DOI:
10.1039/B712330B
(Paper)
Lab Chip, 2008,
8, 134-142
Cell culture arrays using magnetic force-based cell patterning for dynamic single cell analysis
Received
10th August 2007
, Accepted 11th October 2007
First published on 25th October 2007
Abstract
In order to understand the behavior of individual cells, single cell analyses have attracted attention since most cell-based assays provide data with values averaged across a large number of cells. Techniques for the manipulation and analysis of single cells are crucial for understanding the behavior of individual cells. In the present study, we have developed single cell culture arrays using magnetic force and a pin holder, which enables the allocation of the magnetically labeled cells on arrays, and have analyzed their dynamics. The pin holder was made from magnetic soft iron and contained more than 6000 pillars on its surface. The pin holder was placed on a magnet to concentrate the magnetic flux density above the pillars. NIH/3T3 fibroblasts that were labeled with magnetite cationic liposomes (MCLs) were seeded into a culture dish, and the dish was placed over the pin holder with the magnet. The magnetically labeled cells were guided on the surface where the pillars were positioned and allocated on the arrays with a high resolution. Single-cell patterning was achieved by adjusting the number of cells seeded, and the target cell was collected by a micromanipulator after removing the pin holder with the magnet. Furthermore, change in the morphology of magnetically patterned cells was analyzed by microscopic observation, and cell spreading on the array was observed with time duration. Magnetic force-based cell patterning on cell culture arrays would be a suitable technique for the analysis of cell behavior in studies of cell–cell variation and cell–cell interactions.
Introduction
Most cell-based biological assays yield data averaged across large groups of cells; yet it is well known that individual cells, even those that are identical in appearance, differ in numerous characteristics.1 Single-cell measurements often display significant heterogeneity since cellular processes influenced by noise include ion-channel gating, cytoskeleton dynamics, motors-driven transport, intracellular networks, and so on.1–5 Due to this heterogeneity, traditional biochemical assays, which analyze cells in bulk, often overlook the rich information that can be obtained when single cells are studied. In gene expression studies, single-cell polymerase chain reaction (PCR) has made it possible to identify whether two or more genes are coexpressed in the same cell or in different subpopulations of cells.6,7 Single-cell measurements are also important in studying mixed cell populations. In studies of disease states, the analysis of a sample obtained directly from a model organism or patient is complicated by the admixture of normal cells with diseased cells. Single-cell studies of tumor biopsies have shown that the majority of cells within a tumor may be normal and that significant heterogeneity exists even among the abnormal cells.6,8 Thus, single cell analysis has attracted considerable attention.
Flow cytometry is a common and well-established method for analyzing hundreds of thousands of individual cells. This technique uses one or more focused laser beams directed at a glass capillary tube through which a high-speed stream of cells in suspension flow in single file.9 This procedure is often performed using fluorescent probes that act as indicators of cellular constituents or functions. This technique is a high-throughput method for single cell analysis, but lacks the capability for studying cell dynamics and secretions. Therefore, methods that can measure cell dynamics and analyze directly the biological responses of individual cells are desired. Another conventional method for the analysis of individual cells in large numbers involves the use of multiwell plates (i.e., 384- or 96-well plates); however, they are operated by using cumbersome manual or expensive robotics-based operations.10–12 The advent of microengineering has provided biologists with unprecedented opportunities for cell handling and investigation on a cell-to-cell basis.13 Microengineering techniques such as lab-on-a-chip technologies are emerging as the next revolution in tools for single cell analysis for the isolation and rapid analysis of single cells. Recently, cell patterning methods have been developed for single cell culture arrays.13 In general, substrates are micropatterned by using extracellular matrix (ECM), peptides, and polymers in order to control cell attachments on the substrates used for cell patterning.13–19 These methods might not be suitable for the diagnosis and analysis of cell dynamics since cell shapes are restricted to the micropatterned substrates, and induce several types of behaviors such as apoptosis.20 For example, when square patterned substrates are used for single cell analysis, the individual cell shape changes to square, and we can not observe native cell shape. Other methods have also been demonstrated for the fabrication of single cell arrays without the chemical treatment of substrates. In perfused microfluidic systems that can trap single cells on fabricated platforms, large numbers of single cells could be easily studied to yield insight into population dynamics.21 The systems can provide uniform conditions for individual cells with controllable cell–cell interactions, including diffusible and contact elements. Moreover, microwells on the substrate have also been used for the fabrication of cell arrays.22–24 These methods were improved for high-throughput assays that are applicable to drug screening and tissue engineering. The cells remained in place during a variety of wash and cell-loading steps so that metabolism of a fluorescent dye could be measured over time, and the cells could be fixed within the microwells to enable assays such as antibody-based staining of intracellular antigens. However, the structures may cause some changes of cell dynamics. Therefore, developing a technology that can perform single cell assays for the analysis of cell dynamics in an easier high-throughput manner would be beneficial in bioengineering fields utilizing cell assays that range from drug discovery to tissue engineering.
