DOI:
10.1039/D4TB01858C
(Review Article)
J. Mater. Chem. B, 2025,
13, 37-53
Unravelling molecular mechanobiology using DNA-based fluorogenic tension sensors
Received
15th August 2024
, Accepted 4th November 2024
First published on 6th November 2024
Abstract
Investigations of the biological system have revealed many principles that govern regular life processes. Recently, the analysis of tiny mechanical forces associated with many biological processes revealed their significance in understanding biological functions. Consequently, this piqued the interest of researchers, and a series of technologies have been developed to understand biomechanical cues at the molecular level. Notable techniques include single-molecule force spectroscopy, traction force microscopy, and molecular tension sensors. Well-defined double-stranded DNA structures could possess programmable mechanical characteristics, and hence, they have become one of the central molecules in molecular tension sensor technology. With the advancement of DNA technology, DNA or nucleic acid-based robust tension sensors offer the possibility of understanding mechanobiology in the bulk to single-molecule level range with desired spatiotemporal resolution. This review presents a comprehensive account of molecular tension sensors with a special emphasis on DNA-based fluorogenic tension sensors. Along with a detailed discussion on irreversible and reversible DNA-based tension sensors and their application in super-resolution microscopy, a discussion on biomolecules associated with cellular mechanotransduction and key findings in the field are included. This review ends with an elaborate discussion on the current challenges and future prospects of molecular tension sensors.
1. Introduction
Living systems are functional owing to a combination of complex but well-regulated processes. Long before the understanding of molecules and the availability of cell culture technologies, researchers had started to realize that mechanical forces have considerable effects on the biological system. The study of the skeleton and muscle structure from a physical and mathematical perspective was the basis of such early arguments. D. W. Thompson scientifically articulated the physical and mathematical basis of different physical forms of different living organisms in his book ‘On Growth and Form’. Once the molecular and genetic understanding improved and cell culture technologies emerged, along with various biochemical methods, quantitative study of the origin and effects of mechanical forces at the cellular and subcellular level became possible. If we consider the basic unit of life—cells, there are different types of cells, some of them tightly held together, forming tissues of various stiffnesses, some of them moving freely in the bloodstream and some of them changing their behaviour depending on the circumstances. Depending on the cell type, some cells are soft and some are hard and of different sizes and shapes (Fig. 1A).1 As cells are part of the same living system, they often actively interact with each other. The mechanical forces that originate as a result of such interactions are indispensable in regulating a vast range of biological processes.2–6 Systematic understanding of the nature, type and magnitude of mechanical forces and their effects on biological processes is the central theme of mechanobiology. Cells can sense the environment around them based on their physical and chemical characteristics, and mechanical force can be generated at one place and transmitted to a different place through a network of complex biomolecular cues; these are known as mechanosensing and mechanotransduction, respectively.3,5 Mechanotransduction can transpire from various fundamental biological processes, such as cell adhesion on the extracellular matrix (ECM), cell migration, differentiation, cell–cell interactions, cell–pathogen interactions, and immune cell–antigen presenting cell (APC) interactions. (Fig. 1B).6–9 The perception of mechanical force by certain cells can even control the gene expression or so-called mechanogenetics.10 Consequently, comprehensive investigation of the occurrence of the mechanical tension and transmission of the tension through mechanosensitive adaptor proteins and their effect on the entire cellular level decision-making process has become a great area of interest.
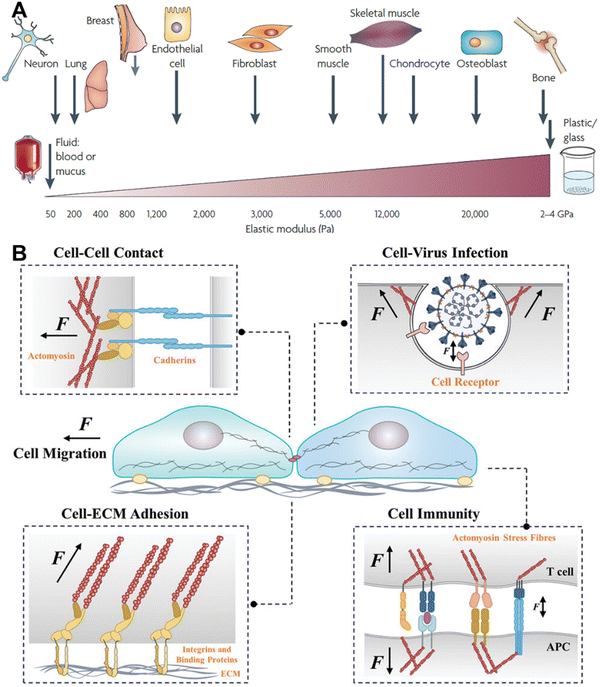 |
| Fig. 1 A. Stiffness of different types of cells and some mechanically static tissues, reproduced with permission from ref. 1, Copyright 1969, Springer Nature Limited. B. Various cell–environment interactions that lead to the generation of mechanical cues; reproduced with permission from ref. 11, Copyright 2024, Wiley. | |
Considering the size of the cellular components and the miniature amount of the generated force, attuned techniques have been developed in the last few decades.11–13 Force in the range of a few piconewton (pN) to nanonewton (nN) is produced by different biomolecules in various events within a very tiny space and time span. In recent years, a range of biocompatible physical substrate and chemical-based techniques have been developed and successfully applied by researchers to study the mechanical tension at the cellular, subcellular, and molecular level in live cells with desired spatial and temporal resolution in real time.11–15
2. Molecules involved in mechanotransduction
Cells sense mechanical stimuli primarily at the cell surface via mechanosensitive receptor molecules or proteins such as integrin, G-protein coupled receptor, growth factor receptor, and Notch receptor, etc.16–18 The interaction or sensing of the stimuli by the cell surface receptors can be majorly divided into cell–matrix and cell–cell interactions.
When a cell come into contact with a surface of suitable stiffness, they form a well-defined and dynamic multiprotein complex called focal adhesion (FA), which helps cells to adhere to the surface.19,20 Apart from cell adhesion, FA also plays a prominent role in cell migration.21,22 FA is the hub of mechanosensing, where cells receive the extracellular mechanical signal and link that to the intracellular cytoskeleton (Fig. 2A).20,23 The intracellular part of the FA is mechanically linked to the ECM via transmembrane protein integrin. The diverse family of integrins function as heterodimers, composed of α and β subunits, with 24 different assemblies known from 18 α and 9 β subunits.24 The extracellular domain of integrin recognizes the specific ligand on the constituents of the ECM, namely fibronectin, collagen, vitronectin, laminins, etc.25,26 When integrins bind to the ligand, mechanical tension is generated, which initiates the outside-in signalling by the structural alteration.27 The strength of the tension and the stiffness of the ECM governs the size and lifetime of the FA.28 During the outside-in signalling, integrin gets activated and the cytoplasmic domain binds to the cytoskeletal actin via adaptor protein talin. The binding between the integrin and actin is reinforced by other adaptor and docking proteins, such as paxillin, vinculin, and zyxin, and forms the cytoplasmic part of the FA.12 In response to the mechanical stimulus, one of the first biochemical events that happens is auto-phosphorylation of the nonreceptor tyrosine kinase, i.e. focal adhesion kinase (FAK).29 The linker protein talin is composed of a globular head, helices, and a flexible rod domain.30 Talin directly links integrin and actin by interacting with the cytoplasmic domain of β-integrin and actin. It also has a binding site for associated proteins, like vinculin. Talin plays an important role in mechanotransduction as its rod domain works as a tension buffer. In the absence of force, the rod domain is completely structured, and vinculin cannot bind, but the binding site is exposed when force is applied. Vinculin, one of the very important proteins in the FA complex, binds to the activated talin with its head domain.31 As tension is required for the recruitment of vinculin, its presence in the FA site implies that FA is under tension.32 Experiments have shown that vinculin is very important for the generation of cellular force (especially at a high level) and regulation of the FA assembly, as vinculin knocked-out cells show hindered contractility and longer-lived FAs.33 Another docking protein of FA is paxillin, which integrates the force originating from the ECM–integrin interaction and propagates this to the cytoskeleton. Zyxin is an FA-associated docking protein, which works as the mechanosensitive regulator of FA.34 It can be released from FA upon releasing the tension and can inhibit actomyosin interaction and can even directly regulate actin polymerization. As mentioned, mechanosensitive FAK is one of the very important molecules that biochemically respond to force by autophosphorylation and generate downstream biochemical signalling.35 FAK has multiple phosphorylation sites, and the degree of phosphorylation depends on the force applied.
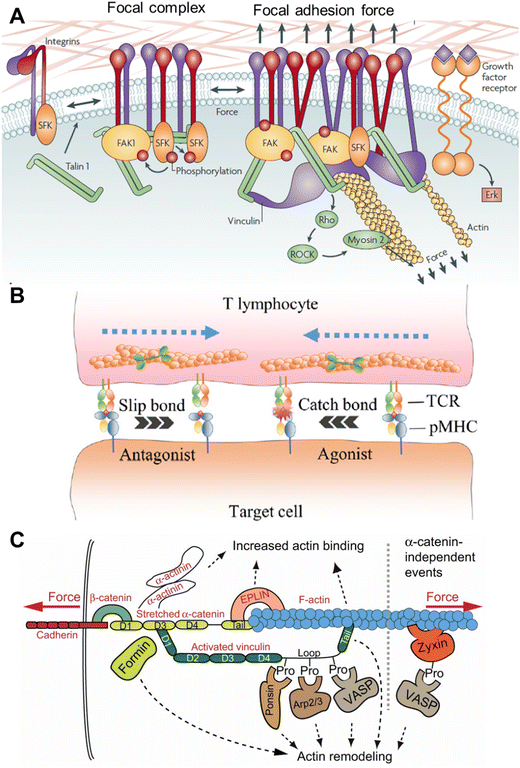 |
| Fig. 2 A. Mechanotransduction and molecules involved in the focal adhesion and focal complex; reproduced with permission from ref. 1, Copyright 1969, Springer Nature Limited. B. T cell activation via the TCR–pMHC linkage, which contributes to discriminating specific antagonists and agonists; reproduced from ref. 12, Copyright 2024, Elsevier. C. Force-induced events during cadherin–F-actin interaction; reproduced from ref. 36, Copyright 2013, The Company of Biologists. | |
The tension originating from the extracellular interaction propagates to the cytoskeleton through a dynamic network of filamentous actin (F-actin) and crosslinking protein actinin.3,28 The force-withstanding cytoskeleton is primarily made of F-actin and held together by crosslinking proteins and slides by the motor protein myosin. Myosin works on F-actin and pulls the entire ECM–FA linkage to propagate to the other part of the cell. The interplay of the mechanotransduction between FA and the cytoskeleton is important for directional cell migration.21,22 Like FA, many F-actin binding proteins, like Arp2/3, and profilin, regulate the F-actin polymerization–depolymerization dynamics in a force-dependent manner.37,38 On the other hand, the activity of myosin II is directly regulated by the phosphorylation of its light chain by Rho-associated coiled-coil kinase (ROCK) as a response to mechanical load.39 Overall, there are plenty of molecules that either directly or indirectly take part in mechanosensing and mechanotransduction during cell–ECM interactions, but only the functions of the key molecules are discussed above.
