DOI:
10.1039/B9NR00364A
(Minireview)
Nanoscale, 2010,
2, 373-380
Carbon nanotubes: promising agents against free radicals
Received
(in Zürich, Switzerland)
20th November 2009
, Accepted 31st December 2009
First published on 1st February 2010
Abstract
The potential role of carbon nanotubes (CNTs) as free-radical scavengers is still an emerging area of research. So far some promising results have been reported strongly suggesting that CNTs can be very efficient for that task. The implications of such a valuable property for applications aimed at biomedical and environmental uses are encouraging. There are still abundant open questions related to the possible use of CNTs for scavenging free radicals. Thus much more work needs to be devoted to this fascinating topic. In this mini review the progress made so far is reviewed and some future perspectives are provided.
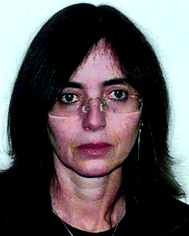 Annia Galano | Annia Galano was born in Havana, Cuba, in 1967. She graduated in Chemistry from Havana University in 1990 and got her PhD degree in 2000. She worked from 2001 to 2007 at the Mexican Institute of Petroleum. Since 2008 she has been a Professor at the Chemistry Department in the Metropolitan Autonomous University, also in Mexico. Her area of expertise is Computational Chemistry and her main research interests are related to mechanisms and kinetics of radical–molecule reactions. In particular those involved in Atmospheric Chemistry, nanometric systems, oxidative stress, and free-radical scavenging processes. |
Introduction
The door into the fascinated world of nanomaterials is now opened. These small, and yet great, structures have deeply and widely impacted science and technology in the last few decades. This can be easily demonstrated by the large amount of research that has been devoted to them. In particular carbon nanotubes (CNTs) have attracted agreat deal of attention, most likely because, despite the fact that they are all mainly made of carbon, they can exhibit very peculiar and diverse properties. These varied, and some times amazing and extreme, properties immediately bring to mind a wide range of potential applications. Moreover they can be tuned by small structural modification of the carbon nanomaterials, which opens an endless world of possibilities.
The history of CNTs is also very interesting. Even though the discovery of CNTs is frequently attributed to Iijima in 1991,1 according to Monthioux and Kuznetsov,2 the first mention of carbon filaments dates back to 1889.3 In that patent Hughes and Chambers proposed the use of such filaments in light bulbs. Two other early reports, mentioning the existence of carbon filaments, were presented at the French Academy of Sciences in 18904 and 1903,5 respectively. However, due to the resolution of the microscopes available at the time, these works can hardly be considered as the first demonstrations of the existence of CNTs since they could not revealed the inner cavity of the filaments.2,6 The first evidence of the tubular nature of nano-sized carbon filaments was achieved thanks to the discovery of the transmission electron microscope (TEM), and was reported in 1952.7 In addition to the above mentioned reports, CNTs were re-discovered again in 19588 and 1973.9 However, none of these early works caught the attention of the scientific community like that by Iijima.1 In fact it was only after his paper that the study of CNTs became one of the most active areas of research worldwide. On the other hand, there is no controversy about the discovery of single-walled carbon nanotubes (SWCNTs). They were simultaneously and independently reported by Iijima and Ichihashi,10 and by Bethune et al. in 1993.11 The number of published research papers devoted to carbon nanotubes since 1991 is simply astonishing. According to the Scopus database,12carbon nanotubes appeared in more than 43
000 papers and in more than 19
000 patents within the 1992–2009 period. Moreover, taking into account that 2009 had not ended at the time of the search, the increasing trend (Fig. 1) does not seem to have reached its limit yet.
