and high concentrations.62,63
In the literature, it is possible to observe investigations that indicate that low concentrations of BPA do not cause harm to human health; however, many claim that increasing exposure over a long period can cause serious damage, mainly due to its bioaccumulation capacity, which would increase its concentration.64 Due to this, an increase in research has been observed looking for more ecological and alternative materials to replace BPA in food-grade plastic containers. Some industrial processes are already manufacturing plastic packaging without the presence of BPA; among the alternative materials are stainless steel and glass.65,66 The great concern is not only associated with human health but also with the impact on the environment; open-air landfills, exposed to high temperatures, are one of the main sources of BPA leaching into the water table, which is intensified in countries with low income.67 Because of this, it is highly urgent to seek new, safer alternatives to applying BPA to food-grade plastics. The change in population behavior over the last 20 years has also changed the main sources of BPA release, as a result of which scientific advances have made it possible to determine the main transformation pathways, which are described in Table 1.
Table 1 Main sources of BPA release in the last 20 years and their mechanisms of action in the environment
BPA release activity into the environment |
Transformation mechanism |
Reference |
Migration of polycarbonates |
The transport of BPA into liquid foods occurs through polycarbonate via two routes. After the polymer hydrolysis process is catalyzed via hydroxide (manufacturing), residual BPA is released by diffusion into foods in contact with water and simulant. The polycondensation of BPA using phosgene (a highly toxic product) is responsible for the production of polycarbonate, which is of greater economic interest. During the hydrolysis and diffusion of polycarbonate, BPA can migrate, with hydrolysis being much more impactful in terms of release; this is due to pH values and the contact of other compounds (cations). An example was polycarbonate bottles with water stored and with a use time of seven years, when exposed to high temperatures, BPA quickly went into the water. In addition, the speed and concentration of BPA migrated will depend on the chemical composition of the solution |
68 |
Translocation through microplastics present in water |
In the manufacture of resins and polymers, BPA is classified as a common precursor, where microplastic particles manufactured with low-density polyethylene together with polycarbonate, easily release BPA into water media. Additives added to water resources (toxic organic compounds) include microplastics and intensified contamination |
69 |
Waste management: inefficient treatments |
In effluent treatment plants, BPA is leached into the hydrolytic of plastic waste. Treatment generally achieves maximum efficiency in removing endocrine disruptive compounds (R = 37–94%), contaminating water, soil, and air. The release occurs in the decomposition and wear of plastics. Waste paint from electronics also releases BPA due to burning computer circuit boards. Bacteria (soil/sediment) under anaerobic conditions can degrade in four steps forming tribromobisphenol A, dibromobisphenol A, and monobromobisphenol A, followed by BPA as the final product |
70 and 71 |
Effect of temperature on plastic degradation |
When polymers are subjected to high temperatures, thermal degradation occurs where BPA is synthesized into bis (4-hydroxyphenyl) methane and 4-hydroxybenzoic acid. As a result of this process, the scission of the weak bonds present in the carbonate chain and alcoholysis/hydrolysis are obtained |
72 |
Food packaging and canned food |
Food consumption is the main route of human contamination. Its contact is due to the exposure of animals and the vegetable raw materials used, therefore, bioaccumulation is due to the presence of polymers and food. Studies estimate that consumption via food contaminated with BPA varies from 0.48 to 1.6 g per kg body weight per day, depending on consumption habits, location, and economic and cultural background. The main routes of contamination of water and food are through epoxy resins and polycarbonates |
73 and 74 |
Electronic waste: disposal and recycling areas |
Developed and underdeveloped countries, especially the latter, are concentrated in large spaces that recycle waste from electronic products, which release large amounts of BPA. This liberation has increased in recent decades due to the constant advancement and dependence of society on electronic products. The recycling of these materials is generally done crudely by dismantling the product and separating the electronic parts (this step uses an incinerator at high temperature), the burning process releases many toxic gases into the atmosphere |
75 |
Dentistry: use of orthodontic adhesives |
Processes related to the dental area use raw materials containing high concentrations of BPA, such as orthodontic adhesives based on Bis-GMA. BPA has an estrogenic behavior that is limited to the double-ring benzoic compound. The breakdown of Bis-GMA releases BPA. Widely used in the restoration of resins, being one of the most dangerous polymers released into the environment. BPA occupies around 70% of the composition of Bis-GMA resins used in enamel restoration and dental obstructions. Its toxicity has the potential for cytotoxicity at the cellular level. Poly-BPA molecules present in the oral route can decompose into BPA, this is accelerated by pH conditions, enzymes and chemical substances present, and temperature |
76 |
Epoxy resins |
Prepolymers contain several epoxide groups and are known as epoxy resins. These resins are placed in contact with various curing agents (aliphatic amines, anhydrides, and polyamides), generating cross-linked polymers in industrial processes. Most of the resins are produced with bisphenol-A diglycidyl ether, a very low molecular weight oligomer (the main component of liquid resins) |
77 |
Paper coins |
High levels of BPA were detected in thermal paper (322 g kg−1). During the handling of received products, contamination can occur dermally, as BPA is sprayed on the leaves (powdery layer). When a receipt is placed next to the coin in the cash register or wallet, or even when a receipt is handled before the money, the contaminant can be transported to the paper currency |
78 and 79 |
Use of anti-rust products |
Products that contain epoxy resin are sources of BPA release, among the most common are epoxy coatings with water and high levels of dust and solids, cans that store drinks and food and are coated with protection, primers used in car bodies automobiles, electrical laminates, composites with fiber-based reinforcement, tools, and castings |
80 |
3.1 Exposure to bisphenol A: toxic potential
The commercialization of epoxy resins and the production of polycarbonate plastics are associated with a series of health problems in humans.81–83 The chemical compound can imitate the estrogen hormone (Fig. 2), and hence, studies report that there may be changes in activities related to reproduction, fertility (polycystic ovary syndrome), development, increased incidence of cancer cells, and risk of obesity and diabetes.84 The effects on the neurological system are intensified in children interfering with learning and behavior.85 Children exposed to BPA in the prenatal period showed reduced bone density.86 Exams analyzed children's urine and observed that individuals who had a higher concentration of BPA were more aggressive and hyperactive. Women exposed to BPA in the prenatal period also had a higher rate of mental disorders, such as depression and anxiety. Studies also link the contaminant with disorders in the immune system, decreasing cellular activity and increasing the likelihood of diseases and infections.87 Concerning the digestive system, it was observed that even at low concentrations, BPA causes dysregulation of the intestinal barrier, reducing the microbiota and composition, which affects the immune system.88,89 Studies carried out on animals have shown a greater risk of neurodegenerative diseases (Alzheimer's disease, Parkinson's disease, and amyotrophic lateral sclerosis) when they are exposed to BPA, in addition to behavioral disorders and cognitive abnormalities.90–92 In men, a decrease in semen quality has been observed, directly affecting their fertility.93 Endocrine-disrupting compounds such as BPA affect endothelial function, resulting in the loss of vascular homeostasis and increasing the chances of cardiovascular diseases.94
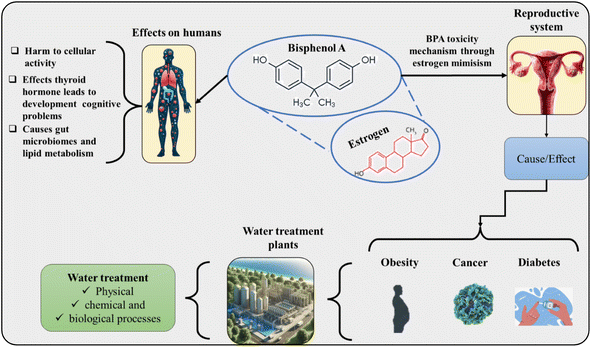 |
| Fig. 2 Mechanism and toxic effects of bisphenol A on human health. | |
The genotoxic effect has been recently observed, which is related to the pro-oxidative potential. The increase in oxidative stress is linked to the increase in the production of species that react with oxygen, the accumulation of oxidation compounds (biomacromolecules), and the change in the activities of antioxidant enzymes.95 This behavior is possible to occur in negative and positive estrogen receptor organisms. Once intracellular oxidation occurs, the chances of DNA damage (double- and single-strand breaks and oxidative damage) increase. Another study describes that BPA, when it also uses non-canonical estrogen as a receptor, is connected to a membrane and the receptor is coupled to the G protein. Therefore, BPA can modulate transcription factors and ion channels, which generates damage such as mitochondrial stress, damage to the endoplasmic reticulum, and damage to the amyloid polypeptide of human islets.96 This causes the activation of NFκB in β cells located in the pancreas, generating unregulated glucagon secretion.96 There are conflicting studies regarding the incidence of asthma in children due to BPA, where three studies show a higher risk of asthma in childhood, and only one reported a low risk.97
Damage is also caused to animals, and in the literature, it is possible to observe studies that analyze the damage caused to the reproductive system of boars and sows and relate it to the levels of oxidative stress in the offspring, the quality of the meat, and muscle metabolism.98 Most studies are related to damage at the level of reproduction (endocrine disruption); however, there is a greater need for analysis regarding the maturation of oocytes and the potential and mechanism of their toxicity in farm animals such as cows and nuts. As in humans, mice that were exposed to BPA showed a reduction in the intestinal microbial composition and richness, where they present distinct metabolic pathways, and thus, the study highlights intestinal toxicity in vertebrates.99 In aquatic organisms, the effects are similar to those generated in humans, and include the following: decreased life expectancy and alteration of fat metabolism,100 alteration of fertility,101 negative regulation of ecdysone genotoxicity,102 ecdysone upregulation,103 endocrine-disrupting cytotoxicity,104 neurodevelopmental toxicity,105 alteration of retinal morphology due to alteration in thyroid hormone levels,106 reproductive alteration genotoxicity,107 modulation of antioxidant enzymes and decreased filtering capacity,108 and physiological and reproductive changes in fish.109
In plants, studies are more limited, however, it is described that BPA generates stress, which produces a large number of species that react with oxygen.110 These new species act as signal messengers and provoke various stress responses in plant antioxidant cells.111 The literature describes that BPA can imitate the cytokine hormone in plants, in addition to the role of ethylene in the oxidative stress process generated by BPA.112 It is also possible to observe studies that describe different changes at both the biochemical and physiological levels in plant cells.87 Generally, biochemical reactions correspond to changes in enzymes at temperatures close to the environment. More studies must be carried out to optimize biomonitoring and describe what the risk situations are. Therefore, plant species that are truly sensitive to different concentrations of BPA must be selected, inserted in a natural habitat, and identified biological changes in different phases of development. This will allow us to obtain answers about the relationship between the BPA dose and the associated effects, corroborating the risk assessments. Due to all these damages, there is still considerable discussion regarding the possible effects generated at low concentrations and long periods of exposure, which strengthens the urgency in finding alternative products for BPA.113,114
3.2 A case study of the fish microbiome and the presence of BPA
Due to the numbers that prove the high human exposure to BPA, the vast majority of studies use animal and human experimental models.115,116 However, it is observed that water compartments can present high concentrations, and this includes both saltwater and freshwater environments. This fact corroborates the detection of BPA in the tissues of fish species in both cases. An example is in the United Kingdom where, due to the disposal of wastewater, the presence of BPA ranging from 37 to 462 ng L−1 was detected in river samples.117 This is not an isolated case, and in the Mediterranean region, the concentrations can reach 30 μg L−1, with a minimum of 4.5 μg L−1.118 The same authors analyzed samples on the northeast coast of the Mediterranean, where a minimum concentration of 8.85 and a maximum of 14.76 μg L−1 (Sea of Marmara and Black Sea) were observed. The authors highlight that the variation is influenced by meteorological variables that correspond to the seasons. Research highlights the influence of the presence of BPA on aquatic biota and its exposure to certain species. The fish species Dicentrarchus labrax, Trachurus, and Scomber colias were analyzed in the northeastern region of the Atlantic Ocean, and both fish tissues showed high levels of BPA proportional to the ingestion of microplastics.119 As a result, several investigations describe that microplastics are one of the main sources of BPA contamination in aquatic environments.120
It is also described that the microbiome of most fish species is potentially sensitive to BPA contamination. Most reports that analyze the impacts of the contaminant on fish are on a laboratory scale using zebrafish as an experimental model. These studies observed that, just as it occurs in mammals, BPA changes the chemical composition and generates behavioral disorders in the living organism.121 Different genera of zebrafish were exposed for 90 days to a concentration of up to 2 μg L−1 of BPA, resulting in a reduction in the pathogenic genus Hyphomicrobium and a significant increase in Lawsonia.122 An impoverishment of the microbiota of the intestinal tract was also reported after the presence of BPA; in contrast, in the host model, a response to oxidative injuries and inflammation was observed. Zebra fish of both sexes were analyzed based on their response to the microbiome in the presence of BPA and its other replacement products, 10 days-old larvae showed a decrease in microbial diversity, especially at higher concentrations.123 BPA derivatives showed diverse effects on the microbiome community. Finally, despite the composition of the microbiota having a behavior opposite to host toxicity, no noticeable effect was observed on the behavior of the larvae.124 A concentration of 2000 μg L−1 was added in contact with adult zebrafish for 35 days. In the end, it was reported that the fish showed an increase in muscle triglycerides and gastrointestinal dysbiosis.125 These studies corroborate the elucidation of how BPA affects the gastrointestinal microbiota of aquatic biota, serving as a support for the comparison of investigations with mammals.126,127
4. Mechanisms of BPA toxicity in food-grade plastics
The scientific community has continuously studied BPA for two reasons: the first is due to its wide use in the most varied industrial processes, and the second is its ability to contaminate food and drinks, generating toxic effects on animals, humans, and plants. The first point is that BPA is considered an endocrine disruptor due to its ability to imitate estrogen. This hormone is fundamental for the functioning of the body's organs and tissues, in addition to being important for the development in the early stages of growth.128,129 Once acting like estrogen, BPA alters the functioning of the endocrine system, generating changes in the activity and hormonal production of the human body. As already mentioned, this action generates several problems, among the most common of which are changes in fertility, diabetes, obesity, and cancer.130 At a cellular level, it alters the cell activities by generating oxidative stress, which can cause damage to membranes, chemical composition, and even ADN.131 Its toxic potential can occur in different signaling pathways in the human body. One example is the disturbance of enzymatic activities involving lipid metabolism, which causes disorders resulting in problems such as diabetes and obesity.132–134 One of the specific receptors present in the human body that BPA can interfere with is that of the thyroid hormone, this causes the interruption of signaling, generating health problems, including behavioral and developmental changes.
A study135 showed that higher levels of thyroid hormone were associated with contact via breast milk in newborns, being more evident in female children. Another point is its change in the activities of microorganisms present in the intestinal tract and digestive system, which causes changes and a possible decrease in the immune system, in addition to altering the dynamics of metabolism and other physiological processes that are involved.91 Therefore, BPA damage can occur through various mechanisms, and its ability to bind to estrogen receptors is the most discussed in the literature, as a result of which changes in cellular functions and microbial activity of the intestinal system are observed, intensified by direct effects on signaling pathways. This entire process causes considerable damage to health, which corroborates the intense efforts to develop new, more sustainable, and safer alternatives to the BPA present in various consumer products. Therefore, a reduction in exposure routes is achieved, reducing damage throughout the food chain.136,137
4.1 Regulatory guidelines and limits for BPA exposure
Due to the serious damage and constant threat of BPA to human health, guidelines have been set and regulatory limits established in some countries. To achieve this, scientific studies were used to protect the society from the potentially harmful effects of the contaminant. The Environmental Protection Agency (APA), located in the United States, decreed 50 μg per kilogram of body weight per day as a reference dose, and this would be the maximum level of exposure considered non-harmful.138 The US Federal Drug Administration also decreed guidelines that limit the exposure to contaminants in products for children's use, such as formula packaging and baby bottles. The guidelines established by the European Union were set by the European Food Safety Authority, where a daily amount of 4 μg per kilogram of body weight per day is allowed. This daily dose indicates the limit that can be exposed without causing harm to health.139 Unlike American legislation, the European Union does not allow the use of the contaminant in cups, straws, and baby bottles. Canada has defined a maximum concentration of up to 0.005 mg of BPA present in water, which is acceptable; however, Health Canada has warned the population about the presence of BPA in baby bottles and baby formula packaging, warning the preference for smaller packaging sustainable. Countries such as Australia, Japan, and China have created guidelines with tolerance limits for BPA in different environments and packaging. Despite public policies that seek to limit contact with BPA, stricter measures are still needed, especially in less developed countries. Together, debates are needed to confirm whether these limits are truly safe for long-term exposure. Therefore, continued research is recommended to obtain a broader understanding of the real potential health risks together with new, more viable, and safer alternatives to BPA.140,141
The presence of BPA in liquids such as beverages generally occurs in products that feature the coating of bottle caps, cans, and other pipes that line the entire water supply.142 As shown in Table 2, when compared to other solid foods, the presence of BPA in beverages is much less reported and at lower concentrations. Two factors can justify this: first the LODs are high in the most varied analytical methods used, and second, the concentration of coating used in beverage cans is lower than that used in solid foods. BPA has never been observed in liquids packaged in glass; however, it has been detected in plastic packaging and other types of canned goods. BPA has been detected in mineral water packaged with polyethylene terephthalate.144 The highly low concentrations suggest that the main sources of BPA are bottle closures.162 The presence of BPA in dairy products can also occur during production due to contact with equipment and other utensils used during food processing. Ruminant feed can also generate BPA contamination, because it contains alkphenols, such as octylphenol and nonylphenol.163 BPA and its derivatives are present in milk, which are presented in Table 2; in this case, the packaging may play a minor role in contamination. This is because variables such as heat treatments and the various utensils used in production can lead to greater contamination. Fatty dairy products that are secreted by cows can have a high presence of BPA. Few studies report the release of BPA throughout the dairy product production chain, and more studies are needed in this regard. In this same sense, more studies are needed regarding BPA in non-canned fruits or vegetables. In the case of canned products, it is possible to observe a considerable and precise estimate regarding their content, as shown in Table 2.155,159,164 In general, canned vegetables constitute a highly heterogeneous group of foods (tomatoes, lentils, and mushrooms), together with water, other preservatives, and even oil in some cases. The variation in these products may reflect a high variation in the concentration of BPA. An earlier study157 observed that people who consume canned vegetables in greater quantities have higher levels of canned vegetables in their urine. Likewise, the presence of BPA in meat occurs due to the migration of the packaging with the solid, and this can be intensified due to the production methods and the animal's diet. Table 2 also describes BPA levels in cereals, which can be detected in plastic packaging and cans; however, more studies must be conducted for these foods.
Table 2 Presence of BPA in various human consumption products
Subcategory |
LOG (ng g−1) |
LOQ (ng g−1) |
Min–max (μg kg−1) |
Country and reference |
Energy and soft cola, drinks, cola, juice, beer |
0.02 |
0.02–8.1 |
— |
Belgium143 |
Water |
0.00075 |
0.00073–0.102 |
— |
Italy144 |
Soft drinks |
0.18 |
0.54 |
0.54–4.98 |
Italy145 |
Soft drinks |
0.10 |
0.40 |
0.4–10.2 |
Greece146 |
Milk |
0.15 |
— |
0.99–2.64 |
Spain 147 |
Milk |
0.30–4.20 |
1–14.00 |
<1–521 |
Italy148 |
Cheese spreads, milk, brown cheese, and hard cheese |
— |
0.10 |
<0.10 |
Norway149 |
Milk |
3 |
9 |
3–169 |
Italy150 |
Soft, energy, and beer |
0.1–9.3 |
— |
0.1–3.4 |
Austria151 |
Beans, haricots, red kidney beans, young peas, crushed tomatoes and lentils |
1.1–7.4 |
— |
8.5–35 |
Austria152 |
Tomato |
15.4–20 |
51.3–66.9 |
20.5–115.3 |
Italy153 |
Carrots, peas, bean shoots, artichokes, and mixed vegetables |
0.016 |
0.55 |
8.90–304 |
Spain 154 |
Green beans, red pepper, asparagus and lentils mushroom |
0.3–1.1 |
0.9–3.5 |
7.1–959 |
Spain 155 |
Tomato |
0.09 |
0.26 |
0.31–235.88 |
Italy156 |
Tomato paste and mushrooms |
0.60 |
1.9 |
4.9–66 |
Greece146 |
Pineapple and peaches |
0.3–1.1 |
0.9–3.5 |
6.1–13 |
Spain 155 |
Meat |
— |
2–4 |
6.9–13 |
Sweden157 |
Tripe meat balls |
0.30–1.10 |
0.90–3.50 |
9.2–341 |
Spain 155 |
Poultry, meat, offals and game, delicatessen meats |
0.02 |
1.2 |
0.09–60.19 |
France158 |
Ravioli, corn |
— |
0.1 |
0.94–73.1 |
Belgium143 |
Pasta, bread, burns, flour, and breakfast cereals |
— |
— |
0.1–0.24 |
Norway149 |
Eggs, oil, products, butter and margarine |
0.21 |
— |
0.045–4.51 |
France159 |
Honey |
270 |
800 |
— |
Italy160 |
Mustard seeds |
10 |
30 |
10–8350 |
Switzerland161 |
Sauces |
0.6 |
1.9 |
— |
Greece146 |
5. Bacterial degradation of bisphenol A
Several studies have been developed, mainly in the last decade, seeking to optimize the BPA decontamination process mainly in water compartments and the soil. An alternative is the application of colonies of different species of bacteria that have the potential to degrade the chemical compound.165 Table 3 describes the variety of species and strains analyzed and their degradation potential. The study by Sasaki et al. (2005), used the species Sphingomonas sp. strain AO1 isolated in a WTP (water treatment plant) located in Asia (Japan). Degradation was efficient since the microorganism used the chemical compound as a carbon source, where intermediates were generated, with a lower toxic potential. The system showed 100% removal for a concentration of 115 μg mL−1. Another study isolated the species Sphingobium sp. BiD32 from sediment and soil samples, the consumption of the chemical compound as the only source of energy allowed degradation through a sequence of chemical reactions, generating water and CO2 (carbon dioxide) as final products.184 Similar degradation results were also obtained with other species such as Rhodococcus sp. and Pseudomonas putida185 and Arthrobacter sp.186 The efficiency of the process at a cost-benefit level depends on the operating conditions of the environment; therefore, the control and manipulation of variables such as pH, temperature, and quantity of nutrients and water is essential. Despite the positive results and many challenges to be overcome, a limitation is ensuring optimal degradation even in the presence of other chemical compounds. Another aspect is the scale of the process, since under real environmental conditions, it requires a large number of bacteria, coupled with the difficult control of environmental variables, which can limit the proliferation of colonies, affecting degradation.
