DOI:
10.1039/B9NR00329K
(Paper)
Nanoscale, 2010,
2, 739-746
Large payloads of gold nanoparticles into the polyamine network core of stimuli-responsive PEGylated nanogels for selective and noninvasive cancer photothermal therapy†
Received
(in Beijing, China)
30th October 2009
, Accepted 15th January 2010
First published on
25th February 2010
Abstract
A biocompatible photothermal nanomedicine based on a PEGylated nanogel containing gold nanoparticles (GNPs) in a cross-linked network core of stimuli-responsive poly[2-(N,N-diethylamino)ethyl methacrylate] (PEAMA) gel for cancer photothermal therapy (PTT) was prepared through the reduction of Au(III) ions without any reducing agents. The influence of the reduction conditions, such as pH, temperature, and N/Au ratio (molar ratio of the amino groups in the PEGylated nanogel to the Au(III) ions), on the formation of the GNPs in the stimuli-responsive PEAMA gel core (reducing environment) was also studied. Note that the PEGylated nanogel containing GNPs prepared at pH 6, 60 °C and N/Au = 1 (PEGylated GNG (1)) was found to have the highest GNP-loading capacity with a diameter of about 8 nm, as observed by TEM; viz., about 27 GNPs formed in a single PEAMA gel core. PEGylated GNG (1) showed a remarkable photothermal efficacy (ΔT = 7.7 °C) under irradiation with Ar ion (Ar+) laser (514.5 nm) at a fluence of 39 W cm−2 for 6 min (14 kJ cm−2). Note that PEGylated GNG (1) showed non-cytotoxicity in the absence of irradiation with Ar+ laser (480 μg mL−1: > 90% cell viability), whereas pronounced cytotoxicity (IC50 = 110 μg mL−1) was observed for PEGylated GNG (1) under irradiation with Ar+ laser at a fluence of 26 W cm−2 for 5 min (7.8 kJ cm−2), because of the heat-generation from the GNPs in the cells, which resulted in selective and noninvasive cancer PTT. Thus, PEGylated GNG (1), which has a high GNP-loading capacity, would be a promising nanomedicine for cancer PTT.
1. Introduction
Photodynamic therapy (PDT) is one of the selective and noninvasive ways of treating malignant tumors using photosensitizers (PSs), which are known to effectively produce highly toxic singlet oxygen (1O2) through excitation by light of a characteristic wavelength.1–4 Nevertheless, most PSs show photo-bleaching5 as well as toxic side-effects,2,6,7 such as prolonged skin photosensitivity to daylight. Furthermore, PDT based on a drug delivery system is also limited in vivo, because the aggregation of the loaded PSs in the carrier leads to the self-quenching of the excited state which decreases the generation of 1O2.8,9 An alternative approach is cancer photothermal therapy (PTT) using gold nanoparticles (GNPs)10,11 and their derivatives, including nanoshells12,13 and nanorods,14–16 since they generate heat efficiently through the electron–phonon and phonon–phonon processes under optical illumination when the energy of the incident photons is close to their unique and sensitive surface plasmon bands (SPBs).17,18 Notably, GNPs lend appealing properties to photothermal nanomedicines, including no toxicity,19,20 no self-quenching, large optical cross-sections exceeding those of conventional PSs, and significantly improved susceptibility to chemical/thermal denaturing and photobleaching.21,22 Additionally, antibodies10,12 and poly(ethylene glycol)s (PEGs)16 can be readily conjugated onto the surface of GNPs via the conventional Au–thiol bond to improve cellular specificity, biocompatibility, blood circulation times and accumulation in solid tumors through enhanced permeability and retention (EPR) effects.23 Nevertheless, the therapeutic value of thiol-modified GNPs is controversial under in vivo conditions, due to the cleavage of the Au–thiol bonds by glutathione (thiol-exchange reaction) in the cytosol24–26 where the concentration of glutathione (1–10 mM) is higher than that in extracellular environments (2 μM).27 Eventually, the heat-generation becomes inefficacious due to the change in the SPBs with broadening through the coagulation of the GNPs in the cytosol. Furthermore, they consist of single GNP (i.e., low drug-loading capacity), which leads to the requirement for high dosages of thiol-modified GNPs. An important goal in cancer PTT is the development of nano-sized (<100 nm), noninvasive, PEGylated particles with large payloads of GNPs (i.e., high loading capacity) in order to increase the concentrations of active GNPs (i.e., non-coagulated GNPs) within cancer cells after smooth accumulation in solid tumor tissues via the intra-venous route.