In the present study, we proposed a technique for the construction of a single cell culture array of adherent cell lines without chemically treating the array surface. Magnetite cationic liposomes (MCLs) are cationic liposomes containing 10 nm magnetite nanoparticles that have been developed for introducing magnetite nanoparticles into target cells based on the electrostatic interactions between MCLs and the cell membrane.25Cells labeled with MCLs can be easily manipulated by magnetic force. We propose an original tissue engineering methodology using magnetically labeled cells and magnetic force and have designated this novel methodology as “magnetic force-based tissue engineering (Mag-TE).”26–30 Previously, we applied the Mag-TE technique for the construction of sheet-like structures comprising three-dimensional multilayered cells and tubular structures such as vascular and urinary tissues, respectively, and developed a magnetic force-based cell patterning methodology by using the Mag-TE technique to fabricate capillary-like structures in a line.26,28 In this study, we made a pin holder that contains more than 6000 pillars (100 µm × 100 µm × 300 µm) from magnetic soft iron; this device and the Mag-TE technique were applied to the patterning of cells on arrays. This device could be operated easily, and single cells were allocated on the arrays in less than 15 s. Furthermore, dynamic cell behaviors were observed under a microscope and the distribution of these cell behaviors was examined by imaging. We believe that magnetic force-based cell patterning is a useful tool for the fabrication of single cell arrays and for the analysis of cell dynamics.
Materials and methods
Fabrication of pin holder device
A wire electrical discharge machine (wire-EDM; FX10; Mitsubishi, Tokyo, Japan) was employed for the fabrication of a pin holder device. A cut wire of 100 µm diameter was used (Sumiden Fine Conductors Co. Ltd., Osaka, Japan). The wire-EDM used electrothermal mechanisms to cut the electrically conductive material.31 Magnetic soft iron (Mashinax, Aichi, Japan) was removed by a series of discrete discharges between the wire electrode and the workpiece in the presence of a dielectric fluid, which creates a path for each discharge as the fluid becomes ionized in the gap. The region in which the discharge occurs was heated to extremely high temperatures in order to melt and remove the work surface. The width, length, and thickness of the device were 20, 20, and 10 mm, respectively, and the surface was microfabricated by using the wire-EDM to construct more than 6000 square pillars with the dimensions 100 µm × 100 µm.
Model of magnetic flux density on the device
The magnetic flux density on the culture surface was calculated using a magnetic simulation software (Super Moment; the software is available at http://www.vector.co.jp/soft/win95/edu/se078148.html). To simplify the simulations a block neodymium magnet (50 mm × 50 mm × 10 mm) and a pin holder device (20 mm × 20 mm × 10 mm) that has pillars (100 µm × 100 µm × 300 µm) by a distance of 150 µm on the surface were used. The device was positioned on the center of the magnet, and the magnetic flux density at 50, 100 and 150 µm above the device was calculated.
Preparation of magnetite cationic liposomes
Magnetite (Fe3O4; average particle size, 10 nm; Toda Kogyo, Hiroshima, Japan) was used as the core of the MCLs. The MCLs were prepared using colloidal magnetite and a lipid mixture of N-(α-trimethylammonioacetyl)-didodecyl-D-glutamate chloride (TMAG, a cationic lipid), dilauroylphosphatidylcholine (DLPC), and dioleoylphosphatidylethanolamine (DOPE) in a molar ratio of 1 : 2 : 2, as described previously.24 The lipid mixture was dried down by evaporation for a minimum of 30 min to form lipid films. These films were hydrated by vortexing in colloidal magnetite nanoparticles to form liposomes, and the liposomes were sonicated for 30 min. Magnetite concentrations were measured by using the potassium thiocyanate method.32
Cell culture and magnetic labeling
Mouse NIH/3T3 fibroblasts were obtained from the American Type Culture Collection (ATCC; Manassas, VA). The 3T3 cells were cultured at 37 °C under a humidified atmosphere of 5% CO2 and 95% air in Dulbecco's Modified Eagle's Medium (DMEM) supplemented with 10% fetal bovine serum (FBS), 0.1 mg ml–1streptomycin sulfate, and 100 U ml–1potassium penicillin G.
For magnetic labeling, the cells were cultured until subconfluent and the medium was replaced with a fresh medium that contained MCLs (net magnetite concentration, 100 pg cell–1). After culturing with MCLs for 4 h, the magnetically labeled cells were collected with 0.25% trypsin EDTA (Gibco, Carlsbad, CA).