Another way of perceiving molecular tension is through cell–cell interactions. Two cells can just collide with each other like two softballs and generate non-specific physical force, but a specific molecular-level interaction is also possible. In this case, the ligand is on the surface of one cell and the receptor is on the membrane of another cell, which interact and generate physical force and initiate mechanical cues known as juxtacrine signaling.9,40 When two cells physically come close to each other, the generation of force can occur because of cellular movement, membrane reorganization, or the cytoskeletal dynamics after receptor–ligand binding. One such kind of mechanical force production is observed when immune and cancer cells interact with each other, and the force plays a very important role in activating the immune cells.9,41,42 Immune cells patrol the body as suspension cells but upon activation, they adhere to the surface and start squeezing through the tissue to reach the target cells. This entire process is regulated intensely by the physical force or stimulus.43 It has been shown by many researchers that immune cells, such as monocytes, macrophages, lymphocytes, and neutrophils, get stimulated by both mechanical and biochemical signals.8,44 Very similar to integrin-mediated mechanosensing and mechanotransduction, the recognition at the outer membrane of the immune cell happens by specific receptors like T cell receptor (TCR), and B cell receptor (BCR). T/B cells are adaptive immune cells that search for harmful materials in the body, specifically infected and cancer cells. Virus- or bacteria-infected cells and cancer cells (APC) present the antigens on the major histocompatibility complex (pMHC), projecting them towards the outer plasma membrane, and TCR has high specificity for those antigens. TCRs are heterodimeric proteins and contain both binding and signalling motifs. Once TCR binds with pMHC, a mechanical signal is generated in the next few minutes as a series of well-regulated phenomena happens (Fig. 2B).45,46 There are coreceptors of TCR, namely CD4 or CD8, that recruit Lck kinase and phosphorylate the tyrosine at the cytoplasmic signalling domain of CD3. This is basically the start of a sequential phosphorylation binding event. Following the CD3 phosphorylation, binding with Zap70 kinase happens, which in turns phosphorylates the linker for activating T cells (LAT). LAT recruits and activates PLCγ1, Grb2, Ras, Rho GTPase, and Rac and induces Ca2+ signalling. The above processes are critical for immune cell activation and for killing APC directly by forming immune synapses or recruiting other immune cells by releasing cytokines.45
Apart from the FA or immune cell activation-related molecules, mechanosensing and mechanotransduction can also occur through other classes of cell surface molecules. Cadherin at the cell surface can sense and transduce shear force through actomyosin coupled with F-actin (Fig. 2C).36,40 Ion channels (one of the fastest mechanosensors) can sense multiple types of stimuli, such as temperature, pH, voltage, and osmotic pressure, along with shear stress and membrane stretching with mechanoresponsive ion channels, such as Piezo1 and Piezo2.47,48 The Notch receptor family can bind to specific delta-like ligands (DLLs) with their extracellular domain and activate the intracellular domain, which is then released and binds to the transcription factor complex and regulates the same.17,49 One study revealed that Yes-associated protein (YAP) and transcriptional coactivator with the PDZ-binding motif TAZ are mechanosensitive transcription factors. YAP/TAZ are activated by external cellular stiffening as mediated through the cytoskeleton. Their activity is crucial for the ECM-induced differentiation of mesenchymal cells and the cell geometry-regulated survival of endothelial cells.50 Also, the pathogen signal-specific receptors on the surface of innate immune cells, such as macrophages, dendritic cells, and neutrophils, can sense and respond to mechanical force.44 CD48 on the filopodia of macrophages can specifically bind to fimbrin, a mannose-specific adhesin of Escherichia coli, and stop it from escaping.51 This is particularly important for the pathophysiology as the physical character and molecular constituents of the pathogen and the cells of our own body have differences. In many cases, the mechanosensitive molecule itself can translocate from one place to another in the cell and work like a chemical signal.49 In summary, flexible and dynamic biomolecules that can respond to mechanical force either by exposing a reactive site, like phosphorylation, or protease site or exposing a binding site are the key to mechanosensing, which interlinks mechanical stimuli and biochemical signalling.
3. Techniques for studying mechanotransduction
Long before any molecular understanding, the effect of force or mechanics was well appreciated from physical and mathematical perspectives. Soon after the advancement of cell culture technologies and molecular biology, and a few early findings, like the ability of cancer cells to grow on soft agar gel unlike healthy cells, and the change of the morphology of erythrocytes in changing the membrane tension inspired researchers to devote more efforts in this field.52,53 Realizing the effects of different types of mechanical forces in the biological system and their effects on decision-making, a long-standing quest was begun to investigate the force at the cellular, subcellular, and molecular levels. As we understood in the previous section, mechanotransduction often originates from the exogenous regime and the magnitude of the force is very small. In this section, the different types of techniques developed to measure the force generated at the cellular and molecular levels with various force and spatial resolutions are discussed (Fig. 3).
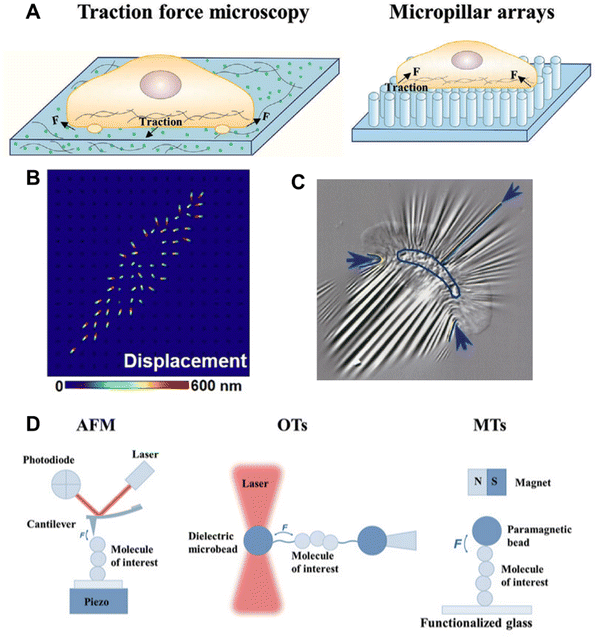 |
| Fig. 3 A. Microbead-embedded and micropillar arrays-based traction force microscopy; reproduced with permission from ref. 11, Copyright 2024, Wiley. B. Displacement signal simulation of the strained PLNA array under CTF; reproduced from ref. 54, Copyright 2021, American Association for the Advancement of Science C. Wrinkle formation on an elastic silicon membrane by a migrating keratocyte; reproduced from ref. 55, Copyright 1999, The American Society for Cell Biology. D. Single-molecule force spectroscopy techniques; reproduced with permission from ref. 11, Copyright 2024, Wiley. | |
3.1. Traction force microscopy
In 1980, one of the very early methods of detecting cellular force was traction force microscopy (TFM), prototypically introduced by A. K. Harris et al.56 They qualitatively observed that cells cultured on an elastic silicon membrane form wrinkles. Although it was qualitative, it was a direct observation of cellular tension generation on a substrate. Later, true quantitative TFM was achieved by integrating computer algorithms, which provided refined force measurement by computation. In a typical TFM set-up, fluorescent microbeads are embedded in an elastic hydrogel, such as polyacrylamide (PMA) gel, and the surface is modified with ECM proteins, such as collagen or fibronectin, and the cells are cultured on this.57 Similar to the elastic silicon membrane, the gel will deform due to the force generated by the cell, which can be seen and quantitated by displacement of the fluorescent beads.55,58–60 Elastic silicon film-based force measurement can be applied to the real-time visualization of force, such as the force generated while a fast-moving fish keratocyte migrates.56 Because they have a higher refractive index, silicone-based elastic platforms are used more often as they can be integrated with total internal reflection fluorescence (TIRF) microscopy. With continuous effort in the field, the resolution and compatibility of TFM are improving. Waterman's lab developed a confocal microscope-compatible version, wherein they used a fibronectin-coated PMA substrate with ∼20 μm thickness.61 In recent times, polymer-based substrates embedded with a high spatial density of small-sized beads (nanobeads) have been used to obtain a resolution up to 40–80 nm.62 In 2018, O. Chaudhuri's lab developed a three-dimensional TFM system and used it to investigate force generation during cell division.63 Their experiments revealed that dividing cells generate protrusive force that deforms the surroundings and creates space for mitotic elongation, and also showed that the lack of force could not generate enough space, leading to mitosis failure. Using the same technology, they also revealed that cancer tissue uses basal force and protease in tandem to invade.64 To avoid the difficulty that arises because of the need for post-imaging complex mathematical analysis to obtain the stress field from the deformation field, another version of TFM was introduced by J. L. Tan et al.58 They used an array of elastic micropillars or microneedles made of poly-dimethylsiloxane (PDMS) in place of the elastic gel/membrane. In this setup, the stress map could be obtained from the information about the elastic characteristics of the PDMS micropillars. A study of the interaction between T cells and APC on a micropillar (6 μm height, 0.7 μm diameter, and 2 μm spacing)-decorated substrate revealed that T cells can exert local and asymmetric force in the immunological synapse.65 The micropillar array-based substrate is not limited by the inert PDMS, but could also be extended to a piezo-phototronic active light nano-antenna made of InGaN/GaN. Using this innovative set-up, they achieved 800 nm spatial and 300 ms temporal resolutions.54
3.2. Bio-membrane force probe technique
Another very interesting type of force measuring technique is the bio-membrane force probe (BFP). Here, red blood cells or artificial lipid vesicles with known membrane rigidity are used as the BFP. In a typical set-up, a ligand-functionalized bead is sandwiched between the BFP (held by a micropipette tip) and the substrate of interest. Pulling or pushing the bead with the micropipette and the deformation of the BFP can provide information about the physical characteristics of the substrate.66
3.3. Atomic force microscopy
In material characterization, atomic force microscopy (AFM) has been used extensively. With the improvement of the cantilever fabrication, AFM is now being used quite commonly to measure single-molecule forces in biological systems. Pulling a well-calibrated flexible cantilever (functionalized with a molecule of interest, such as a receptor-specific ligand) after binding with the target protein immobilized on the cell surface or artificial substrate can provide information about the strength of the force and lifetime of the bond.67 In the early 1990s, researchers started using AFM for mechanobiology studies and achieved 1 pN to 100 nN force sensitivity.68 An AFM study of the mechanical response of TCR using a pMHC-functionalized cantilever showed the effect of mechanical stimuli on the chemical feedback loop of T cell activation.42 Recently, a remarkable Angstrom level resolution was achieved using localization AFM (LAFM), an algorithmically optimized version.69
3.4. Optical tweezer technique
To study relatively lower-level forces as low as 1–60 pN, optical tweezers (OTs) are used. With OTs, the motion of a micron to sub-micron-sized dielectric particle is trapped and manipulated by a laser beam.70 Moving the laser beam or displacement of the particle from the focal point of the laser beam can provide information about the mechanical properties of the particle linked with a biomolecule of interest. An OT was successfully used to activate T cell using a pMHC-functionalized particle by applying about 10 pN of shear load.71 A. D. Bershadsky's group studied the sustainability and growth of filopodia under mechanical pulling using an OT.72 Unlike AFM, OTs work in non-contact mode, and hence enable a contamination-free application and detection of force. However, the high light absorbance and varying refractive index of biological tissues hinder the extensive use of OTs for mechanobiological experiments.