Recently, we reported a new class of PEGylated GNPs28 constructed using a pH-responsive PEGylated nanogel29,30 made from a cross-linked network core of poly[2-(N,N-diethylamino)ethyl methacrylate] (PEAMA) gel and tethered PEG chains that possess a carboxylic acid or acetal group as a platform for the installation of tumor-specific ligand molecules. The pH-responsive PEGylated nanogels show excellent solubility in aqueous media as well as minimum interaction with biological components, owing to the steric stabilization of the flexible and hydrophilic PEG palisade layers surrounding the PEAMA gel core. Note, that the pH-responsive PEGylated nanogel derivatives have unique functions, such as the controlled release of anticancer drugs,31 on–off regulation of 19F magnetic resonance signals,32,33 and endosomal disruption for gene and siRNA delivery systems34,35 synchronizing with the volume phase transition (swelling–deswelling) of the PEAMA gel core in response to the pH. Additionally, the PEAMA segment has been used as the pH-responsive component of the drug and gene carrier due to its minimal cytotoxicity.36,37 Worth noticing is that the formation of multi-GNPs in the PEAMA gel core of the PEGylated nanogel spontaneously occurred at pH 6 and room temperature through an ion exchange between AuCl4−/H+ and the protonated PEAMA gel core (–N+Et2H/Cl−), followed by the reduction of the Au(III) ions to Au(0) particles in the PEAMA gel core without any additional reducing agents or purification.28 The Au(III) ions are presumably reduced by the tertiary amino group of the nanogel through one-electron transfer from the nitrogen atom to Au(III) ion (one-electron reduction), viz., tertiary amino groups present in the cross-linked PEAMA core act as reducing agent. Thus, the tertiary amino groups in the PEAMA gel core play a crucial role in the reduction of the Au(III) ions (reducing environment) as well as the immobilization of the GNPs (nano-matrix). More recently, a caspase-3-responsive and fluorescence-quenching smart apoptosis nanoprobe based on PEGylated nanogel containing GNPs as a fluorescence quencher in the PEAMA gel core and fluorescein isothiocyanate (FITC)-labeled DEVD peptide at the tethered PEG chain end was prepared for monitoring apoptosis.38 This smart apoptosis nanoprobe is capable of detecting apoptotic cells with high specificity and sensitivity. These facts strongly suggest that PEGylated nanogel containing GNPs has excellent stability even in the cytosol, due to the existence of multipoint coordination between the GNPs and the cross-linked PEAMA gel core (amino groups) as well as the cross-linking network. Additionally, the PEGylated nanogels containing GNPs showed heat-generation under irradiation of the laser, allowing the photothermally-triggered release of anionic dyes from the core, synchronizing with the volume phase transition (deprotonation) of the core of PEGylated nanogels containing GNPs.39 However, detailed photothermal properties of PEGylated nanogels containing GNPs and their therapeutic photothermal effects have not been studied yet. The influence of the reaction temperature (i.e., change in the reducing environment) on the formation of GNPs is also an interesting topic for the control of various aspects of the architecture, such as the number of GNPs in a single PEAMA gel core, and shape, size and size distribution, since PEGylated nanogels also show a volume phase transition in response to temperature.29
Herein, we wish to describe the preparation, characterization, photothermal properties, biocompatibility and photothermal therapeutic effects of PEGylated nanogels containing GNPs (PEGylated GNGs) synthesized by the reduction of Au(III) ions in the presence of thermo-responsive PEGylated nanogels (nano-reactor) at pH 6 for various molar ratios of amino groups in the PEGylated nanogel to Au(III) ions (N/Au ratio) under several reaction temperature conditions (5, 25 and 60 °C). The PEGylated GNGs prepared at 60 °C, N/Au =1 and pH 6 were found to contain the highest number of GNPs in a single PEAMA gel core (i.e., the highest drug-loading capacity), allowing high biocompatibility as well as remarkable antitumor activity only in the laser-irradiation regions based on the heat-generation from the GNPs, demonstrating their capacity for noninvasive and selective PTT of human ovarian carcinoma cells (Fig. 1). The PEGylated GNGs described herein present a promising strategy for constructing smart nanomedicines for cancer PTT.
 |
| Fig. 1 Schematic illustration of the preparation of PEGylated GNGs for cancer PTT. | |
2. Materials and method
2.1 Materials and instruments
Ethylene glycol dimethacrylate (EGDMA; Kanto Chemical, Japan) and 2-(N,N-diethylamino)ethyl methacrylate (EAMA, Wako, Japan) were distilled over CaH2 under reduced pressure. Potassium persulfate (KPS, Wako, Japan) was recrystallized from water, and then dried in vacuum. Tetrachloroauric acid (HAuCl4, Wako, Japan), WST-8 (DOJINDO, Japan), Calcein-AM (DOJINDO, Japan) and propidium iodide (PI, DOJINDO, Japan) were used without further purification. Water was purified using a Milli-Q system (Millipore). Dynamic light scattering measurements were carried out using a Zetasizer Nano-ZS instrument (Malvern, UK) equipped with a 4.0 mW He–Ne laser (633 nm). Elemental analysis was carried out using a series II CHNS/O Analyzer 2400 (Perkin-Elmer, Waltham, MA). TEM analysis was carried out using a JEM-100CX microscope (JEOL, Japan) operating at 100 kV, and the average diameter and number of GNPs were calculated from the images using the Image J software (National Institutes of Health, US). The cell viability was determined using Cell Counting Kit-8 (WST-8, DOJINDO, Japan) to measure the absorbance at 450 nm on an ARVO MX microplate reader (Perkin Elmer, Waltham, MA) equipped with a 450 nm filter. The UV-vis spectra were recorded using a UV-2400PC spectrometer (Shimadzu, Japan). Irradiation with Ar ion (Ar+) laser was done using a Stabilite 2017-AR system (beam diameter = 1.4 mm at the 1/e2 points, Spectra Physics, US) with a 514.5 nm filter. Fluorescence microscope images were obtained on an Axiovert 200 M with appropriate filter sets (Carl Zeiss, Germany).
2.2 Synthesis and characterization of the PEGylated nanogel
The α-acetal-ω-vinylbenzyl-poly(ethylene glycol) macromonomer (acetal–PEG–Ph–CH
CH2, Mn = 7,200, Mw/Mn = 1.03) was synthesized as described in our previous report.32,33,35,38,39 The obtained acetal–PEG–Ph–CH
CH2 (500 mg, 69 μmol) and KPS (29.3 mg, 108 μmol) were loaded into a one-necked round-bottom flask, which was evacuated and purged with nitrogen three times. Then, a previously degassed mixture of deionized distilled water (33 mL), EGDMA (20.5 μL, 0.11 mmol, 1.0 mol%) and EAMA (2.18 mL, 10.8 mmol) was added to the flask. Emulsion copolymerization was carried out at room temperature overnight. In this emulsion polymerization, the tertiary amino groups of the EAMA monomer and the KPS spontaneously formed a redox complex (initiator) through the electron transfer from EAMA to KPS at room temperature. Purification was carried out by ultrafiltration (MWCO = 200
000) using methanol (MeOH), followed by the use of water to remove the unreacted PEG and other chemicals. The average diameter of the obtained nanogel as a function of pH and temperature was measured by DLS. Elemental analysis of the PEGylated nanogel was carried out to determine the amine content (calcd: C 62.8, H 10.1, N 6.0; found: C 61.7, H 9.5, N 5.9), and the resulting concentration of amines in the purified nanogel solution (30.4 mg mL−1) was calculated to be 128 mM. To determine the pKa value of the PEGylated nanogel, a solution of PEGylated nanogel in distilled water (40 mL, 0.152 mg mL−1) was adjusted to pH 3.5 by using 0.01 M HCl(aq), and the resulting solution was titrated with 0.1 M NaOH(aq). An automatic titrator (DL-25, METTLER) was used for the titration. In this case, the titrant was added in quantities of 50 μL after the pH values were stabilized (minimal interval: 30 s). The α/pH curves were determined from the obtained titration curves.