Cell
patterning on arrays using magnetic force
The pin holder device was placed on a cylindrical neodymium magnet (diameter, 50 mm; height, 10 mm; magnetic flux density, 0.38 T). In order to investigate the effect of the thickness of the cell culture dish on cell patterning, magnetically labeled 3T3 cells (50 cells mm–2) were seeded into a glass-based culture dish that consisted of a silicon frame with a micro glass plate of thickness 50, 100, or 150 µm (Matsunami Glass Ind., Ltd., Osaka, Japan). In order to fabricate the culture dish, a silicon sheet (thickness. 5 mm) was hollowed out, and square window formed in the silicon sheet (long, 10 mm; wide, 10 mm), and then the silicon sheet was mounted on these glass plates. The culture dishes were placed on the magnetized pin holder device (Fig. 1A). The cells were cultured for 10 min and then observed by using a phase microscope (Olympus, Tokyo, Japan). During culture, the cells were attracted onto the surfaces above the pillars, and spots with cells (or blanks) appeared on the surfaces. In order to acquire the time-course images of cell patterning on the arrays, the cells (8.0 × 102cells mm–2) were seeded into the dish that was placed on the magnetized pin holder device, and observed by using a stereomicroscope (Keyence Co., Osaka, Japan). In order to fabricate single cell culture arrays, the concentration of the seeding cells was varied from 10 cells mm–2 (0.7 cells spot–1) to 88 cells mm–2 (5.5 cells spot–1). After culturing for 30 min, images were obtained by a phase microscope and the cells in individual spots were counted. In order to collect a target cell that was allocated on the arrays, the magnetized pin holder device was removed from the culture dishes, and we collected the target cell using a micromanipulator (Eppendorf, Humberg, Germany).
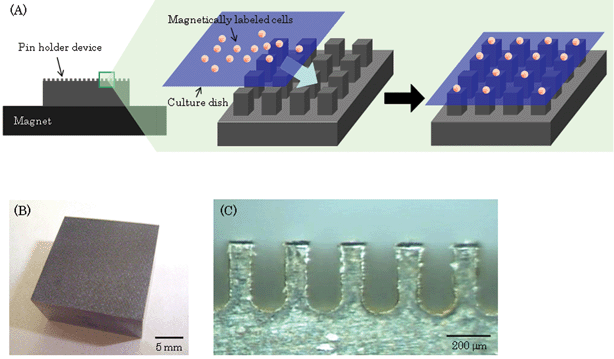 |
| Fig. 1 Fabrication of cell culture arrays using magnetic force. (A) Scheme of patterning cells on arrays. The pin holder device was placed on a cylindrical neodymium magnet to magnetize it. The magnetically labeled cells were seeded into a culture dish that was then placed on the magnetized device. The cells were attracted to the culture surface where the pillars were positioned. Thus, the cells were allocated on the arrays. (B) A photograph of a magnetic soft iron device microfabricated by a wire-EDM is shown (width, 20 mm; length, 20 mm; height, 10 mm). (C) The device contains more than 6000 square pillars (width, 100 µm; length, 100 µm; height, 300 µm) that are separated by a distance of 150 µm on the surface. | |
Image analysis
In order to evaluate morphological changes in the cells, image analysis was performed. The 3T3 cells were stained with CellTracker Green (CMFDA, 5-chloromethylfluorescein diacetate; Molecular Probes, Eugene, OR) and then magnetically labeled as mentioned above. The labeled cells were seeded into a 35 mm culture dish (hydrophilic lumox dish; Greiner Bio-One, Frickenhausen, Germany), which consisted of a polystyrene frame with an ultra-thin film (25 µm), and were allocated on arrays as described above. The film is made of a resin film, and mammalian cells can adhere and spread on the film. Fluorescence images were acquired by using a fluorescence microscope (Olympus) after culturing for 0, 1, 3, and 5 h. The images were used for analyzing the morphology of individual cells using the following procedures. First, the images were clipped in order to observe only 1 spot with cells (or blanks); next, the images showing spots with a single cell were selected (the number of images was 60–130 in 1 experiment at each time point); finally, the area, shape factor, and elliptical form factor of the individual cells were automatically measured by using an image analysis software (MetaMorph software; Universal Imaging Co., Downingtown, PA). The shape factor reveals how closely the cell represents a circle (circle = 1) and is given by the area (A) and perimeter (P) of the individual cells:
The elliptical form factor is the ratio of the short axis (breadth) to the long axis of the individual cells.
In order to investigate the dynamics of single cells treated with the DNA damaging agent mitomycin C (MMC; Nacalai Tesque Co., Kyoto, Japan), the 3T3 cells stained with CellTracker Green were magnetically labeled with MCLs and then treated with MMC (1, 10 µg ml–1) for 14 h. The cells were then allocated, and their morphology was analyzed as above.
Results and discussion
Fabrication of pin holder device
Magnetic soft iron was used for constructing the pin holder device since it is a magnetizable material and can be easily microfabricated. The magnetic susceptibility of this material is higher than that of other magnetizable materials such as steel. The magnetic susceptibility is the degree of magnetization of a material in response to an applied magnetic field. A magnetic flux density on magnetic soft iron with the magnet is stronger than one on iron with the magnet due to the magnetic susceptibility of the magnetic soft iron. Therefore, in this study, we used magnetic soft iron for fabricating single cell arrays using magnetic force. Magnetic soft iron was microfabricated by using wire-EDM. The device consisted of more than 6000 pillars on the surface; the length, width, and height of the pillars were 100, 100, and 300 µm, respectively, and were separated by a distance of 150 µm (Fig. 1B, C). The device was placed on a magnet in order to control and strengthen the magnetic flux density above the pillars.