Table 3 Biodegradation of BPA in food-grade plastics by bacteria
Bacterial strains |
Concentration |
Bisphenol A removal (%) |
Reference |
KU-3 bacterial strains (Chennai coastal region, India) |
250–1000 ppm |
74 |
166 |
K-6 bacterial strains (Chennai coastal region, India) |
250–1000 ppm |
78 |
167 |
KU-8 bacterial strains (Chennai coastal region, India) |
250–1000 ppm |
81 |
167 |
Aeromonas hydrophila |
60–120 mg L−1 |
79 |
168 |
Chlorella vulgaris |
20 mg L−1 |
>50 |
169 |
Sphingomonas sp. strain AO1 |
115 μg mL−1 |
100 |
170 |
Bacillus sp. GZB |
10 mg L−1 |
100 |
171 |
Bacillus pumilus |
10 ppm |
100 |
172 |
Pseudomonas putida YC-AE1 |
1000 mg L−1 |
93 |
173 |
Bacillus sp.GZ |
100 mg L−1 |
93 |
174 |
Pseudomonas putida KT2440 |
— |
46 |
175 |
Pseudomonas sp. LBC1 |
160 μM |
100 |
176 |
Bacterial consortium |
50 mg L−1 |
90 |
177 |
Sphingobium sp. BiD32 |
2 mg L−1 |
10 |
178 |
Pseudoxanthomonas sp. strain NyZ600 |
50 mg L−1 |
45 |
179 |
Sphingomonadaceae |
5000 μg L−1 |
99 |
180 |
Bacillus sp. AM1 |
25 ppb |
84 |
181 |
Dracaena sanderiana |
100 mg L−1 |
99 |
182 |
Sphingomonas sp. strain AO1 |
115 μg mL−1 |
100 |
170 |
Chlorella pyrenoidosa |
30 mg L−1 |
67 |
183 |
5.1 Degradation mechanism
The process of degradation of the BPA molecule by bacteria involves several routes, which allow the molecule to be broken down into less toxic and simpler compounds. These routes encompass non-enzymatic and enzymatic processes and include a diversity of colony species. The production of BPA hydrolase enzymes is one of the main mechanisms and consists of the ability of these enzymes to break the bonds that unite the BPA molecule.187 Hydrolases are produced by bacteria that develop when using BPA as a source of energy and carbon. These enzymes were found in several colonies (Sphingobium japonicum, Pseudomonas putida, and Rhodococcus sp.) that degraded the compound efficiently.188–190 Non-enzymatic processes are also observed, among which biotransformation and adsorption stand out. In the case of adsorption, the interaction of BPA (adsorbate) molecules occurs, which are attracted via physical interactions to the surface of the bacteria cells, followed by absorption and degradation. Biotransformation is related to chemical changes that occur in the contaminant through the activity of enzymes produced by bacteria, and the products generated in the end are less complex and less toxic.191 In this process, metabolic pathways are formed that enable the use of the compound as a source of nutrients. In the literature, the species Pseudomonas putida uses BPA as the sole source of energy and carbon; however, the species Sphingobium japonicum consumes the compound as part of a broader energy and carbon system.192,193
The degradation of BPA and the mechanisms used by bacteria may vary depending on the species used and the environmental conditions (pH, temperature, humidity, etc.) in which they were found. In general, the entire mechanism occurs through a combination of non-enzymatic and enzymatic processes, in which BPA bonds are broken.194,195 Achieving total control over the mechanisms that involve degradation is fundamental in planning new strategies that aim to remedy and mitigate the environmental damage generated by the release of BPA. With the correct species and the mechanisms that the process involves, researchers can achieve maximum efficiency and the best cost-benefit in applying the method.196
Owing to the increasing contamination and exposure to compounds containing BPA, there is a growing need and urgency for remediation technologies such as bacterial degradation.197,198 The colonies of bacteria with the potential to degrade BPA into less toxic compounds have been reported in diverse environments including sediments, treatment plants, and soil samples. Once new intermediate compounds are formed, they can be reused or discarded in safe places.199 With these practices, it is possible to reduce the volume of waste from plastics and microplastics, corroborating with less exposure, mainly to less toxic chemical molecules. An area of research that encompasses degradation by bacteria and the management of solid waste is the use of bioreactors, and these structures provide the best environmental conditions for rapid colony growth through accelerated metabolism.200 Bioreactors can isolate cultures where BPA is then added for subsequent degradation.201 Another natural process is composting. These cases also make use of microorganisms that decompose organic material (garden waste and fruit and vegetable waste). This technique also makes it possible to break down BPA molecules, supporting the management of plastic waste and consequently reducing the environmental impact and mitigating harmful effects on humans, plants, animals, and aquatic organisms.202
These processes still need to overcome gaps such as, for example, isolating a certain species or colony capable of degrading several chemical compounds as well as its identification. This is the first step towards achieving ideal conditions for rapid metabolism and the application of a practical and cost-effective method that can be applied to large-volume BPA waste management.203 To overcome these barriers, degradation with bacteria has great potential in the application of bioreactor development, including the possibility of composting. These are still possible alternatives and examples of sustainable activities in combating contamination by solid waste.
6. Overview of technologies for the remediation of BPA-contaminated water
6.1 Physical processes
Physical treatment processes such as adsorption, membrane filtration, and electrocoagulation are commonly used to remove BPA contaminants from water and wastewater due to their flexibility, simplicity, and effectiveness. However, methods other than adsorption have drawbacks such as high energy and maintenance costs, lower removal capabilities, and increased risk of membrane fouling.204 Coagulation or flocculation treatment, a physicochemical process, is employed to remove BPA that attaches to sludge during the primary treatment stage followed by the secondary treatment stage in wastewater treatment.205 However, the effectiveness of these methods is limited, and its highest recorded removal record rate is 1%. In the secondary treatment stage, BPA is subjected to advanced processes, including adsorption, oxidation, advanced oxidation, and membrane technologies which have high removal efficiency. Among the water treatment processes, coagulation or flocculation is the simplest technology with low installation costs.206 Over the past decade, adsorption has emerged as the most widely used method for removing BPA. The raw materials for adsorbents, such as graphene, activated carbons, zeolites, and agricultural wastes, are diverse, inexpensive, and easy to maintain. These materials have proven to be promising for large-scale BPA removal applications.207 Managing adsorbents effectively is essential for the efficient operation of the adsorption process. This includes selection, storage, regeneration, and treatment. Such comprehensive management significantly affects the absorbent's service life, reduces costs, and improves the overall efficiency.208 Successfully employing adsorption methods for BPA removal requires thorough technical preparation. This includes smooth operation of equipment and diligent monitoring of parameters such as adsorbent saturation, temperature, and flow rate. Continuous assessment ensures efficient BPA removal.209 Advancements in adsorption techniques promise cost-effective BPA removal. Successful implementation depends on innovation in materials/methods and meticulous management/monitoring throughout the process.
6.1.1 Membrane processes. Membrane separation processes (MSPs) are advanced treatments gaining popularity in water and sewage treatment plants for achieving high water purity levels and facilitating water reuse. These processes encompass two primary mechanisms: sieving and adsorption.210 Since the 1990s, membrane filtration has gained a lot of interest as a drinking water treatment technology due to its ability to achieve high throughput in a continuous operation mode. There are four main types of the process: reverse osmosis (RO), nanofiltration (NF), ultrafiltration (UF), and microfiltration (MF).211 NF and RO are usually utilized in removing organic carbon, dissolved solids, and inorganic ions. They can also be used in removing EDCS and micropollutants.212 They are operated at high pressures when compared to MF and UF membranes.213 As much as 60 million m3 per day of water is being treated by RO, while MF and UF have capacities of 20 million m3 per day, accounting for 60% of total drinking water production.214 UF is more popular than the other types of membrane separation technologies because it is cheaper, and can handle particles, macromolecules, suspended solids, colloids, bacteria, and viruses.215 Both NF and RO membranes can effectively remove various types of micropollutants (MPs) not only via charge effects and adsorption but also through size exclusion.216 Various types of membranes employed in treatments of BPA are presented in Table 4.
Table 4 Various membrane technologies and their removal efficienciesa
Membrane |
Type |
Parameter condition |
Remove efficiency |
Reference |
NDA: no data available. |
Layer-by-layer (LBL) biocatalytic |
NF |
85 cm2; pH 6; operation pressure, 2 bar; temperature, 25 ± 0.5 °C, salt concentration, 1000 ppm; crossflow velocity, 0.2 m s−1 |
92.5% |
(Li et al., 2020)223 |
Polyamide NF membrane |
NF |
2–3 cm (water level), 25 °C, pH = 7–9.7, 200 rpm for 2 min |
88.5% |
(Wang et al., 2020)224 |
Dynamic electrodeposited CuO/carbon membrane (DECuO/CM) |
MF |
RhB concentration 300 mg L−1, voltage 2.5 V, electrode distant 5.75 cm, flow rate 1.2 mL min−1, electrolyte (Na2SO4) concentration 5 g L−1, and feed pH 5.3 |
98.04% |
(Li et al., 2020)225 |
PVDFMW catalytic-membrane |
MF |
12 cm2, pH: 7.0, 531.6 eV |
40% |
217 |
PVC membrane |
UF |
35 cm2, 300 mL, 100 kPa for 10 min |
60% |
218 |
PES-SiO2 hollow fibre membranes |
UF |
1 bar, pH: 7, feed concentration: 10 and 100 μL−1 |
95% |
211 |
Forward osmosis membrane |
Forward osmosis |
15 bar, permeate flow of 1.6 mL min−1, concentrate flow of 80 mL min−1 and a RO flux of 7 L m−2 h−1 |
40% |
219 |
TW30 membrane |
RO |
15 bar, 10 μg L−1, pH: 7 |
84% |
220 |
TW30-4040, polyamide |
RO |
NDA |
90% |
221 |
MF (0.2 μm) and UF (0.05 μm) may retain BPA by the interaction of the chemical with particulates, as the particulate's size is larger than the pore sizes of MF and UF membranes.222 Up to 92.5% BPA removal efficiency was achieved due to the combined effect of laccase catalysis, membrane rejection, and adsorption.223 This performance was obtained under optimal conditions of 2 bar pressure, competitive laccase loading (238.8 ± 3.5 μg cm−2), and laccase activity (0.6 U cm−2) (Li et al., 2020).223 Wang et al., (2020)224 proved that in the treatment of Songhua river water spiked with micropollutants, the percentage removal of BPA significantly increased to 88.5%, surpassing the efficiency of single NF without coagulation, which achieved only 60.7% removal. After the fourth usage cycle, Li et al., (2020)225 observed a remarkable 99.8% removal efficiency for BPA using combined electrocatalytic oxidation and MF. The DECuO/CM membrane, featuring a permeability of 823.03 L (m2 h bar)−1, was fabricated by depositing CuO onto the carbon membrane. The integration of PAA/PVDF membranes with the catalytic process using nZVI/H2O2 under optimal conditions (50 L m−2 h−1, 10 mM of H2O2) led to a notable 80% improvement in BPA treatment efficiency.217
UF is achieved through pores ranging between 5 and 20 nm in size, with molecular weight cutoffs of 1000 to 100
000 Da.226 In both MF and UF processes, higher BPA concentrations in the feed solution led to increased adsorption efficiency. The estimated BPA removal rates were 36–72% for MF and 45–67% for UF.227 To enhance the membrane performance, researchers have incorporated inorganic materials during membrane fabrication alongside MWCNTs and PVC-II. Under optimal conditions of 35 cm2, 300 mL, and 100 kPa for 10 minutes, BPA degradation reached 60%. Additionally, the researcher noted that the retention of BPA decreases with the increase in pressure.218 Tian et al., (2021)228 developed a piezoelectric membrane using SnS2 nanosheet-coated carbon nanofibers (CNFs) to generate hydroxyl free radicals for BPA degradation under ultrasound. They found the highest BPA adsorption with the UF membrane, followed by NF and RO membranes. The efficient reduction of BPA degradation was achieved with 97% efficiency within 80 minutes, respectively, through piezocatalysis of 0.5-SnS2/CNFs under ultrasonication in the darkness. However, Muhamad et al., (2016b) demonstrated that the UF membrane system effectively removes BPA, with the membrane surface containing SiO2 nanoparticles and hydroxyl bonding groups of BPA. The variations in feed pH notably influenced BPA elimination, achieving the highest rejection (90%) at pH 7 and the lowest removal (20%) at pH 10. The optimization process of the model identified the following optimum conditions for BPA removal: 1 bar pressure, pH 6.7, 10 μg L−1 BPA concentration, and 10 minutes of filtration, resulting in 99.61% removal efficiency. Data from the RSM design confirmed that BPA removal under these optimum conditions was consistent at 99.61%, aligning with experimental values obtained in the UF membrane system.229
RO, primarily used for desalination due to its effective particulate rejection capabilities, has been suggested as an additional step in sewage treatment. Multiple research articles have identified RO as the most promising and efficient method for removing organic micropollutants (OMPs), including BPA.230 In terms of forward osmosis rejection efficiency for BPA, the polyamide thin-film composite membrane (PA-TFC) achieved 25% removal, while the polysulfone (PSf) membrane substrate demonstrated 91% removal from the feed solution.231 The concentrate produced from municipal wastewater recycling processes, known as reverse osmosis concentrate (ROC), presents notable environmental and health hazards due to its elevated levels of harmful compounds, such as phenolic chemicals like BPA. Under optimal conditions of 25 °C, a [Fe(VI)]/[BPA] ratio of 50, and an initial pH of 8.0 in the ROC, BPA degradation surpassed 90% after a 90 minutes reaction period.232 However, Moreira et al. (2019a) reported BPA removal from water via RO membrane separation. The RO membrane showed superior efficiency in removing BPA from samples containing 10 μg L−1 at 15 bar pressure, and the removal efficiency rate was 84%.
6.1.2 Adsorption. Adsorption is the transfer of adsorbate from the liquid or gas phase to the surface or interface of the adsorbent, representing a surface phenomenon. Adsorption serves as a primary mechanism facilitating the removal of BPA through membrane filtration, along with size exclusion and charge repulsion. This process is anticipated to be influenced by hydrophobic interactions and hydrogen bonding. Notably, adsorption has demonstrated efficacy owing to its affordability, simplicity, environmentally friendly operation, the availability of various adsorbents, and the potential for renewal and reusability.233 The adsorption rate depends on adsorbent properties, adsorbate nature, and solution conditions. Factors affecting adsorption include temperature, experimental parameters (e.g., time, pH), characteristics of adsorbate and adsorbent, and the presence of other pollutants.234 Researchers utilize various adsorbents such as zeolites, clay minerals, nanomaterials, graphene, activated carbons, imprinted polymers, biopolymers, and agricultural wastes for removing BPA from water. The effectiveness depends on their adsorption capacity.235 Adsorbents are grouped into natural ones (e.g., chitosan, clays), activated carbons, graphene-based materials, nanomaterials, composite materials, imprinted polymers, agricultural wastes, hybrid particles, and inorganic-organic-modified bentonite. However, challenges including difficulty in separating very small-sized materials from the treated matrix remain.236The performance of various adsorbents for BPA uptake is summarized in Table 5. The ρ-phenylenediamine-functionalized magnetic graphene oxide (PPD-MGO) nanocomposite nanomaterial exhibited good adsorption ability for BPA and good re-usability. Under optimum conditions of 45 °C and pH 7, the maximum adsorption capacity reached 155.0 mg g−1. The removal rate was 99.2% after three times of adsorption with the new nanomaterials.251 Under Langmuir models, self-flocculated powdered activated carbon (PAC-PNIPAM) exhibited the maximum adsorption capacity of BPA by 247.532 and 116.298 mg g−1 when conducted at 25 °C and 40 °C, respectively.252 Chen et al. (2015) reported that the maximum adsorption capacities of BPA by the Langmuir model were 182 mg g−1 at pH 5.0 and 196 mg g−1 at pH 7.0, respectively.253 The adsorption of BPA onto activated carbons was employed at 298 K and pH 7.0. Carbons W20 and W20N had higher adsorption capacities (382.12 and 432.34 mg g−1) compared to the other tested carbons. Adsorption capacity was influenced by acidic oxygen-containing groups and surface charge density.254 BPA removal from aqueous solutions via graphene adsorption showed a maximum adsorption capacity (qm) for BPA of 182 mg g−1 at 302.15 K, the highest reported value among carbonaceous adsorbents.255 The optimal operating parameters for the degradation of BPA were determined: 0.3 mL of H2O2, pH 3, and 180 min, in the presence of Fe3O4@illite. The maximum degradation capacities of 816 mg g−1, 364 mg g−1, and 113 mg g−1 for epoxy BPA concentrations in resin wastewater (266 mg L−1), synthetic wastewater (80 mg L−1), and Hefei City Swan Lake (25 mg L−1), respectively.256 Moreover, Hernández-Abreu et al. (2020)236 demonstrated that activated carbons (F400 and KLP) had significantly higher maximum BPA adsorption capacities (400 and 220 mg g−1, respectively) compared to xerogel (78 mg g−1). This difference was probably due to the greater microporous volume observed in F400 and KLP-activated carbons. Similarly, at an initial BPA concentration of 100 mg L−1, the maximum adsorption capability of 285 mg g−1 is 9.3 times higher than that of commercially available activated carbon adsorbents.250 When the BPA concentration exceeded 342 mg L−1, the adsorption capacity of multi-walled carbon nanotubes modified with iron oxide and manganese dioxide (MWCNTs-Fe3O4–MnO2) reached saturation. The static adsorption capacities were 76.75 mg g−1 for MWCNTs, 44.52 mg g−1 for MWCNTs-Fe3O4, and 132.89 mg g−1 for MWCNTs-Fe3O4–MnO2.246 Activated carbon (AC) efficiently removes endocrine-disrupting chemicals from water. It shows high removal rates of BPA even at low initial concentrations (1 mg L−1; pH: 6.5; adsorbent: 0.2 g L−1) and increased substantial adsorption capacity for BPA, estimated at 254.9 ± 16.2 mg g−1.238
Table 5 BPA treatments using various adsorbents materials
Adsorbent |
Detection of BPA |
Performance |
Reference |
Calcium alginate/activated carbon (A-AC) |
FESEM, TGA, XRD, FTIR, BET |
368.3 mg g−1 |
237 |
Activated carbon |
N2 adsorption, desorption isotherm, FTIR, zeta potential |
100% |
238 |
Activated carbon |
TEM, VSM, EDS, XRD, XPS, FTIR |
100% |
239 |
Xerogel (RFX), a chemically activated carbon from kraft lignin (KLP), commercial activated carbon (F400) |
SEM, FTIR |
F400 = 407 mg g−1, KLP = 220 mg g−1, xerogel = 78 mg g−1 |
236 |
Cellulose acetate (cigarette butt) activated carbon |
FTIR, Raman, TGA, XRD, BET, SEM and XPS |
364.21 mg g−1 |
240 |
ρ-Phenylenediamine-functionalized magnetic graphene oxide nanocomposites (PPD-MGO) |
TEM, FT-IR, VSM |
155.0 mg g−1 (99.2%) |
241 |
Biomass-activated carbon (Tithonia diversifolia) |
SEM and XRD |
15.69 mg g−1 |
242 |
nZVI-chitosan |
FTIR, TEM, XRD, SEM |
65.16 mg g−1 |
243 |
Phosphonate Halomonas levan (PhHL) |
FTIR, XPS |
26.6 mg g−1 |
244 |
Sulfonic acid functionalized carbonaceous adsorbent (TW-SO3H) from tea leaves |
FTIR, SEM, EDS, TGA, EDS, TGA, Raman spectroscopy, zeta potential |
236.80 mg g−1 |
245 |
Modification of multi-walled carbon nanotube with iron oxide and manganese dioxide (MWCNTs-Fe3O4 MnO2) |
SEM, TEM, EDX, FTIR, VSM |
132.9 mg g−1 |
246 |
Calcium alginate/activated carbon (A-AC) |
pHPZC, FTIR, SEM |
368.3 mg g−1 |
247 |
Cu-BDC MOFs, Cu-BDC@GrO (graphene oxide) |
XRD, TEM, SEM, EDS, Raman, FTIR, TGA, XPS, Zetasizer and ICP-OES |
182 mg g−1 |
248 |
Magnetic vermiculite modified (MV) poly(trimesoyl chloridemelamine) (MP) |
FTIR, SEM, EDX |
273.67 mg g−1 |
249 |
Nitrogen-containing covalent organic framework (PyTTA-Dva-COF) |
QM/MM, XPS |
285 mg g−1 |
250 |
Transmission electron microscopy (TEM), Fourier transform infrared (FT-IR) spectroscopy, vibrating sample magnetometry (VSM), X-ray diffraction (XRD), X-ray photoelectron spectroscopy (XPS), thermogravimetric analysis (TGA), energy-dispersive X-ray spectroscopy (EDS), zero-point charge determination (pHPZC), inductively coupled plasma-optical emission spectroscopy (ICP-OES), and quantum mechanics/molecular mechanics (QM/MM) were performed.