2.3 Preparation and characterization of the PEGylated GNGs
As a typical procedure, the preparation of PEGylated nanogel (PEGylated GNG (1)) containing GNPs at an N/Au (number of amino groups/number of Au atoms) ratio of 1 at pH 6 and 60 °C is described. Aqueous solution of HAuCl4 (1.0 mL, 200 μg mL−1, [Au] = 486 μM) at pH 6 was added to a PEGylated nanogel solution (1.0 mL, 115.4 μg mL−1, [N] = 486 μM) at pH 6, and the resulting mixture was incubated at 60 °C until the absorbance at the SPB reached a plateau. PEGylated GNG(2), PEGylated GNG(4) and PEGylated GNG(8) were also prepared according to the same procedure using different concentrations of HAuCl4 (1.0 mL: 100 μg mL−1 for PEGylated GNG(2), 50 μg mL−1 for PEGylated GNG(4), 25 μg mL−1 for PEGylated GNG(8)) and 1.0 mL of PEGylated nanogel solution (115.4 μg mL−1). Purification was carried out by ultracentrifugation (145
000 g) using water to remove the unreacted Au(III) ions and concentrate the PEGylated GNG solution for use the in vitro experiment. TEM samples were prepared by mounting 10-fold diluted drops of the solutions on the carbon-coated copper grids and allowing them to dry in air. TEM analysis was carried out using a JEM-100CX microscope to measure the size of the GNPs. Both the average number of GNPs in a single PEGylated nanogel (n > 50 PEGylated GNGs) and the average diameter of the GNPs (n > 200 GNPs) were calculated from the TEM images using the Image J software statistically.
2.4 Photothermal properties of the PEGylated GNGs
Solutions (2 mL, [PEGylated nanogel] = 57 μg mL−1) of PEGylated GNG at different gold concentrations ([Au] = 48 μg mL−1 for PEGylated GNG(1), 24 μg mL−1 for PEGylated GNG(2), 12μg mL−1 for PEGylated GNG(4), 6 μg mL−1 for PEGylated GNG(8)) at 37 °C were stirred and irradiated with the Ar+ laser at 514.5 nm at a fluence of 39 W cm−2 for 6 min (14 kJ cm−2) using the Stabilite 2017-AR system and the FieldMaster-GS Power and Energy Meter (Coherent, Inc., US). The temperature of the samples was monitored using a 9669-10D microelectrode (HORIBA, Ltd., Japan).
2.5 Cytotoxicity assay of the PEGylated GNGs
HeLa human cancer cells derived from a human cervical epithelial carcinoma cell line were obtained from the RIKEN BioResource Center. The cells were grown in Dulbecco's modified Eagle's medium (DMEM, Sigma, St. Louis, MO) supplemented with 10% fetal bovine serum (FBS), 100 units/mL penicillin and 100 mg mL−1 streptomycin at 37 °C in a humidified atmosphere containing 5% CO2. The cells were placed in a 96-well microplate (104 cells/well) and incubated for 24 h. After changing to fresh DMEM with 10% FBS (90 μL/well), appropriate amounts (10 μL/well) of PEGylated GNGs or the PEGylated nanogels were added to each well, and the cells were incubated for 24 h. After the medium replacement, 100 μL of flesh medium and 10 μL of WST-8 reagent were added to each well. After further incubation for 1 h, the optical absorbance at 450 nm contributed to the formazan produced by living cells was measured using a microplate reader, and it was converted to a percentage relative to control (untreated) cells.
2.6 Photothermal therapy using PEGylated GNGs
HeLa cells were placed in a 96-well microplate (104 cells/well) and incubated for 24 h. After changing to fresh DMEM with 10% FBS (90 μL/well), appropriate amounts (10 μL/well) of PEGylated GNGs were added to each well, and the cells were incubated for 24 h. The cells were washed twice with PBS, and then 90 μL of fresh medium (phenol red free) were added to each well. The cells were exposed to Ar+ laser emitted by the Stabilite 2017-AR system (514.5 nm) at a fluence of 26 W cm−2 for 5 min (7.8 kJ cm−2) to induce photothermal cell damage. After irradiation with the laser, 10 μL of Cellstain-Double Staining Kit (DOJINDO, Japan) solution composed of calcein-AM (4 μM in PBS) and PI (8 μM in PBS) were added to each well to evaluate the cell viability. After incubation for 1 h, the cells were imaged directly in the cell culture medium using a Zeiss Axiovert 200 M equipped with appropriate filter sets. The cell viability was determined from the obtained images using Image J software.