Model of magnetic flux density on the device
The magnetic flux density on the culture surface was calculated using a magnetic simulation software (Fig. 2). When a culture dish with a thickness of 150 µm was placed on the pin holder device, the magnetic flux density on the culture surface was almost flat (Fig. 2A). On the other hand, when we used a culture dish with a thickness of 50 µm, the difference between the top and the bottom magnetic flux densities was approximately 0.2 T (Fig. 2C). These results indicate that the distance between the pin holder device and the culture surface is very important for allocating magnetically labeled cells on arrays. Magnetic force-based cell patterning was demonstrated using 3 types of culture dishes that were 50, 100, and 150 µm thick (Fig. 3). The magnetically labeled 3T3 cells were seeded into the culture dishes with different thicknesses, and the dishes were placed on the pin holder device with the magnet. Although the cells were not allocated completely on the arrays when using the culture dish with a thickness of 150 µm, magnetic cell patterning on the array was successfully achieved with the culture dish of 50 µm thickness (Fig. 3). The simulation results showed that the magnetic flux density above the center of the pillars was higher than that in other regions, and the experimental data reveal that the cell was allocated above the center of the pillar (Fig. 3D). The magnetic force acting on the magnetic particles depends on the intensity of magnetization of the particles, and the magnetization also depends on the magnetic flux density. Therefore, in general, the magnetic force depends on the magnetic flux density. Furthermore, the force vector directs to high magnetic flux density from low magnetic density. Therefore, the cells travel from positions of low magnetic flux density to high ones so that the cells can be attracted to the high magnetic flux density. The difference between top and bottom magnetic flux density is important for magnetic force-based cell patterning. The difference exponentially reduced as the distance between the device and the culture surface increase in length (unpublished data), and a slight difference in the distance significantly affected cell patterning. When the distance from the device was long, the cells went down vertically to the culture surface since the difference was low. On the other hand, when the distance from device was short (<100 µm), the cells were rapidly attracted to the center of the pillar since the magnetic flux density above the center of the pillars was higher than that in other regions (Fig. 2, 3). Thus, the cells traveled at the center of the pillar when the cells came near the device. The magnetic force depends on the amount of MCLs, and the amount affects cell patterning.
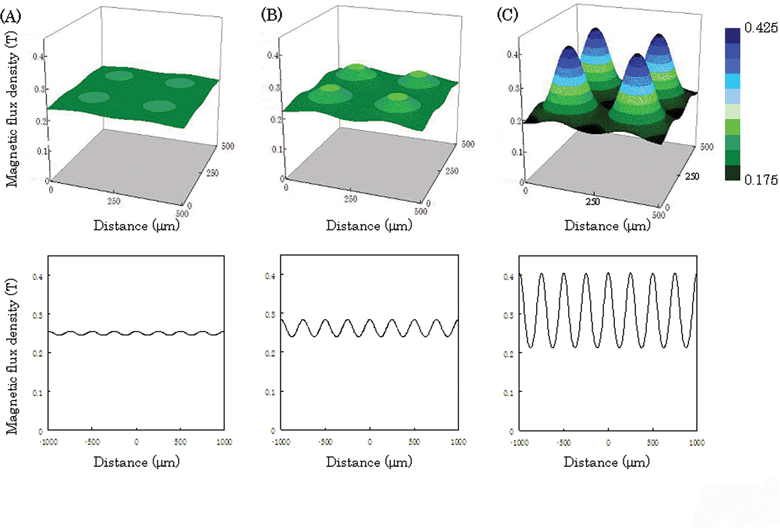 |
| Fig. 2 Magnetic flux density on the culture surface in simulations. The top- and bottom-view images show 3D and cross-section magnetic flux density at the center of the dish, respectively. The magnetic flux density was calculated for culture dishes with thicknesses of 150 (A), 100 (B), and 50 µm (C). The pillars (100 µm × 100 µm) were placed at 150 µm intervals. | |
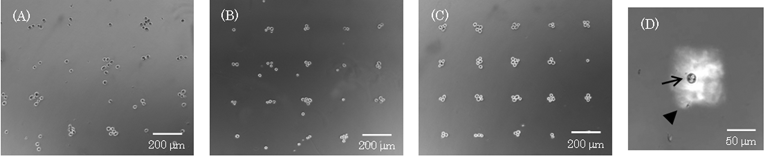 |
| Fig. 3
Cell culture array fabricated using magnetic force. The phase microscopic images of cells on arrays using culture dishes of thickness 150 µm (A), 100 µm (B), and 50 µm are shown. The images were observed after placing the dish on the magnetized pin holder device for 10 min. (D) A cell and a square pillar of the device were observed using a stereoscopic microscope. The arrow and arrow head show the cell and the pillar, respectively. | |
Since these experimental results corresponded with the simulation results, the simulation is considered to be approximately correct. Therefore, we used the culture dish with a thickness of less than 50 µm in subsequent experiments.