6.1.3 Coagulation/flocculation treatment. Electrocoagulation involves passing an electric current through water, generating coagulant precursors through the electrolytic oxidation of anode materials, usually aluminum or iron. This technology effectively reduces pollutant levels. The performance of various electrocoagulation systems for BPA treatment is summarized in Table 6. Kumar et al. (2022)257 reported that an electrocoagulation (EC) device equipped with aluminum blades was used to eliminate BPA, resulting in the removal of approximately 42% of the compound. Locust bean gum (LBG) was utilized for coagulation/flocculation as a pre-treatment to reduce BPA levels in leachate prior to advanced treatments. It was reported that at a pH of 7.5, an LBG dosage of 500 mg L−1 resulted in a reduction efficiency of 76% for BPA.266 In the modified coagulation sludge process, a BPA concentration of 20 mg L−1 underwent complete degradation within 120 minutes at room temperature, with a pH of 7, a dosage of 3 g L−1 for PMS, and a dosage of 0.3 g L−1 for MCS.267 According to Dolatabadi et al. (2021), under optimal conditions—namely, a BPA concentration of 3.25 mg L−1, a current density of 12.0 mA cm−2, a pH of 8.5, a reaction time of 23 minutes, and an electrical energy consumption of 0.308 kW h m−3—the maximum removal efficiency of BPA from the aqueous environment reached 98.2%.260 The efficacy of electrocoagulation employing iron and aluminum electrodes for eliminating phenol from aqueous surroundings was investigated. The maximum removal efficiencies of phenol were achieved at 60 V, 80 minutes, 5 mg L−1, 3000 μS cm−1, and pH levels of 5 and 7 with aluminum and iron electrodes, respectively. The removal efficiencies were reported at 94.72% for aluminum electrodes and 98.0% for iron electrodes, respectively.268,269 Ambauen et al. (2020) reported a 99% removal of BPA within the experimental duration of 240 minutes using both tested anode materials (Pt and BDD) and at both tested temperatures (6 and 20 °C), with a current efficiency applied at 86 mA cm−2, and BDD where 43 mA cm−2 was employed. Parastar et al. (2018a)270 reported that under the optimal conditions (10 mg L−1, 1500 mA, pH 7, and 0.5 cm), the removal efficiency observed varied between 60% and 90%. This research study concludes that employing the electrocoagulation process with iron electrodes under these optimal conditions can effectively remove BPA from aqueous solutions.
Table 6 Studies on BPA removal via coagulation/flocculation
Method used |
Operation condition |
Removal efficiency |
Reference |
Peroxy electrocoagulation |
45 ppm, 0.1 g per L Na2SO4, 125 g per L H2O2, pH: 2, 0.11 mA cm−2, 45 min |
80.48% |
258 |
Photocatalysis |
10 mg L−1, TiO2@MIL-101(Cr), 240 min |
99.4% |
259 |
Electrocoagulation |
3.25 mg L−1, pH: 8.5, 12.0 mA cm−2, 23 min, 0.308 kW h m−3 |
98.2% |
260 |
Photocatalytic |
5.0 mg L−1, pH 5.0, 500 W, 0.3 g L−1, 180 min |
100% |
261 |
Electro-fenton |
10 ppm, 10, 25 mg L−1, pH: 2.9, Na2SO4, Fe2SO4, 50 min |
>90 |
262 |
Membrane electrochemical microfiltration |
2.0 V, 50 ppm, 0.1 mol L−1 Na2SO4, 0.88 min |
97% |
263 |
Microwave-Mn-fenton |
100 ppm, 34.0 mg L−1 H2O2, 300.0 W, 6.0 min, pH: 4 |
99.7% |
264 |
Iron electrode electrocoagulation |
10 mg L−1, 25 °C, 30 V, pH = 7, 1500 mA |
90% |
265 |
6.2 Chemical processes
In wastewater treatment, the primary treatment mostly functions by the sorption of BPAs onto sludge via coagulation/flocculation and subsequent sedimentation.271 However, chemical treatment is also equipped to enhance the BPA removal efficiency. Some of the treatments include ultraviolet irradiation and ozonation,222 which are summarized in Table 7. Sulfate radical (SO4˙−)-based advanced oxidation processes (AOPs) have gained considerable interest in recent years for their capacity to effectively remove recalcitrant organic molecules, including BPA, in water and wastewater due to their strong oxidation properties. SO4˙− has a high standard redox potential (2.5–3.1 V) at a neutral pH and shows greater sensitivity towards certain organic contaminants.284 SO4˙− can be generated by activating persulfate (PS) or peroxymonosulfate (PMS) using many processes including heat, transition metals, alkaline conditions, and ultraviolet (UV) irradiation.282 The activation by UV irradiation was also considered to be a very successful and promising technique.285 The degradation rate of BPA by UV irradiation reached 270 ± 6/10−3 min−1 with 55% removal from 0.44 mmol L−1 initial concentration.282 Furthermore, the ozonation method, which involves a redox mechanism, also has several advantages including high removal efficiency, non-selectivity, and disinfection effects.222 BPA can be destroyed by a direct reaction with molecular ozone or indirect reaction with hydroxyl radicals, which are generated from the alkaline breakdown of ozone. Hydroxyl radicals are a more powerful oxidant with lesser selectivity, as compared to molecular ozone. Umar et al.272 reported that ozonation achieved up to 100% of BPA removal from water with the initial concentration of a maximum of 0.5 mg L−1. Out of all the methods, photocatalytic degradation offers an unlimited potential for BPA degradation, especially when it comes to the choice of the nanocomposite materials.279 Table 7 shows that the photocatalytic degradation of BPA reached more than 90% efficiencies and can be achieved within a short period.
Table 7 Previous studies on the chemical process involved in the removal of BPA
Treatment unit |
Performance removal |
Reference |
Ozonation |
100% |
272 |
Ozonation |
17–100% |
273 |
Ozonation |
40% |
274 |
Ozonation |
33% |
275 |
Ozonation |
21.5% |
276 |
Photocatalytic degradation (Bi2WO6/g-C3N4/BPQDs) |
95.6% |
277 |
Photocatalytic degradation (CPD-TiO2) |
99% |
278 |
Photocatalytic degradation (Fe–TiO2) |
99.9% |
279 |
Photocatalytic degradation (Fe–TiO2/rGO) |
99.9% |
279 |
Photocatalytic degradation (MIL-100(Fe)/PDI) |
100% |
280 |
Photodegradation |
50–52% |
281 |
Photodegradation |
58% |
274 |
UV irradiation |
270 ± 6/10−3 min−1 55% |
282 |
UV irradiation |
25–52% |
283 |
UV irradiation |
75–98% |
281 |
6.3 Integrated processes
Integration treatment was used to enhance the removal of contaminants from various contaminated water or to encounter issues that arise with the single treatment. Integrated or hybrid systems were developed to meet the regulations, such as those, for law enforcement removing substances and producing drinkable water. Another advantage of hybrid systems is their ability to address the limitations of procedures by incorporating alternative methods.286 Table 8 shows the integration treatment for the removal of BPA. The integration treatment involved a combination of physical, chemical, and biological processes and also the sequential of the single process. From Table 8, most integration studies showed a great removal of BPA. However, the integration of chemical and biological processes represents only 58.23% of removal. However, this removal percentage surpasses the treatment of 21.59% and 14.48% achieved with Phanerochaete chrysosporium alone and the Fenton treatment, respectively.
Table 8 Previous studies on the integration process involved in the removal of BPA
Integration |
Process |
Performance removal |
Reference |
Physical and chemical |
Ceramic membranes incorporating manganese-cobalt oxides (MCOs) to catalyze the ozone-based degradation |
90.6% |
287 |
Membrane filtration coupled with advanced oxidation processes (AOPs) and adsorption. Ceramic membrane filtration coupled with PMS activation and adsorption |
— |
288 |
Ultrafiltration-catalysis membrane |
95% |
289 |
Adsorption and catalytic ozonation: MWCNTs/Fe3O4 |
25 to 75 ppm (98%) |
290 |
Physical and biological |
Adsorption and biodegradation |
98.1% at 5 mg per L BPA |
291 |
Membrane-assisted enzyme treatment |
89% removal of BPA |
292 |
Chemical and biological |
Fenton process and Phanerochaete chrysosporium |
58.23% at 1 mg per L BPA |
293 |
MBR and ozonation |
90% removal of BPA 80 min at 8 mg O3 per L |
294 |
Sequential treatment of biological reactors |
An up-flow anaerobic sludge blanket (UASB), submerged aerated biological filters (SABF), and horizontal subsurface flow constructed wetland (HSSF-CW) reactors |
99% |
295 |
According to Tarafdar et al., (2022), the effective strategies that can be employed to eliminate BPA are biodegradation and adsorptive processes.296 However, there is a need to address the issues of increased adsorption ability and rapid adsorption rates for both conventional and non-conventional adsorbents.297 Abu Hasan et al., (2023) reviewed the proposed biological treatment and adsorption for BPA concentrations in the effluent that exceeds 1 mg L−1.286 For concentrations below 1 mg L−1, the utilization of an advanced oxidation process (AOP) and MF may be more appropriate, enabling load reduction and consequently decreasing operational and maintenance expenses. Godiya and Park (2022)297 stated that the integration of membrane separation with additional oxidation/biodegradation methods has the potential to mitigate contamination and mass transfer constraints. According to Zielińska et al. (2019), the integration of chemical and biological gained widespread interest in removing BPA from contaminated water.298 These methods primarily involve the integration of Advanced Oxidation Processes (AOPs) such as photocatalysis, ozone oxidation, Fenton and photo-Fenton oxidation, and wet air oxidation (WAO) supplementing the conventional biological treatment. Fundamentally, an effective treatment approach for the elimination of particular EDCs should involve the integration and optimization of sophisticated physical, chemical, and biological treatment techniques.299 It is essential to conduct an exploration and investigation of every advanced treatment process to identify any unidentified potential limitations.
7. Breakdown/removal mechanisms of BPA in water
The remediation process of BPA present in water can be understood from three points of view. The first involves the biodegradation mechanism, the second corresponds to the removal of mass transfer (adsorption) and the third is the degradation of the molecule by advanced oxidative processes.
7.1 Adsorption mechanism
The adsorption of BPA and the mechanisms that govern this process depend on some factors including the nature of the pollutant, the chemical and physical properties of the solid, and the adsorption results. The morphology and structure analyzed through characterization techniques (adsorbent) are also necessary to determine the mechanism.300 The coprecipitation method was used to develop magnetic nanoparticles decorated with graphene oxide nanosheets, and this material was the BPA adsorbent.301 The main interaction that occurred between the graphene skeleton and the benzene ring of the pollutant was π–π. In another study, graphene-coated magnetic iron oxide nanoparticles were used to remove BPA, and the fabricated aerogel (Fe3O4/GE) was subsequently reduced to ethylenediamine.302 The interactions were π–π, hydrogen bonding, and electrostatic, both present between the adsorbate ring and the conjugated carbon network (Fig. 3). The Fe3O4/GO adsorbent was obtained by the hydrothermal method where the BPA ring interacted with the surface via π–π interactions, and sp3 hybridization also corroborated the remediation process.303 In summary, we can see that the nature of the interactions can be widely varying. However, BPA does not breakdown per se but is attached to the adsorbent surface via these physico-chemical interactions and thus removed.
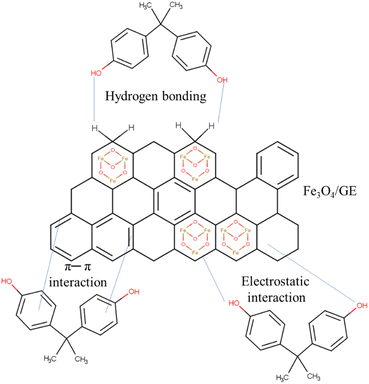 |
| Fig. 3 Representation of the BPA adsorption mechanism onto graphene coated with Fe3O4 nanoparticles. | |
7.2 Membrane/nano-filtration mechanism
Interactions such as hydrophobicity, hydrogen bonding, and electrostatic interactions are essential in the remediation of BPA in membrane filters or even nanofilters. One study used a synthetic effluent with BPA, which was remediated in a submerged membrane bioreactor.304 The researchers reported that almost complete removal (99%) was obtained at a low volumetric loading rate (0–21.6 g per m3 per day). Biodegradation and adsorption mechanisms were responsible for the efficient result for the membrane use. The adsorption process showed endothermic behavior favorable to high temperatures and hydrophobic character, with van der Waals and electrostatic interactions. These were present between the BPA molecules and the reactor sludge. Electrospinning was used to obtain a 6,6-nanofibrous membrane for BPA remediation.305 The maximum capacity of 91 mg g−1 was obtained via hydrogen interactions between the adsorbate hydroxyl and the membrane carbonyl and hydrophobic interactions. BPA is nanoscale in size, so size exclusion through the nanofibrous membrane could not support any removal. In another study, a nanofibrous membrane carrying the laccase enzyme was synthesized on polyethersulfone and polyethersulfone membranes to remove BPA.306 The hydrophilic nature of the laccase membrane enabled 90% removal, being governed by hydrogen bonds of the amine group present in the membrane with BPA. Hydrophobic interaction between polyethyleneimine/polyethersulfone and the adsorbate and electrostatic interaction with the cationic group of the polyethyleneimine/polyethersulfone and the pollutant molecule. The mechanism of reverse osmosis and nanofiltration membranes was analyzed concerning BPA removal.307 The authors highlight that membranes made of polyamide present greater rejection of BPA when compared to those made of cellulose acetate. The mechanisms correspond to hydrogen bonding, electrostatic, and hydrophobic interaction (Fig. 3).
7.3 Photocatalytic degradation and its mechanism
The photodegradation of BPA involves mechanisms that correspond to the generation of electron holes present in the conduction band and also the electrons contained in the valence zone after the irradiation step.308 Studies have concluded that depending on the type of photocatalyst, the oxidation of the valence band and hydroxyls are mainly responsible for the degradation.309 Therefore, the hydroxyl initiates the degradation by directly attacking the carbon present in the phenyl group, and then 4-isopropylphenyl and hydroquinone are generated as intermediates. In the case of 4-isopropylphenyl, it is again converted to 4-(2-hydroxypropan-2-yl) phenol, and then, after hydroxylation and dehydration, it generates 4-hydroxybenzoic acid and 4-hydroxybenzaldehyde (Fig. 4). Researchers describe that the products can also undergo oxidation producing various organic acids that are also degraded, finally forming products that are not toxic such as water and CO2.310,311
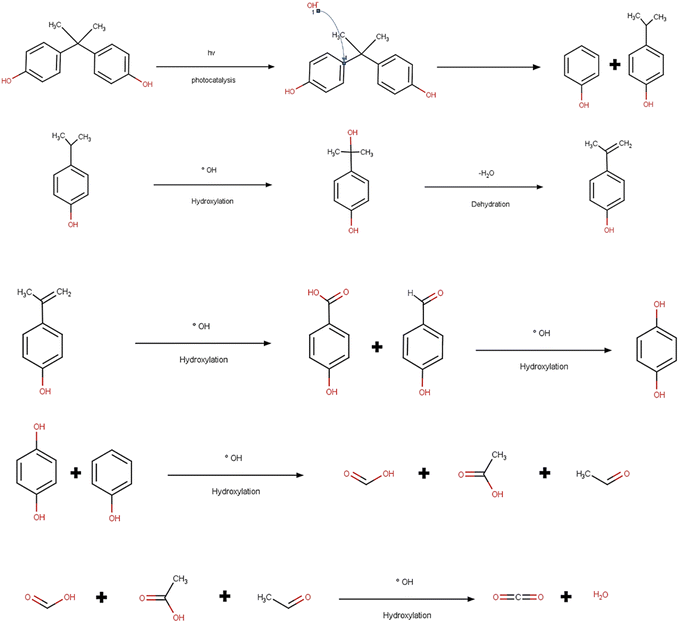 |
| Fig. 4 Schematic representation of the photocatalytic degradation process of BPA. | |
7.4 Advanced oxidation processes and its mechanism
Due to its properties, ozone is highly oxidizing and is present in the oxidation of several organic compounds, such as BPA. The reactions can be indirect or direct. The oxidation process of BPA and molecular ozone occurs in a basic environment through an indirect reaction, while in an acidic environment, decomposition and oxidation occur through the elimination of radicals in a direct reaction. Molecular ozone also attacks structures rich in electrons (non-protonated amines, activated aromatic compounds, and unsaturated bonds such as C
C). The presence of phenolic groups increases the chances of a reaction. In this sense, degradation via ozonation obtained complete decomposition and 30% mineralization of BPA under acidic conditions.312,313 After 25 min, the stoichiometric ratio between ozone and BPA was 10.3. The production of ozonoids and the attack of ozone on C
C were the main mechanisms involved. Malonic and oxalic acids accelerate the degradation of ozonides, corroborating with simultaneous mineralization. A nanocomposite (AC/CeO2/ZnO) was synthesized to remove BPA.314 Under optimal conditions, total carbon removal was observed (time of 60 min, pH of 8, and 500 μg per L catalyst dose) with the use of five degradation products. OH radicals were dominant in the process and increased under basic conditions. The generation of organic acids with the addition of OH reduced the pH. Under pH conditions of 11, BPA removal was 100% using pumice that deposited 4 g L−1 of O3/nTiO2/H2O2 and 12 g L−1 of O3/nZrO2/H2O2 in 30 min of contact.315 The increase in peroxide at first increased the generation of OH, improving BPA removal; in a second moment, the efficiency reduced due to the generation of fewer oxidizing peroxide radicals. The catalytic degradation of BPA was analyzed with the Fe3O4–MnO2 catalyst via ozonation.316 Under conditions close to neutrality and 100 mL min−1 flow rate, approximately 97% of BPA was removed. Regarding the specific mechanism, it should be noted that different mechanisms were proposed. Zhang et al. (2020) proposed that the ozonation process leads to the decomposition of the BPA (Fig. 5) and generates 11 intermediates before the ring cleavage and generation of carbon dioxide and water.316
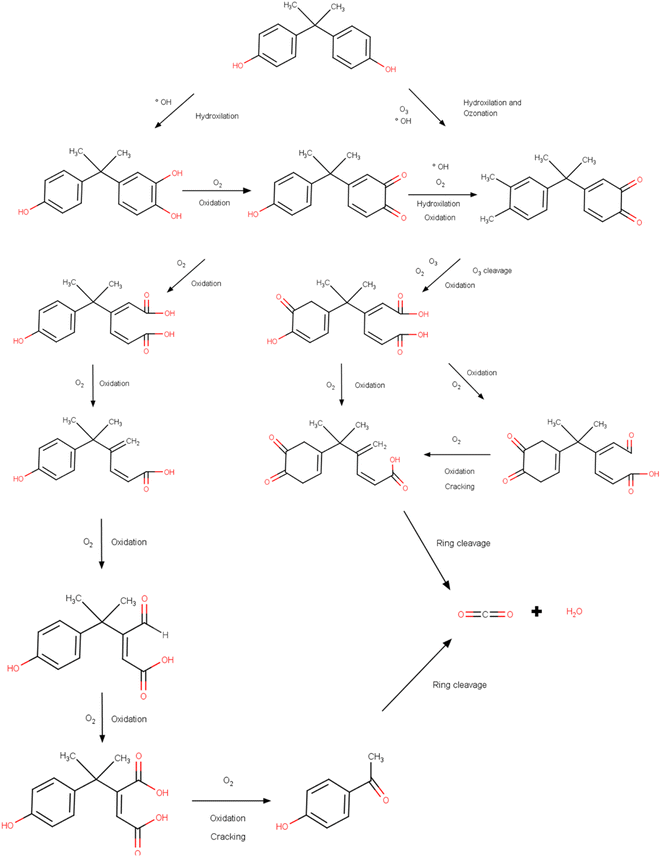 |
| Fig. 5 BPA decomposition mechanism in the presence of Fe3O4–MnO2 and ozone. | |
7.5 Electrochemical degradation and its mechanism
In a pulsed plasma discharge system containing activated carbon, 95% was obtained through electrochemical degradation in one hour of reaction and with a 20 kV discharge.317 In the presence of oxygen and acidic conditions, the degradation was superior. First, the process began with attacks of the hydroxyls and the cleavage of the isopropylidene bridge. The electrophilic substitution reaction by O* led to the oxidation of the pollutant, and then the products generated by the oxidation were transformed into single-ring aromatic compounds. Then, the cleavage of the ring generated oxalic, formic, and acetic acids, and finally, they were mineralized into carbon dioxide and water. Graphite/β-PbO2 anodes and granular activated carbons were used to remove BPA.318 The maximum efficiency of 99% was obtained via two mechanisms that use OH. One of them starts with the loss of the hydroxyl group and a ring-opening reaction leading to the final production of acetic acid and ethane. In the second pathway, the beginning occurs by the oxidation of 4-(2-hydroxypropan-2-yl) phenol with the sequence of ring opening producing carbon dioxide and water at the end. An experiment also studied electrochemical peroxidation to remediate BPA present in groundwater.319 The BPA electrochemical degradation can be summarized as given in Fig. 6. It starts with the BPA hydroxylation, due to the OH radicals present. Due to the instability of the new intermediate, cleavage occurs at the isopropylidene bridge, generating one-ring compounds. These new aromatic groups undergo the cleavage process, which are further mineralized onto CO2 and H2O.320,321
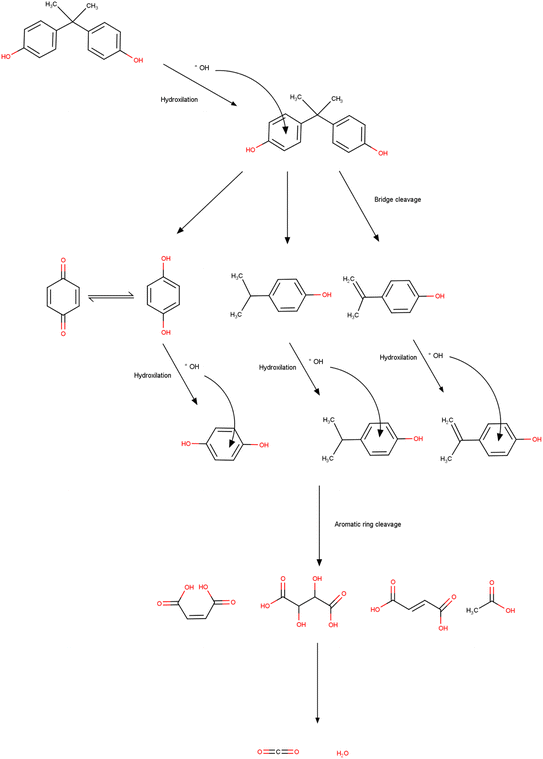 |
| Fig. 6 BPA electrochemical degradation routes. | |
7.6 Photo-fenton process and its mechanism
The BPA degradation mechanism through the photo-fenton process has been reported by different authors. da Silveira Salla et al.322 have proposed the mechanism of BPA in the presence of CuFeS2 and visible light, as given in Fig. 7. First, the BPA is converted into hydrolyxated bisphenol, due to the ˙OH radicals. After that the ˙OH radicals tend to interact once again by promoting the BPA bridge cleavage. Leading to the formation of 4-isopropylphenol, 4-hydroxyacetophenone, 2-methoxyhydroquinone, and 4-(1-hydroxy-1-methylethyl)benzene-1,2-diol. The later intermediates suffer from another hydroxylation, which leads to the ring opening and the formation of acids in the form of oxalic, fumaric, and isobutryic acids. Last, the acids are mineralized forming CO2 and H2O. Other differences generated on the mechanism pathway can be attributed to the catalyst employed. Zhu et al. (2018) indicate that the catalyst based on Ag/AgCl nanoparticles onto ferrite can produce electron–hole pairs due to the surface plasmon resonance.29 These electron pairs are further transferred to the ferrite, which increases the conversion of Fe3+ into Fe2+. The latter also affects the number of radicals produced due to Fe2+ reacting with the peroxide (H2O2). The same mechanism was also confirmed by Liu et al.323 when they employed Ag/AgCl/Ferrite-S, indicating that the electron–hole pair generation migrates to the conduction band before acting in the Fe3+-to-Fe2+ conversion mechanism.