3. Results and discussion
3.1 Preparation and characterization of pH- and thermo-responsive PEGylated nanogel
Stimuli-responsive PEGylated nanogel was synthesized at room temperature by the emulsion copolymerization of 2-(N,N-diethylamino)ethyl methacrylate (EAMA) with PEG macromonomer possessing an acetal group at the α-end and a 4-vinybenzyl group at the ω-end (acetal–PEG–Ph–CH
CH2: Mn = 7,200, Mw/Mn = 1.03) in the presence of potassium persulfate as initiator and ethylene glycol dimethacrylate (1.0 mol%) as cross-linker, as described previously.28,29,31,32,35,38,39Fig. 2a shows the pH dependency of the diameter and α (degree of protonation)/pH curve of the PEGylated nanogel at 25 °C. The diameter of the PEGylated nanogel increased significantly with decreasing pH from 7 to 6, reaching an 8.9-fold larger hydrodynamic volume at pH 6 (diameter = 180 nm) compared to that at pH 7 (diameter = 87 nm). This pH-induced size variation of the PEGylated nanogel is almost consistent with its α/pH curve. This fact strongly suggests that the protonation of the tertiary amino groups in the PEAMA core triggers the swelling of the PEGylated nanogel due to the increase in the ion osmotic pressure as well as the solvation of the PEAMA core. Fig. 2b shows the temperature dependency of the diameter and α/temperature curve of the PEGylated nanogel at pH 6. The diameter of the PEGylated nanogel also increased with decreasing temperature from 50 to 15 °C, reaching an 8.4-fold larger hydrodynamic volume at 5 °C (diameter = 187 nm) compared to that at 60 °C (diameter = 92 nm). Since the thermo-induced size variation of the PEGylated nanogel was almost consistent with its α/temperature curve, the thermo-sensitivity of the PEGylated nanogel was most likely due to the change in the degree of protonation of the tertiary amino groups in the PEAMA core (5 °C: pKa = 6.8, 25 °C: pKa = 5.9, 60 °C: pKa = 4.7; see Fig. S1 in the ESI†). Note that the obtained PEGylated nanogel showed an almost similar swelling ratio (8–9) synchronizing with the pH as well as the temperature; viz., the PEGylated nanogel acted as a pH- and thermo-responsive nano-reactor for the preparation of GNPs through the reduction of Au(III) ions.
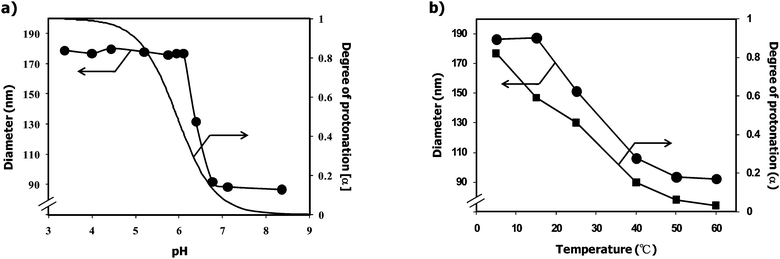 |
| Fig. 2 a) pH dependency of the diameter (circle) and degree of protonation (α) of the PEGylated nanogels at 25 °C. b) Temperature dependency of the diameter (circle) and degree of protonation (α) of the PEGylated nanogels at pH 6. | |
3.2 Preparation and characterization of PEGylated GNGs
We recently reported the reduction of Au(III) ions using slightly cross-linked PEGylated nanogel (cross-linking density: 0.1 mol%) to prepare PEGylated GNGs.28 Indeed, the PEGylated GNGs were successfully prepared at pH = 6, room temperature (ca. 25 °C), and various N/Au ratios. Note that the average number of GNPs in a single PEAMA gel core can be readily controlled by varying the N/Au ratio. In this study, we used PEGylated nanogel with a higher cross-linking density (1.0 mol%) as the nano-reactor and nano-matrix. Once again, the effect of the pH conditions on the preparation of PEGylated GNGs was studied, because the swelling ratio of PEGylated nanogels is known to be significantly dependent on the cross-linking density (cross-linking density: 0.1 mol%; swelling ratio = ca. 45).34 The final concentration of the PEGylated nanogel in all reaction solutions was constant ([PEGylated nanogel] = 57.7 μg mL−1, [N] = 243 μM). The reduction of the Au(III) ions using the PEGylated nanogels was monitored on a UV-vis spectrometer, since the SPB of the GNP is known to be sensitive to the shape and size of the GNP.40Fig. 3 shows the UV-vis spectra of the reaction solutions of the PEGylated GNGs prepared at N/Au = 1 (defined as PEGylated GNGs (1)) and 25 °C under different pH conditions (pH 4, 6, 7 and 10). The reaction solution at pH 10 showed a little absorbance at the SPB, indicating that a small amount of GNPs was formed. On the contrary, an increase in absorbance at the SPB was observed for the reaction solution at pH 4, with an increase in the absorbance at >650 nm (i.e., the color of the solution changed to purple) caused by the coagulation of GNPs. In sharp contrast, the reaction solutions at pH 6 and 7 gradually changed in color from yellow to pinkish-red, and a significant increase in the absorbance at the SPB was observed at pH 6 without coagulation, strongly indicating that the formation of PEGylated GNG (1) occurred through the reduction of HAuCl4 using the PEGylated nanogel. The pH conditions affected the formation of PEGylated GNG (1) presumably due to the change in both the degree of protonation (hydrophilic–hydrophobic environments) of the PEAMA core of the PEGylated nanogel (pH 4: α = 1.0, pH 6: α = 0.5, pH 7: α = 0.1, pH 10: α = 0 in Fig. 1a)) and the Au(III)-complex species (AuClX(OH)4−X−; X = 0–4) which have different redox potentials.41–43 It can be concluded that the optimal pH for the reduction of Au(III) ions using the PEGylated nanogel is 6, which is in agreement with our previous report.28 Therefore, the effect of temperature on the preparation of PEGylated GNGs was studied at pH 6.