Time-lapse images of cell culture arrays
The time-lapse images of the cells patterned into arrays were acquired every 3 min (Fig. 4). The time taken to pattern the cells completely into arrays was 15 s; this is faster than other techniques. Conventional methods that involved the use of chemically and/or biologically modified substrates required washing processes to remove the excess cells from the non-treated regions; however, in our method, cells could be allocated on the arrays in a single step (Fig. 4). Thus, a noteworthy point in constructing cell culture arrays using magnetic force is that magnetically labeled cells can be rapidly allocated on arrays without washing to remove the excess cells.
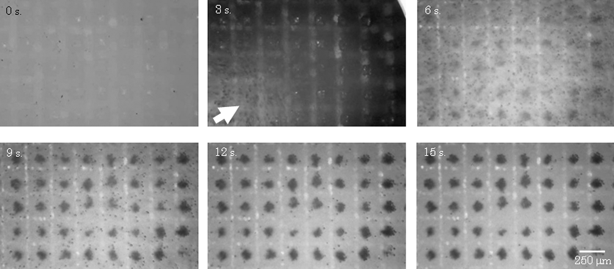 |
| Fig. 4
Cell trapping images. Time-course images were obtained by stereomicroscopy. After seeding magnetically labeled cells into a culture dish with the magnetized device, the cell suspension flowed (3 s). The cells were trapped above the pillars on the device and were completely allocated on the arrays after 15 s. These dark spots in the figure were the cells, and these white lines were the grooves of the device. | |
Single cell culture array
In order to investigate the effect of the number of cells seeded on the individual platforms, the seeding concentration of 3T3 cells was investigated. When the seeding concentration was 88 cells mm–2 (5.5 cell spot–1), the percentage of platforms containing a single cell was only 5% (Fig. 5A). In contrast, when the cells were seeded at a concentration of 16 cells mm–2 (1 cell spot–1), the percentage of platforms containing a single cell was 48% (Fig. 5A, B). Thus, it was demonstrated that single cell culture arrays could be fabricated and the number of cells seeded on individual platforms could be adjusted by varying the cell concentration. Poisson distribution was computed using average number of cells per spot, and the expected Poisson distributions were plotted in Fig. 5A. These results indicate that cells are sorted randomly in an independent fashion into individual spot. In our method, cells were allocated on arrays with a high resolution (Fig. 5B), and are suitable for phenotypic cell assays for detecting individual cell behavior in the cell culture dish by simply harvesting the magnetically labeled cells. When cell culture dishes are modified by ECM, polymers and peptides, the cells on the arrays cannot be collected since the cells strongly adhere to the surface of the biomaterials. On the other hand, in our method, the cells could be collected by a micromanipulator since the cells were not attracted to the culture surface after removing the device with the magnet from the culture dish (Fig. 5C). In order to easily collect a target cell, we are now investigating a method for collecting target cells in a population by using magnetic force. Thus, our method employing MCLs and magnetic force would be useful for single cell analysis.
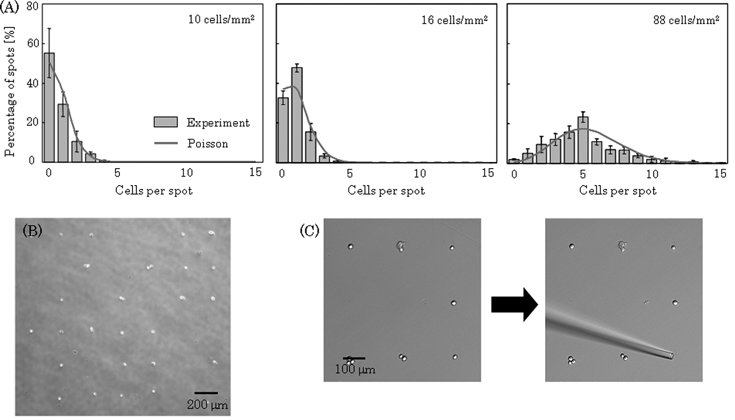 |
| Fig. 5 Measurement of the number of cells in individual spots. The cells (10–88 cells mm–2) were seeded into a culture dish and allocated as described previously. Phase micrographs were acquired after 30 min of culture, and we counted the cells at individual spots using the images. (A) Histogram of the number of spots that contained a particular number of cells. Data points represent means ± SD of 3 independent experiments. Poisson distribution was computed using average number of cells per spot, and the expected Poisson distributions were plotted. (B) A phase micrograph of the cells on arrays when the cells were seeded at a density of 16 cells mm–2. (C) The target cell was collected by a cell manipulator. | |
Cell
patterning using magnetic force and magnetic beads have also been investigated by other groups.33,34 Magnetic beads were bound to the cell surface, and the cells were trapped on a magnet. However, the amount of magnetic beads on the cells was not very high, and the cells exhibited low sensitivity to the magnetic force. The use of MCLs that can be efficiently engulfed by target cells resulted in a high sensitivity of the cells to the magnetic force.