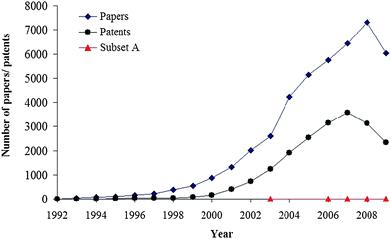 |
| Fig. 1 Number of papers/patents with carbon nanotubes as the topic, according to the Scopus database (consulted November 19th, 2009). Red triangles correspond to the subset A: papers dealing with the possible free-radical scavenging activity of CNTs. | |
However, if we add free radicals to our search, the number of publications become significantly lower, only 210 of the 43
000 papers mentioned above. If the search is refined by adding the criteria scavengers OR antioxidant only 12 articles fit the search. Moreover if they are closely examined actually only five of them13–17 look into the free-radical scavenging activity (or antioxidant activity) of carbon nanotubes (Fig. 1). This is quite surprising. Therefore it is the main goal of this paper to draw attention to this, rather unexplored, potential application of CNTs. With that purpose in mind the present manuscript is intended to cover recent progress and future directions in the exciting, but still emerging, area of free-radical scavenging by carbon nanotubes.
Carbon nanotubes, their amazing properties and functionalization through reactions with free radicals
Single-walled carbon nanotubes (SWCNTs) are cylindrical molecules composed of carbon atoms that can be thought of as a rolled-up graphene sheet. Their structures (diameter and helicity) can be unambiguously defined by a chiral vector that represents the roll up direction: | Ch = (n,m) = na1 + ma2 | (1) |
where a1 and a2 denote equivalent lattice vectors of the graphene sheet, and n and m are integers (0 ≤ |m| ≤ n) (Fig. 2).
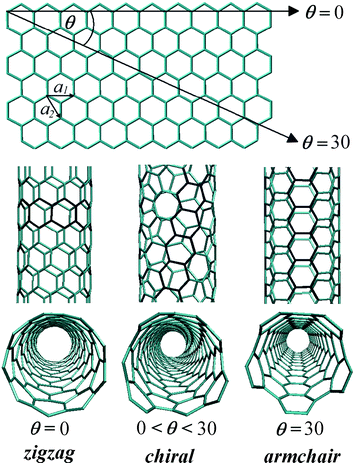 |
| Fig. 2 Definition of the roll-up vector as linear combinations of base vectors a1 and a2. Zigzag: θ = 0 (n, 0), armchair: θ = 30 (n, n), chiral: 0 < θ < 30 (n, m). | |
The fascination with CNTs is well justified by their remarkable mechanical and electrical properties,18–20 which arise from their peculiar structures. These unique properties make them ideal candidates for diverse nanotechnological applications. They have been proposed as nanopipettes,21 nanotweezers,22 nanocontainers,23 and field-emission devices,24–26 as well as for chemical detection,27,28 gas storage,29–31 membrane separation,32,33 and drug delivery and diagnostic,34 among many other applications. However, their lack of solubility limits the use of CNTs. Actually, pristine CNTs are insoluble in all organic solvents and aqueous solutions.35 Chemical functionalization of CNTs is one of the possible ways to overcome this problem. The strategies for such a process can be categorized in three general groups:35 (a) covalent attachment of chemical groups to the CNTs' walls; (b) noncovalent exohedral adsorption (wrapping) of various functional molecules; and (c) endohedral filling of the inner empty cavity.
CNTs constitute large arrays of conjugated double bonds, and therefore they are expected to show great electron donor and acceptor capabilities. Accordingly, they are expected to easily cope with a lack or excess of electrons. In a similar way, fullerenes are often referred to as electron sponges.36,37 Therefore CNTs are expected to react easily with free radicals. In fact such reactions have been proven to be an efficient way to achieve sidewall covalent functionalization.38–46 Some common radical precursors are diazonium salts 47–50 and peroxides,40,51–54 but there are also other approaches based on perfluoroalkyl iodides,55,56 perfluoro azo compounds,57,58hydroxylation,53 thiolation,59 microwave discharge of ammonia,60 and alkoxy radicals.46 Therefore it seems fair to say that there is abundant information on the chemistry of CNTs + free radicals reactions. In addition, other compounds with long conjugated C
C chains, like carotenoids for example, have been proven to be excellent free-radical scavengers.61,62 There are also several reports on the ability of fullerenes,63–65graphite,66 and graphene67 to easily react with free radicals. Thus, it is logical to wonder if CNTs can also be used as free-radical traps. This would be a very useful application since free radicals are known to be highly damaging species in very diverse systems, such as the atmosphere or living organisms.