3.5. Magnetic tweezer technique
A functionalized magnetic bead (similar to the dielectric particle in OT) is physically manipulated using a magnetic field in the magnetic tweezer (MT) technique. This is also a non-contact mode and single-molecule microscopy method, which additionally has the ability to measure force in the range of pN to a few nN.73,74 The binding between talin and chaperon was studied using MT, revealing that the talin unfolds in the range of 7–15 pN force.75
4. Molecular tension sensors
Using TFM, one can only achieve information on cellular to subcellular level bulk force, which lacks molecular level information. On the other hand, AFM, OT, and MT can be used to study one molecular complex at a time. However, these suffer from limitations based on their low-throughput nature along with their dependence on heavy algorithm-based analysis tools, tedious and costly sample preparation and experiment, and limited compatibility. To address these limitations, molecular tension sensors (MTSs) have emerged.9,11,12,15 The force in the biological system often originates from molecule-to-molecule interactions and accumulates as a system-level force. The overall downstream effect is a cumulative effect. It is worth mentioning that a probe that could sense the force originating at a single-molecular level and also report the total force map would be of great interest. In this section, the emergence, working principles and application of MTSs are comprehensively discussed.
As we have seen, the entire quest for the detection of force revolves around seeing the force when it arises, with the major challenge attributed to its invisible nature. In order to design an MTS, the aim is to convert invisible force into a visible signal, aka an optical signal. An MTS is a molecule with a well-defined structure, which, upon application of external force, ideally in the regime of biological force, will alter its structure. Fluorescent molecules, which can respond to the alteration of this structural change in real time, have become the automatic choice to monitor this force.11,14 The variable response of the fluorescent molecule or the fluorescence signal can occur due to the distance-dependent fluorescence quenching or fluorescence resonance energy transfer (FRET) phenomenon.76 In FRET, a suitable donor and acceptor pair of fluorophores is chosen (FRET pair). The donor is excited by an external light source and the emission from the donor and acceptor is monitored. When the donor and acceptor are present in close vicinity, the energy absorbed by the donor can transfer to the acceptor and the acceptor can then emit fluorescence. The energy transfer during FRET or the FRET efficiency also depends on the spectral overlap between the emission spectrum of the donor and the absorption spectrum of the acceptor. The choice of the FRET pair is very important for understanding the physical phenomenon under investigation. As a result of the energy transfer, the emission probability from the donor is reduced in a distance-dependent manner. The ratio of the emission intensity of the donor and acceptor can give information about their spatial orientation in terms of their distance apart. In the case of fluorescence quenching, a fluorophore and quencher are chosen, whereby the fluorophore can emit fluorescence only when it is far from the quencher. Let us assume that the FRET pair or fluorophore-quencher pairs are tethered to a tension-sensitive molecule in such a way that in the absence of force, the pair will remain in close proximity, but upon the application of force, they will go apart.16,77 If the emission from the fluorophore (fluorescence-quencher pair) or the acceptor (FRET pair) is not seen, then it can be inferred that the force is absent or force is present otherwise. As the FRET or quenching is a very fast phenomenon (a few ns), the response can be seen almost instantaneously.
4.1. Polyethylene glycol-based sensor
Polyethylene glycol (PEG) is a polymer made of sequential hydrophilic and hydrophobic moieties and as a consequence, sufficiently long PEG chains exist, like a spring in water.78 PEG is an attractive molecule in constructing MTSs because it can be chemically modified, and it is biocompatible and stable. The molecular spring can be unwound by applying 0–20 pN external force. In 2012, K. Salaita's lab used PEG as an MTS (Fig. 4A).16 They functionalized PEG molecules with a fluorophore, quencher and epidermal growth factor (EGF) in such a way that when the EGF receptor (EGFR) on the cell surface binds to the EGF and generate forces, the PEG will open, and the fluorophore will be free from the quenching (Fig. 4A). The force can be observed by a conventional fluorescence microscope. The above experiment provided direct evidence that a cell surface receptor, like EGFR, mechanically pulls the ligand. Later the same group devised a gold nanoparticle decorated with a tension-responsive PEG molecule containing the integrin recognition motif cyclo-Arg-Gly-Asp-Phe-Lys (cRGDfk) and a fluorophore.79 They used this probe to visualize the molecular force exerted by the integrin under a fluorescence microscope; whereby, in the presence of force, the fluorophore goes far from the quencher gold nanoparticle.
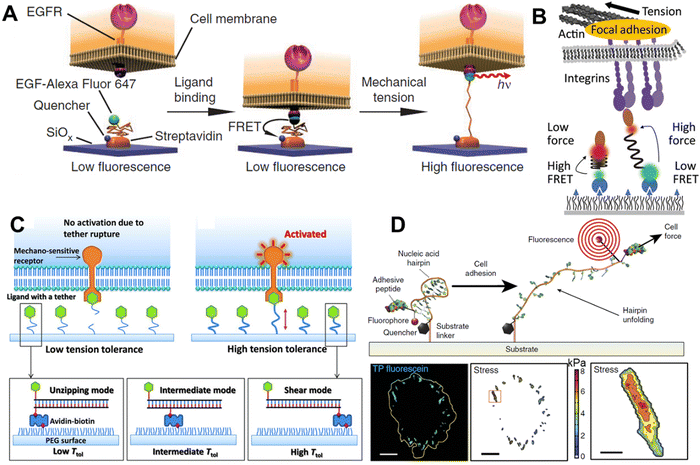 |
| Fig. 4 Molecular tension sensors A. PEG-based molecular tension sensor for imaging EGFR-mediated force; reproduced with permission from ref. 16, Copyright 2011, Springer Nature America, Inc. B. Molecular tension sensor based on spider silk-inspired peptide (GPGGA)8 to image the integrin force; reproduced from ref. 80, Copyright 2016, American Chemical Society. C. Design of tension gauge tethers (TGTs), which can activate receptors at the single-molecule level; reproduced with permission from ref. 17, Copyright 2013, American Association for the Advancement of Science. D. Schematic representation of functionalized hpDNA and the fluorogenic mechanism with the focal adhesion force reported by fluorescence signal; reproduced with permission from ref. 81, Copyright 2014, Springer Nature America, Inc. | |
4.2. Peptide and protein-based sensors
Peptides are also easy to chemically modify, and also possess good stability and biocompatibility. With a suitable amino acid sequence, the peptide can have a physically openable helix or spring-like structure, and hence can be used as a molecular tension probe. Surprisingly, peptide-based tension sensors have been used for reporting the force inside cells before they were employed for extracellular force imaging. In 2008, F. Meng et al. designed a genetically encoded FRET-based tension sensor by coupling a cyan fluorescent protein (CFP) and yellow fluorescent protein (YFP) with an α-helix.82 When transfected into cells, it could not only be expressed successfully but could also sense the strain change in actinin and filamin as imaged using a fluorescence microscope. Later, Grashoff and co-workers used the spider silk-inspired nano-spring peptide sequence GPGGXn (with several pN to as high as 800–900 pN force sensitivity, as comprehensively characterized by the AFM study) as a tension sensor.32 They genetically inserted the CFP-YFP FRET pair coupled with this nano-spring into vinculin, a well-known force-bearing protein in FA. The study revealed that vinculin bears ∼2.5 pN tension in a stable FA structure. Similar to the vinculin tension sensor, M. A. Schwartz's group developed a genetically encoded talin tension sensor and observed that the presence of vinculin increases the load on talin.83 In 2016, A. R. Dunn's research group directly measured the extracellular molecular force produced by integrin by using the previously known nano-spring spider silk peptide GPGGA8 (Fig. 4B).80,84 They equipped the peptide with a FRET pair and the integrin-specific peptide ligand cRGDfk. They found that once the integrin binds to the ligand and physically pulls it, the peptide spring was extended, and the FRET was lost between the donor and acceptor because of the increased distance, hence they could report the force via a visualizable light signal. They found that most of the integrins bear loads of 1–3 pN, while another small population bears higher loads within the FA. Later, Galior et al. designed a titin peptide-based tension sensor with a higher tension sensitivity (>80 pN).85 Here, they used the immunoglobulin 27th (I27) domain of cardiac titin as the force-sensing motif and equipped this with a fluorophore and quencher (gold nanoparticles). In this report, they showed that a small population of integrin can generate a force as high as 80 pN.