Quantitative analysis of the dynamics of cell shapes
Morphological changes in individual cells were investigated by the image analysis of fluorescence micrographs. We used fluorescence images for image analysis for the precise and easy evaluation of cell morphology. The area, shape factor, and elliptical form factor of individual cells were measured for 5 h, and histograms of the morphological value of the cells were plotted. After culturing for 5 h, the area and the elliptical form factor of individual cells increased, and the shape factor of individual cells dramatically decreased, indicating that the cells on the cell culture array had adhered and had begun to spread on the culture surface (Fig. 6A, B, C). The shape factor reveals how closely the cell represents a circle. A shape factor of 1 represents a cell with a circular shape. The percentage of cells with a shape factor of greater than 0.9 after seeding was 92%. After culturing for 5 h, the percentage dramatically decreased (14%), and the histogram of the shape factor changed from a sharp distribution to a broad distribution (Fig. 6B). This change in the histogram was due to the heterogeneity of the cells. Thus, in our method, the dynamics and heterogeneity of the cells were easily detected. The morphology of the adhered cells was confirmed by comparing cell behavior in arrays that were magnetically patterned using the pin holder device with cells cultured under similar conditions in the control experiments (Fig. 6D, E, F). It was confirmed that similar adherent and elongated morphology was observed in these images (Fig. 6). It should be noted that each pin holder can be identified and the same individual cell can be observed during culture in the case of the morphological analysis of single cells using the pin holder device. In the case of cell culture using a conventional culture dish, however, the morphological value of individual cells in each incubation time was measured from different cells due to the difficulty in cell identification.
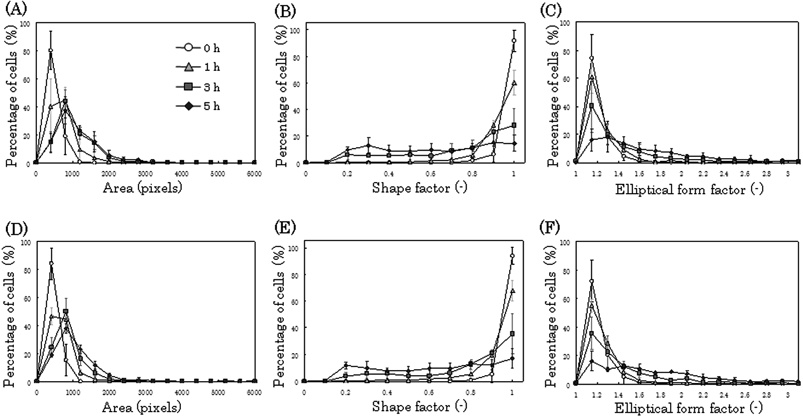 |
| Fig. 6 Morphological analysis of single cells. The fluorescently and magnetically labeled cells were seeded into a culture dish, and the dish was placed on the magnetized pin holder device. Fluorescence images were periodically acquired, and morphological analysis was performed (A, B, C). As a control, the cells were seeded without using the device, and changes in their morphology were analyzed (D, E, F). We measured the area (A, D), shape factor (B, E), and elliptical form factor (C, F) of individual cells using an image analysis software, and the corresponding histograms were generated. The maximum value of the elliptical form factor was 8.95, and after culturing for 5 h, the percentage of cells with an elliptical form factor of more than 3.1 was 7% with cell culture arrays and 5% without cell culture arrays. 1 pixel = 1.67 µm2. Data points represent means ± SD of 4 independent experiments. | |
Single-cell studies of tumor biopsies have shown that the majority of cells within a tumor may be normal, and that significant heterogeneity exists. Therefore, it is important to investigate responses against drug agents for single cell analysis.13 In this study, the cellular responses of individual cells treated with the DNA damaging agent MMC was investigated. The viability of cells treated with MMC (10 µg ml–1) for 14 h remained greater than 95% and was comparable to that of the non-treated cells; this indicated that the concentration of MMC had no effect on cell viability. Since MMC inhibits cell DNA synthesis and has an effect on cell proliferation, the morphology of single cells treated with MMC (1, 10 µg ml–1) differed from that of untreated single cells (Fig. 7). After culturing for 5 h on the arrays, the percentage of cells with a shape factor of greater than 0.9 increased from 14% to 31% on treatment with MMC (10 µg ml–1), and the number of cells with a change in shape decreased; the change in cell shape depended on a concentration of MMC (Fig. 7). Thus, using cell culture arrays, we observed morphological changes brought about by a chemical agent. In our method, arrayed cells are in the same medium and uniform environments can be provided to individual cells.
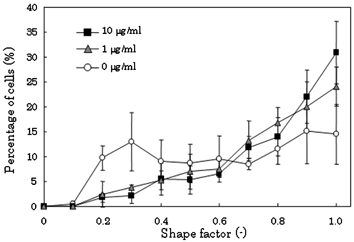 |
| Fig. 7 Morphological analysis of single cells treated with the DNA damaging agent MMC. The fluorescently and magnetically labeled cells were treated with MMC (1, 10 µg ml–1) for 14 h. The cells were allocated on the arrays and their morphology was analyzed as described previously. Data points represent means ± SD of 4 independent experiments. | |
Assessing the toxicity of magnetic nanoparticles and magnetic force is very important for several applications using this technique. We previously investigated the toxicity of MCLs and reported that MCLs did not affect the proliferation of several cell types within the range of MCL concentrations tested (<100 pg of magnetite/cell).29,35 In previous studies, no toxic effects were observed on the growth of 3T3 cells.27,28 We have also compared the morphology of single cells on the arrays with that of cells on a control substrate in this study and demonstrated that there are no differences in cell behavior (Fig. 6). These results indicate that magnetic force-based cell patterning can allocate cells without excess cellular damage. Therefore, we believe that this method is useful for the diagnosis and study of cell–cell interactions.