Free radicals and the damage they can cause
Chemically free radicals (FRs) are species containing one or more unpaired electrons, that are capable of independent existence. Most radicals that occur in vivo are, or arise from, reactive oxygen species (ROS) or reactive nitrogen species (RNS). ROS include oxygen-based free radicals, like the superoxide anion (O2˙−), hydroxyl (OH˙), alkoxyl (RO˙), peroxyl (ROO˙) and hydroperoxyl (ROOH˙) radicals. RNS include peroxynitrite (ONOO−), nitric oxide (NO˙) and nitrogen dioxide (NO2˙). ROS and RNS can be either harmful or beneficial to living systems,68 depending on their concentration. In healthy organisms there is a delicate balance between the production and the removal of free radicals, which guarantees that they remain in low/moderate concentrations. Under such conditions free radicals have beneficial effects. They are necessary for the maturation process of cellular structures,69 and induce mitogenic response.70–73 FRs are also involved in the defense system, phagocytes release them to destroy pathogenic microbes.74,75 They also participate in cellular signaling systems,70,72,73,76 and seem to play a role in the regulation of insulin receptorkinase activity74 and in the apoptosis of defective cells.77 In summary, free radicals at low/moderate levels are essential to human health.
In contrast, at high concentrations FRs can be very harmful to living organisms. Such high concentrations are caused by an imbalance between the production and the consumption of free radicals, which is commonly referred to as oxidative stress (OS). More than 50 years ago Gerschman and coworkers78 found out that FRs were the toxic intermediates associated with oxygen poisoning and ionizing radiation. Surprisingly, despite its implications, not enough attention was paid to this discovery at the time. Nowadays the role of FRs in the development of a large variety of chronic and degenerative diseases is well documented. It has been established that oxidative damage to DNA is responsible for cancer development.68,72,73,76,79–83 OS is involved in several neurological disorders such as Parkinson's disease, Alzheimer's disease, multiple sclerosis, memory loss, and depression.84–91 There is also evidence to support the role of OS in several cardiovascular diseases like atherosclerosis, cardiac hypertrophy, ischemia, hypertension, cardiomyopathy, and congestive heart failure.74,92–100 In addition OS has been associated with ocular,101,102 renal,74,103 and pulmonary104–107 diseases; rheumatoid arthritis,108 fetal growth restriction and pre-eclampsia in prenatal medicine.109–112 According to such overwhelming data it is evident that an excess of free radicals can be very dangerous to human beings.
But why can free radicals be present at dangerous concentrations in our body? FRs are not only produced by endogenous sources but also by exogenous ones. Endogenous free radicals are generated from immune cell activation, inflammation, mental and physical stress, excessive exercise, ischemia, infection, cancer, and aging. Exogenous FRs come from environmental pollution, cigarette smoke, alcohol, heavy or transition metals, certain drugs, and radiation.69–76,83,113–116 Therefore efficiently fighting free radicals to keep them at healthy low/moderate concentrations is a difficult task. To accomplish this task, it is important to guarantee a high enough concentration of scavengers within us and find ways to reduce the concentration of free-radicals around us. Could CNTs be used for both tasks?