 |
| Fig. 3 UV-vis spectra of PEGylated GNG (1) prepared at 25 °C at pH 4 (black), 6 (red), 7 (green) and 10 (blue). | |
To clarify the effect of the reaction temperature on the preparation of the PEGylated GNGs, the reduction of Au(III) ions using the PEGylated nanogel was carried out at various N/Au ratios (1, 2, 4 and 8), pH 6, and different temperatures (5 °C, 25 °C, and 60 °C). The reaction solution at 60 °C immediately changed in color from yellow to pinkish-red within 30 min, whereas the color of the reaction solutions at 5 °C and 25 °C gradually changed to pinkish-red after incubation for 100 h and 15 h, respectively. Thus, the velocity of the reduction was dependent on the reaction temperature. Fig. 4 shows typical transmission electron microscopy (TEM) images of PEGylated GNG (1) and PEGylated GNG (4) prepared at 5 °C, 25 °C and 60 °C. The average diameter of the GNPs and the average number of GNPs in a single PEAMA gel core were calculated from the TEM images shown in Fig. 4 and Fig. S2 (see Fig. S2 in the ESI†). As seen in Fig. 4 and S2, the GNPs (high contrast) as well as the cross-linked PEAMA gel core (lower contrast; <100 nm) were observed, and the GNPs were found to localize in the PEAMA gel core, obviously indicating that the PEGylated nanogels acted as a reducing environment as well as a nano-matrix to produce and immobilize the GNPs in the PEAMA core. Note, that the average diameter of the GNPs (ca. 10–13 nm) in all the PEGylated GNGs prepared at 5 °C was slightly larger than that of the GNPs (ca. 8 nm) in all the PEGylated GNGs prepared at 25 °C and 60 °C, but an N/Au ratio dependency of the average diameter of the GNPs was not observed for all PEGylated GNGs (Fig. 5a). As the N/Au ratio decreased, the average number of GNPs in a single PEGylated GNG increased for all reaction temperatures (Fig. 5b). Worth noticing is that the average number of GNPs in a single PEAMA gel core of PEGylated GNG (1) prepared at N/Au = 1 increased significantly with increasing reaction temperature (5 °C: 6 GNPs; 25 °C: 15 GNPs; 60 °C: 27 GNPs); viz., the reduction of Au(III) ions using the PEGylated nanogel at N/Au = 1 and 60 °C afforded the PEGylated GNG (1) containing the highest number of GNPs. Although the effect of the reaction temperature on the formation of PEGylated GNGs is still not fully clear in detail, we presume that the change in the degree of protonation (hydrophilic–hydrophobic environments) as well as the nano-space of the PEAMA core of the PEGylated nanogel (5 °C: α = 0.8, 186 nm; 25 °C: α = 0.5, 151 nm; 60 °C: α = 0.03, 92 nm) plays a crucial role in the formation of GNPs. Further mechanistic study on the reduction is now in progress and the results will be published elsewhere. Additionally, Fig. 6 shows the UV-vis spectra of the PEGylated GNGs (1, 2, 4 and 8) prepared at 60 °C. The N/Au ratio dependency of the average number of GNPs in a single PEGylated GNG was simply confirmed by the increase in absorbance at the SPB, because the average diameter of the GNPs for all PEGylated GNGs was constant (ca. 8 nm). It should be noticed that no increase in absorbance at >650 nm was observed even for PEGylated GNG (1) containing a large number of GNPs (ca. 27 GNPs) in single PEAMA gel core, strongly indicating that hardly any aggregation of GNPs occurred in the PEAMA core.
 |
| Fig. 4 Typical TEM images of PEGylated GNG (1) and PEGylated GNG (4) prepared at 5 °C, 25 °C and 60 °C. | |
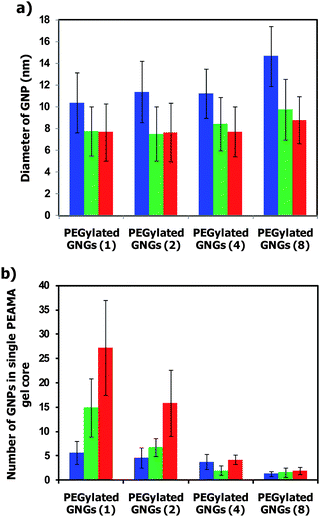 |
| Fig. 5 a) Average diameter of the GNPs (n > 200 GNPs) and b) average number of GNPs in a single PEGylated GNG (n > 50 PEGylated GNGs) prepared at pH 6 at 5 °C (blue bar), 25 °C (green bar), and 60 °C (red bar). | |
![UV-vis spectra of PEGylated GNG (1) (black), (2) (red), (4) (green) and (8) (blue) prepared at pH 6 at 60 °C ([PEGylated nanogel] = 57 μg mL−1).](/image/article/2010/NR/b9nr00329k/b9nr00329k-f6.gif) |
| Fig. 6 UV-vis spectra of PEGylated GNG (1) (black), (2) (red), (4) (green) and (8) (blue) prepared at pH 6 at 60 °C ([PEGylated nanogel] = 57 μg mL−1). | |
3.3 Photothermal properties of PEGylated GNGs
GNPs are known to generate heat efficiently under optical illumination when the energy of the incident photons is close to their surface plasmon band at around 520 nm;10,11,39,44 thus, they offer appealing properties as photothermal therapeutic reagents. The photothermal properties of PEGylated GNGs (1, 2, 4 and 8) prepared at 60 °C were evaluated by irradiation with a laser at the same PEGylated nanogel concentration (57 μg mL−1), because all PEGylated GNGs prepared at 60 °C have almost same average size of the GNPs (ca. 10–13 nm), viz., the effect of the size of GNPs on the photothermal properties is a negligible. Fig. 7 shows the temperature increase (ΔT) of water in the presence of PEGylated GNGs (1, 2, 4 and 8) or PEGylated nanogel at 37 °C after irradiation with a 600 mW Ar ion (Ar+) laser (λ = 514.5 nm) at a fluence of 39 W cm−2 for 6 min (14 kJ cm−2). Note, that increase in solution temperature were observed for all PEGylated GNGs (1, 2, 4 and 8) under irradiation with the Ar+ laser. In sharp contrast, the PEGylated nanogels without GNPs showed negligible increment in the solution temperature even under irradiation with the Ar+ laser. Notably, the photothermal efficacy of the PEGylated GNGs is dependent on the number of GNPs in a single PEGylated GNG (i.e., the N/Au ratio); viz., the highest increment of the solution temperature (ΔT = 7.7 °C) was observed for PEGylated GNG (1). Additionally, all PEGylated GNGs showed almost the same increment of the solution temperature (ΔT = 6.4 °C) at a constant Au concentration ([Au] =48 μg mL−1) after irradiation with the Ar+ laser at a fluence of 39 W cm−2 for 4 min (9.4 kJ cm−2) (see Fig. S3 in the ESI†), suggesting that the photothermal efficacy depends on the Au concentration. These facts strongly indicate that the increments in solution temperature under irradiation with the Ar+ laser are due to the efficient generation of heat from the GNPs in the PEGylated GNGs.