4.3. DNA-based molecular tension sensors
4.3.1. Mechanical properties of DNA.
Before going into the detail on the use of DNA as a tension sensing unit, it is necessary to understand and recap the mechanical properties of DNA. DNA is a biopolymer, and two such polymer chains can be held together by H-bonding with a suitable complementary base sequence. The canonical double-stranded DNA (dsDNA) is a thermodynamically stable structure, which can be separated or denatured into random coil single strands (ssDNA) by heating (Fig. 5A) or thermal denaturation. This thermal denaturation is reversible in nature and can be controlled by heating/cooling cycles. Based on the difference in light-absorption ability of the bases in the ssDNA and dsDNA structure, the denaturation or melting temperature (Tm) can be empirically determined by absorption spectroscopy. As the thermodynamic stability of the dsDNA mainly comes from the H-bonding between the bases, Tm depends on the base content, the length of the chain, pH, solvent, ionic strength, etc. It is worth noting that the stability of the DNA duplex of very short DNA is not thermodynamically stable at room temperature, whereby they can just dissociate thermally, and so the predominant population remains as ssDNA (spontaneous dissociation).86 We will see later in this review that the length (15–21 bases) of the dsDNA or the duplex part of the hpDNA is chosen judicially, such that the spontaneous dissociation does not cause an artifact in the results. dsDNA and DNA single strands can be stable in a wide range of pH (5–9) but the stability is compromised at extreme pH.87 At extreme pH, the phosphodiester linkage can be hydrolysed, H-bonding between the bases is impaired, and the purine bases can undergo structural changes (depurination). Along with the pH, the ionic strength has an effect on the duplex stability of the DNA. At lower ionic strength, the association rate of the duplex is slower, and the dissociation rate is faster compared to the same at higher ionic strength.88 To make any DNA-based probe for biological experiment or any other per se, the above pH dependency should be taken into consideration. Also, the conditions should also be considered, not only during the experiment but also during the modification and purification of the probe in terms of the above stability concerns. The relevance of dsDNA in the field of mechanobiology is that dsDNA can be mechanically melted or separated into ssDNA (Fig. 5B). The mechanical properties of DNA with various types of structures have been well studied using single-molecule force spectroscopy techniques such as AFM, OT, and MT. Considering the need for mechanobiology, among the DNA structures studied by force spectroscopy, a few are being used in the mechanobiology field.
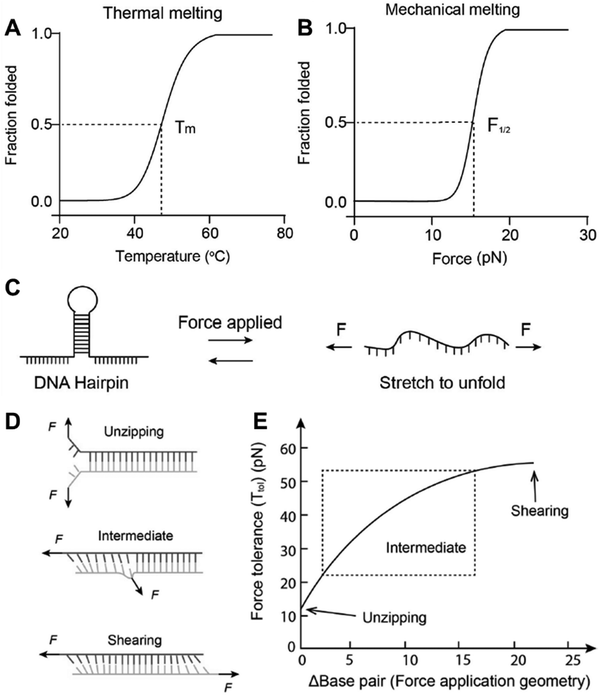 |
| Fig. 5 Thermal (A) vs. mechanical (B) denaturation of dsDNA. (C) Schematic representation of hpDNA under mechanical load (D) Separation of DNA duplexes of different geometries under force. (E) Base pair number-dependent melting curve of different DNA duplexes; reproduced with permission from ref. 9, Copyright 2023, Wiley. | |
The dsDNA motif, which is subject to be separated under tensile force, can come from a single chain, where it folds back and forms the motif known as DNA hairpin (hpDNA, Fig. 5B). As OT-based experimental results suggest, the energy landscape of mechanical separation of hpDNA can be simplified into two minima corresponding to the open and folded form. Although at room temperature the folded state is relatively more stable than the open state, under tensile force the equilibrium starts to shift towards the open state. At a given amount of force (varies with other factors), the probability of being in an open and folded state becomes equal, whereby the force is described as F1/2.89 This external force works against the free energy of hybridization and stretches the nucleotides (favours the folded state). So F1/2 can be mathematically defined as
F1/2 = (ΔGunfold + ΔGstretch)/Δx |
where Δ
Gunfold and Δ
Gstretch are the free energy of unfolding at no force and free energy of stretching of the ssDNA, respectively, and Δ
x is the opening distance. For hpDNA, the experimental value of
F1/2 is ∼12 to 14 pN, while Δ
x is 0.44 ± 0.02 nm per nucleotide plus a correction of 2 nm (effective helix width). As ssDNA is a random polymer without the possibility of hybridization, Δ
Gstretch can be calculated using a worm-like-chain (WLC) model as follows:
where
kb is Boltzmann constant,
T is temperature
Lp and
L0 are the DNA persistent length and ssDNA contour length, respectively, and
x is the extension from the equilibrium position. As hpDNA is made of a single chain, the open and folded states are reversible, hence it can be used as a reversible DNA tension sensor.
81
On the other hand, the two strands of a dsDNA can be two different ssDNA chains, known as a DNA duplex (Fig. 5D). Here the mechanical or thermal separation is irreversible. Two dsDNA with a similar base sequence and length will have the same thermal stability but the mechanical stability will depend on the way the force is applied (Fig. 5D and E). One way is applying the force at the same end of the duplex where two strands are going far from each other, also known as the ‘unzipping’ mode. In this case, the H-bonded bases get separated one by one like a zip. This mode of separation is independent of the DNA length. The required force to rupture a dsDNA in the unzipping mode is ∼12 pN. Another way of separating the dsDNA is by applying force at the different ends of the duplex in an antiparallel manner, or the so-called ‘shearing’ mode. In the shearing mode, the rupture force increases monotonically with the length of the duplex and is saturated around 60–70 pN (Fig. 5E). The de Gennes model prevails in interpreting the rupture force required in the shearing mode. In this model, a finite length of the entire duplex evenly experiences the force characterized as χ−1 and
, where Q is the spring constant of the duplex and R is the spring constant of the H-bonds between the base pair. The rupture force required in the shearing mode can be predicted as follows:
where 2
fc is the force required to rupture one base pair,
L is the number of base pairs,
fc is 3.9 pN, and is
χ−1 6.8 base pair. By rational design of the DNA duplex and the geometry (mixture of both shearing and unzipping modes) of the applied force, the rupture force can be tuned between 12–63 pN.
17,90
4.3.2. DNA-based probes for tension sensing.
As mentioned in the last section that the mechanical melting of DNA is possible and, more interestingly, the required force falls well within the range of molecular tension in the biological regime. Not only the suitability in terms of the tension range but also the tunability of the same, along with the high programmability of DNA structure and rich chemistry of DNA synthesis and modification, made DNA a useful choice to construct molecular mechanosensors.
4.3.2.1. Irreversible probe.
In 2003, C. Albrecht and co-workers reported the use of dsDNA as a programmable force sensor for the first time.91 In tandem with AFM, they studied the mechanical strength of antibody–antigen bonds using a DNA force sensor as the reference. They reported that they could also discriminate between specific and non-specific antibody–antigen interactions. Notice here that the force is not produced by any cellular machinery, but by an AFM cantilever. X. Wang et al. in 2013 demonstrated the use of dsDNA as a tuneable tension sensor, where the force was generated by cellular machinery in live cells.17 Their irreversibly rupturable dsDNA-based probe, namely the tension gauge tether (TGT), was made of 21 base pairs and could be immobilized on the cell culture surface using streptavidin–biotin chemistry (Fig. 4C). Based on the position of the immobilization (at a lower strand) and the ligand (chosen based on the interest of force of the receptor–ligand pair to be studied) attachment site (upper strand), the mechanical separation can be an unzipping or shearing mode or a mixture of both. This provides a rupture force or tension tolerance (Ttol) range between 12–56 pN. Using TGT, they reported that during cell adhesion, a single integrin–ligand bond experiences a universal ∼40 pN force. They also found that the Notch receptor requires less than 12 pN force to be activated. Inspired by the mechanical and basic structural features of TGT, many versions of dsDNA-based force sensors have been developed and explored in the context of investigating force in biological systems. Later using the TGT, the same group found that the single integrin force is more detrimental than the substrate stiffness for cell spreading.92 Fluorescently labelled TGT has been used to study the heterogeneous tension distribution of integrin, and the motility of FA in pairs was observed.93 It was observed that actomyosin-dependent >54 pN tension was generated in the FA and actomyosin-independent ∼40 pN tension was generated in the pre-FA or FA complex formation phase. A covalent ligation-free TGT based on protein G was designed for the universal mechano-sensitive receptor.94 While previously reported fluorescently labelled TGTs work on the fluorescence-loss mechanism, X. Wang's lab designed a fluorophore and quencher-conjugated TGT, namely the integrative tension sensor (ITS), which works in a fluorescence gain or fluorogenic manner.77 In ITS, the lower and upper strands are conjugated with a fluorophore and quencher, respectively. As a force-bearing handle, the integrin ligand cRGDfk is also conjugated with the upper strand. When the dsDNA structure is unruptured the quencher and fluorophore reside in close vicinity, and hence no fluorescence is observed. Once the integrin binds to the ligand and transmits force, the upper strand along with the quencher goes away and now the fluorescence signal comes out of the fluorophore. The use of ITS-based microscopy revealed a polarized integrin tension distribution under a single platelet with a better spatial resolution (∼400 nm) of the force map than that of the other state-of-the-art systems at the time. The same group used ITS to perform real-time force imaging in fast-moving keratocytes and revealed the migration mechanism.95 Leveraging the rupturable property of ITS, a very interesting phenomenon was explored by A. Sarkar et al., whereby non-motile cells were made motile by placing them on a ligand labile surface.96 ITS can store the historical force footprint as the dsDNA rupture is irreversible, and this apparent disadvantage was made useful by Y. Tu et al., whereby they analysed the cell migration parameters, such as range, speed, and persistence without any time-lapse fluorescence imaging.97 As in many cases the population of force generation events is too low to detect by conventional methods, any way of achieving amplification of the mechanical signal is highly desired and is a powerful technique. Y. Duan et al. developed a molecular system that could mechanically trigger a hybridization chain reaction.98 In this system, a hindered cryptic initiator is exposed by the force generated by the cell surface receptor and the chain reaction starts, which can be monitored using a plate reader.