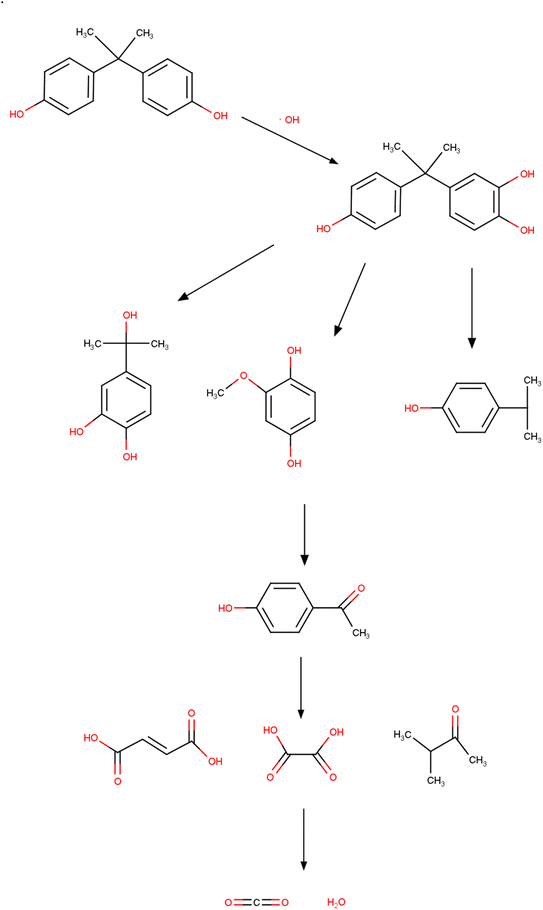 |
| Fig. 7 BPA degradation mechanism for the photo-Fenton reaction. | |
7.7 Biodegradation and its mechanism
Following the other mechanism trends, the degradation of BPA has similar routes regarding the hydroxylation and bridge/aromatic cleavage; however, new routes tend to emerge due to the microorganism present. The removal of BPA in biodegradation mechanisms involves aerobic and anaerobic conditions, together with the action of microorganisms. The use of Bacillus sp. cultivated in anaerobic and aerobic media was applied in the remediation of BPA.324 In this study, the strain that was extracted obtained the best performance with approximately 51% mineralization using 5 mg L−1 of pollutant concentration. The aerobic process was the most efficient, generating approximately 5 final products. The entire process was dominated by electron acceptors such as Fe3+, SO42−, and NO3 for the anaerobic system, while the acceptors of the aerobic system were O2. The acceptors SO42− and NO3 did not influence biodegradation; however, Fe3+ was the most efficient when added to the anaerobic system. Despite the good performance, the rate was not higher than that obtained in the system containing oxygen. In another study, the strain YC-JY1 of Sphingobium sp. was obtained to remove BPA; the results show that at a pH of 6.5, degradation was maximum.325
Golshan et al.326 investigated the BPA degradation mechanism in the presence of several microorganisms. From all the investigated microorganisms, the author evaluated Sphingomonas sp. as given in Fig. 8. At a temperature of 30 °C, the process involved two mechanisms, the first of which corresponds to the conversion of the pollutant into 1,2-bis(4-hydroxyphenyl)-2-propanol, which is then transformed into 4-hydroxybenzaldehyde and 4′hydroxyacetophenone, for final use by the bacteria, and the second mechanism corresponds to the conversion of BPA, sequentially generating the accumulation of conversion products (process medium). These products correspond to the same ones obtained in the degradation and photocatalytic biotransformation of BPA described previously. The addition of salt-tolerant species corresponds to the biological treatment carried out with the concentration of 50 mg L−1 of pollutant, contained in a sequential batch reactor with saline wastewater.326
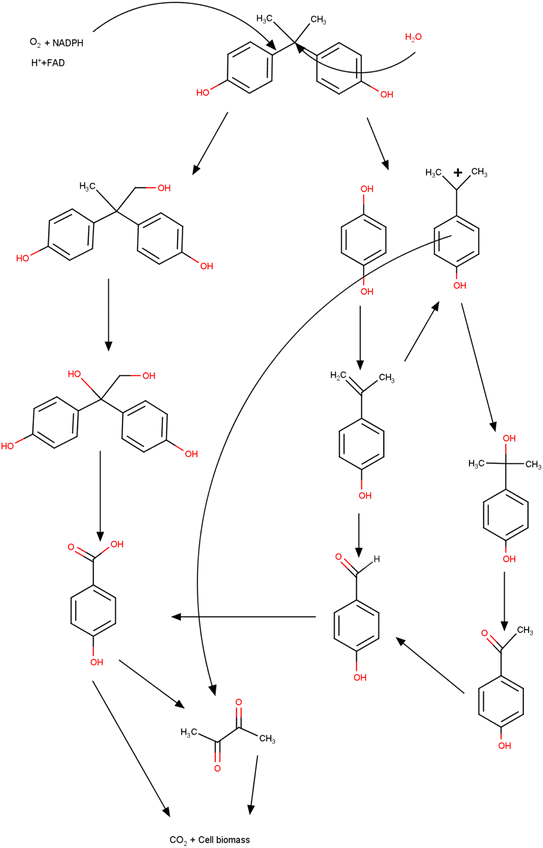 |
| Fig. 8 BPA biodegradation routes with Sphingomonas sp. present in the solution. | |
Similarly, two biodegradation processes that correspond were also found by Wang et al.327 The first is the cleavage of the C
C bond and hydroxylation. In the latter, BPA is first transformed into 2,3-bis(4-hydroxyphenyl)-1,2-propanediol and 2,2-bis(4-hydroxyphenyl)-1-propanol, which are mineralized in cell biomass and carbon dioxide. The second corresponds to the cleavage step of the C
C bond, where degradation begins in the benzene ring and oxidative cleavage, and aliphatic acids are subsequently generated, which are mineralized in cell biomass and carbon dioxide. In another study, green algae Desmodesmus sp. was used to detoxify and biodegrade BPA.327 The mechanisms encompass three dynamics. The first corresponds to the oxidative cleavage of the pollutant to monophenols, followed by their mineralization. The second corresponds to hydroxylation generating monohydroxybisphenol-A, followed by hydroxymethylation forming 2-hydroxy-3-hydroxymethylbisphenol-A. The third is glycosylation (a biochemical process that consists of the addition of sugars to proteins, lipids, or other organic compounds, resulting in the formation of glycoconjugates).
8. Challenges and future directions
When searching the phrase ‘BPA’, it is observed that it is a highly studied compound and that it presents a large number of studies, ranging from its presence in the environment, entry routes, and toxic potential in animals, humans, aquatic life and plants, to biological, physical, and chemical processes for their environmental mitigation. All of these efforts and interests are related to their constant presence and release into the environment, due to the means of production and the contemporary culture of consumerism. When analyzing toxic effects, a vast field is observed, where different pathways in the human body can be affected, where it presents a strong correlation with other factors, such as concentration, exposure time, and route of contact. Another aspect is the age at which humans come into contact with BPA. In this sense, it is clear that babies and children in the development phase are the most affected.
Based on this, the implicit mechanisms require better understanding and more investigations, this is one of the challenges that BPA remediation processes face. More reliable technologies for detecting and measuring plastic exposure are also needed. The complexity lies in the different routes of exposure due to its presence in various products, so it can be through dermal contact or oral or olfactory ingestion. Experiencing the availability of highly accurate methods of measuring exposure makes it possible to estimate the level of danger and determine the best interventions to achieve high efficiency in reducing damage. More studies are also needed to analyze and describe the damage along the food chain and prolonged exposure to low concentrations of BPA. Although it is possible to observe studies that show that low concentrations do not present a health risk, other researchers highlight the cumulative potential of BPA over years of exposure. Only by understanding long-term damage and at low concentrations is it possible to establish safe guidelines and regulations by authorities through competent bodies. Among the countless challenges to be overcome, there is also the field that seeks to develop new, more sustainable materials that can replace BPA in the most diverse consumer products. The points observed by the researchers are economic viability and health risk, an example being vegetable polymers. Even if BPA were completely replaced, its presence in the environment would persist for a long time; therefore, more studies are needed to describe its impacts at the aquatic and plant level. These studies must go hand in hand with new BPA mitigation strategies.
Bacterial degradation has been shown to be 45–100% efficient depending on the strain of bacteria and the choice of process parameters. To obtain total optimization of the biodegradation process using bacteria, it is necessary to carry out continuous investigations in different regions of the world, as meteorological variables are different and have a high influence on colony growth. The seasons must also be taken into account as well as the presence of other chemical molecules in addition to BPA. Regarding other remediation strategies, BPA can be removed from water by physical processes such as membrane filtration, adsorption, and coagulation and by chemical processes such as ozonation and photocatalytic degradation. We see that membrane processes can remove 40–95% BPA in water with a high level of throughput. Other physical processes such as adsorption and coagulation can remove up to 100% BPA in water but throughput is significantly lesser. Chemical processes such as ozonation and photocatalytic degradation have achieved similar performance levels. However, we believe the use of hybrid/integrated processes can simultaneously achieve high removal efficiency at high water throughput due to the intensification of the process. It is encouraged that researchers explore various hybrid technologies for BPA removal from water with the aim of achieving process efficiency and scalability.
9. Conclusion
A detailed analysis of the literature was conducted to determine the toxicity levels and concentration of BPA in environment-quality plastic packaging. The degradation process using different species of bacteria made it possible to describe the damage to humans, animals, and plants that BPA can cause through prolonged exposure, and the role that these organisms play. It was observed that food-grade packaging contains considerable concentrations of BPA, a utilization route that had inadvertently led to biomagnification and its ubiquitous nature in the aqueous environment. This has increased the need for remediation techniques to reduce its incidence in the aqueous environment. Researchers should focus their efforts on providing a better understanding of the mechanisms of the degradation of BPA and its toxic effects on the ecosystem. It was observed that physical processes are able to achieve >90% removal efficiency of BPA from water but tend to perform better under a low pollutant load in the influent stream. Chemical processes show wider variation in performance with 20–100% removal efficiency, while integrated processes show 90–100% removal efficiency of BPA from water. It is highly necessary to obtain new routes to replace BPA in various consumer products, without it losing its quality. Therefore, it is highly important to explore new solutions by developing efficient and viable strategies for plastic waste management. In soil, BPA can be effectively remediated by bacterial degradation. Aqueous phase remediation of BPA can be achieved with processes such as membrane filtration, adsorption, coagulation, ozonation, and photocatalytic degradation. It was proposed that hybrid/integrated processes are a strategy that can help achieve high removal efficiency at high wastewater throughput.
Ethical statement
This article does not contain any studies involving human or animal subjects.
Data availability
No primary research results, software or code has been included and no new data were generated or analysed as part of this review.
Conflicts of interest
The authors declare that there are no conflicts of interest.
Acknowledgements
Fig. 3–8 were designed using the MarvinSketch software.
References
- E. B. Jadhav, M. S. Sankhla, R. A. Bhat and D. S. Bhagat, Microplastics from food packaging: An overview of human consumption, health threats, and alternative solutions, Environ. Nanotechnol., Monit. Manage., 2021, 16, 100608 CAS.
- A. Wanag, A. Sienkiewicz, P. Rokicka-Konieczna, E. Kusiak-Nejman and A. W. Morawski, Influence of modification of titanium dioxide by silane coupling agents on the photocatalytic activity and stability, J. Environ. Chem. Eng., 2020, 8(4), 103917 CrossRef CAS.
- C. Monneret, What is an endocrine disruptor?, C. R. Biol., 2017, 340(9–10), 403–405 CrossRef.
- E. B. Jadhav, M. S. Sankhla, R. A. Bhat and D. S. Bhagat, Microplastics from food packaging: An overview of human consumption, health threats, and alternative solutions, Environ. Nanotechnol., Monit. Manage., 2021, 16, 100608 CAS.
- E. R. Kabir, M. S. Rahman and I. Rahman, A review on endocrine disruptors and their possible impacts on human health, Environ. Toxicol. Pharmacol., 2015, 40(1), 241–258 CrossRef CAS PubMed.
- C. Sonnenschein and A. M. Soto, An updated review of environmental estrogen and androgen mimics and antagonists, J. Steroid Biochem. Mol. Biol., 1998, 65(1–6), 143–150 CrossRef CAS.
- H. R. Lee, E. B. Jeung, M. H. Cho, T. H. Kim, P. C. K. Leung and K. C. Choi, Molecular mechanism(s) of endocrine-disrupting chemicals and their potent oestrogenicity in diverse cells and tissues that express oestrogen receptors, J. Cell. Mol. Med., 2013, 17(1), 1–11 CrossRef CAS.
- J. J. Heindel, B. Blumberg, M. Cave, R. Machtinger, A. Mantovani and M. A. Mendez, et al., Metabolism disrupting chemicals and metabolic disorders, Reprod. Toxicol., 2017, 68, 3–33 CrossRef CAS.
- Marine Anthropogenic Litter, ed. M. Bergmann, L. Gutow, M. Klages, M. Anthropogenic Litter, M. Bergmann, L. Gutow and M. Klages, Springer International Publishing, Cham, 2015, pp. 1–447 Search PubMed.
- J. N. Hahladakis, C. A. Velis, R. Weber, E. Iacovidou and P. Purnell, An overview of chemical additives present in plastics: Migration, release, fate and environmental impact during their use, disposal and recycling, J. Hazard. Mater., 2018, 344, 179–199 CrossRef CAS PubMed.
- T. S. Horan, H. Pulcastro, C. Lawson, R. Gerona, S. Martin and M. C. Gieske, et al., Replacement Bisphenols Adversely Affect Mouse Gametogenesis with Consequences for Subsequent Generations, Curr. Biol., 2018, 28(18), 2948–2954 CrossRef CAS PubMed.
- D. Chen, K. Kannan, H. Tan, Z. Zheng, Y. L. Feng and Y. Wu, et al., Bisphenol Analogues Other Than BPA: Environmental Occurrence, Human Exposure, and Toxicity - A Review, Environ. Sci. Technol., 2016, 50(11), 5438–5453 CrossRef CAS.
- Q. Li, F. Pan, W. Li, D. Li, H. Xu and D. Xia, et al., Enhanced adsorption of bisphenol a from aqueous solution with 2-vinylpyridine functionalized magnetic nanoparticles, Polymers, 2018, 1136 CrossRef.
- G. K. Deshwal and N. R. Panjagari, Review on metal packaging: materials, forms, food applications, safety and recyclability, J. Food Sci. Technol., 2020, 57(7), 2377–2392 CrossRef PubMed.
- C. Bach, X. Dauchy, M. C. Chagnon and S. Etienne, Chemical compounds and toxicological assessments of drinking water stored in polyethylene terephthalate (PET) bottles: A source of controversy reviewed, Water Res., 2012, 46(3), 571–583 CrossRef CAS.
- R. Proshad, T. Kormoker, M. S. Islam, M. A. Haque, M. M. Rahman and M. M. R. Mithu, Toxic effects of plastic on human health and environment : A consequences of health risk assessment in Bangladesh, Int. J. Health, 2017, 6(1), 1–5 CrossRef.
- K. V. S. Rajmohan, C. Ramya, M. Raja Viswanathan and S. Varjani, Plastic pollutants: effective waste management for pollution control and abatement, Curr. Opin. Environ. Sci. Health., 2019, 12, 72–84 CrossRef.
- C. D. Kassotis, L. N. Vandenberg, B. A. Demeneix, M. Porta, R. Slama and L. Trasande, Endocrine-disrupting chemicals: economic, regulatory, and policy implications, Lancet Diabetes Endocrinol., 2020, 8(8), 719–730 CrossRef CAS.
- O. O. Ayeleru, S. Dlova, O. J. Akinribide, F. Ntuli, W. K. Kupolati and P. F. Marina, et al., Challenges of plastic waste generation and management in sub-Saharan Africa: A review, Waste Manage., 2020, 110, 24–42 CrossRef PubMed.
- O. Banerjee, S. Singh, I. Saha, S. Pal, M. Banerjee and S. Kundu, et al., Molecular dissection of cellular response of pancreatic islet cells to Bisphenol-A (BPA): A comprehensive review, Biochem. Pharmacol., 2022, 201, 115068 CrossRef CAS PubMed.
- T. S. Horan, H. Pulcastro, C. Lawson, R. Gerona, S. Martin and M. C. Gieske, et al., Replacement Bisphenols Adversely Affect Mouse Gametogenesis with Consequences for Subsequent Generations, Curr. Biol., 2018, 28(18), 2948–2954 CrossRef CAS.
- M. Y. Lee, I. Ahmed, K. Yu, C. S. Lee, K. K. Kang and M. S. Jang, et al., Aqueous adsorption of bisphenol A over a porphyrinic porous organic polymer, Chemosphere, 2021, 265, 129161 CrossRef CAS PubMed.
- T. Geens, L. Goeyens, K. Kannan, H. Neels and A. Covaci, Levels of bisphenol-A in thermal paper receipts from Belgium and estimation of human exposure, Sci. Total Environ., 2012, 435–436, 30–33 CrossRef CAS.
- B. E. Tokula, A. O. Dada, A. A. Inyinbor, K. S. Obayomi, O. S. Bello and U. Pal, Agro-waste based adsorbents as sustainable materials for effective adsorption of Bisphenol A from the environment: A review, J. Cleaner Prod., 2023, 388, 135819 CrossRef CAS.
- S. Karuppaiah, R. Annamalai, A. Muthuraj, S. Kesavan, R. Palani and S. Ponnusamy, et al., Efficient photocatalytic degradation of ciprofloxacin and bisphenol A under visible light using Gd 2 WO 6 loaded ZnO/bentonite nanocomposite, Appl. Surf. Sci., 2019, 481, 1109–1119 CrossRef CAS.