![Increments of the temperature (ΔT) of PEGylated GNG (1, 2, 4, and 8) solutions and the PEGylated nanogel solution after irradiation with the 600 mW Ar+ laser (514.5 nm) at a fluence of 39 W cm−2 for 6 min (14 kJ cm−2) ([PEGylated nanogel] = 57 μg mL−1).](/image/article/2010/NR/b9nr00329k/b9nr00329k-f7.gif) |
| Fig. 7 Increments of the temperature (ΔT) of PEGylated GNG (1, 2, 4, and 8) solutions and the PEGylated nanogel solution after irradiation with the 600 mW Ar+ laser (514.5 nm) at a fluence of 39 W cm−2 for 6 min (14 kJ cm−2) ([PEGylated nanogel] = 57 μg mL−1). | |
3.4 Cytotoxicity of PEGylated GNGs
The preparation of highly biocompatible nanoparticles has been one of the most challenging issues in their application to nanomedicines in vivo. The cytotoxicity of the PEGylated GNGs (1, 2, 4 and 8) prepared at 60 °C and that of the PEGylated nanogel were evaluated by WST assay in cultured HeLa cells (human cervical epithelial carcinoma cell line). Fig. 8 shows the viability of HeLa cells as a function of the concentration of the PEGylated nanogels. PEGylated nanogel with cross-linking density (1.0 mol%) showed relatively high cytotoxicity in a dose-dependent manner despite the existence of PEG chains surrounding the PEAMA gel core; viz., the 50% inhibitory concentration (IC50) was found to be 7 μg mL−1. In contrast, PEGylated GNG (8) (IC50 = 23 μg mL−1) and PEGylated GNG (4) (IC50 = 160 μg mL−1) showed lower cytotoxicity than the PEGylated nanogel. Note, that PEGylated GNG (1) and PEGylated GNG (2) showed no cytotoxicity even at a concentration of 480 μg mL−1 (>90% cell viability). These results suggest that the cytotoxicity of the PEGylated GNGs (1, 2, 4 and 8) was obviously dependent on the number of GNPs in a single PEAMA gel core (i.e., the N/Au ratio). The observed high cytotoxicity of the PEGylated nanogel is most likely due to the partial exposure of the PEAMA core to the outside and/or the existence of dangling PEAMA chains caused by a low cross-linking density. Since the cytotoxicity of the PEGylated nanogel is known to be significantly dependent on the cross-linking density,34 we presume that the appearance of a non-covalent cross-link constructed from multipoint coordination between the GNP and the tertiary amino groups in the PEAMA gel core increased with the increase in the number of GNPs in a single PEAMA gel core. Thus, PEGylated GNG (1) and PEGylated GNG (2) performed the effective compartmentalization of the PEAMA gel core by surrounding it with by PEG chains to prevent both exposure of the PEAMA core to the outside and the formation of dangling PEAMA chains, leading to a significantly low cytotoxicity. It can be concluded that PEGylated GNG (1), which contains the highest number of GNPs in a single PEAMA gel core (i.e., high drug-loading capacity), seems to be suitable as a photothermal nanomedicine for cancer PTT due to its efficient photothermal properties (ΔT = 7.7 °C) as well as its high biocompatibility (IC50 > 480 μg mL−1).