4.3.2.2. Reversible probes.
As discussed in the earlier section, the base-pairing partners in hpDNA come from single ssDNA, hence they are never lost. As a matter of fact, the thermal or mechanical separation is reversible in nature. Researchers have leveraged this and developed hpDNA-based reversible cellular tension sensors. Indeed, hpDNA-based cellular tension sensors were reported independently by C. Chen and K. Salaita's groups at the same time in 2014.81,99 Interestingly both groups used this reversible sensor to study integrin-mediated cellular tensile force at FA. However, the probes they used for the integrin force measurements were structurally different, Chen's group attached the integrin ligand, surface immobilization handle, fluorophore, and quencher to a single DNA strand. Salaita's group used three different strands containing all these components and self-assembled these into a tension probe. Both the molecular tension probes could report the force, but the three-strand-based probe presented a lower synthetic challenge. Salaita's group combined gold nanoparticles (also can work as a quencher) and hpDNA to map the pN level force in the TCR–pMHC complex during T cell activation.100 They found that the T cell generates 12–19 pN tension on the antigen within seconds after binding and starts calcium signalling. The mechanical force or the strength was found to be detrimental in distinguishing specific vs. non-specific antigens and structural reorganization of the TCR and cooperative CD8 binding to reinforce the TCR–pMHC bond. The same group also developed a better-mimicked cellular membrane-like lipid bilayer platform to measure the receptor–ligand tension unlike on rigid glass surfaces that far.101 Using their ratiometric tension sensor on a fluidic surface, they not only measured the TCR–pMHC force but also observed the clustering and lateral transport at the model cell–cell junction. P. Tolar and co-workers used a similar hpDNA probe to measure force during BCR signalling activation in both naïve and germinal centre B cells.102 Their results suggest that the germinal centre B cells produce much stronger tension on the antigen than that of the naïve B cells. The mechanical pulling by Myosin II is believed to be the source of force that produced during BCR-antigen interaction and only high affinity BCR cluster process antigen. In 2017, M. You's lab used an hpDNA-based probe to visualize the intercellular tensile force when the anchoring group is a lipid.103 The same group extended the applicability of the hpDNA probe to image intercellular tensile force using fluorescence lifetime imaging in spheroids, and cell adhesion force via an electrochemical sensor.104–106 Another integrin receptor, namely lymphocyte function-associated antigen 1 (LFA-1), is gaining attention because of its presence on T cells and role in stabilizing the interaction between T cells and APC.9 Using an hpDNA-based tension sensor, V. P. Y. Ma et al. imaged LFA-1 tension.107 Their results suggested that a tension >12 pN by LFA-1 is important for antigen-mediated T cell activation by enhancing its engagement with the substrate. One of the shortcomings in their study was the hpDNA-based reversible tension sensor they used was unable to measure force in a >20 pN regime. Z. Liu's lab designed a reversible shearing DNA-based tension probe (RSDTP) to resolve this problem.108 Using RSDTP, they could measure integrin tension without perturbing the cellular adhesion biology.
4.3.2.3. DNA-based sensors for use in super-resolution microscopy.
Recent technical developments in the field of microscopy have been remarkable, and it is believed that cellular tension imaging could also take advantage of the same advances. Indeed in 2020, X. Wang's group demonstrated single-molecule integrin tension imaging and obtained a force map with an unprecedented 50 nm spatial resolution by cellular force nanoscopy (CFN).109 Using an ITS type of tension probe in tandem with a single-molecule localization microscopy technique, they used CFN for the real-time single-molecule force imaging in fast-moving live cells (Fig. 6A). Later that same year, K. Salaita's group developed a platform for imaging molecular tension at the sub-100 nm resolution. They used an hpDNA-based tension probe and adopted the working principle for DNA point accumulation for imaging in nanoscale topography (DNA-PAINT), and called this tension PAINT (tPAINT).110 Like CFN, tPAINT can also be used for super-resolution cellular tension imaging in live cells (Fig. 6B). The same group developed a technique combining structured illumination microscopy and molecular force microscopy (SIM-MFM) for super-resolution molecular force imaging.111 Using CFN, Wang's group imaged the single-molecule integrin force at sub-micron-sized podosome adhesion ring and cellular extension structured filopodia.112,113 They showed that, unlike FA, the structural maturation of podosomes does not depend on the extracellular integrin–ligand tension, and that filopodia generate nodal integrin force along their length.
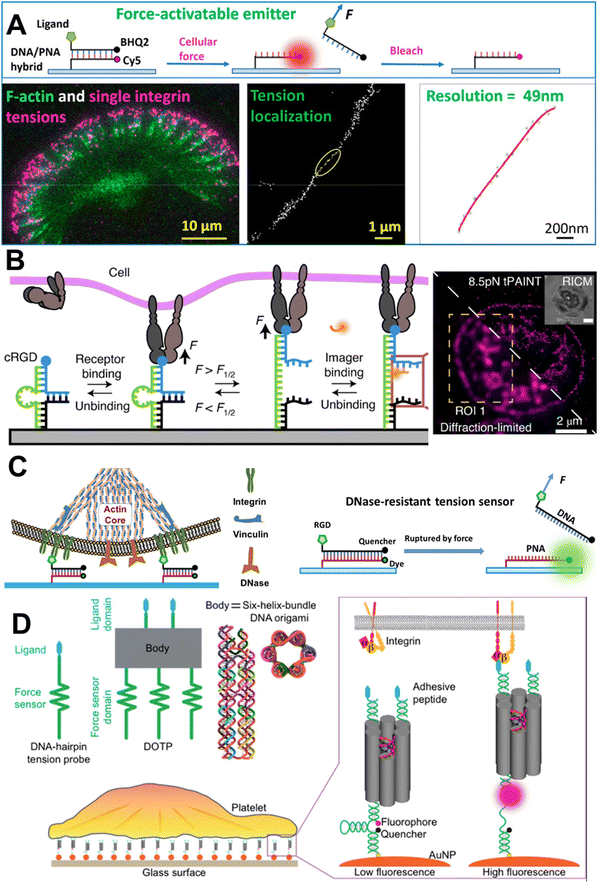 |
| Fig. 6 A. Schematic representation of the mechanism of CFN and single-molecule integrin force imaging under migrating keratocyte; reproduced with permission from ref. 109, Copyright 2020, American Chemical Society. B. Schematic representation of the tPAINT working mechanism and diffraction-unlimited image of integrin force under a platelet; reproduced with permission from ref. 110, Copyright 2020, Springer Nature America, Inc. C. Imaging of integrin force in the podosome adhesion ring using DNase-resistant ITS; reproduced with permission from ref. 112, Copyright 2022, American Chemical Society. D. Imaging of cooperative integrin force with programmable multivalent DNA-origami tension probes; reproduced with permission from ref. 114, Copyright 2018, American Chemical Society. | |
It is worth noting that the rupture force or tension tolerance of a dsDNA/hpDNA probe is not a single-point number. The probability of separating two strands depends on the loading rate or how quickly the force builds up on the probe. With the same amount of force, if the loading rate is different, then the outcome might look different. As observed by Ha's group, the loading rate varied between 0.5 to 4 pN s−1 and in leukocytes it was three times higher than that of epithelial cells.115 They tried to standardize the probe with respect to the kinetics of the force being generated. This was a good complementary study to the existing studies providing data on the physical standardization of DNA-based MTS under mechanical and thermal support.86 For reporting a data set obtained from DNA-based MTS, it should be considered that only the rupture force and probability of spontaneous dissociation do not yet have sufficient information available. Also, the reproducibility of the data can depend on the cell type, the event under investigation, and the GC content of the duplex. So, in the protocol for measuring molecular force using MTS, information about the kinetic nature of the force along with TTol must be considered.
4.4. Beyond canonical double-stranded DNA
While a tension sensor designed based on the basic structural characteristics of TGT is well suited for the study of extracellular receptor–ligand mediated mechanical cues, the lower limit of detection of the TGT is 12 pN. This limitation was addressed by T. Ha's group by developing a ‘nano yoyo’ system with a rupture force of 4 pN.116 The nano yoyo consisted of an E. coli ssDNA binding protein wrapped with a ssDNA, whereby once force is applied, the ssDNA will unwrap. Using this sensor, they concluded that the Notch receptor activation requires a physical force between 4 and 12 pN. One of the main challenges in working with DNA-based tension sensors is the stability of the probe itself; especially, when the regime of sensing is biochemically harsh, like the release of reactive oxygen species (ROS), or where nuclease is possible. One such example is measuring integrin force at the podosome adhesion ring under macrophages. Macrophages can recruit membrane-bound DNase at the podosome site and readily destroy any dsDNA, so the dsDNA-based tension sensor is not suitable therein.117 X. Wang's lab come up with the idea of replacing one of the strands in the duplex with peptide nucleic acid (PNA). PNA contains nucleic bases like ssDNA but the phosphodiester and sugar backbone are replaced with a peptide. The DNA/PNA hybrid duplex showed remarkable stability under a nuclease-abundant environment and could report the integrin force without any artifact (Fig. 6C).112 The same group used the DNase-resistant DNA/PNA hybrid to image integrin tension during the phagocytosis of surface-bound sticky objects by macrophages.118 The study revealed that macrophages form an integrin-mediated adhesion ring, which helps the phagocytosis of objects tightly adhered to the surface. To tackle the probe stability issue under nuclease-abundant circumstances, S. A. Rashid et al. designed a tension sensor where the sensors were covalently tethered to PEG hydrogels and were resistant to nuclease reactions and able to report cell traction force.119 Often biomechanical force is not generated on a planar geometry but in a three-dimensional space during the contact of two entities. To measure such force, Y. Hu et al. designed a microparticle tension sensor (μTS),120 which was made of a micron-sized particle, and the surface was decorated with dsDNA tension sensor molecules, presenting a three-dimensional curved surface when encountering a cell. To measure the low-to-high magnitude of integrin tension simultaneously, A. Sarkar et al. designed a ratiometric tension sensor (RTS).121 The RTS was comprised of two tension sensing dsDNA units with different chain lengths that work in tandem and can report the low-to-high magnitude of integrin force at the same time. As a proof of concept, they revealed the integrin force during platelet adhesion and the role of vinculin in high-level integrin tension generation. P. K. Dutta et al. developed a DNA-origami tension probe (DOTP), which they deployed in a nanoscale organization (Fig. 6D).114 DOTP is a DNA-origami unit with a 6 nm width and tailored number of tension-sensing hpDNA units, which could successfully report the cooperative integrin tension in human platelet adhesion and activation. Later, similar technology was used to study the T cell receptor mechanics at a membrane junction using a more dynamic cell membrane-like substrate.122 Along with the experimental setup that can help researchers understand the generation of molecular forces and their effects, parallel theoretical models have also been developed. A mathematical model revealed that the phosphorylation of FAK-Y397 is regulated by integrin nanoclustering and the substrate stiffness.123
As we have seen, a plethora of strategies have been reported to sense mechanical forces, both out-of-the-cell sensors and genetic sensors, and for working in different force-sensing domains. Sensors can be synthesized with biological materials, like DNA and peptides, and synthetic non-biological molecules, like PEG. MTSs constructed with biological materials are biocompatible, but can be recognized by several enzymes and can be destroyed in the long term, which may cause problems, especially for in vivo applications. On the other hand, MTSs made with non-biological molecules could be enzymatically stable but they themselves could be harmful to the biological system. So, to make a suitable MTS, one should keep the above trade-off in mind. Force detection systems using extracellular molecular sensors work in a wide detection range of 1–100 pN [PEG (1–20 pN), peptides (1–100 pN), hpDNA (7–14 pN), dsDNA (12–54 pN)], while genetically encoded intracellular molecular sensors are also applicable for sensing low [spider silk peptide (1–3 pN)] and high [titin (>80 pN)] range molecular forces. Not only the range of force sensing, but also depending on the experimental conditions, the stability of the probe also needs to be taken into consideration. In particular, DNA-based probes are vulnerable to extreme pH, ionic strength, and the presence of enzymes. Also, if the probe is intended to be used for an in vivo experiment, then its stability needs to be assessed separately from that of one to be used for an in vitro experiment.