- A. Selvakumar and S. Rangabhashiyam, Biosorption of Rhodamine B onto novel biosorbents from Kappaphycus alvarezii, Gracilaria salicornia and Gracilaria edulis, Environ. Pollut., 2019, 255, 113291 CrossRef CAS.
- O. Onyekwere, C. J. Okonkwo, A. B. Okoroafor and C. J. Okonkwo, Occurrence and risk assessment of phenolic endocrine disrupting chemicals in shallow groundwater resource from selected Nigerian rural settlements, Ovidius Univ. Ann. Chem., 2019, 30(2), 101–107 CrossRef CAS.
- J. N. Okereke, O. I. Ogidi and K. O. Obasi, International Journal of Advanced Research in Biological Sciences Environmental and Health Impact of Industrial Wastewater Effluents in Nigeria-A Review, Int. J. Adv. Res. Biol. Sci., 2016, 3(6), 55–67 CAS.
- H. Zhu, Z. Li and J. Yang, A novel composite hydrogel for adsorption and photocatalytic degradation of bisphenol A by visible light irradiation, Chem. Eng. J., 2018, 334, 1679–1690 CrossRef CAS.
- J. Corrales, L. A. Kristofco, W. Baylor Steele, B. S. Yates, C. S. Breed and E. Spencer Williams, et al., Global assessment of bisphenol a in the environment: Review and analysis of its occurrence and bioaccumulation, Dose-Response, 2015, 13(3), 1–29 CrossRef CAS PubMed.
- S. Karuppaiah, R. Annamalai, A. Muthuraj, S. Kesavan, R. Palani and S. Ponnusamy, et al., Efficient photocatalytic degradation of ciprofloxacin and bisphenol A under visible light using Gd 2 WO 6 loaded ZnO/bentonite nanocomposite, Appl. Surf. Sci., 2019, 481, 1109–1119 CrossRef CAS.
- C. C. Wu, J. N. Shields, C. Akemann, D. N. Meyer, M. Connell and B. B. Baker, et al., The phenotypic and transcriptomic effects of developmental exposure to nanomolar levels of estrone and bisphenol A in zebrafish, Sci. Total Environ., 2021, 757, 143736 CrossRef CAS PubMed.
- N. Shobnam, Y. Sun, M. Mahmood, F. E. Löffler and J. Im, Biologically mediated abiotic degradation (BMAD) of bisphenol A by manganese-oxidizing bacteria, J. Hazard. Mater., 2021, 417, 1–7 CrossRef.
- M. Sarkhosh, M. Sadani, M. Abtahi, H. Azarpira, H. Alidadi and Z. Atafar, et al., Photo-biological degradation of Bisphenol A, UV/ZnO/Iodide process at the center of biological reactor, J. Photochem. Photobiol., A, 2019, 374, 115–124 CrossRef CAS.
- R. Frankowski, J. Płatkiewicz, E. Stanisz, T. Grześkowiak and A. Zgoła-Grześkowiak, Biodegradation and photo-Fenton degradation of bisphenol A, bisphenol S and fluconazole in water, Environ. Pollut., 2021, 289, 117947 CrossRef CAS PubMed.
- J. Sharma, I. M. Mishra and V. Kumar, Mechanistic study of photo-oxidation of Bisphenol-A (BPA) with hydrogen peroxide (H2O2) and sodium persulfate (SPS), J. Environ. Manage., 2016, 12–22 CrossRef CAS.
- G. A. Ashraf, R. T. Rasool, S. Al-Sulaimi, R. U. Rasool, N. Hassan and Z. Ajmal, et al., Construction of type-II scheme SnO@HfC photocatalyst for bisphenol A degradation via peroxymonosulfate activation; DFT and self-cleaning analysis, Chemosphere, 2023, 341, 140095 CrossRef CAS PubMed.
- F. Cheng and J. Wang, Removal of bisphenol a from wastewater by adsorption and membrane separation: Performances and mechanisms, Chem. Eng. J., 2024, 484, 149414 CrossRef CAS.
- A. Shishov, D. Israelyan and A. Bulatov, Automated deep eutectic solvent-based chromatomembrane microextraction: Separation and preconcentration of bisphenols from aqueous samples, Sep. Purif. Technol., 2024, 338, 126480 CrossRef CAS.
- R. J. Pan, J. Wu, J. Qu, T. Zhang, F. Z. Jiao and M. Zhao, et al., Peak-like three-dimensional CoFe2O4/carbon nanotube decorated bamboo fabrics for simultaneous solar-thermal evaporation of water and photocatalytic degradation of bisphenol A, J. Mater. Sci. Technol., 2024, 179, 40–49 CrossRef CAS.
- L. Tian, C. G. Goodyer, J. Zheng and S. Bayen, Thermal degradation of bisphenol A and bisphenol S in water and fish (cod and basa) fillets, Food Chem., 2020, 328, 126999 CrossRef CAS.
- S. Tiwari, C. L. Gehlot, K. Srivastava and D. Srivastava, Simulation of the thermal degradation and curing kinetics of fly ash reinforced diglycidyl ether bisphenol A composite, J. Indian Chem. Soc., 2021, 98(6), 100077 CrossRef CAS.
- S. Rani, H. Sabharwal, P. Kumar, A. K. Chauhan, K. S. Khoo and N. Kataria, Comparative behavior of carbon-based materials for the removal of emerging bisphenol A from water: adsorption modelling and mechanism, Groundw. Sustain. Dev., 2024, 25, 101121 CrossRef.
- T. Ahmad, M. S. Manzar, S. Khan, M. A. Al-Sharafi, J. Georgin and D. S. P. Franco, et al., Enhanced adsorption of bisphenol-A from water through the application of isocyanurate based hyper crosslinked resin, J. Mol. Liq., 2024, 395, 123861 CrossRef CAS.
- S. A. H. Shah, M. R. Ramachandran, S. A. Mansur, N. M. Saleh and S. Asman, Selective recognition of bisphenol a using molecularly imprinted polymer based on choline chloride-methacrylic acid deep eutectic solvent monomer: Synthesis characterization and adsorption study, Polymer, 2023, 283, 126279 CrossRef CAS.
- S. Chouhan, S. K. Yadav, J. Prakash, Swati and S. P. Singh, Effect of Bisphenol A on human health and its degradation by microorganisms: A review, Ann. Microbiol., 2014, 64(1), 13–21 CrossRef CAS.
- C. Kubwabo, I. Kosarac, B. Stewart, B. R. Gauthier, K. Lalonde and P. J. Lalonde, Migration of bisphenol A from plastic baby bottles, baby bottle liners and reusable polycarbonate drinking bottles, Food Addit. Contam.: Part A, 2009, 26(6), 928–937 CrossRef CAS.
- C. Campanale, C. Massarelli, I. Savino, V. Locaputo and V. F. Uricchio, A detailed review study on potential effects of microplastics and additives of concern on human health, Food Addit. Contam.: Part A, 2020, 17(4), 1212 CAS.
- R. M. Cywar, N. A. Rorrer, C. B. Hoyt, G. T. Beckham and E. Y.
X. Chen, Bio-based polymers with performance-advantaged properties, Nat. Rev. Mater., 2022, 7(2), 83–103 CrossRef CAS.
- Marine Anthropogenic Litter, ed. M. Bergmann, L. Gutow, M. Klages, M. Anthropogenic Litter. M. Bergmann, L. Gutow and M. Klages, Springer International Publishing, Cham, 2015, pp. 1–447 Search PubMed.
- J. Muncke, Exposure to endocrine disrupting compounds via the food chain: Is packaging a relevant source?, Sci. Total Environ., 2009, 407(16), 4549–4559 CrossRef CAS.
- V. Mustieles, S. C. D'Cruz, S. Couderq, A. Rodríguez-Carrillo, J. B. Fini and T. Hofer, et al., Bisphenol A and its analogues: A comprehensive review to identify and prioritize effect biomarkers for human biomonitoring, Environ. Int., 2020, 144, 105811 CrossRef CAS PubMed.
- M. A. Coniglio, C. Fioriglio and P. Laganà, Non stances in PET-Bottled Mineral Water, Springerbriefs in Molecular Science, 2020, pp. 1–66 Search PubMed.
- P. Singh and V. P. Sharma, Integrated Plastic Waste Management: Environmental and Improved Health Approaches, Procedia Environ. Sci., 2016, 35, 692–700 CrossRef CAS.
- J. N. Hahladakis, C. A. Velis, R. Weber, E. Iacovidou and P. Purnell, An overview of chemical additives present in plastics: Migration, release, fate and environmental impact during their use, disposal and recycling, J. Hazard. Mater., 2018, 344, 179–199 CrossRef CAS.
- D. K. Schneiderman and M. A. Hillmyer, 50th Anniversary Perspective: There Is a Great Future in Sustainable Polymers, Macromolecules, 2017, 50(10), 3733–3749 CrossRef CAS.
- J. O. Tijani, O. O. Fatoba, O. O. Babajide and L. F. Petrik, Pharmaceuticals, endocrine disruptors, personal care products, nanomaterials and perfluorinated pollutants: a review, Environ. Chem. Lett., 2016, 14(1), 27–49 CrossRef CAS.
- J. E. Cooper, E. L. Kendig and S. M. Belcher, Assessment of bisphenol A released from reusable plastic, aluminium and stainless steel water bottles, Chemosphere, 2011, 85(6), 943–947 CrossRef CAS PubMed.
- C. Kubwabo, I. Kosarac, B. Stewart, B. R. Gauthier, K. Lalonde and P. J. Lalonde, Migration of bisphenol A from plastic baby bottles, baby bottle liners and reusable polycarbonate drinking bottles, Food Addit. Contam.: Part A, 2009, 26(6), 928–937 CrossRef CAS.
- L. M. Iribarne-Durán, F. M. Peinado, C. Freire, I. Castillero-Rosales, F. Artacho-Cordón and N. Olea, Concentrations of bisphenols, parabens, and benzophenones in human breast milk: A systematic review and meta-analysis, Sci. Total Environ., 2022, 806, 150437 CrossRef PubMed.
- H. H. Le, E. M. Carlson, J. P. Chua and S. M. Belcher, Bisphenol A is released from polycarbonate drinking bottles and mimics the neurotoxic actions of estrogen in developing cerebellar neurons, Toxicol. Lett., 2008, 176(2), 149–156 CrossRef CAS PubMed.
- A. Goodson, W. Summerfield and I. Cooper, Survey of bisphenol A and bisphenol F in canned foods, Food Addit. Contam., 2002, 19(8), 796–802 CrossRef CAS.
- H. C. Erythropel, M. Maric, J. A. Nicell, R. L. Leask and V. Yargeau, Leaching of the plasticizer di(2-ethylhexyl)phthalate (DEHP) from plastic containers and the question of human exposure, Appl. Microbiol. Biotechnol., 2014, 98(24), 9967–9981 CrossRef CAS.
- J. O. Tijani, O. O. Fatoba, O. O. Babajide and L. F. Petrik, Pharmaceuticals, endocrine disruptors, personal care products, nanomaterials and perfluorinated pollutants: a review, Environ. Chem. Lett., 2016, 14(1), 27–49 CrossRef CAS.
- N. Bumbudsanpharoke and S. Ko, Nano-Food Packaging: An Overview of Market, Migration Research, and Safety Regulations, J. Food Sci., 2015, 80(5), R910–R923 CrossRef CAS PubMed.
- G. K. Deshwal and N. R. Panjagari, Review on metal packaging: materials, forms, food applications, safety and recyclability, J. Food Sci. Technol., 2020, 57(7), 2377–2392 CrossRef PubMed.
- K. C. Ugoeze, E. O. Amogu, K. E. Oluigbo and N. Nwachukwu, Environmental and public health impacts of plastic wastes due to healthcare and food products packages: A Review, J. Environ. Sci. Publ. Health, 2021, 05(01), 1–31 Search PubMed.
- E. J. Hoekstra and C. Simoneau, Release of Bisphenol A from Polycarbonate-A Review, Crit. Rev. Food Sci. Nutr., 2013, 53(4), 386–402 CrossRef CAS PubMed.
- X. Liu, H. Shi, B. Xie, D. D. Dionysiou and Y. Zhao, Microplastics as Both a Sink and a Source of Bisphenol A in the Marine Environment, Environ. Sci. Technol., 2019, 53(17), 10188–10196 CrossRef CAS PubMed.
- K. Y. Foo and B. H. Hameed, An overview of landfill leachate treatment via activated carbon adsorption process, J. Hazard. Mater., 2009, 171(1–3), 54–60 CrossRef CAS PubMed.
- P. Rajasulochana and V. Preethy, Comparison on efficiency of various techniques in treatment of waste and sewage water – A comprehensive review, Resour.-Effic. Technol., 2016, 2(4), 175–184 Search PubMed.
- N. Morin, H. P. H. Arp and S. E. Hale, Bisphenol A in Solid Waste Materials, Leachate Water, and Air Particles from Norwegian Waste-Handling Facilities: Presence and Partitioning Behavior, Environ. Sci. Technol., 2015, 49(13), 7675–7683 CrossRef CAS PubMed.
- S. Almeida, A. Raposo, M. Almeida-González and C. Carrascosa, Bisphenol A: Food Exposure and Impact on Human Health, Compr. Rev. Food Sci. Food Saf., 2018, 17(6), 1503–1517 CrossRef PubMed.
- P. Cao, H.-n. Zhong, K. Qiu, D. Li, G. Wu and H.-x. Sui, et al., Exposure to bisphenol A and its substitutes, bisphenol F and bisphenol S from canned foods and beverages on Chinese market, Food Control, 2021, 120, 107502 CrossRef CAS.
- D. Y. Huang, H. Q. Zhao, C. P. Liu and C. X. Sun, Characteristics, sources, and transport of tetrabromobisphenol A and bisphenol A in soils from a typical e-waste recycling area in South China, Environ. Sci. Pollut. Res., 2014, 21(9), 5818–5826 CrossRef CAS.
- M. Deviot, I. Lachaise, C. Högg, J. Durner, F. X. Reichl and J. P. Attal, et al., Bisphenol A release from an orthodontic resin composite: A GC/MS and LC/MS study, Dent. Mater., 2018, 34(2), 341–354 CrossRef CAS.
- D. H. Tsai, F. W. Delrio, A. M. Keene, K. M. Tyner, R. I. MacCuspie and T. J. Cho, et al., Adsorption and conformation of serum albumin protein on gold nanoparticles investigated using dimensional measurements and in situ spectroscopic methods, Langmuir, 2011, 27(6), 2464–2477 CrossRef CAS PubMed.
- C. Liao and K. Kannan, Widespread occurrence of bisphenol A in paper and paper products: Implications for human exposure, Environmental Science and Technology, Environ. Sci. Technol., 2011, 45(21), 9372–9379 CrossRef CAS PubMed.
- T. Geens, L. Goeyens, K. Kannan, H. Neels and A. Covaci, Levels of bisphenol-A in thermal paper receipts from Belgium and estimation of human exposure, Sci. Total Environ., 2012, 435–436, 30–33 CrossRef CAS PubMed.
- B. E. Tokula, A. O. Dada, A. A. Inyinbor, K. S. Obayomi, O. S. Bello and U. Pal, Agro-waste based adsorbents as sustainable materials for effective adsorption of Bisphenol A from the environment: A review, J. Cleaner Prod., 2023, 388, 135819 CrossRef CAS.
- İ. İyigündoğdu, A. Üstündağ and Y. Duydu, Toxicological evaluation of bisphenol a and its analogues, Turk. J. Pharm. Sci., 2020, 17(4), 457–462 CrossRef.
- C. Pivonello, G. Muscogiuri, A. Nardone, F. Garifalos, D. P. Provvisiero and N. Verde, et al., Bisphenol A: An emerging threat to female fertility, Reprod. Biol. Endocrinol., 2020, 18(1), 4–11 CrossRef.
- C. Sonnenschein and A. M. Soto, An updated review of environmental estrogen and androgen mimics and antagonists, J. Steroid Biochem. Mol. Biol., 1998, 65(1–6), 143–150 CrossRef CAS PubMed.
- F. S. Vom Saal and L. N. Vandenberg, Update on the Health Effects of Bisphenol A: Overwhelming Evidence of Harm, Endocrinology, 2021, 162(3), 1–25 CrossRef CAS PubMed.
- F. S. Vom Saal and L. N. Vandenberg, Update on the Health Effects of Bisphenol A: Overwhelming Evidence of Harm, Endocrinology, 2021, 162(3), 1–25 CrossRef CAS.
- J. Liang, L. Pang, C. Yang, J. Long, Q. Liao and P. Tang, et al., Effects of prenatal single and mixed bisphenol exposure on bone mineral density in preschool children: A population-based prospective cohort study, Ecotoxicol. Environ. Saf., 2023, 267, 115665 CrossRef CAS.
- Y. Ma, H. Liu, J. Wu, L. Yuan, Y. Wang and X. Du, et al., The adverse health effects of bisphenol A and related toxicity mechanisms, Environ. Res., 2019, 108575 CrossRef CAS.
- M. Zhu, R. Zeng, D. Wu, Y. Li, T. Chen and A. Wang, Research progress of the effects of bisphenol analogues on the intestine and its underlying mechanisms: A review, Environ. Res., 2024, 243, 117891 CrossRef CAS PubMed.
- J. Xing, S. Zhang, M. Zhang and J. Hou, A critical review of presence, removal and potential impacts of endocrine disruptors bisphenol A, Comp. Biochem. Physiol., Part C: Toxicol. Pharmacol., 2022, 254, 109275 CAS.
- Y. Xu, J. Nie, C. Lu, C. Hu, Y. Chen and Y. Ma, et al., Effects and mechanisms of bisphenols exposure on neurodegenerative diseases risk: A systemic review, Sci. Total Environ., 2024, 919, 170670 CrossRef CAS.
- A. Tarafdar, R. Sirohi, P. A. Balakumaran, R. Reshmy, A. Madhavan and R. Sindhu, et al., The hazardous threat of Bisphenol A: Toxicity, detection and remediation, J. Hazard. Mater., 2022, 127097 CrossRef CAS PubMed.
- A. Kowalczyk, M. Wrzecińska, E. Czerniawska-Piątkowska, J. P. Araújo and P. Cwynar, Molecular consequences of the exposure to toxic substances for the endocrine system of females, Biomed. Pharmacother., 2022, 155, 113730 CrossRef CAS PubMed.
- A. Kortenkamp, O. Martin, S. Ermler, A. Baig and M. Scholze, Bisphenol A and declining semen quality: A systematic review to support the derivation of a reference dose for mixture risk assessments, Int. J. Hyg. Environ. Health, 2022, 241, 113942 CrossRef CAS.
- Y. Liu, Y. Yao, H. Li, F. Qiao, J. Wu and Z. Y. Du, et al., Influence of endogenous and exogenous estrogenic endocrine on intestinal microbiota in zebrafish, PLoS One, 2016, 11(10), 1–12 Search PubMed.
- I. Ďurovcová, S. Kyzek, J. Fabová, J. Makuková, E. Gálová and A. Ševčovičová, Genotoxic potential of bisphenol A: A review, Environ. Pollut., 2022, 306, 119346 CrossRef PubMed.
- O. Banerjee, S. Singh, I. Saha, S. Pal, M. Banerjee and S. Kundu, et al., Molecular dissection of cellular response of pancreatic islet cells to Bisphenol-A (BPA): A comprehensive review, Biochem. Pharmacol., 2022, 201, 115068 CrossRef CAS PubMed.
- M. Y. Xie, H. Ni, D. S. Zhao, L. Y. Wen, K. S. Li and H. H. Yang, et al., Exposure to bisphenol A and the development of asthma: A systematic review of cohort studies, Reprod. Toxicol., 2016, 65, 224–229 CrossRef CAS PubMed.
- I. Bahelka, R. Stupka, J. Čítek and M. Šprysl, The impact of bisphenols on reproductive system and on offspring in pigs – A review 2011–2020, Chemosphere, 2021, 263, 128203 CrossRef CAS PubMed.
- L. Y. Meng, W. F. Tao, J. Li, M. Zhu, D. B. Zhong and J. Zhou, et al., Effects of Bisphenol A and Its Substitute, Bisphenol F, on the Gut Microbiota in Mice, Biomed. Environ. Sci., 2024, 37(1), 19–30 CAS.
- A. Seyoum and A. Pradhan, Effect of phthalates on development, reproduction, fat metabolism and lifespan in Daphnia magna, Sci. Total Environ., 2019, 654, 969–977 CrossRef CAS PubMed.
- A. Seyoum and A. Pradhan, Effect of phthalates on development, reproduction, fat metabolism and lifespan in Daphnia magna, Sci. Total Environ., 2019, 654, 969–977 CrossRef CAS.
- R. Planelló, O. Herrero, J. L. Martínez-Guitarte and G. Morcillo, Comparative effects of butyl benzyl phthalate (BBP) and di(2-ethylhexyl) phthalate (DEHP) on the aquatic larvae of Chironomus riparius based on gene expression assays related to the endocrine system, the stress response and ribosomes, Aquat. Toxicol., 2011, 105(1–2), 62–70 CrossRef.
- R. Planelló, O. Herrero, J. L. Martínez-Guitarte and G. Morcillo, Comparative effects of butyl benzyl phthalate (BBP) and di(2-ethylhexyl) phthalate (DEHP) on the aquatic larvae of Chironomus riparius based on gene expression assays related to the endocrine system, the stress response and ribosomes, Aquat. Toxicol., 2011, 105(1–2), 62–70 CrossRef.