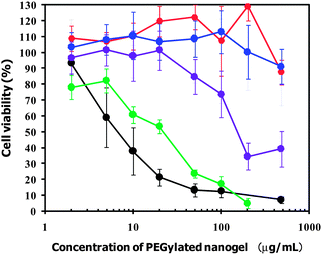 |
| Fig. 8 Cytotoxic profiles of PEGylated GNG (1) (red), (2) (blue), (4) (purple) and (8) (green) and the PEGylated nanogel (black). The viability of the HeLa cells is expressed as a function of the PEGylated nanogel concentration. The plotted data are averages of 6 experiments ± SD. | |
3.5 Cancer photothermal therapy using PEGylated GNG
The photothermal therapeutic effects of PEGylated GNG (1) and PEGylated GNG (2) against HeLa cells were evaluated by live/dead staining assay (double staining assay) after irradiation with the Ar+ laser. The living and dead cells were individually detected as green fluorescence and red fluorescence, respectively, and the cell viability was calculated by counting the living versus dead cells. As a preliminary experiment, HeLa cells in the absence of any PEGylated GNGs were exposed to Ar+ laser at a fluence of 13, 26 and 52 W cm−2 for 5 min (3.9, 7.8, 15.6 kJ cm−2, respectively). Almost all HeLa cells were viable (green fluorescence) after irradiation with the Ar+ laser even at 52 W cm−2 (15.6 kJ cm−2) (see Fig. S4 in the ESI†), indicating that a fluence below 52 W cm−2 over a period less than 5 min (15.6 kJ cm−2) can be used for the PTT of HeLa cells. The photothermal therapy of HeLa cells using PEGylated GNG (1) or PEGylated GNG (2) was carried out by irradiation with an Ar+ laser at a fluence of 26 W cm−2 for 5 min (7.8 kJ cm−2). Prior to irradiation with the Ar+ laser, the HeLa cells were incubated with the PEGylated GNGs, followed by washing the cells with PBS. Fig. 9 shows the viability and fluorescence microscope images of the HeLa cells as a function of the concentration of PEGylated nanogel with or without irradiation using the Ar+ laser. The data of the WST assay for the dark cytotoxicity (i.e., without irradiation using the Ar+ laser) of the PEGylated GNGs in Fig. 8 are restated again in Fig. 9 to clarify the photothermal efficacy of the PEGylated GNGs. The HeLa cells treated with the PEGylated GNGs alone without irradiation using Ar+ laser had a cell viability of approximately 90% even at a PEGylated nanogel concentration of 480 μg mL−1, as mentioned above for Fig. 8. In sharp contrast, the viability of the HeLa cells treated with PEGylated GNG (1) or the PEGylated GNG (2) decreased with increasing dosage after irradiation with the Ar+ laser. Notably, the IC50 values were found to be 110 μg mL−1 ([Au] = 93 μg mL−1: See Fig. S5 in the ESI†) and 317 μg mL−1 ([Au] = 132 μg mL−1: See Fig. S5 in the ESI†) for PEGylated GNG (1) and the PEGylated GNG (2), respectively. Thus, PEGylated GNG (1) showed a 2.9-fold higher photothermal therapeutic effect compared to PEGylated GNG (2). Furthermore, the absorbance at the SPB (535 nm) of PEGylated GNG (1) and PEGylated GNG (2) in the HeLa cells increased in a dose-dependent manner (see Fig. S6 in the ESI†); viz., the cellular association with and/or the internalization of PEGylated GNG (1) and PEGylated GNG (2) into the HeLa cells were obviously observed. Also, the amount of the cell-associated and/or internalized PEGylated GNG (1) was larger than that of PEGylated GNG (2). The pronounced photothermal efficacy of PEGylated GNG (1) was most likely due to the fact that PEGylated GNG (1) had a larger number of GNPs in a single PEAMA gel core (i.e., higher drug-loading capacity) compared to PEGylated GNG (2). Notably, the fluorescence microscope images of the HeLa cells treated with PEGylated GNG (1) at 200 μg mL−1 showed cell death (red fluorescence) only in the circular regions after irradiation with the Ar+ laser, whereas living cells (green fluorescence) were observed only outside of the circular regions. The diameters of these circular regions match the laser spot size, confirming that the observed cell death was confined to the areas irradiated with the Ar+ laser in the presence of PEGylated GNG (1). These facts reveal that the decrease in viability of the HeLa cells treated with PEGylated GNG (1) and irradiated with the Ar+ laser was due to the photothermal effect of PEGylated GNG (1). This means that PEGylated GNG (1) can be used as a selective and noninvasive photothermal nanomedicine for cancer PTT.
 |
| Fig. 9 a) Viability of the HeLa cells treated with PEGylated GNG (1) (closed circle) and PEGylated GNG (2) (open circle) at various concentrations with (red) or without (green) irradiation using Ar+ laser (514.5 nm) at a fluence of 26 W cm−2 for 5 min (7.8 kJ cm−2). b) Fluorescence microscope images of HeLa cells treated with the PEGylated GNG (1) at various concentrations with irradiation using Ar+ laser. The living and dead cells emit green and red fluorescence, respectively. | |
4. Conclusions
Novel PEGylated nanogels containing GNPs (PEGylated GNGs) were successfully prepared through the reduction of Au(III) ions using the stimuli-responsive PEGylated nanogels as a reducing environment and nano-matrix. PEGylated GNG (1) prepared at pH 6, 60 °C and N/Au = 1 was found to have the highest number of GNPs in a single PEAMA gel core (ca. 27 GNPs/PEAMA gel core) as well as high biocompatibility. It is worth noticing that the PEGylated GNG (1) showed a remarkable photothermal efficacy; viz., selective and noninvasive cancer PTT in response to light was achieved. Therefore, the PEGylated GNGs with high GNP-loading capacity described here would be promising nanomedicines for cancer PTT.
Acknowledgements
This work was partially supported by a Gran in Aid of Scientific Research (A) (#1800033) and the World Premier International Research Center Initiative Program from the Ministry of Education, Culture, Sports, Science and Technology of Japan (MEXT).
References
- R. R. Allison, R. Cuenca, G. H. Downie, M. E. Randall, V. S. Bagnato and C. H. Sibata, Photodiagn. Photodyn. Ther., 2005, 2, 51 CrossRef CAS.
- D. E. J. G. J. Dolmans, D. Fukumura and R. K. Jain, Nat. Rev. Cancer, 2003, 3, 380 CrossRef CAS.
- I. J. Macdonald and T. J. Dougherty, J. Porphyrins Phthalocyanines, 2001, 5, 105 CrossRef CAS.
- J. M. Jr. McCaughan, Drugs Aging, 1999, 15, 49 CrossRef CAS.
- R. Bonnett and G. Martinez, Tetrahedron, 2001, 57, 9513 CrossRef.
- M. R. Hamblin and E. L. Newman, J. Photochem. Photobiol., B, 1994, 23, 3 CrossRef CAS.
- T. J. Dougherty, M. T. Cooper and T. S. Mang, Lasers Surg. Med., 1990, 10, 485 CAS.
- C. J. F. Rijcken, J.-W. Hofman, F. van Zeeland, W. E. Hennink and C. F. van Nostrum, J. Controlled Release, 2007, 124, 144 CrossRef CAS.
- I. E. Borissevitch, T. T. Tominaga and C. C. Schmitt, J. Photochem. Photobiol., A, 1998, 114, 201 CrossRef CAS.
- I. H. El-Sayed, X. Huang and M. A. El-Sayed, Cancer Lett., 2006, 239, 129 CrossRef CAS.