As it was analyzed above the very structure of CNTs suggests that they should be very reactive towards free radicals. Other compounds with long conjugated C
C chains, like carotenoids for example, have been proven to be excellent free-radical scavengers.61,62FullereneC60 has been proven to retard the azoisobutyronitrile (AIBN)-initiated polymerization of styrene117 and methyl methacrylate,118 and to act as an antioxidant by reacting with oxygen-centered radicals.119
Thus, it seems logical to hypothesize that CNTs could also be very efficient as free-radical scavengers. Therefore it is quite surprising that despite of the importance of the possible applications of such a valuable chemical property very little research has been devoted to it. CNTs, and particularly SWCNTs, lie in the low-size frontier of experimental techniques and in the large-size frontier of theoretical methods. Both approaches complement each other and can contribute different insights to enhance the knowledge, and consequently the potential applications of these small structures
Chronologically, Watts et al.13 were the first to report that multi-walled carbon nanotubes (MWCNTs) and boron-doped CNTs (BCNTs), with boron content of ∼1%, can act as antioxidants. These authors found that the oxidation of polystyrene, polyethylene, polypropylene and poly(vinylidene fluoride) is retarded by the presence of carbon nanotubes. This effect was reported to be weak compared to conventional phenolic antioxidants but comparable with the effect of carbon black. Based on previous studies that had shown that BCNTs have localized states due to the presence of lattice defects,120 and the inference from refs. 121 and 122 that localized states on CNTs are acceptor-like, Watts et al.13 concluded that the acceptor-like electronic properties of CNTs are mainly responsible for the radical termination during polymer degradation. In addition they also found that BCNT–polymer composites have higher onset oxidation temperatures than non-doped CNTs. This finding is in line with the suggestion of Hsu et al.120 that the radical-accepting capacity of the CNTs can be further enhanced via incorporation of boron into the CNT lattice. In the case of poly(vinylidene fluoride) Watts et al.13 found that CNTs can also act as halogen absorbers.
Three years latter Fenoglio et al.14 tested the scavenging activity of MWCNTs for hydroxyl and superoxide anion radicals. They were initially concerned about the toxicity of CNTs. Some previous reports had proposed that CNTs exhibit low or no toxicity in vitro123,124 or in vivo,125 while other studies had raised serious concerns about the potential hazard associated with the exposure of workers, users, or consumers to this type of material.126–139 According to the data gathered by Fenoglio et al.14MWCNTs in aqueous suspension do not generate oxygen or carbon-centered free radicals in the presence of H2O2 or formate, respectively. On the contrary, they observed that MWCNTs exhibit a remarkable radical scavenging capacity, when in contact with an external source of hydroxyl or superoxide radicals. Apparently, inflammatory reactions reported in vivo are related to something other than particle-derived free-radical generation. Unpurified SWCNTs, containing a large amount of iron were able to stimulate and induce the production of ROS.140,141 On the other hand, no ROS production was detected when a macrophagecell line was stimulated with purified SWCNTs.142 Thus it seems that impurities and not CNTs themselves are responsible for the reported toxicity. In any case, the study of CNTs aimed at biomedical applications is still and active area of research.125,143,144
After the experimental works by Watts et al.13 and Fenoglio et al.,14 the first theoretical study on the free-radical scavenging activity of SWCNTs was reported in 2008.15 In that work Density Functional Theory (DFT) was used to model the reactions of a finite (5,5) armchair SWCNT, about 8 Å long, with six different radicals: ˙OH, ˙OOH, ˙CH3, ˙OCH3, ˙OOCH3, and ˙CHO. Subsequent additions, up to four ˙OH radicals were also modeled. All the studied reactions were found to be exothermic. They also were found to be exergonic, except those involving ˙OOH and ˙OOCH3 radicals, which were reported to be slightly endergonic (Fig. 3). The scavenging processes were predicted to occur in a very fast way. With the exception of reactions involving ˙OOH and ˙OOCH3 radicals the rate constants per addition site were predicted to be very close to the diffusion limit. The presence of non-polar environments was suggested to enhance the reactivity of SWCNT fragments towards free radicals, compared to the gas phase.
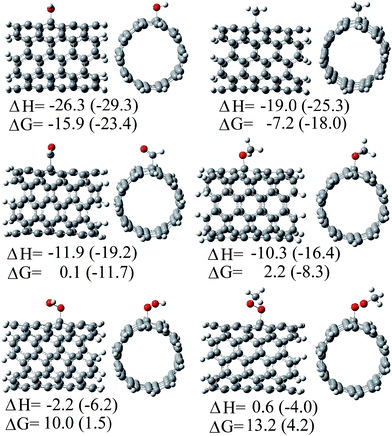 |
| Fig. 3 Addition products of the reactions between a (5,5) SWCNT fragment and different free radicals, as predicted in ref. 15. Energy values are reported in kcal mol−1. | |
Probably the most relevant finding from ref. 15 was that once a first radical is attached to the tube, further additions are increasingly feasible, taking place in a cascade way (Fig. 4). This suggests that SWCNTs can act as free-radical sponges. For multiple ˙OH additions the products of reaction were predicted to have their OH moieties distributed in groups rather than regularly spread on the carbon lattice. This was rationalized in terms of intramolecular interactions involving neighboring OH groups. In the same work it was proposed that the properties of the SWCNTs can be manipulated by controlling the number of free radicals attached to their walls.