- B. Yurdakok-Dikmen, Y. Turgut, A. Gunal, R. Uyar, O. Kuzukıran and A. Filazi, et al., In vitro effects of selected endocrine disruptors (DEHP, PCB118, BPA) on narrow-clawed crayfish (Astacus leptodactylus) primary cells, In Vitro Cell. Dev. Biol.: Anim., 2020, 56(9), 783–791 CrossRef CAS PubMed.
- I. D. L. Gomes, I. Gazo, D. Nabi, L. Besnardeau, C. Hebras and A. McDougall, et al., Bisphenols disrupt differentiation of the pigmented cells during larval brain formation in the ascidian, Aquat. Toxicol., 2019, 216, 105314 CrossRef CAS.
- S. N. Volz, R. Poulsen, M. Hansen and H. Holbech, Bisphenol A alters retinal morphology, visually guided behavior, and thyroid hormone levels in zebrafish larvae, Chemosphere, 2024, 348, 140776 CrossRef CAS PubMed.
- M. A. Martín-Lara, M. Calero, A. Ronda, I. Iáñez-Rodríguez and C. Escudero, Adsorptive behavior of an activated carbon for bisphenol A removal in single and binary (bisphenol A-heavy metal) solutions, Water, 2020, 12(8), 2150 CrossRef.
- M. Seoane, Á. Cid, C. Herrero and M. Esperanza, Comparative acute toxicity of benzophenone derivatives and bisphenol analogues in the Asian clam Corbicula fluminea, Ecotoxicology, 2021, 30(1), 142–153 CrossRef CAS PubMed.
- M. Faheem and R. K. Bhandari, Detrimental Effects of Bisphenol Compounds on Physiology and Reproduction in Fish: A Literature Review, Environ. Toxicol. Pharmacol., 2021, 81, 103497 CrossRef CAS.
- C. Xiao, L. Wang, Q. Zhou and X. Huang, Hazards of bisphenol A (BPA) exposure: A systematic review of plant toxicology studies, J. Hazard. Mater., 2020, 384, 121488 CrossRef CAS.
- W. Czarnocka and S. Karpiński, Friend or foe? Reactive oxygen species production, scavenging and signaling in plant response to environmental stresses, Free Radic. Biol. Med., 2018, 122, 4–20 CrossRef CAS.
- İ. İyigündoğdu, A. Üstündağ and Y. Duydu, Toxicological evaluation of bisphenol a and its analogues, Turk. J. Pharm. Sci., 2020, 17(4), 457–462 CrossRef.
- T. T. Schug and L. S. Birnbaum, Human Health Effects of Bisphenol A, Molecular and Integrative Toxicology, 2014, pp. 1–29 Search PubMed.
- L. S. Birnbaum, J. R. Bucher, G. W. Collman, D. C. Zeldin, A. F. Johnson and T. T. Schug, et al., Consortium-based science: The NIEHS's multipronged, collaborative approach to assessing the health effects of Bisphenol A, Environ. Health Perspect., 2012, 120(12), 1640–1644 CrossRef CAS PubMed.
- J. W. Brock, Y. Yoshimura, J. R. Barr, V. L. Maggio, S. R. Graiser, H. Nakazawa and L. L. Needham, Measurement of bisphenol A levels in human urine, J. Exposure Sci. Environ. Epidemiol., 2001, 11(4), 323–328 CrossRef CAS PubMed.
- C. Zeng, W. Sweet and Q. Cheng, Ecological Citizenship and Green Burial in China, J. Agric. Environ. Ethics, 2016, 29(6), 985–1001 CrossRef.
- B. Petrie, L. Lopardo, K. Proctor, J. Youdan, R. Barden and B. Kasprzyk-Hordern, Assessment of bisphenol-A in the urban water cycle, Sci. Total Environ., 2019, 900–907 CrossRef CAS.
- K. Ozhan and E. Kocaman, Temporal and Spatial Distributions of Bisphenol A in Marine and Freshwaters in Turkey, Arch. Environ. Contam. Toxicol., 2019, 76(2), 246–254 CrossRef CAS PubMed.
- L. G. A. Barboza, S. C. Cunha, C. Monteiro, J. O. Fernandes and L. Guilhermino, Bisphenol A and its analogs in muscle and liver of fish from the North East Atlantic Ocean in relation to microplastic contamination. Exposure and risk to human consumers, J. Hazard. Mater., 2020, 393, 122419 CrossRef CAS.
- X. Liu, H. Shi, B. Xie, D. D. Dionysiou and Y. Zhao, Microplastics as Both a Sink and a Source of Bisphenol A in the Marine Environment, Environ. Sci. Technol., 2019, 53(17), 10188–10196 CrossRef CAS.
- A. B. Javurek, W. G. Spollen, S. A. Johnson, N. J. Bivens, K. H. Bromert and S. A. Givan, et al., Effects of exposure to bisphenol A and ethinyl estradiol on the gut microbiota of parents and their offspring in a rodent model, Gut Microb., 2016, 7(6), 471–485 CrossRef CAS.
- L. Chen, Y. Guo, C. Hu, P. K. S. Lam, J. C. W. Lam and B. Zhou, Dysbiosis of gut microbiota by chronic coexposure to titanium dioxide nanoparticles and bisphenol A: Implications for host health in zebrafish, Environ. Pollut., 2018, 234, 307–317 CrossRef CAS PubMed.
- T. R. Catron, S. P. Keely, N. E. Brinkman, T. J. Zurlinden, C. E. Wood and J. R. Wright, et al., Host Developmental Toxicity of BPA and BPA Alternatives Is Inversely Related to Microbiota Disruption in Zebrafish, Toxicol. Sci., 2019, 167(2), 468–483 CrossRef CAS PubMed.
- T. R. Catron, S. P. Keely, N. E. Brinkman, T. J. Zurlinden, C. E. Wood and J. R. Wright, et al., Host Developmental Toxicity of BPA and BPA Alternatives Is Inversely Related to Microbiota Disruption in Zebrafish, Toxicol. Sci., 2019, 167(2), 468–483 CrossRef CAS.
- Y. Liu, Y. Yao, H. Li, F. Qiao, J. Wu and Z. Y. Du, et al., Influence of endogenous and exogenous estrogenic endocrine on intestinal microbiota in zebrafish, PLoS One, 2016, 11(10), 1–12 Search PubMed.
- K. P. Lai, Y. T. Chung, R. Li, H. T. Wan and C. K. C. Wong, Bisphenol A alters gut microbiome: Comparative metagenomics analysis, Environ. Pollut., 2016, 218, 923–930 CrossRef CAS PubMed.
- A. B. Javurek, W. G. Spollen, S. A. Johnson, N. J. Bivens, K. H. Bromert and S. A. Givan, et al., Effects of exposure to bisphenol A and ethinyl estradiol on the gut microbiota of parents and their offspring in a rodent model, Gut Microb., 2016, 7(6), 471–485 CrossRef CAS.
- Y. M. Lee, M. J. Seong, J. W. Lee, Y. K. Lee, T. M. Kim and S. Y. Nam, et al., Estrogen receptor independent neurotoxic mechanism of bisphenol A, an environmental estrogen, J. Vet. Sci., 2007, 8(1), 27–38 CrossRef PubMed.
- T. Wang, J. Han, X. Duan, B. Xiong, X. S. Cui and N. H. Kim, et al., The toxic effects and possible mechanisms of bisphenol A on oocyte maturation of porcine in vitro, Oncotarget, 2016, 7(22), 32554–32565 CrossRef.
- J. Guo, M. H. Zhao, K. T. Shin, Y. J. Niu, Y. D. Ahn and N. H. Kim, et al., The possible molecular mechanisms of bisphenol A action on porcine early embryonic development, Sci. Rep., 2017, 7(1), 1–9 CrossRef.
- I. Cimmino, F. Fiory, G. Perruolo, C. Miele, F. Beguinot and P. Formisano, et al., Potential mechanisms of bisphenol A (BPA), Int. J. Mol. Sci., 2020, 21(16), 1–22 Search PubMed.
- K. Baralić, K. Živančević, D. Jorgovanović, D. Javorac, J. Radovanović and T. Gojković, et al., Probiotic reduced the impact of phthalates and bisphenol A mixture on type 2 diabetes mellitus development: Merging bioinformatics with in vivo analysis, Food Chem. Toxicol., 2021, 154, 112325 CrossRef.
- S. Rahmani, N. Pour Khalili, F. Khan, S. Hassani, E. Ghafour-Boroujerdi and M. Abdollahi, Bisphenol A: What lies beneath its induced diabetes and the epigenetic modulation?, Life Sci., 2018, 214, 136–144 CrossRef CAS PubMed.
- S. Siddique, G. Zhang and C. Kubwabo, Exposure to bisphenol a and risk of developing type 2 diabetes: A mini review, Emerging Contam., 2020, 6, 274–282 CrossRef.
- C. Xiong, L. Xu, X. Dong, Z. Cao, Y. Wang and K. Chen, et al., Trimester-specific associations of maternal exposure to bisphenols with neonatal thyroid stimulating hormone levels: A birth cohort study, Sci. Total Environ., 2023, 880, 163354 CrossRef CAS PubMed.
- R. Meli, A. Monnolo, C. Annunziata, C. Pirozzi and M. C. Ferrante, Oxidative stress and BPA toxicity: An antioxidant approach for male and female reproductive dysfunction, Antioxidants, 2020, 9(5), 405 CrossRef CAS PubMed.
- S. Vigneshwaran, J. Preethi and S. Meenakshi, Interface engineering of ultrathin multi-functional 2D draped chitosan for efficient charge separation on degradation of paraquat – A mechanistic study, J. Environ. Chem. Eng., 2020, 8(5), 104446 CrossRef CAS.
- V. Sharma, D. Jain, A. R. Rai, P. Kumari, V. Nagar and A. Kaur, et al., Toxicological assessment and concentration analysis of Bisphenol A in food grade plastics: A systematic review, Mater. Today: Proc., 2023, 95, 18–25 CAS.
- J. Q. Chen, T. R. Brown and J. Russo, Regulation of energy metabolism pathways by estrogens and estrogenic chemicals and potential implications in obesity associated with increased exposure to endocrine disruptors, Biochim. Biophys. Acta, Mol. Cell Res., 2009, 1793(7), 1128–1143 CrossRef CAS.
- N. D. Mandel, B. Gamboa-Loira, M. E. Cebrián, Á. Mérida-Ortega and L. López-Carrillo, Challenges to regulate products containing bisphenol A: Implications for policy, Salud Publica Mex., 2019, 61(5), 692–697 CrossRef.
- W. T. Tsai, Human health risk on environmental exposure to bisphenol-A: A review, J. Environ. Sci. Health C Environ. Carcinog. Ecotoxicol. Rev., 2006, 24(2), 225–255 CrossRef CAS PubMed.
- D. Amiridou and D. Voutsa, Alkylphenols and phthalates in bottled waters, J. Hazard. Mater., 2011, 185(1), 281–286 CrossRef CAS PubMed.
- T. Geens, T. Z. Apelbaum, L. Goeyens, H. Neels and A. Covaci, Intake of bisphenol A from canned beverages and foods on the Belgian market, Food Addit. Contam.: Part A, 2010, 27(11), 1627–1637 CrossRef CAS.
- S. Maggioni, P. Balaguer, C. Chiozzotto and E. Benfenati, Screening of endocrine-disrupting phenols, herbicides, steroid estrogens, and estrogenicity in drinking water from the waterworks of 35 Italian cities and from PET-bottled mineral water, Environ. Sci. Pollut. Res., 2013, 20(3), 1649–1660 CrossRef CAS PubMed.
- E. Fasano, F. Esposito, G. Scognamiglio, F. Di Francesco, P. Montuori and C. R. Amodio, et al., Bisphenol A contamination in soft drinks as a risk for children's health in Italy, Food Addit. Contam.: Part A, 2015, 32(7), 1207–1214 CrossRef CAS.
- M. N. Tzatzarakis, V. Karzi, E. Vakonaki, M. Goumenou, M. Kavvalakis and P. Stivaktakis, et al., Bisphenol A in soft drinks and canned foods and data evaluation, Food Addit. Contam., Part B, 2017, 10(2), 85–90 CrossRef CAS PubMed.
- N. Casajuana and S. Lacorte, New methodology for the determination of phthalate esters, bisphenol A, bisphenol A diglycidyl ether, and nonylphenol in commercial whole milk samples, J. Agric. Food Chem., 2004, 52(12), 3702–3707 CrossRef CAS.
- L. Grumetto, O. Gennari, D. Montesano, R. Ferracane, A. Ritieni and S. Albrizio, et al., Determination of five bisphenols in commercial milk samples by liquid chromatography coupled to fluorescence detection, J. Food Prot., 2013, 76(9), 1590–1596 CrossRef CAS PubMed.
- A. K. Sakhi, I. T. L. Lillegaard, S. Voorspoels, M. H. Carlsen, E. B. Løken and A. L. Brantsæter, et al., Concentrations of phthalates
and bisphenol A in Norwegian foods and beverages and estimated dietary exposure in adults, Environ. Int., 2014, 73, 259–269 CrossRef CAS PubMed.
- T. Cirillo, G. Latini, M. A. Castaldi, L. Dipaola, E. Fasano and F. Esposito, et al., Exposure to di-2-ethylhexyl phthalate, di-n-butyl phthalate and bisphenol A through infant formulas, J. Agric. Food Chem., 2015, 63(12), 3303–3310 CrossRef CAS PubMed.
- Z. Brenn-Struckhofova and M. Cichna-Markl, Determination of bisphenol A in wine by sol-gel immunoaffinity chromatography, HPLC and fluorescence detection, Food Addit. Contam., 2006, 23(11), 1227–1235 CrossRef CAS.
- J. M. Braun, A. E. Kalkbrenner, A. M. Calafat, J. T. Bernert, X. Ye and M. J. Silva, et al., Variability and predictors of urinary bisphenol a concentrations during pregnancy, Environ. Health Perspect., 2011, 119(1), 131–137 CrossRef CAS PubMed.
- L. Grumetto, D. Montesano, S. Seccia, S. Albrizio and F. Barbato, Determination of bisphenol A and bisphenol B residues in canned peeled tomatoes by reversed-phase liquid chromatography, J. Agric. Food Chem., 2008, 56(22), 10633–10637 CrossRef CAS.
- P. Viñas, N. Campillo, N. Martínez-Castillo and M. Hernández-Córdoba, Comparison of two derivatization-based methods for solid-phase microextraction-gas chromatography-mass spectrometric determination of bisphenol A, bisphenol S and biphenol migrated from food cans, Anal. Bioanal. Chem., 2010, 397(1), 115–125 CrossRef PubMed.
- A. Alabi, N. Caballero-Casero and S. Rubio, Quick and simple sample treatment for multiresidue analysis of bisphenols, bisphenol diglycidyl ethers and their derivatives in canned food prior to liquid chromatography and fluorescence detection, J. Chromatogr. A, 2014, 1336, 23–33 CrossRef CAS PubMed.
- S. Errico, M. Bianco, L. Mita, M. Migliaccio, S. Rossi and C. Nicolucci, et al., Migration of bisphenol A into canned tomatoes produced in Italy: Dependence on temperature and storage conditions, Food Chem., 2014, 160, 157–164 CrossRef CAS PubMed.
- I. Gyllenhammar, A. Glynn, P. O. Darnerud, S. Lignell, R. van Delft and M. Aune, 4-Nonylphenol and bisphenol A in Swedish food and exposure in Swedish nursing women, Environ. Int., 2012, 43(1), 21–28 CrossRef CAS PubMed.
- S. Gorecki, N. Bemrah, A. C. Roudot, E. Marchioni, B. Le Bizec and F. Faivre, et al., Human health risks related to the consumption of foodstuffs of animal origin contaminated by bisphenol A, Food Chem. Toxicol., 2017, 110, 333–339 CrossRef CAS.
- N. Bemrah, J. Jean, G. Rivière, M. Sanaa, S. Leconte and M. Bachelot, et al., Assessment of dietary exposure to bisphenol A in the French population with a special focus on risk characterisation for pregnant French women, Food Chem. Toxicol., 2014, 72, 90–97 CrossRef CAS PubMed.
- V. Lo Turco, G. Di Bella, A. G. Potortì, A. Tropea, E. K. Casale and M. R. Fede, et al., Determination of plasticisers and BPA in Sicilian and Calabrian nectar honeys by selected ion monitoring GC/MS, Food Addit. Contam.: Part A, 2016, 33(11), 1693–1699 CrossRef CAS PubMed.
- O. Zoller, B. J. Brüschweiler, R. Magnin, H. Reinhard, P. Rhyn and H. Rupp, et al., Natural occurrence of bisphenol F in mustard, Food Addit. Contam.: Part A, 2015, 33(1), 137–146 Search PubMed.
- C. Bach, X. Dauchy, M. C. Chagnon and S. Etienne, Chemical compounds and toxicological assessments of drinking water stored in polyethylene terephthalate (PET) bottles: A source of controversy reviewed, Water Res., 2012, 46(3), 571–583 CrossRef CAS.
- E. Ferrer, E. Santoni, S. Vittori, G. Font, J. Mañes and G. Sagratini, Simultaneous determination of bisphenol A, octylphenol, and nonylphenol by pressurised liquid extraction and liquid chromatography-tandem mass spectrometry in powdered milk and infant formulas, Food Chem., 2011, 126(1), 360–367 CrossRef CAS.
- S. C. Cunha, R. N. Alves, J. O. Fernandes, S. Casal and A. Marques, First approach to assess the bioaccessibility of bisphenol A in canned seafood, Food Chem., 2017, 232, 501–507 CrossRef CAS PubMed.
- M. Sasaki, J. I. Maki, K. I. Oshiman, Y. Matsumura and T. Tsuchido, Biodegradation of bisphenol A by cells and cell lysate from Sphingomonas sp. strain AO1, Biodegradation, 2005, 16(5), 449–459 CrossRef CAS PubMed.
- M. Kamaraj, R. Sivaraj and R. Venckatesh, Biodegradation of Bisphenol A by the tolerant bacterial species isolated from coastal regions of Chennai, Tamil Nadu, India, Int. Biodeterior. Biodegrad., 2014, 93, 216–222 CrossRef CAS.
- M. Kamaraj, R. Sivaraj and R. Venckatesh, Biodegradation of Bisphenol A by the tolerant bacterial species isolated from coastal regions of Chennai, Tamil Nadu, India, Int. Biodeterior. Biodegrad., 2014, 93, 216–222 CrossRef CAS.
- O. Gulnaz and S. Dincer, Biodegradation of Bisphenol a by Chlorella vulgaris and Aeromonas Hydrophilia, J. Appl. Biol. Sci., 2009, 3(2), 79–84 Search PubMed.
- O. Gulnaz and S. Dincer, Biodegradation of Bisphenol a by Chlorella vulgaris and Aeromonas Hydrophilia, J. Appl. Biol. Sci., 2009, 3(2), 79–84 Search PubMed.
- M. Sasaki, J. I. Maki, K. I. Oshiman, Y. Matsumura and T. Tsuchido, Biodegradation of bisphenol A by cells and cell lysate from Sphingomonas sp. strain AO1, Biodegradation, 2005, 16(5), 449–459 CrossRef CAS PubMed.
- R. Das, G. Li, B. Mai and T. An, Spore cells from BPA degrading bacteria Bacillus sp. GZB displaying high laccase activity and stability for BPA degradation, Sci. Total Environ., 2018, 640–641, 798–806 CrossRef CAS PubMed.
- H. Yamanaka, K. Moriyoshi, T. Ohmoto, T. Ohe and K. Sakai, Degradation of bisphenol a by Bacillus pumilus isolated from kimchi, a traditionally fermented food, Appl. Biochem. Biotechnol., 2007, 136(1), 39–51 CrossRef CAS PubMed.
- Y. Jia, A. Eltoukhy, J. Wang, X. Li, T. S. Hlaing and M. M. Aung, et al., Biodegradation of bisphenol a by sphingobium sp. YC-JY1 and the essential role of cytochrome P450 Monooxygenase, Int. J. Mol. Sci., 2020, 21(10), 3588 CrossRef CAS.
- Y. Yang, X. G. Chen, L. Wang, Z. H. Chu, Z. Liu and Y. C. Dong, et al., Sliding wear behavior of in-situ FeAl2O4 matrix nanocomposite coating fabricated by plasma spraying, Tribol. Int., 2015, 81, 97–104 CrossRef CAS.
- M. Takeo, J. Akizuki, A. Kawasaki and S. Negoro, Degradation potential of the nonylphenol monooxygenase of Sphingomonas sp. NP5 for bisphenols and their structural analogs, Microorganisms, 2020, 8(2), 284 CrossRef CAS.
- A. A. Telke, D. C. Kalyani, U. U. Jadhav, G. K. Parshetti and S. P. Govindwar, Purification and characterization of an extracellular laccase from a Pseudomonas sp. LBC1 and its application for the removal of bisphenol A, J. Mol. Catal. B Enzym., 2009, 61(3–4), 252–260 CrossRef CAS.
- E. J. Eio, M. Kawai, K. Tsuchiya, S. Yamamoto and T. Toda, Biodegradation of bisphenol A by bacterial consortia, Int. Biodeterior. Biodegrad., 2014, 96, 166–173 CrossRef.
- N. A. Zhou, H. Kjeldal, H. L. Gough and J. L. Nielsen, Identification of Putative Genes Involved in Bisphenol A Degradation Using Differential Protein Abundance Analysis of Sphingobium sp. BiD32, Environ. Sci. Technol., 2015, 49(20), 12231–12241 CrossRef PubMed.