The uptake of magnetite is important for cell patterning using magnetic force. We previously reported that magnetite uptake in 3T3 cells was 19 pg of magnetite per cell after incubation with MCLs (magnetite concentration, 100 pg cell–1) for 4 h.27Magnetite uptake by several types of cells such as normal and cancer cells was previously measured. Sufficient amount of MCLs was taken up by these cells as a result of the electrostatic interactions between the cells and MCLs, and a sufficient number of cells could be manipulated using the magnet (magnetic flux density, 0.4 T). Therefore, we believe that this method can be applied to several cell types and can be used for the fabrication of single cell culture arrays. In our preliminary study, B16 melanoma cells were also allocated on arrays using the present method (data not shown). Thus, our method has great utility as a useful tool for single cell analysis.
Our technique makes it possible to perform genetic selections and isolate desired phenotypes/genotypes. A recently established transfected cell array has opened new experimental dimensions in the field of functional genomics.36Cell arrays allow transfection of several thousands of different DNA molecules in microarray format. We believe that our technique would be also suitable for genetic selections and isolate desired phenotypes/genotypes.
Albrecht et al. have previously presented a 3D micropatterning technique that rapidly localizes live cells within hydrogels using dielectrophoretic (DEP) forces, and have demonstrated the ability to modulate tissue function through the control of microscale cell architecture.37 However, many conventional cell culture biomaterials, such as collagen, alginate, and Matrigel, may be less suitable for use with DEP electropatterning because they are more conductive, more viscous, or difficult to gel in a closed chamber. A noteworthy point is that magnetic force-based cell patterning is not limited by the property of the culture surface; various culture surfaces may be used, including biological gels, cells and non-absorbent surfaces, because our method can physically attract cells onto the culture surface. In order to analyze cell–cell interactions at the single cell level and evaluate the morphologies at single cell level, we allocated 3T3 cells onto the dish where other 3T3 cells were confluent, and observed the morphological changes of the seeded single cells. It was revealed that the seeded single cells adhered and extended more rapidly than the cells that did not contact to other cells (unpublished data). These results show that the 3T3 cells that contacted the other cells had cell–cell interactions in a few hours. Further experiments on cell–cell interactions at the single cell level are now being investigated using our cell culture arrays.
Conclusions
In conclusion, we have demonstrated a magnetic force-based cell patterning method for creating arrays of single adherent cells. A pin holder device with more than 6000 pillars on the surface was developed from magnetic soft iron for magnetic attraction. Magnetically labeled 3T3 cells were allocated on arrays with a high resolution, and the target cells were easily collected by using a micromanipulator. Additionally, the morphology of individual cells was analyzed and was comparable to that of cells in a static culture. To observe cells allocated on the device, microscopic observation is only possible using an erecting microscope. However, most of our results in this manuscript used an inverted microscope after detaching the device from the culture dish. We anticipate that this technique will be useful in cell diagnosis employing single cell arrays and in studies of cell–cell interactions.
Acknowledgements
One of the authors, Kosuke Ino, is a research fellow of the Japan Society for the Promotion of Science (JSPS). This work was partly supported by JSPS. This work was also supported in part by a grant-in-aid for scientific Research (No. 17760622) and by a grant-in-aid for scientific research on priority areas (No. 17066003) from the Ministry of Education, Sports, Science and Technology, Japan.
References
- C. V. Rao, D. M. Wolf and A. P. Arkin, Nature, 2002, 420, 231–237 CrossRef CAS.
- J. A. White, J. T. Rubinstein and A. R. Kay, Trends Neurosci., 2000, 23, 131–137 CrossRef CAS.
- C. Allen and C. F. Stevens, Proc. Natl. Acad. Sci. U. S. A., 1994, 91, 10380–10383 CrossRef CAS.
- A. van Oudenaarden and J. A. Theriot, Nat. Cell Biol., 1999, 1, 493–499 CrossRef.
- S. M. Simon, C. S. Peskin and G. F. Oster, Proc. Natl. Acad. Sci. U. S. A., 1992, 89, 3770–3774 CAS.
- L. Fink, G. Kwapiszewska, J. Wilhelm and R. M. Bohle, Exp. Toxicol. Pathol., 2006, 57, 25–29 Search PubMed.
- S. D. Ginsberg, Methods, 2005, 37, 229–237 CrossRef CAS.
- B. Bodey, Expert Opin. Biol. Ther., 2002, 2, 371–393 Search PubMed.
- B.F. Brehm-Stecher and E.A. Johnson, Microbiol. Mol. Biol. Rev., 2004, 68, 538–559 CrossRef CAS.