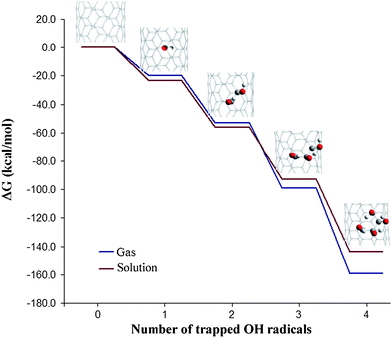 |
| Fig. 4 Cascade-like energetic profile corresponding to consecutive ˙OH additions to the wall of a (5,5) SWCNT fragment. | |
Shortly after the previously discussed theoretical work, Lucente-Schultz et al.16 reported a new experimental research on the antioxidant ability of SWCNTs. Their results supported the theoretical prediction that pristine SWCNTs are powerful antioxidants.15 As mentioned before the scarce solubility of CNTs is a limitation to their potential uses that can be overcome by proper functionalization. Lucente-Schultz et al.16 took into account this important feature and performed a comparative study between the free-radical scavenging activity of pristine and functionalized SWCNTs and ultra-short SWCNTs (US-SWCNTs) with derivatives of the phenolic antioxidant, butylated hydroxytoluene (BHT). For that purpose they used the oxygen radical absorbance capacity (ORAC) assay . Their results demonstrated that both types of SWCNTs are extremely effective antioxidants. In fact the oxygen-radical scavenging activity of the SWCNTs was reported to be about 40 times greater than that of the radioprotective dendritic fullerene. Their ORAC results also revealed that the trends in the antioxidant capability of SWCNTs depends on the type of functionalization. It was found that their antioxidant activity increases when the functionalization occurs through existing functionalities on the sidewall. On the other hand, the antioxidant activity decreases if the BHT-derivative functionalization takes place through covalent addition to the sidewall. Thus it was concluded that the non-functionalized SWCNTs are better radical scavengers than their BHT derivatives. In addition Lucente-Schultz et al.16 also carried out cytotoxicity assays , which showed that concentrations of both non-functionalized and BHT-derivatized SWCNTs, up to 330 nM or 83 mg L−1, have little or no deleterious effect on cell viability. Accordingly they proposed SWCNTs as attractive agents for antioxidant materials and medical therapeutics research.
As proven by Lucente-Schultz et al.,16 for functionalized SWCNTs, their FR scavenging activity can be very sensitive to structural modifications. How important is this? Actually it is extremely important for SWCNTs. It is known that their production does not yield only one kind of well-defined molecule. On the contrary, SWNTs preferentially aggregate into bundles of different characteristics. These mixtures of SWNTs widely vary in length, diameter, helicity and kind, location, and number of defects.145–147 Actually producing SWNTs of defined structures is a major technological challenge.148,149 Therefore it is important to estimate the influence of the above mentioned features on the free-radical scavenging activity of SWCNTs.
A theoretical work dealing with this matter has been reported very recently.17 In this study the reactions of the ˙OCH3 radical with SWCNT fragments of different lengths, diameters and helicity (also referred to as chirality) were modeled using DFT. The studied SWCNTs were armchair and zigzag fragments with diameters and lengths ranging from 0.4 to 1.1 nm, and from 0.7 to 2.0 nm (3 to 8 hexagons long), respectively. It was proposed that SWCNTs with wide distributions of diameter, length and helicity can have good free radical scavenging activity, and that they can be used as free radical traps with potential application in environmental and biological systems. In general thinner tubes were described to have better antiradical activities. The role of the curvature of the tubes was found to be particularly important for armchair nanotubes. Consequently for wide distributions of tube diameter zigzag SWCNTs are expected to be more efficient as free radical scavengers than the armchair ones. It was also found that the length of the tubes has a minor influence in the free radical trapping efficiency of SWCNTs. Taking into account all these findings together, thin and zig-zag nanotubes were recommended as those with the best antiradical activity, regardless of their length.