- W. Yue, C. F. Yin, L. Sun, J. Zhang, Y. Xu and N. Y. Zhou, Biodegradation of bisphenol-A polycarbonate plastic by Pseudoxanthomonas sp. strain NyZ600, J. Hazard. Mater., 2021, 416, 125775 CrossRef CAS PubMed.
- S. Oh and D. Choi, Microbial Community Enhances Biodegradation of Bisphenol A Through Selection of Sphingomonadaceae, Microb. Ecol., 2019, 77(3), 631–639 CrossRef CAS PubMed.
- A. López-Moreno, A. Torres-Sánchez, I. Acuña, A. Suárez and M. Aguilera, Representative Bacillus sp. AM1 from Gut Microbiota Harbor Versatile
Molecular Pathways for Bisphenol A Biodegradation, Int. J. Mol. Sci., 2021, 22(9), 4952 CrossRef PubMed.
- B. Suyamud, P. Thiravetyan, G. M. Gadd, B. Panyapinyopol and D. Inthorn, Bisphenol A removal from a plastic industry wastewater by Dracaena sanderiana endophytic bacteria and Bacillus cereus NI, Int. J. Phytoremediation, 2020, 22(2), 167–175 CrossRef CAS PubMed.
- D. He, Y. Zeng and G. Zhou, The influence of microplastics on the toxic effects and biodegradation of bisphenol A in the microalgae Chlorella pyrenoidosa, Aquat. Ecol., 2022, 56(4), 1287–1296 CrossRef CAS.
- N. A. Zhou, H. Kjeldal, H. L. Gough and J. L. Nielsen, Identification of Putative Genes Involved in Bisphenol A Degradation Using Differential Protein Abundance Analysis of Sphingobium sp. BiD32, Environ. Sci. Technol., 2015, 49(20), 12231–12241 CrossRef.
- R. Hu, H. Zhao, X. Xu, Z. Wang, K. Yu and L. Shu, et al., Bacteria-driven phthalic acid ester biodegradation: Current status and emerging opportunities, Environ. Int., 2021, 154, 106560 CrossRef CAS.
- L. Ren, Y. Jia, R. Zhang, Z. Lin, Z. Zhen and H. Hu, et al., Insight into metabolic versatility of an aromatic compounds-degrading arthrobactersp. YC-RL1, Front. Microbiol., 2018, 9, 1–15 CrossRef PubMed.
- A. Ismanto, T. Hadibarata, R. A. Kristanti, L. Maslukah, N. Safinatunnajah and W. Kusumastuti, Endocrine disrupting chemicals (EDCs) in environmental matrices: Occurrence, fate, health impact, physio-chemical and bioremediation technology, Environ. Pollut., 2022, 302, 119061 CrossRef CAS PubMed.
- W. Dong, Q. Chen, Y. Hou, S. Li, K. Zhuang and F. Huang, et al., Metabolic pathway involved in 2- methyl-6-ethylaniline degradation by Sphingobium sp. strain MEA3-1 and cloning of the novel flavin-dependent monooxygenase system meaBA, Appl. Environ. Microbiol., 2015, 81(24), 8254–8264 CrossRef CAS PubMed.
- C. Filote, M. Roşca, R. M. Hlihor, P. Cozma, I. M. Simion and M. Apostol, et al., Sustainable application of biosorption and bioaccumulation of persistent pollutants in wastewater treatment: Current practice, Processes, 2021, 9(10), 1–38 CrossRef.
- V. D'Argenio, G. Casaburi, V. Precone and F. Salvatore, Comparative metagenomic analysis of human gut microbiome composition using two different bioinformatic pipelines, BioMed Res. Int., 2014, 2014, 325340 Search PubMed.
- D. P. Mohapatra, S. K. Brar, R. D. Tyagi and R. Y. Surampalli, Physico-chemical pre-treatment and biotransformation of wastewater and wastewater Sludge - Fate of bisphenol A, Chemosphere, 2010, 78(8), 923–941 CrossRef CAS.
- W. Dong, Q. Chen, Y. Hou, S. Li, K. Zhuang and F. Huang, et al., Metabolic pathway involved in 2- methyl-6-ethylaniline degradation by Sphingobium sp. strain MEA3-1 and cloning of the novel flavin-dependent monooxygenase system meaBA, Appl. Environ. Microbiol., 2015, 81(24), 8254–8264 CrossRef CAS.
- V. D'Argenio, G. Casaburi, V. Precone and F. Salvatore, Comparative metagenomic analysis of human gut microbiome composition using two different bioinformatic pipelines, BioMed Res. Int., 2014, 2014, 325340 Search PubMed.
- L. G. C. Villegas, N. Mashhadi, M. Chen, D. Mukherjee, K. E. Taylor and N. Biswas, A Short Review of Techniques for Phenol Removal from Wastewater, Curr. Pollut. Rep., 2016, 2(3), 157–167 CrossRef CAS.
- U. Kües, Fungal enzymes for environmental management, Curr. Opin. Biotechnol., 2015, 33, 268–278 CrossRef.
- Y. Jia, A. Eltoukhy, J. Wang, X. Li, T. S. Hlaing and M. M. Aung, et al., Biodegradation of bisphenol a by sphingobium sp. YC-JY1 and the essential role of cytochrome P450 Monooxygenase, Int. J. Mol. Sci., 2020, 21(10), 3588 CrossRef CAS PubMed.
- J. Bhagat, L. Zang, N. Nishimura and Y. Shimada, Zebrafish:
An emerging model to study microplastic and nanoplastic toxicity, Sci. Total Environ., 2020, 728, 138707 CrossRef CAS PubMed.
- S. G. Nkhata, E. Ayua, E. H. Kamau and J. B. Shingiro, Fermentation and germination improve nutritional value of cereals and legumes through activation of endogenous enzymes, Food Sci. Nutr., 2018, 6(8), 2446–2458 CrossRef CAS.
- R. V. Moharir and S. Kumar, Challenges associated with plastic waste disposal and allied microbial routes for its effective degradation: A comprehensive review, J. Cleaner Prod., 2019, 208, 65–76 CrossRef CAS.
- S. Oh and D. Choi, Microbial Community Enhances Biodegradation of Bisphenol A Through Selection of Sphingomonadaceae, Microb. Ecol., 2019, 77(3), 631–639 CrossRef CAS PubMed.
- R. Villemur, S. Cristina, J. Ouellette, P. Juteau, F. Lépine and E. Déziel, et al., Biodegradation of endocrine disruptors in solid-liquid two-phase partitioning systems by enrichment cultures, Appl. Environ. Microbiol., 2013, 79(15), 4701–4711 CrossRef CAS PubMed.
- K. V. S. Rajmohan, C. Ramya, M. Raja Viswanathan and S. Varjani, Plastic pollutants: effective waste management for pollution control and abatement, Curr. Opin. Environ. Sci. Health., 2019, 12, 72–84 CrossRef.
- P. A. Opoku, H. Jingyu, L. Yi, D. Ewusi-Mensah and N. Miwornunyuie, Scalability of the multi-anode plug flow microbial fuel cell as a sustainable prospect for large-scale design, Renew. Energy, 2023, 207(1), 693–702 CrossRef CAS.
- M. Zielińska, I. Wojnowska-Baryła and A. Cydzik-Kwiatkowska, Bisphenol A Removal from Water and Wastewater, Springer, 2019 Search PubMed.
- Y. Zhao, C. W. Cho, L. Cui, W. Wei, J. Cai and G. Wu, et al., Adsorptive removal of endocrine-disrupting compounds and a pharmaceutical using activated charcoal from aqueous solution: kinetics, equilibrium, and mechanism studies, Environ. Sci. Pollut. Res., 2019, 26, 33897–33905 CrossRef CAS PubMed.
- M. S. Muhamad, M. R. Salim, W. J. Lau, Z. Yusop and T. Hadibarata, The removal of bisphenol A in water treatment plant using ultrafiltration membrane system, Water, Air, Soil Pollut., 2016, 227, 1–12 CrossRef CAS.
- C. E. Almeida-Naranjo, V. H. Guerrero and C. A. Villamar-Ayala, Emerging contaminants and their removal from aqueous media using conventional/non-conventional adsorbents: a glance at the relationship between materials, processes, and technologies, Water, 2023, 15(8), 1626 CrossRef CAS.
- B. S. Rathi and P. S. Kumar, Application of adsorption process for effective removal of emerging contaminants from water and wastewater, Environ. Pollut., 2021, 280, 116995 CrossRef CAS PubMed.
- Z. Fang, Y. Hu, J. Cheng and Y. Chen, Continuous removal of trace bisphenol A from water by high efficacy TiO2 nanotube pillared graphene-based macrostructures in a photocatalytically fluidized bed, Chem. Eng. J., 2019, 372, 581–589 CrossRef CAS.
- S. Zinadini, A. A. Zinatizadeh, M. Rahimi, V. Vatanpour and K. Bahrami, Energy recovery and hygienic water production from wastewater using an innovative integrated microbial fuel cell–membrane separation process, Energy, 2017, 141, 1350–1362 CrossRef CAS.
- M. S. Muhamad, M. R. Salim, W. J. Lau, Z. Yusop and T. Hadibarata, The removal of bisphenol A in water treatment plant using ultrafiltration membrane system, Water, Air, Soil Pollut., 2016, 227, 1–12 CrossRef CAS.
- Y. S. Khoo, P. S. Goh, W. J. Lau, A. F. Ismail, M. S. Abdullah and N. H. M. Ghazali, et al., Removal of emerging organic micropollutants via modified-reverse osmosis/nanofiltration membranes: A review, Chemosphere, 2022, 305, 135151 CrossRef CAS.
- N. A. Khan, S. Singh, E. A. López-Maldonado, N. Pavithra, P. F. Méndez-Herrera and J. R. López-López, et al., Emerging membrane technology and hybrid treatment systems for the removal of micropollutants from wastewater, Desalination, 2023, 565, 116873 CrossRef CAS.
- M. S. Muhamad, M. R. Salim, W. J. Lau and Z. Yusop, A review on bisphenol
A occurrences, health effects and treatment process via membrane technology for drinking water, Environ. Sci. Pollut. Res., 2016, 23, 11549–11567 CrossRef CAS PubMed.
- C. Charcosset, Ultrafiltration, microfiltration, nanofiltration and reverse osmosis in integrated membrane processes, Integr. Membr. Syst. Processes, 2016, 1–22 CAS.
- Y. S. Khoo, P. S. Goh, W. J. Lau, A. F. Ismail, M. S. Abdullah and N. H. M. Ghazali, et al., Removal of emerging organic micropollutants via modified-reverse osmosis/nanofiltration membranes: A review, Chemosphere, 2022, 305, 135151 CrossRef CAS.
- L. L. S. Silva, W. Abdelraheem, M. N. Nadagouda, A. R. Maria, D. D. Dionysiou and F. V. Fonseca, et al., Novel microwave-driven synthesis of hydrophilic polyvinylidene fluoride/polyacrylic acid (PVDF/PAA) membranes and decoration with nano zero-valent-iron (nZVI) for water treatment applications, J. Membr. Sci., 2020, 116544 Search PubMed.
- H. Wu, X. Niu, J. Yang, C. Wang and M. Lu, Retentions of bisphenol A and norfloxacin by three different ultrafiltration membranes in regard to drinking water treatment, Chem. Eng. J., 2016, 410–416 CrossRef CAS.
- R. Valladares Linares, V. Yangali-Quintanilla, Z. Li and G. Amy, Rejection of micropollutants by clean and fouled forward osmosis membrane, Water Res., 2011, 6737–6744 CrossRef.
- C. G. Moreira, M. H. Moreira, V. M. O. C. Silva, H. G. Santos, D. M. Bila and F. V. Fonseca, Treatment of Bisphenol A (BPA) in water using UV/H2O2 and reverse osmosis (RO) membranes: assessment of estrogenic activity and membrane adsorption, Water Sci. Technol., 2019, 80(11), 2169–2178 CrossRef CAS.
- C. G. Moreira, L. C. de Souza, T. C. Castor Neto, G. Gomes, D. M. Bila and F. V. Fonseca, Combined reverse osmosis and UV/H2O2 treatment of aqueous solutions of bisphenol A and 17α-ethinylestradiol: assessment of estrogenic activity, Environ. Technol., 2023, 44(20), 3108–3120 CrossRef CAS PubMed.
- Y. Hu, Q. Zhu, X. Yan, C. Liao and G. Jiang, Occurrence, fate and risk assessment of BPA and its substituents in wastewater treatment plant: A review, Environ. Res., 2019, 178, 108732 CrossRef CAS PubMed.
- X. Li, Y. Xu, K. Goh, T. H. Chong and R. Wang, Layer-by-layer assembly based low pressure biocatalytic nanofiltration membranes for micropollutants removal, J. Membr. Sci., 2020, 118514 CrossRef CAS.
- P. Wang, F. Wang, H. Jiang, Y. Zhang, M. Zhao and R. Xiong, et al., Strong improvement of nanofiltration performance on micropollutant removal and reduction of membrane fouling by hydrolyzed-aluminum nanoparticles, Water Res., 2020, 115649 CrossRef CAS PubMed.
- C. Li, G. Feng, Z. Pan, C. Song, X. Fan and P. Tao, et al., High-performance electrocatalytic microfiltration CuO/Carbon membrane by facile dynamic electrodeposition for small-sized organic pollutants removal, J. Membr. Sci., 2020, 601, 117913 CrossRef CAS.
- P. T. P. Aryanti, A. N. Hakim, S. Widodo, I. N. Widiasa and I. G. Wenten, Prospect and challenges of tight ultrafiltration membrane in drinking water treatment, in IOP Conference Series: Materials Science and Engineering, IOP Publishing, 2018, p. 12012 Search PubMed.
- M. Zielińska, A. Cydzik-Kwiatkowska, K. Bułkowska, K. Bernat and I. Wojnowska-Baryła, Treatment of Bisphenol A-Containing Effluents from Aerobic Granular Sludge Reactors with the Use of Microfiltration and Ultrafiltration Ceramic Membranes, Water, Air, Soil Pollut., 2017, 228(8), 282 CrossRef.
- W. Tian, J. Qiu, N. Li, D. Chen, Q. Xu and H. Li, et al., Efficient piezocatalytic removal of BPA and Cr (VI) with SnS2/CNFs membrane by harvesting vibration energy, Nano Energy, 2021, 86, 106036 CrossRef CAS.
- M. S. Muhamad, N. Hamidon, M. R. Salim, Z. Yusop, W. J. Lau and T. Hadibarata, Response surface methodology for modeling bisphenol a removal using ultrafiltration membrane system, Water, Air, Soil Pollut., 2018, 229, 1–11 CrossRef CAS.
- M. Qasim, M. Badrelzaman, N. N. Darwish, N. A. Darwish and N. Hilal, Reverse osmosis desalination: A state-of-the-art review, Desalination, 2019, 459, 59–104 CrossRef CAS.
- T. D. Aiyelabegan, S. N. A. Shafie, S. M. Hizam and N. A. H. Nordin, The removal of bisphenol-A from synthetic wastewater using thin-film composite membrane, Malaysian J. Anal. Sci., 2022, 26(3), 507–519 Search PubMed.
- F. Widhiastuti, L. Fan, J. Paz-Ferreiro and K. Chiang, Oxidative treatment of bisphenol A in municipal wastewater reverse osmosis concentrate using Ferrate (VI), J. Environ. Chem. Eng., 2021, 9(4), 105462 CrossRef CAS.
- F. Cheng and J. Wang, Removal of bisphenol a from wastewater by adsorption and membrane separation: Performances and mechanisms, Chem. Eng. J., 2024, 149414 CrossRef CAS.
- P. Pourhakkak, M. Taghizadeh, A. Taghizadeh, M. Ghaedi, Adsorbent, in Interface Science and Technology, Elsevier, 2021, pp. 71–210 Search PubMed.
- A. E. D. Mahmoud, Graphene-based nanomaterials for the removal of organic pollutants: Insights into linear versus nonlinear mathematical models, J. Environ. Manage., 2020, 270, 110911 CrossRef.
- A. B. Hernández-Abreu, S. Álvarez-Torrellas, V. I. Águeda, M. Larriba, J. A. Delgado and P. A. Calvo, et al., Enhanced removal of the endocrine disruptor compound Bisphenol A by adsorption onto green-carbon materials. Effect of real effluents on the adsorption process, J. Environ. Manage., 2020, 110604 CrossRef.
- C. E. Choong, S. Ibrahim and W. J. Basirun, Mesoporous silica from batik sludge impregnated with aluminum hydroxide for the removal of bisphenol A and ibuprofen, J. Colloid Interface Sci., 2019, 541, 12–17 CrossRef CAS PubMed.
- Y. Zhao, C. W. Cho, L. Cui, W. Wei, J. Cai and G. Wu, et al., Adsorptive removal of endocrine-disrupting compounds and a pharmaceutical using activated charcoal from aqueous solution: kinetics, equilibrium, and mechanism studies, Environ. Sci. Pollut. Res., 2019, 26, 33897–33905 CrossRef CAS PubMed.
- J. Heo, Y. Yoon, G. Lee, Y. Kim, J. Han and C. M. Park, Enhanced adsorption of bisphenol A and sulfamethoxazole by a novel magnetic CuZnFe2O4–biochar composite, Bioresour. Technol., 2019, 281, 179–187 CrossRef CAS.
- N. S. Alhokbany, M. Naushad, V. Kumar, S. Al hatim, S. M. Alshehri and T. Ahamad, Self-Nitrogen doped carbons aerogel derived from waste cigarette butts (cellulose acetate) for the adsorption of BPA: Kinetics and adsorption mechanisms, J. King Saud Univ. Sci., 2020, 3351–3358 CrossRef.
- X. Tang, P. Tang, S. Si and L. Liu, Adsorption and removal of bisphenol A from aqueous solution by p-phenylenediamine modified magnetic graphene oxide, J. Serb. Chem. Soc., 2017, 82(1), 39–50 CrossRef CAS.
- A. Supong, P. C. Bhomick, M. Baruah, C. Pongener, U. B. Sinha and D. Sinha, Adsorptive removal of Bisphenol A by biomass activated carbon and insights into the adsorption mechanism through density functional theory calculations, Sustainable Chem. Pharm., 2019, 100159 CrossRef.
- M. H. Dehghani, R. R. Karri, M. Alimohammadi, S. Nazmara, A. Zarei and Z. Saeedi, Insights into endocrine-disrupting Bisphenol-A adsorption from pharmaceutical effluent by chitosan immobilized nanoscale zero-valent iron nanoparticles, J. Mol. Liq., 2020, 113317 CrossRef CAS.
- G. G. Hacıosmanoğlu, T. Doğruel, S. Genç, E. T. Oner and Z. S. Can, Adsorptive removal of bisphenol A from aqueous solutions using phosphonated levan, J. Hazard. Mater., 2019, 43–49 CrossRef.
- M. A. Ahsan, M. T. Islam, C. Hernandez, H. Kim, Y. Lin and M. L. Curry, et al., Adsorptive Removal of Sulfamethoxazole and Bisphenol A from Contaminated Water using Functionalized Carbonaceous Material Derived from Tea Leaves, J. Environ. Chem. Eng., 2018, 4215–4225 CrossRef CAS.
- X. Guo, Y. Huang, W. Yu, X. Yu, X. Han and H. Zhai, Multi-walled carbon nanotubes modified with iron oxide and manganese dioxide (MWCNTs-Fe3O4−MnO2) as a novel adsorbent for the determination of BPA, Microchem. J., 2020, 104867 CrossRef CAS.
- K. Noufel, N. Djebri, N. Boukhalfa, M. Boutahala and A. Dakhouche, Removal of bisphenol A and trichlorophenol from aqueous solutions by adsorption with organically modified bentonite, activated carbon composites: A comparative study in single and binary systems, Groundw. Sustain. Dev., 2020, 100477 CrossRef.
- M. A. Ahsan, V. Jabbari, M. T. Islam, R. S. Turley, N. Dominguez and H. Kim, et al., Sustainable synthesis and remarkable adsorption capacity of MOF/graphene oxide and MOF/CNT based hybrid nanocomposites for the removal of Bisphenol A from water, Sci. Total Environ., 2019, 306–317 CrossRef CAS.
- T. A. Saleh, M. Tuzen and A. Sarı, Magnetic vermiculite-modified by poly (trimesoyl chloride-melamine) as a sorbent for enhanced removal of bisphenol A, J. Environ. Chem. Eng., 2019, 7(6), 103436 CrossRef CAS.
- J. Hao, Q. Zhang, Y. Liu, P. Chen, X. Zheng and X. Zhuang, et al., A novel nitrogen-containing covalent organic framework adsorbent for the efficient removal of bisphenol A from aqueous solution, J. Taiwan Inst. Chem. Eng., 2020, 113, 204–213 CrossRef CAS.
- X. Tang, P. Tang, S. Si and L. Liu, Adsorption and removal of bisphenol A from aqueous solution by p-phenylenediamine modified magnetic graphene oxide, J. Serb. Chem. Soc., 2017, 82(1), 39–50 CrossRef CAS.
- Z. Gong, S. Li, J. Ma and X. Zhang, Synthesis of recyclable powdered activated carbon with temperature responsive polymer for bisphenol A removal, Sep. Purif. Technol., 2016, 157, 131–140 CrossRef CAS.