5. What has been decoded so far
The last decade has seen remarkable developments and advances in the molecular mechanobiology field, and studies have provided many unprecedented insights. The most studied cellular receptor in mechanobiology is integrin on its ligand cRGDfk. Integrin tension is required for cell adhesion, spreading, and migration. Tension originating from integrin–ligand binding propagates to the cytoskeleton via the initial structural reorganization of integrin and downstream binding of adaptor and docking proteins, like talin, vinculin, paxillin, zyxin, F-actin, Arp2/3, actinin, and myosin. Initially, during the focal complex formation, the integrin force is lower (∼40 pN), but once they cluster together to form FA the tension increases (>56 pN). The maturation of FA depends on the strength of the integrin–ligand bond and the stiffness of the substrate. Vinculin can reinforce the FA complex and help in producing high-level integrin tension. FA can move in pairs based on their location in the cell. The labile integrin ligand surface can convert non-motile cells into motile cells as a result of integrin-mediated rupturing of the ligand from the surface. Cellular extension structured filopodia can sense the microenvironment by producing integrin force nodes, and their behaviour changes in the presence or absence of tension. Integrin force is required for the activation of platelets, which is polarized in individual platelets. Unlike FA, the extracellular integrin–ligand tension at the podosome adhesion ring is dispensable for podosomal maturation. Macrophages use the integrin adhesion force to phagocytose surface-bound objects. Another well-studied pair of receptors and ligands is TCR and pMHC. It was observed that the pN tension generation during TCR–pMHC binding and clustering can initially help T cells to distinguish specific agonist antigens and the structural reorganization of TCR happens. This structural change leads to the cooperative binding of CD8, which consolidates the TCR–pMHC bond and activation of the T cell. Downstream biochemical signalling, overall reorganization of the cytoskeleton, the formation of immune synapses, the cytokine release and/or recruitment of other immune cells all start from the initial molecular tension. Similar observations were made for B cells during BCR antigen binding, where high affinity and BCR clustering are crucial for antigen processing. Another mechanosensitive cell surface receptor is the Notch receptor. As a result of tension generated during the interaction between the Notch receptor and its ligand (DLL), the enzymatic site is exposed, and the cleaved protein then translocates and binds to the transcription factor. It is evident that cell surface receptors similar to integrin, such as TCR, BCR, Notch, and other receptors, like EGFR, and cadherin, can in general exert mechanical tension on their ligands. Accumulating the molecular force cells can produce overall cellular level and tissue level forces during many events, like cancer invasion, and collective cell migration. During cell division in a tissue-like environment, the cell generates force to create the space for mitotic elongation, and if the cell is unable to create the required space because of a lack of force, the mitosis fails.
6. Conclusions and future outlook
In this review, the development of molecular tension sensors have been summarized with a special emphasis on DNA-based fluorogenic tension sensors. A brief discussion on the other mechanobiology techniques, the molecules responsible for mechanosensing and mechanotransduction in the biological system, and the mechanical properties of the DNA were included for ease of understanding. A comprehensive discussion of the obtained result using reversible and irreversible DNA-based tension sensors was presented followed by a discussion of the key findings in the field. The study of cellular- and subcellular-level forces by other mechanobiology technologies, like TFM, suffers from resolution issues. Therefore, there has always been a need for a tension probe that can report molecular-level tension. A study using a pioneering dsDNA-based probe TGT showed the importance and promise of the field, which was then taken to the next level by inserting a FRET or fluorophore-quencher pair into the system. While reversible hpDNA probes can be used to image dynamic low-level molecular force, to record the high-level force irreversible DNA duplex probes are useful. DNA-based molecular tension sensors have been developed to image molecular tension at the single-molecule level and offer diffraction-unlimited resolution.
Evidently, the developments so far in the field of mechanobiology are encouraging but a few challenges remain. With a tension sensor, while researchers can talk about the range of force, or state a given force is beyond a certain threshold, the precise quantification of force is not possible so far. In some cases, like studying immune synapses or phagocytosis, the stability of the molecular tension sensor can be improved. Molecular tension probes, especially DNA-based ones, are primarily used in extracellular and two-dimensional settings. The efficient delivery of DNA-based tension sensors inside the cell and measuring the force there would be a great advancement. On the other hand, incorporating tension sensors in a three-dimensional space and measuring the force would be challenging, yet interesting. So far, the field of mechanobiology has been a field with a focus on diagnosis, but improved tension probes would enable their use in therapeutics (as there is an evident effect of mechanical force on immune cell activation and regulating transcription factors), which could offer remarkable opportunities for the future.
The increasing popularity of the mechanobiology field and the parallel development of new materials and sensing probes raise the question of sustainability, where the health and environmental impact of various kinds of MTSs may be questioned. This question is relevant because some of the MTSs are intended to be used in in vivo experiments. So far, the materials used in the preparation of tension sensors have majorly been of biological origin, like DNA, peptides, or proteins, along with some non-biological polymers, like PEG. The amount of MTS material required for any experiment is very small and given the less-hazardous nature of DNA, peptides, and PEG, they should not induce any environmental threat. Nevertheless, for in vivo experiments, small DNA, peptides, and synthetic non-biological materials can induce immunological or systemic activation of the immune system.124 While using a tension sensor for an in vivo experiment, all these unwanted potential impacts should be considered, especially as they will stay in the system for a longer time and may reach an off-target location. Also, for the large-scale preparation of materials (like InGaN/GaN-based piezo-phototronic materials) for in vitro experiments, the long-term environmental and health impacts should be considered as a matter of concern.
To overcome these challenges, the development of new types of materials and designs of MTSs is required. DNase-resistant sensors (equipped with the ligand of two entities under study) that could be transfected to cells would be a great innovation for studying intracellular mechanical force-generation events. The development of a three-dimensional tissue-like matrix equipped with a force sensor and spiked with a typical cell culture medium would be a useful platform for studying the mechanobiology of many events, like cell–cell interactions, cell–pathogen interactions, cell proliferation, and the pluripotency of stem cells.
Having said this, the author believes that with advanced designs, mathematical modelling, and emerging technologies, more efficient molecular tension sensors will be developed. Also, the mechanobiology field will not remain restricted to in vitro experiments but will be extended to in vivo studies. A detailed spatiotemporal understanding at the molecular level of biological processes will become possible and many new principles of life will surface.
Data availability
No primary research results, software or code has been included and no new data were generated or analysed as part of this review. The reproduced figures are appropriately cited and copyright permission is taken.
Conflicts of interest
There are no conflicts to declare.
Acknowledgements
KP Acknowledges the Department of Chemistry, Indian Institute of Technology (IIT) Tirupati for early career financial and infrastructural support.
References
- D. T. Butcher, T. Alliston and V. M. Weaver, Nat. Rev. Cancer, 2009, 9, 108–122 CrossRef CAS PubMed.
- D. E. Discher, P. Janmey and Y. Wang, Science, 2005, 310, 1139–1143 CrossRef CAS PubMed.
- T. Iskratsch, H. Wolfenson and M. P. Sheetz, Nat. Rev. Mol. Cell Biol., 2014, 15, 825–833 CrossRef CAS.
- K. H. Vining and D. J. Mooney, Nat. Rev. Mol. Cell Biol., 2017, 18, 728–742 CrossRef CAS PubMed.
- D. Mohammed, M. Versaevel, C. Bruyère, L. Alaimo, M. Luciano, E. Vercruysse, A. Procès and S. Gabriele, Front. Bioeng. Biotechnol., 2019, 7, 162 CrossRef PubMed.
- H. De Belly, E. K. Paluch and K. J. Chalut, Nat. Rev. Mol. Cell Biol., 2022, 23, 465–480 CrossRef CAS PubMed.
- A. Saraswathibhatla, D. Indana and O. Chaudhuri, Nat. Rev. Mol. Cell Biol., 2023, 24, 495–516 CrossRef CAS.
- V. Jaumouillé and C. M. Waterman, Front. Immunol., 2020, 11, 1097 CrossRef.
- Y. Hu, Y. Duan and K. Salaita, Angew. Chem., Int. Ed., 2023, 62, e202302967 CrossRef CAS PubMed.
- R. J. Nims, L. Pferdehirt and F. Guilak, Curr. Opin. Biotechnol., 2022, 73, 374–379 CrossRef CAS.
- Y. Huang, T. Chen, X. Chen, X. Chen, J. Zhang, S. Liu, M. Lu, C. Chen, X. Ding, C. Yang, R. Huang and Y. Song, Small, 2024, 20, 2310330 CrossRef CAS.
- C. Yang, R. Xie, T. Cao, Y. Zhang, X. Wang, Y. Xu, Q. Fan and F. Ye, Fundam. Res., 2024 DOI:10.1016/j.fmre.2024.04.008.
-
J. Nakanishi and K. Uto, ed., Material-based mechanobiology, Royal Society of Chemistry, Cambridge, 2022 Search PubMed.
- Q. Tian, P. Keshri and M. You, Chem. Commun., 2022, 58, 4700–4710 RSC.
- Y. Tu and X. Wang, Sensors, 2020, 20, 7128 CrossRef.
- D. R. Stabley, C. Jurchenko, S. S. Marshall and K. S. Salaita, Nat. Methods, 2011, 9, 64–67 CrossRef PubMed.
- X. Wang and T. Ha, Science, 2013, 340, 991–994 CrossRef CAS PubMed.
- J. Sadoshima, Y. Xu, H. S. Slayter and S. Izumo, Cell, 1993, 75, 977–984 CrossRef CAS PubMed.
- A. Woods and J. R. Couchman, Collagen Relat. Res., 1988, 8, 155–182 CrossRef CAS PubMed.