- V. P. Zharov, V. Galitovsky and M. Viegas, Appl. Phys. Lett., 2003, 83, 4897 CrossRef CAS.
- C. Loo, A. Lowery, N. Halas, J. West and R. Drezek, Nano Lett., 2005, 5, 709 CrossRef CAS.
- L. R. Hirsch, R. J. Stafford, J. A. Bankson, S. R. Sershen, B. Rivera, R. E. Price, J. D. Hazle, N. J. Halas and J. L. West, Proc. Natl. Acad. Sci. U. S. A., 2003, 100, 13549 CrossRef CAS.
- T. Kawano, Y. Niidome, T. Mori, Y. Katayama and T. Niidome, Bioconjugate Chem., 2009, 20, 209 CrossRef CAS.
- X. Huang, I. H. El-Sayed, W. Qian and M. A. El-Sayed, J. Am. Chem. Soc., 2006, 128, 2115 CrossRef CAS.
- T. Niidome, M. Yamagata, Y. Okamoto, Y. Akiyama, H. Takahashi, T. Kawano, Y. Katayama and Y. Niidome, J. Controlled Release, 2006, 114, 343 CrossRef CAS.
- S. Link and M. A. El-Sayed, J. Phys. Chem. B, 1999, 103, 8410 CrossRef CAS.
- D. Boyer, P. Tamarat, A. Maali, B. Lounis and M. Orrit, Science, 2002, 297, 1160 CrossRef CAS.
- I. H. El-Sayed, M. Singer and F. Civantos, Otolaryngologic Clin. of North Am., 2005, 38, 145 Search PubMed.
- A. I. Sherman and M. Ter-Pogossian, Cancer, 1953, 6, 1238 CrossRef CAS.
- E. E. Connor, J. Mwamuka, A. Gole, C. J. Murphy and M. D. Wyatt, Small, 2005, 1, 325 CrossRef CAS.
- M. C. Daniel and D. Astruc, Chem. Rev., 2004, 104, 293 CrossRef CAS.
- Y. Matsumura and H. Maeda, Cancer Res., 1986, 46, 6387 CAS.
- M. Oishi, J. Nakaogami, T. Ishii and Y. Nagasaki, Chem. Lett., 2006, 35, 1046 CrossRef CAS.
- R. Hong, G. Han, J. M. Fernandez, B.-J. Kim, N. S. Forbes and V. M. Rotello, J. Am. Chem. Soc., 2006, 128, 1078 CrossRef CAS.
- A. Verma, J. M. Simard, J. W. Worrall and V. M. Rotello, J. Am. Chem. Soc., 2004, 126, 13987 CrossRef CAS.
- A. Meister and M. E. Anderson, Annu. Rev. Biochem., 1983, 52, 711 CrossRef CAS.
- M. Oishi, H. Hayashi, T. Uno, T. Ishii, M. Iijima and Y. Nagasaki, Macromol. Chem. Phys., 2007, 208, 1176 CrossRef CAS.
- M. Oishi and Y. Nagasaki, React. Funct. Polym., 2007, 67, 1311 CrossRef CAS.
- H. Hayashi, M. Iijima, K. Kataoka and Y. Nagasaki, Macromolecules, 2004, 37, 5389 CrossRef CAS.
- M. Oishi, H. Hayashi, M. Iijima and Y. Nagasaki, J. Mater. Chem., 2007, 17, 3720 RSC.
- M. Oishi, S. Sumitani, T. K. Bronich, A. V. Kabanov, M. D. Boska and Y. Nagasaki, Chem. Lett., 2009, 38, 128 CrossRef CAS.
- M. Oishi, S. Sumitani and Y. Nagasaki, Bioconjugate Chem., 2007, 18, 1379 CrossRef CAS.
- M. Oishi, H. Hayashi, K. Itaka, K. Kataoka and Y. Nagasaki, Colloid Polym. Sci., 2007, 285, 1055 CrossRef CAS.
- A. Tamura, M. Oishi and Y. Nagasaki, Biomacromolecules, 2009, 10, 1818 CrossRef CAS.
- Y. Hu, T. Litwin, A. R. Nagaraja, B. Kwong, J. Katz, N. Watson and D. J. Irvine, Nano Lett., 2007, 7, 3056 CrossRef CAS.
- P. Xu, E. A. Van Kirk, W. J. Murdoch, Y. Zhan, D. D. Isaak, M. Radosz and Y. Shen, Biomacromolecules, 2006, 7, 829 CrossRef CAS.
- M. Oishi, A. Tamura, T. Nakamura and Y. Nagasaki, Adv. Funct. Mater., 2009, 19, 827 CrossRef CAS.
- M. Oishi, T. Nakamura, Y. Jinji, K. Matsuishi and Y. Nagasaki, J. Mater. Chem., 2009, 19, 5909 RSC.
- A. Henglein, J. Phys. Chem., 1993, 97, 5457 CrossRef CAS.
- J. D. Henao, Y.-W. Suh, J.-K. Lee, M. C. Kung and H. H. Kung, J. Am. Chem. Soc., 2008, 130, 16142 CrossRef CAS.
- M. C. Henry, C. C. Hsueh, B. P. Timko and M. S. Freund, J. Electrochem. Soc., 2001, 148, D155 CrossRef.
- D. V. Goia and E. Matijevic, Colloids Surf., A: Physiochem. Eng. Aspects, 1999, 146, 139 Search PubMed.
- Y. Haba, C. Kojima, A. Harada, T. Ura, H. Horinaka and K. Kono, Langmuir, 2007, 23, 5243 CrossRef CAS.
Footnote |
† Electronic supplementary information (ESI) available: Additional figures. See DOI: 10.1039/b9nr00329k |
|
This journal is © The Royal Society of Chemistry 2010 |
Click here to see how this site uses Cookies. View our privacy policy here.