- X. Chen, T. Fujiwara, S. Fukahori and T. Ishigaki, Factors affecting the adsorptive removal of bisphenol A in landfill leachate by high silica Y-type zeolite, Environ. Sci. Pollut. Res., 2015, 22, 2788–2799 CrossRef CAS.
- M. H. Dehghani, A. H. Mahvi, N. Rastkari, R. Saeedi, S. Nazmara and E. Iravani, Adsorption of bisphenol A (BPA) from aqueous solutions by carbon nanotubes: kinetic and equilibrium studies, Desalination Water Treat., 2015, 54(1), 84–92 CrossRef CAS.
- J. Xu, L. Wang and Y. Zhu, Decontamination of bisphenol A from aqueous solution by graphene adsorption, Langmuir, 2012, 28(22), 8418–8425 CrossRef CAS.
- T. Bao, M. M. Damtie, W. Wei, H. N. P. Vo, K. H. Nguyen and A. Hosseinzadeh, et al., Simultaneous adsorption and degradation of bisphenol A on magnetic illite clay composite: Eco-friendly preparation, characterizations, and catalytic mechanism, J. Cleaner Prod., 2021, 287, 125068 CrossRef CAS.
- R. Kumar, M. Qureshi, D. K. Vishwakarma, N. Al-Ansari, A. Kuriqi and A. Elbeltagi, et al., A review on emerging water contaminants and the application of sustainable removal technologies, Case Stud. Chem. Environ. Eng., 2022, 6, 100219 CrossRef CAS.
- F. Boysan and A. Çavunt, Investigation of bisphenol A removal using peroxy electrocoagulation method, Desalin. Water Treat., 2021, 222, 426–433 CrossRef CAS.
- Y. Tang, X. Yin, M. Mu, Y. Jiang, X. Li and H. Zhang, et al., Anatase TiO2@ MIL-101 (Cr) nanocomposite for photocatalytic degradation of bisphenol A, Colloids Surf., A, 2020, 596, 124745 CrossRef CAS.
- M. Dolatabadi, R. Malekahmadi, A. Ghorbanian and S. Ahmadzadeh, Investigation of electrocoagulation process for efficient removal of bisphenol A from the aqueous environment: Promising treatment strategy, J. Environ. Health Sustainable Dev., 2021, 1275–1283 CAS.
- Y. Huang, M. Su, Y. Zhou, D. Chen, Z. Xu and H. Zhang, et al., LiCl–CN nanotubes ceramic films with highly efficient visible light - Driven photocatalytic active for bisphenol A degradation and efficient regeneration process, Ceram. Int., 2020, 26492–26501 CrossRef CAS.
- A. Chmayssem, S. Taha and D. Hauchard, Scaled-up electrochemical reactor with a fixed bed three-dimensional cathode for electro-Fenton process: Application to the treatment of bisphenol A, Electrochim. Acta, 2017, 225, 435–442 CrossRef CAS.
- Z. Pan, F. Yu, L. Li, C. Song, J. Yang and C. Wang, et al., Electrochemical microfiltration treatment of bisphenol A wastewater using coal-based carbon membrane, Sep. Purif. Technol., 2019, 227, 115695 CrossRef CAS.
- S. Li, G. Zhang, P. Wang, H. Zheng and Y. Zheng, Microwave-enhanced Mn-Fenton process for the removal of BPA in water, Chem. Eng. J., 2016, 294, 371–379 CrossRef CAS.
- S. Parastar, A. Ebrahimi, H. Mohammadi, N. Mengelizadeh, I. Parseh and N. Rafiei, et al., Removal of BPA from aqueous solutions by electrocoagulation using iron electrodes and optimization, J. Environ. Health Eng., 2018, 5(3), 264–276 CrossRef CAS.
- A. Aziz, P. Agamuthu and S. H. Fauziah, Removal of bisphenol A and 2, 4-Di-tert-butylphenol from landfill leachate using plant-based coagulant, Waste Manag. Res., 2018, 36(10), 975–984 CrossRef CAS.
- J. Bai, Y. Li, B. Song and Q. Wang, Activation of peroxymonosulfate by modified coagulation sludge for bisphenol A degradation, Environ. Sci. Pollut. Res., 2022, 29(52), 78832–78847 CrossRef CAS PubMed.
- E. Bazrafshan, H. Biglari and A. H. Mahvi, Phenol removal by electrocoagulation process from aqueous solutions, Fresenius Environ. Bull., 2012, 21(2), 364–371 CAS.
- N. Ambauen, C. Weber, J. Muff, C. Hallé and T. Meyn, Electrochemical removal of Bisphenol A from landfill leachate under Nordic climate conditions, J. Appl. Electrochem., 2020, 50, 1175–1188 CrossRef CAS.
- S. Parastar, A. Ebrahimi, H. Mohammadi, N. Mengelizadeh, I. Parseh and N. Rafiei, et al., Removal of BPA from aqueous solutions by electrocoagulation using iron electrodes and optimization, J. Environ. Health Eng., 2018, 5(3), 264–276 CrossRef CAS.
- P. Guerra, M. Kim, S. Teslic, M. Alaee and S. A. A. Smyth, Bisphenol-A removal in various wastewater treatment processes: Operational conditions, mass balance, and optimization, J. Environ. Manage., 2015, 152, 192–200 CrossRef CAS PubMed.
- M. Umar, F. Roddick, L. Fan and H. A. Aziz, Application of ozone for the removal of bisphenol A from water and wastewater – A review, Chemosphere, 2013, 90(8), 2197–2207 CrossRef CAS PubMed.
- X. Si, Z. Hu and S. Huang, Combined Process of Ozone Oxidation and Ultrafiltration as an Effective Treatment Technology for the Removal of Endocrine-Disrupting Chemicals, Appl. Sci., 2018, 8(8), 1240 CrossRef.
- M. Dudziak and E. Burdzik-Niemiec, Comparative studies on elimination of estrogens and xenoetrogens by the oxidation processes, Ecol. Chem. Eng. A, 2014, 21(2), 189–198 CAS.
- M. Deborde, S. Rabouan, P. Mazellier, J. P. P. Duguet and B. Legube, Oxidation of bisphenol A by ozone in aqueous solution, Water Res., 2008, 42(16), 4299–4308 CrossRef CAS PubMed.
- T. Garoma, S. A. Matsumoto, Y. Wu and R. Klinger, Removal of Bisphenol A and its Reaction-Intermediates from Aqueous Solution by Ozonation, Ozone: Sci. Eng., 2010, 32(5), 338–343 CrossRef CAS.
- F. Du, Z. Lai, H. Tang, H. Wang and C. Zhao, Construction of dual Z-scheme Bi2WO6/g-C3N4/black phosphorus quantum dots composites for effective bisphenol A degradation, J. Environ. Sci., 2023, 124, 617–629 CrossRef CAS PubMed.
- E. García-Díaz, D. Zhang, Y. Li, R. Verduzco and P. J. J. Alvarez, TiO2 microspheres with cross-linked cyclodextrin coating exhibit improved stability and sustained photocatalytic degradation of bisphenol A in secondary effluent, Water Res., 2020, 183, 116095 CrossRef PubMed.
- H. Wang, N. Zhang, G. Cheng, H. Guo, Z. Shen and L. Yang, et al., Preparing a photocatalytic Fe doped TiO2/rGO for enhanced bisphenol A and its
analogues degradation in water sample, Appl. Surf. Sci., 2020, 505, 144640 CrossRef CAS.
- Z. You, Z. Wang, K. Tian and X. Yao, Activation of persulfate by MIL-100(Fe) coated with perylene diimide heterojunction photocatalyst for efficient removal of Bisphenol A in visible light, J. Environ. Chem. Eng., 2023, 11(3), 110159 CrossRef CAS.
- Y. Zhang and J. L. Zhou, Occurrence and removal of endocrine disrupting chemicals in wastewater, Chemosphere, 2008, 73(5), 848–853 CrossRef CAS.
- I. Sánchez-Montes, N. Wachter, B. F. Silva and J. M. Aquino, Comparison of UVC-based advanced oxidation processes in the mineralization of bisphenol A: Identification of oxidation by products and toxicity evaluation, Chem. Eng. J., 2020, 386, 123986 CrossRef.
- B. Huang, X. Li, W. Sun, D. Ren, X. Li and X. Li, et al., Occurrence, removal, and fate of progestogens, androgens, estrogens, and phenols in six sewage treatment plants around Dianchi Lake in China, Environ. Sci. Pollut. Res., 2014, 21(22), 12898–12908 CrossRef CAS.
- A. Wang, Z. Hua, Z. Wu, C. Chen, S. Hou and B. Huang, et al., Insights into the effects of bromide at fresh water levels on the radical chemistry in the UV/peroxydisulfate process, Water Res., 2021, 197, 117042 CrossRef CAS.
- A. Cai, J. Deng, X. Ling, C. Ye, H. Sun and Y. Deng, et al., Degradation of bisphenol A by UV/persulfate process in the presence of bromide: Role of reactive bromine, Water Res., 2022, 215, 118288 CrossRef CAS.
- H. Abu Hasan, M. H. Muhamad, S. Budi Kurniawan, J. Buhari and O. Husain Abuzeyad, Managing Bisphenol A Contamination: Advances in Removal Technologies and Future Prospects, Water, 2023, 15(20), 3573 CrossRef CAS.
- E. Zhang, P. Zhao, G. Xu, F. Meng, X. Wang and Y. Gao, et al., High efficiency manganese cobalt spinel structure catalytic ozonation ceramic membrane for in situ BPA degradation and membrane fouling elimination, J. Environ. Chem. Eng., 2024, 12(1), 111774 CrossRef CAS.
- S. Wang, Q. Wu, B. Yan, Y. Guo, W. Xia and J. Li, et al., A novel integrated process of ceramic membrane filtration coupled with peroxymonosulfate activation and adsorption for water treatment, Sep. Purif. Technol., 2022, 120874 CrossRef CAS.
- J. Xie, Z. Liao, M. Zhang, L. Ni, J. Qi and C. Wang, et al., Sequential ultrafiltration-catalysis membrane for excellent removal of multiple pollutants in water, Environ. Sci. Technol., 2021, 2652–2661 CrossRef CAS.
- Y. Huang, W. Xu, L. Hu, J. Zeng, C. He and X. Tan, et al., Combined adsorption and catalytic ozonation for removal of endocrine disrupting compounds over MWCNTs/Fe3O4 composites, Catal. Today, 2017, 297, 143–150 CrossRef CAS.
- Z. Li, Z. Yang, Q. Shi and Y. Sun, Removal of bisphenol A by integrated adsorption and biodegradation using immobilized laccase onto defective PCN-224, J. Environ. Chem. Eng., 2023, 11(3), 110166 CrossRef CAS.
- I. Escalona, J. de Grooth, J. Font and K. Nijmeijer, Removal of BPA by enzyme polymerization using NF membranes, J. Membr. Sci., 2014, 192–201 CrossRef CAS.
- C. Hu, D. Huang, G. Zeng, M. Cheng, X. Gong and R. Wang, et al., The combination of Fenton process and Phanerochaete chrysosporium for the removal of bisphenol A in river sediments: Mechanism related to extracellular enzyme, organic acid and iron, Chem. Eng. J., 2018, 432–439 CrossRef CAS.
- G. Bertanza, R. Pedrazzani, M. Dal Grande, M. Papa, V. Zambarda and C. Montani, et al., Effect of biological and chemical oxidation on the removal of estrogenic compounds (NP and BPA) from wastewater: An integrated assessment procedure, Water Res., 2011, 2473–2484 CrossRef CAS.
- J. F. d. Oliveira, R. Fia, F. N. Rodrigues, F. R. L. Fia, M. P. d. Matos and L. A. B. Siniscalchi, et al., Quantification, removal and potential ecological risk of emerging contaminants in different organic loads of swine wastewater treated by integrated biological reactors, Chemosphere, 2020, 260, 127516 CrossRef CAS.
- A. Tarafdar, R. Sirohi, P. A. Balakumaran, R. Reshmy, A. Madhavan and R. Sindhu, et al., The hazardous threat of Bisphenol A: Toxicity, detection and remediation, J. Hazard. Mater., 2022, 127097 CrossRef CAS.
- C. B. Godiya and B. J. Park, Removal of bisphenol A from wastewater by physical, chemical and biological remediation techniques. A review, Environ. Chem. Lett., 2022, 20(3), 1801–1837 CrossRef CAS.
- M. Zielińska, I. Wojnowska-Baryła and A. Cydzik-Kwiatkowska, Integrated Systems for Removal of BPA from Wastewater, in Bisphenol A Removal from Water and Wastewater, 2019 Search PubMed.
- M. F. M. A. Zamri, R. Bahru, F. Suja', A. H. Shamsuddin, S. K. Pramanik and I. M. R. Fattah, Treatment strategies for enhancing the removal of endocrine-disrupting chemicals in water and wastewater systems, J. Water Proc. Eng., 2021, 102017 CrossRef.
- F. M. Mpatani, R. Han, A. A. Aryee, A. N. Kani, Z. Li and L. Qu, Adsorption performance of modified agricultural waste materials for removal of emerging micro-contaminant bisphenol A: a comprehensive review, Sci. Total Environ., 2021, 780, 146629 CrossRef CAS PubMed.
- Y. Zhang, Y. Cheng, N. Chen, Y. Zhou, B. Li and W. Gu, et al., Recyclable removal of bisphenol A from aqueous solution by reduced graphene oxide–magnetic nanoparticles: adsorption and desorption, J. Colloid Interface Sci., 2014, 421, 85–92 CrossRef CAS PubMed.
- N. H. Dang, T. H. Tu, V. N. P. Linh, L. T. M. Thy, H. M. Nam and M. T. Phong, et al., Preparation of magnetic iron oxide/graphene aerogel nanocomposites for removal of bisphenol A from water, Synth. Met., 2019, 255, 116106 CrossRef.
- K. Ouyang, C. Zhu, Y. Zhao, L. Wang, S. Xie and Q. Wang, Adsorption mechanism of magnetically separable Fe3O4/graphene oxide hybrids, Appl. Surf. Sci., 2015, 355, 562–569 CrossRef CAS.
- B. Seyhi, P. Drogui, G. Buelna, A. Azaïs and M. Heran, Contribution of a submerged membrane bioreactor in the treatment of synthetic effluent contaminated by Bisphenol-A: mechanism of BPA removal and membrane fouling, Environ. Pollut., 2013, 180, 229–235 CrossRef CAS.
- M. J. F. Jasni, M. Arulkumar, P. Sathishkumar, A. R. M. Yusoff, N. A. Buang and F. L. Gu, Electrospun nylon 6, 6 membrane as a reusable nano-adsorbent for bisphenol A removal: Adsorption performance and mechanism, J. Colloid Interface Sci., 2017, 508, 591–602 CrossRef CAS.
- L. E. Koloti, N. P. Gule, O. A. Arotiba and S. P. Malinga, Laccase-immobilized dendritic nanofibrous membranes as a novel approach towards the removal of bisphenol A, Environ. Technol., 2018, 39(3), 392–404 CrossRef CAS PubMed.
- S. Yüksel, N. Kabay and M. Yüksel, Removal of bisphenol A (BPA) from water by various nanofiltration (NF) and reverse osmosis (RO) membranes, J. Hazard. Mater., 2013, 263, 307–310 CrossRef.
- P. A. K. Reddy, P. V. L. Reddy, E. Kwon, K. H. Kim, T. Akter and S. Kalagara, Recent advances in photocatalytic treatment of pollutants in aqueous media, Environ. Int., 2016, 91, 94–103 CrossRef CAS.
- V. Repousi, A. Petala, Z. Frontistis, M. Antonopoulou, I. Konstantinou and D. I. Kondarides, et al., Photocatalytic degradation of bisphenol A over Rh/TiO2 suspensions in different water matrices, Catal. Today, 2017, 284, 59–66 CrossRef CAS.
- P. V. L. Reddy, K. H. Kim, B. Kavitha, V. Kumar, N. Raza and S. Kalagara, Photocatalytic degradation of bisphenol A in aqueous media: A review, J. Environ. Manage., 2018, 213, 189–205 CrossRef CAS.
- X. Xiao, S. Tu, M. Lu, H. Zhong, C. Zheng and X. Zuo, et al., Discussion on the reaction mechanism of the photocatalytic degradation of organic contaminants from a viewpoint of semiconductor photo-induced electrocatalysis, Appl Catal B, 2016, 198, 124–132 CrossRef CAS.
- M. Umar, F. Roddick, L. Fan and H. A. Aziz, Application of ozone for the removal of bisphenol A from water and wastewater–a review, Chemosphere, 2013, 90(8), 2197–2207 CrossRef CAS PubMed.
- E. Kusvuran and D. Yildirim, Degradation of bisphenol A by ozonation and determination of degradation intermediates by gas chromatography–mass spectrometry and liquid chromatography–mass spectrometry, Chem. Eng. J., 2013, 220, 6–14 CrossRef CAS.
- H. Pokkiladathu, S. Farissi, A. Sakkarai and M. Muthuchamy, Degradation of bisphenol A: a contaminant of emerging concern, using catalytic ozonation by activated carbon impregnated nanocomposite-bimetallic catalyst, Environ. Sci. Pollut. Res., 2022, 29(48), 72417–72430 CrossRef CAS PubMed.
- R. Shokoohi, H. Zolghadrnasab, S. Shanehsaz, M. Leili, M. Shaygan and F. Azizi, Efficiency of the catalytic ozonation processes using nanoparticles deposited on pumice in the removal of bisphenol A, Int. J. Environ. Anal. Chem., 2023, 103(13), 3121–3137 CrossRef CAS.
- H. Zhang, Y. He, L. Lai, G. Yao and B. Lai, Catalytic ozonation of Bisphenol A in aqueous solution by Fe3O4–MnO2 magnetic composites: Performance, transformation pathways and mechanism, Sep. Purif. Technol., 2020, 245, 116449 CrossRef CAS.
- H. Guo, H. Wang, Q. Wu and J. Li, Degradation and mechanism analysis of bisphenol A in aqueous solutions by pulsed discharge plasma combined with activated carbon, Sep. Purif. Technol., 2018, 190, 288–296 CrossRef CAS.
- M. R. Samarghandi, A. Ansari, A. Dargahi, A. Shabanloo, D. Nematollahi and M. Khazaei, et al., Enhanced electrocatalytic degradation of bisphenol A by graphite/β-PbO2 anode in a three-dimensional electrochemical reactor, J. Environ. Chem. Eng., 2021, 9(5), 106072 CrossRef CAS.
- S. Ahmadzadeh and M. Dolatabadi, Modeling and kinetics study of electrochemical peroxidation process for mineralization of bisphenol A; a new paradigm for groundwater treatment, J. Mol. Liq., 2018, 254, 76–82 CrossRef CAS.
- Y.-h. Cui, L. X. yan and G. Chen, Electrochemical degradation of bisphenol A on different anodes, Water Res., 2009, 43(7), 1968–1976 CrossRef CAS.
- M. D. Simić, T. P. Brdarić, B. G. S. Rosić, Ľ. Švorc, D. J. Relić and D. D. Aćimović, Degradation of bisphenol A via the electro–Fenton process using nanostructured carbon-metal oxide anodes: Intermediates and reaction mechanisms study, J. Environ. Chem. Eng., 2024, 12(5), 113369 CrossRef.
- J. da Silveira Salla, K. da Boit Martinello, G. L. Dotto, E. García-Díaz, H. Javed and P. J. J. Alvarez, et al., Synthesis of citrate–modified CuFeS2 catalyst with significant effect on the photo–Fenton degradation efficiency of bisphenol a under visible light and near–neutral pH, Colloids Surf., A, 2020, 595, 124679 CrossRef.
- Y. Liu, Y. Mao, X. Tang, Y. Xu, C. Li and F. Li, Synthesis of Ag/AgCl/Fe-S plasmonic catalyst for bisphenol A degradation in heterogeneous photo-Fenton system under visible light irradiation, Chin. J. Catal., 2017, 38(10), 1726–1735 CrossRef CAS.
- G. Li, L. Zu, P. K. Wong, X. Hui, Y. Lu and J. Xiong, et al., Biodegradation and detoxification of bisphenol A with one newly-isolated strain Bacillus sp. GZB: kinetics, mechanism and estrogenic transition, Bioresour. Technol., 2012, 114, 224–230 CrossRef CAS PubMed.
- Y. Jia, A. Eltoukhy, J. Wang, X. Li, T. S. Hlaing and M. M. Aung, et al., Biodegradation of bisphenol A by Sphingobium sp. YC-JY1 and the essential role of cytochrome P450 monooxygenase, Int. J. Mol. Sci., 2020, 21(10), 3588 CrossRef CAS PubMed.
- M. Golshan, S. Jorfi, N. J. Haghighifard, A. Takdastan, S. Ghafari and S. Rostami, et al., Development of salt-tolerant microbial consortium during the treatment of saline bisphenol A-containing wastewater: Removal mechanisms and microbial characterization, J. Water Proc. Eng., 2019, 32, 100949 CrossRef.
- R. Wang, P. Diao, Q. Chen, H. Wu, N. Xu and S. Duan, Identification of novel pathways for biodegradation of bisphenol A by the green alga Desmodesmus sp. WR1, combined with mechanistic analysis at the transcriptome level, Chem. Eng. J., 2017, 321, 424–431 CrossRef CAS.
|
This journal is © The Royal Society of Chemistry 2024 |
Click here to see how this site uses Cookies. View our privacy policy here.