- B. Geiger, J. P. Spatz and A. D. Bershadsky, Nat. Rev. Mol. Cell Biol., 2009, 10, 21–33 CrossRef CAS PubMed.
- D. Kim and D. Wirtz, FASEB J., 2013, 27, 1351–1361 CrossRef CAS PubMed.
- A. J. Ridley, M. A. Schwartz, K. Burridge, R. A. Firtel, M. H. Ginsberg, G. Borisy, J. T. Parsons and A. R. Horwitz, Science, 2003, 302, 1704–1709 CrossRef CAS PubMed.
- K. Burridge, K. Fath, T. Kelly, G. Nuckolls and C. Turner, Annu. Rev. Cell Biol., 1988, 4, 487–525 CrossRef CAS PubMed.
- S. V. Hudson, C. E. Dolin, L. G. Poole, V. L. Massey, D. Wilkey, J. I. Beier, M. L. Merchant, H. B. Frieboes and G. E. Arteel, Sci. Rep., 2017, 7, 12444 CrossRef PubMed.
- Z. Sun, M. Costell and R. Fässler, Nat. Cell Biol., 2019, 21, 25–31 CrossRef CAS PubMed.
- A. Sonnenberg, Curr. Top Microbiol. Immunol., 1993, 184, 7–35 Search PubMed.
- A. Banno and M. H. Ginsberg, Biochem. Soc. Trans., 2008, 36, 229–234 CrossRef CAS PubMed.
- Z. Li, H. Lee and C. Zhu, Exp. Cell Res., 2016, 349, 85–94 CrossRef CAS PubMed.
- J.-L. Guan and D. Shalloway, Nature, 1992, 358, 690–692 CrossRef CAS.
- B. T. Goult, X.-P. Xu, A. R. Gingras, M. Swift, B. Patel, N. Bate, P. M. Kopp, I. L. Barsukov, D. R. Critchley, N. Volkmann and D. Hanein, J. Struct. Biol., 2013, 184, 21–32 Search PubMed.
- A. P. Gilmore and K. Burridge, Nature, 1996, 381, 531–535 CrossRef CAS.
- C. Grashoff, B. D. Hoffman, M. D. Brenner, R. Zhou, M. Parsons, M. T. Yang, M. A. McLean, S. G. Sligar, C. S. Chen, T. Ha and M. A. Schwartz, Nature, 2010, 466, 263–266 Search PubMed.
- J. Austin, Y. Tu, K. Pal and X. Wang, Biophys. J., 2023, 122, 156–167 CrossRef CAS PubMed.
- H. Hirata, H. Tatsumi and M. Sokabe, Commun. Integr. Biol., 2008, 1, 192–195 CrossRef PubMed.
- J. Zhou, C. Aponte-Santamaría, S. Sturm, J. T. Bullerjahn, A. Bronowska and F. Gräter, PLoS Comput. Biol., 2015, 11, e1004593 Search PubMed.
- S. Huveneers and J. De Rooij, J. Cell Sci., 2013, 126, 403–413 CrossRef CAS.
- E. D. Goley, A. Rammohan, E. A. Znameroski, E. N. Firat-Karalar, D. Sept and M. D. Welch, Proc. Natl. Acad. Sci. U. S. A., 2010, 107, 8159–8164 Search PubMed.
- K. Krishnan and P. D. J. Moens, Biophys. Rev., 2009, 1, 71–81 Search PubMed.
-
J. O.-D. La Cruz, F. Martino, S. Pagliari and G. Forte, in Material-based Mechanobiology, ed. J. Nakanishi and K. Uto, The Royal Society of Chemistry, 2022, 21–43 Search PubMed.
- A. S. Yap and E. M. Kovacs, J. Cell Biol., 2003, 160, 11–16 CrossRef CAS PubMed.
- P. Tolar and K. M. Spillane, Adv. Immunol., 2014, 123, 69–100 CAS.
- K. H. Hu and M. J. Butte, J. Cell Biol., 2016, 213, 535–542 CrossRef CAS PubMed.
- C. Zhu, W. Chen, J. Lou, W. Rittase and K. Li, Nat. Immunol., 2019, 20, 1269–1278 Search PubMed.
- H. Nishi, K. Furuhashi, X. Cullere, G. Saggu, M. J. Miller, Y. Chen, F. Rosetti, S. L. Hamilton, L. Yang, S. P. Pittman, J. Liao, J. M. Herter, J. C. Berry, D. J. DeAngelo, C. Zhu, G. C. Tsokos and T. N. Mayadas, J. Clin. Invest., 2017, 127, 3810–3826 CrossRef PubMed.
- A. H. Courtney, W.-L. Lo and A. Weiss, Trends Biochem. Sci., 2018, 43, 108–123 CrossRef CAS PubMed.
- J. B. Huppa and M. M. Davis, Nat. Rev. Immunol., 2003, 3, 973–983 CrossRef CAS.
- B. Minke and B. Cook, Physiol. Rev., 2002, 82, 429–472 CrossRef CAS.
- B. Martinac, J. Adler and C. Kung, Nature, 1990, 348, 261–263 CrossRef CAS PubMed.
- B. Varnum-Finney, L. Wu, M. Yu, C. Brashem-Stein, S. Staats, D. Flowers, J. D. Griffin and I. D. Bernstein, J. Cell Sci., 2000, 23, 4313–4318 CrossRef.
- S. Dupont, L. Morsut, M. Aragona, E. Enzo, S. Giulitti, M. Cordenonsi, F. Zanconato, J. Le Digabel, M. Forcato, S. Bicciato, N. Elvassore and S. Piccolo, Nature, 2011, 474, 179–183 CrossRef CAS.
- J. Möller, T. Lühmann, M. Chabria, H. Hall and V. Vogel, Sci. Rep., 2013, 3, 2884 CrossRef.
- K. K. Sanford, G. D. Likely and W. R. Early, J. Natl. Cancer Inst., 1954, 15, 215–237 CAS.
-
J. Nakanishi and K. Uto, in Material-based Mechanobiology, ed. J. Nakanishi and K. Uto, The Royal Society of Chemistry, 2022, 1–20 Search PubMed.
- Q. Zheng, M. Peng, Z. Liu, S. Li, R. Han, H. Ouyang, Y. Fan, C. Pan, W. Hu, J. Zhai, Z. Li and Z. L. Wang, Sci. Adv., 2021, 7, eabe7738 CrossRef CAS PubMed.
- K. Burton, J. H. Park and D. L. Taylor, Mol. Biol. Cell, 1999, 10, 3745–3769 CrossRef CAS PubMed.
- A. K. Harris, P. Wild and D. Stopak, Science, 1980, 208, 177–179 CrossRef CAS PubMed.
- M. Dembo and Y.-L. Wang, Biophys. J., 1999, 76, 2307–2316 CrossRef CAS PubMed.
- J. L. Tan, J. Tien, D. M. Pirone, D. S. Gray, K. Bhadriraju and C. S. Chen, Proc. Natl. Acad. Sci. U. S. A., 2003, 100, 1484–1489 CrossRef CAS.
- P. Pandey, W. Hawkes, J. Hu, W. V. Megone, J. Gautrot, N. Anilkumar, M. Zhang, L. Hirvonen, S. Cox, E. Ehler, J. Hone, M. Sheetz and T. Iskratsch, Dev. Cell, 2018, 44, 326–336.e3 CrossRef CAS PubMed.
- J. Fu, Y.-K. Wang, M. T. Yang, R. A. Desai, X. Yu, Z. Liu and C. S. Chen, Nat. Methods, 2010, 7, 733–736 CrossRef CAS PubMed.
- B. Sabass, M. L. Gardel, C. M. Waterman and U. S. Schwarz, Biophys. J., 2008, 94, 207–220 CrossRef CAS PubMed.
- H. Colin-York, D. Shrestha, J. H. Felce, D. Waithe, E. Moeendarbary, S. J. Davis, C. Eggeling and M. Fritzsche, Nano Lett., 2016, 16, 2633–2638 CrossRef CAS PubMed.
- S. Nam and O. Chaudhuri, Nat. Phys., 2018, 14, 621–628 Search PubMed.
- J. Chang, A. Saraswathibhatla, Z. Song, S. Varma, C. Sanchez, N. H. K. Alyafei, D. Indana, R. Slyman, S. Srivastava, K. Liu, M. C. Bassik, M. P. Marinkovich, L. Hodgson, V. Shenoy, R. B. West and O. Chaudhuri, Nat. Mater., 2024, 23, 711–722 CrossRef CAS.
- R. Basu, B. M. Whitlock, J. Husson, A. Le Floc’h, W. Jin, A. Oyler-Yaniv, F. Dotiwala, G. Giannone, C. Hivroz, N. Biais, J. Lieberman, L. C. Kam and M. Huse, Cell, 2016, 165, 100–110 CrossRef CAS PubMed.
- E. Evans, K. Ritchie and R. Merkel, Biophys. J., 1995, 68, 2580–2587 CrossRef CAS PubMed.
- G. Binnig, C. F. Quate and C. Gerber, Phys. Rev. Lett., 1986, 56, 930–933 CrossRef PubMed.
- M. Radmacher, R. W. Tillamnn, M. Fritz and H. E. Gaub, Science, 1992, 257, 1900–1905 CrossRef CAS PubMed.
- G. R. Heath, E. Kots, J. L. Robertson, S. Lansky, G. Khelashvili, H. Weinstein and S. Scheuring, Nature, 2021, 594, 385–390 CrossRef CAS.
- A. Ashkin, Biophys. J., 1992, 61, 569–582 CrossRef CAS.
- Y. Feng, K. N. Brazin, E. Kobayashi, R. J. Mallis, E. L. Reinherz and M. J. Lang, Proc. Natl. Acad. Sci. U. S. A., 2017, 114, E8204–E8213 CAS.
- A. K. Efremov, M. Yao, Y. Sun, Y. H. Tee, M. P. Sheetz, A. D. Bershadsky, B. Martinac and J. Yan, Biomaterials, 2022, 284, 121477 CrossRef CAS PubMed.
- C. Gosse and V. Croquette, Biophys. J., 2002, 82, 3314–3329 CrossRef CAS PubMed.
- R. Tapia-Rojo, M. Mora and S. Garcia-Manyes, Nat. Protoc., 2024, 19, 1779–1806 CrossRef CAS.
- S. Chakraborty, D. Chaudhuri, S. Banerjee, M. Bhatt and S. Haldar, Commun. Biol., 2022, 5, 307 CrossRef CAS PubMed.