- S. B. Chen, Q. S. Zhang, X. Wu, P. G. Schultz and S. Ding, J. Am. Chem. Soc., 2004, 126, 410–411 CrossRef CAS.
- X. Wu, S. Ding, G. Ding, N. S. Gray and P. G. Schultz, J. Am. Chem. Soc., 2004, 126, 1590–1591 CrossRef CAS.
- X. Wu, S. Ding, G. Ding, N. S. Gray and P. G. Schultz, J. Am. Chem. Soc., 2002, 124, 14520–14521 CrossRef CAS.
- C. E. Sims and N. L. Allbritton, Lab Chip, 2007, 7, 423–440 RSC.
- J. Fukuda, A. Khademhosseini, J. Yeh, G. Eng, J. Cheng, O. C. Farokhzad and R. Langer, Biomaterials, 2006, 27, 1479–1486 CrossRef CAS.
- D. Di Carlo and L. P. Lee, Anal. Chem., 2006, 78, 7918–7925 CrossRef.
- Y. Nahmias, A. Arneja, T. T. Tower, M. J. Renn and D. J. Odde, Tissue Eng., 2005, 11, 701–708 CrossRef CAS.
- A. Khademhosseini, R. Langer, J. Borenstein and J. P. Vacanti, Proc. Natl. Acad. Sci. U. S. A., 2006, 103, 2480–2487 CrossRef CAS.
- H. Hatakeyama, A. Kikuchi, M. Yamato and T. Okano, Biomaterials, 2007, 28, 3632–3643 CrossRef CAS.
- M. Veiseh, O. Veiseh, M. C. Martin, F. Asphahani and M. Zhang, Langmuir, 2007, 23, 4472–4479 CrossRef CAS.
- C. S. Chen, M. Mrksich, S. Huang, G. M. Whitesides and D. E. Ingber, Science, 1997, 276, 1425–1428 CrossRef CAS.
- D. D. Carlo, L. Y. Wu and L. P.Lee, Lab Chip, 2006, 6, 1445–1449 RSC.
- A. Khademhosseini, J. Yeh, G. Eng, J. Karp, H. Kaji, J. Borenstein, O. C. Farokhzad and R. Langer, Lab Chip, 2005, 5, 1380–1386 RSC.
- S. Yamamura, H. Kishi, Y. Tokimitsu, S. Kondo, R. Honda, S. R. Rao, M. Omori, E. Tamiya and A. Muraguchi, Anal. Chem., 2005, 77, 8050–8056 CrossRef CAS.
- J. C. Love, J. L. Ronan, G. M. Grotenbreg, A. G.
van der Veen and H. L. Ploegh, Nat. Biotechnol., 2006, 24, 703–707 CrossRef CAS.
- M. Shinkai, M. Yanase, H. Honda, T. Wakabayashi, J. Yoshida and T. Kobayashi, Jpn. J. Cancer Res., 1996, 87, 1179–1183 CAS.
- K. Ino, A. Ito, H. Kumazawa, H. Kagami, M. Ueda and H. Honda, J. Chem. Eng. Jpn., 2007, 40, 51–58 Search PubMed.
- A. Ito, K. Ino, M. Hayashida, T. Kobayashi, H. Matsunuma, H. Kagami, M. Ueda and H. Honda, Tissue Eng., 2005, 11, 1553–1561 CrossRef CAS.
- K. Shimizu, A. Ito and H. Honda, J. Biomed. Mater. Res. B: Appl. Biomater., 2006, 77, 265–272 Search PubMed.
- K. Ino, A. Ito and H. Honda, Biotechnol. Bioeng., 2007, 97, 1309–1317 CrossRef CAS.
- K. Shimizu, A. Ito, T. Yoshida, Y. Yamada, M. Ueda and H. Honda, J. Biomed. Mater. Res. B: Appl. Biomater., 2007, 82, 471–480 Search PubMed.
- K. Kanlayasiri and S. Boonmung, J. Mater. Process. Technol., 2007, 187, 26–29 CrossRef.
- C. S. Owen and N. L. Sykes, J. Immunol. Methods, 1984, 73, 41–48 CrossRef CAS.
- S. Hashioka, T. Obata, Y. Tokimutsu, S. Fujiki, H. Nakazato, A. Muraguchi, H. Kishi and K. Tanino, Jpn. J. Appl. Phys., 2006, 45, 2850–2853 CrossRef CAS.
- D. C. Pregibon, M. Toner and P. S. Doyle, Langmuir, 2006, 22, 5122–5128 CrossRef CAS.
- A. Ito, M. Hayashida, H. Honda, K. Hata, H. Kagami, M. Ueda and T. Kobayashi, Tissue Eng., 2004, 10, 873–880 CrossRef CAS.
- D. Vanhecke and M. Janitz, Oncogene, 2004, 23, 8353–8358 CrossRef CAS.
- D. R. Albrecht, G. H. Underhill, A. Mendelson and S. N. Bhatia, Lab Chip, 2007, 7, 702–709 RSC.
|
This journal is © The Royal Society of Chemistry 2008 |
Click here to see how this site uses Cookies. View our privacy policy here.