-
J. R. Lakowicz, Principles of fluorescence spectroscopy, Springer, New York, NY, 3rd edn, 2010 Search PubMed.
- Y. Wang, D. N. LeVine, M. Gannon, Y. Zhao, A. Sarkar, B. Hoch and X. Wang, Biosens. Bioelectron., 2018, 100, 192–200 CrossRef CAS PubMed.
- F. Oesterhelt, M. Rief and H. E. Gaub, New J. Phys., 1999, 1, 6 CrossRef.
- Y. Liu, R. Medda, Z. Liu, K. Galior, K. Yehl, J. P. Spatz, E. A. Cavalcanti-Adam and K. Salaita, Nano Lett., 2014, 14, 5539–5546 CrossRef CAS PubMed.
- A. C. Chang, A. H. Mekhdjian, M. Morimatsu, A. K. Denisin, B. L. Pruitt and A. R. Dunn, ACS Nano, 2016, 10, 10745–10752 CrossRef CAS.
- B. L. Blakely, C. E. Dumelin, B. Trappmann, L. M. McGregor, C. K. Choi, P. C. Anthony, V. K. Duesterberg, B. M. Baker, S. M. Block, D. R. Liu and C. S. Chen, Nat. Methods, 2014, 11, 1229–1232 CrossRef CAS.
- F. Meng, T. M. Suchyna and F. Sachs, FEBS J., 2008, 275, 3072–3087 CrossRef CAS PubMed.
- A. Kumar, M. Ouyang, K. Van Den Dries, E. J. McGhee, K. Tanaka, M. D. Anderson, A. Groisman, B. T. Goult, K. I. Anderson and M. A. Schwartz, J. Cell Biol., 2016, 213, 371–383 CrossRef CAS.
- M. Morimatsu, A. H. Mekhdjian, A. S. Adhikari and A. R. Dunn, Nano Lett., 2013, 13, 3985–3989 CrossRef CAS.
- K. Galior, Y. Liu, K. Yehl, S. Vivek and K. Salaita, Nano Lett., 2016, 16, 341–348 CrossRef CAS.
- S. Cocco, R. Monasson and J. F. Marko, Proc. Natl. Acad. Sci. U. S. A., 2001, 98, 8608–8613 CrossRef CAS PubMed.
-
T. E. Creighton, The biophysical chemistry of nucleic acids, Helvetian Press, Eastbourne, 2011 Search PubMed.
- N. F. Dupuis, E. D. Holmstrom and D. J. Nesbitt, Biophys. J., 2013, 105, 756–766 CrossRef CAS PubMed.
- M. T. Woodside, W. M. Behnke-Parks, K. Larizadeh, K. Travers, D. Herschlag and S. M. Block, Proc. Natl. Acad. Sci. U. S. A., 2006, 103, 6190–6195 CrossRef CAS.
- K. Hatch, C. Danilowicz, V. Coljee and M. Prentiss, Phys. Rev. E: Stat., Nonlinear, Soft Matter Phys., 2008, 78, 011920 CrossRef CAS PubMed.
- C. Albrecht, K. Blank, M. Lalic-Mülthaler, S. Hirler, T. Mai, I. Gilbert, S. Schiffmann, T. Bayer, H. Clausen-Schaumann and H. E. Gaub, Science, 2003, 301, 367–370 CrossRef CAS PubMed.
- F. Chowdhury, I. T. S. Li, B. J. Leslie, S. Doğanay, R. Singh, X. Wang, J. Seong, S.-H. Lee, S. Park, N. Wang and T. Ha, Integr. Biol., 2015, 7, 1265–1271 CrossRef CAS.
- X. Wang, J. Sun, Q. Xu, F. Chowdhury, M. Roein-Peikar, Y. Wang and T. Ha, Biophys. J., 2015, 109, 2259–2267 CrossRef CAS PubMed.
- X. Wang, Z. Rahil, I. T. S. Li, F. Chowdhury, D. E. Leckband, Y. R. Chemla and T. Ha, Sci. Rep., 2016, 6, 21584 CrossRef CAS PubMed.
- Y. Zhao, Y. Wang, A. Sarkar and X. Wang, iScience, 2018, 9, 502–512 CrossRef CAS PubMed.
- A. Sarkar, D. N. LeVine, N. Kuzmina, Y. Zhao and X. Wang, Curr. Biol., 2020, 30, 4022–4032.e5 CrossRef CAS PubMed.
- Y. Tu and X. Wang, Biosens. Bioelectron., 2021, 193, 113533 CrossRef CAS.
- Y. Duan, R. Glazier, A. Bazrafshan, Y. Hu, S. A. Rashid, B. G. Petrich, Y. Ke and K. Salaita, Angew. Chem., Int. Ed., 2021, 60, 19974–19981 CrossRef CAS PubMed.
- Y. Zhang, C. Ge, C. Zhu and K. Salaita, Nat. Commun., 2014, 5, 5167 CrossRef CAS PubMed.
- Y. Liu, L. Blanchfield, V. P.-Y. Ma, R. Andargachew, K. Galior, Z. Liu, B. Evavold and K. Salaita, Proc. Natl. Acad. Sci. U. S. A., 2016, 113, 5610–5615 CrossRef CAS PubMed.
- V. P.-Y. Ma, Y. Liu, L. Blanchfield, H. Su, B. D. Evavold and K. Salaita, Nano Lett., 2016, 16, 4552–4559 CrossRef CAS.
- C. R. Nowosad, K. M. Spillane and P. Tolar, Nat. Immunol., 2016, 17, 870–877 CrossRef CAS PubMed.
- B. Zhao, C. O’Brien, A. P. K. K. K. Mudiyanselage, N. Li, Y. Bagheri, R. Wu, Y. Sun and M. You, J. Am. Chem. Soc., 2017, 139, 18182–18185 CrossRef CAS.
- P. Keshri, B. Zhao, T. Xie, Y. Bagheri, J. Chambers, Y. Sun and M. You, Angew. Chem., Int. Ed., 2021, 60, 15548–15555 CrossRef CAS PubMed.
- Q. Tian, F. Yang, H. Jiang, P. Bhattacharyya, T. Xie, A. A. Ali, Y. Sun and M. You, Front. Cell Dev. Biol., 2023, 11, 1220079 CrossRef PubMed.
- M. Amouzadeh Tabrizi, P. Bhattacharyya, R. Zheng and M. You, Biosens. Bioelectron., 2024, 253, 116185 CrossRef CAS PubMed.
- V. P.-Y. Ma, Y. Hu, A. V. Kellner, J. M. Brockman, A. Velusamy, A. T. Blanchard, B. D. Evavold, R. Alon and K. Salaita, Sci. Adv., 2022, 8, eabg4485 CrossRef CAS.
- H. Li, C. Zhang, Y. Hu, P. Liu, F. Sun, W. Chen, X. Zhang, J. Ma, W. Wang, L. Wang, P. Wu and Z. Liu, Nat. Cell Biol., 2021, 23, 642–651 CrossRef CAS.
- Y. Zhao, K. Pal, Y. Tu and X. Wang, J. Am. Chem. Soc., 2020, 142, 6930–6934 CrossRef CAS.
- J. M. Brockman, H. Su, A. T. Blanchard, Y. Duan, T. Meyer, M. E. Quach, R. Glazier, A. Bazrafshan, R. L. Bender, A. V. Kellner, H. Ogasawara, R. Ma, F. Schueder, B. G. Petrich, R. Jungmann, R. Li, A. L. Mattheyses, Y. Ke and K. Salaita, Nat. Methods, 2020, 17, 1018–1024 CrossRef CAS PubMed.
- A. Blanchard, J. D. Combs, J. M. Brockman, A. V. Kellner, R. Glazier, H. Su, R. L. Bender, A. S. Bazrafshan, W. Chen, M. E. Quach, R. Li, A. L. Mattheyses and K. Salaita, Nat. Commun., 2021, 12, 4693 CrossRef CAS PubMed.
- K. Pal, Y. Tu and X. Wang, ACS Nano, 2022, 16, 2481–2493 CrossRef CAS PubMed.
- Y. Tu, K. Pal, J. Austin and X. Wang, Curr. Biol., 2022, 32, 4386–4396.e3 CrossRef CAS PubMed.
- P. K. Dutta, Y. Zhang, A. T. Blanchard, C. Ge, M. Rushdi, K. Weiss, C. Zhu, Y. Ke and K. Salaita, Nano Lett., 2018, 18, 4803–4811 CrossRef CAS PubMed.
- M. H. Jo, P. Meneses, O. Yang, C. C. Carcamo, S. Pangeni and T. Ha, Science, 2024, 383, 1374–1379 CrossRef CAS.
- F. Chowdhury, I. T. S. Li, T. T. M. Ngo, B. J. Leslie, B. C. Kim, J. E. Sokoloski, E. Weiland, X. Wang, Y. R. Chemla, T. M. Lohman and T. Ha, Nano Lett., 2016, 16, 3892–3897 CrossRef CAS PubMed.
- K. Pal, Y. Zhao, Y. Wang and X. Wang, J. Cell Biol., 2021, 220, e202008079 CrossRef CAS PubMed.
-
K. Pal, S. Kundu and X. Wang, bioRxiv, preprint, 2023 DOI:10.1101/2023.08.01.551462.
- S. A. Rashid, Y. Dong, H. Ogasawara, M. Vierengel, M. E. Essien and K. Salaita, ACS Appl. Mater. Interfaces, 2023, 15, 33362–33372 CrossRef CAS PubMed.
- Y. Hu, V. P. Ma, R. Ma, W. Chen, Y. Duan, R. Glazier, B. G. Petrich, R. Li and K. Salaita, Angew. Chem., Int. Ed., 2021, 60, 18044–18050 CrossRef CAS.
- A. Sarkar, G. Niraula, D. LeVine, Y. Zhao, Y. Tu, K. Mollaeian, J. Ren, L. Que and X. Wang, ACS Sens., 2023, 8, 3701–3712 CrossRef CAS PubMed.
- Y. Hu, J. Rogers, Y. Duan, A. Velusamy, S. Narum, S. Al Abdullatif and K. Salaita, Nat. Nanotechnol., 2024, 19, 1674–1685 CrossRef CAS PubMed.
- B. Cheng, W. Wan, G. Huang, Y. Li, G. M. Genin, M. R. K. Mofrad, T. J. Lu, F. Xu and M. Lin, Sci. Adv., 2020, 6, eaax1909 CrossRef CAS PubMed.
- H. Lou and M. C. Pickering, Cell. Mol. Immunol., 2018, 15, 746–755 CrossRef CAS.
|
This journal is © The Royal Society of Chemistry 2025 |
Click here to see how this site uses Cookies. View our privacy policy here.