Based on the above discussed works, it can be said that some progress has been made on the understanding of the potential role of CNTs as efficient free-radical scavengers. However this promising area of research is still in its initial stage and much more work should be devoted to it before practical applications can be implemented.
Future directions
There are still many open questions that need to be answered before practical applications can be implemented that involve CNTs as free-radical scavengers. Thus further experimental and theoretical investigations in this area would be of great importance. But how can we use CNTs to scavenge free radicals and hopefully reduce oxidative stress? Even though practical applications do not lie within my area of expertise, I can imagine them grouped into two main classes: for inner use (FIU, those application that imply that at some point CNTs will be inside living organisms) and for outer use (FOU, those warranting that CNTs will never enter living organisms). The main role of the FOU class would be to reduce the amount of free radicals that reach leaving organisms from exogenous sources. Some hypothetical applications within this class that easily come to mind are: as fillings in smog masks and in cigarettes filters, or even as a component of the exhaust system for vehicles. The main role of the applications of CNTs within the FIU class, on the other hand, would be to help fight the excess of free radicals already inside the human body. Such applications would imply the intake of CNTs. Therefore major concerns about toxicity arise.
In fact the similarity between carbon nanotubes and asbestos, regarding their fiber shape, has raised serious concerns related to the risks from respiratory exposure. Poland et al. have found that exposing the mesothelial lining of the body cavity of mice, to long multi-walled carbon nanotubes results in asbestos-like, length-dependent, pathogenic behaviour.150 They also pointed out the need for further research on this topic. Takagi et al. have also suggested the possibility that carbon-made fibrous or rod-shaped micrometre particles may share the carcinogenic mechanisms postulated for asbestos.151 However, as Kane and Hurt have pointed out, it would be premature to declare carbon nanotubes a major risk factor for mesothelioma in humans.152 In the same publication they explained in detail the reasons for this claim. In addition they have also drawn attention to the fact that in the case of carbon nanotubes there is still a ‘window of opportunity’ to develop safe material design and manufacturing strategies before commercialization becomes widespread. This obviously implies that the cause of the potential toxicity must be clearly identified.
Toxicity is definitively an area of major concern regarding the use of CNTs and should be thoroughly investigated before we even dream about any application within the FIU class.
Some questions related to the toxicity of CNTs that still need to be answered are for example:
• Are pristine CNTs intrinsically toxic or does their toxicity arise from metal impurities and structural defects?
• Are SWCNTs more or less toxic than MWCNTs?
• Are doped CNTs toxic? If so, which dopants increase toxicity?
• Is it possible to find dopants that decrease CNT toxicity?
• When CNTs are functionalized to increase their solubility, what is the effect of each particular functionalization on the toxicity?
Two very recent and simultaneous works have been reported dealing with the first of the above mentioned questions. Fenoglio et al. have found that structural defects play a major role in the acute lung toxicity of MWCNTs.153 They showed that the presence of metals (Fe, Co), defects, hydrophilic oxygen functionalities, or adsorbed toxic molecules is related to the toxicity of MWCNTs. Similar conclusions are discussed in the companion paper by Muller et al.154
Before we start thinking about practical applications it is also necessary to fully understand the FR-scavenging mechanism of CNTs. Moreover it is important to know if there are viable ways to enhance such a desirable capability. As mentioned before the production of CNTs yields a wide variety of structures and for many other properties there is a direct relationship between structure and behaviour. Therefore it is important to know if there are optimum structures for the FR-scavenging activity of CNTs and another set of questions arises:
• What is the influence of length, diameter and helicity on the FR-scavenging activity of CNTs?
• Are SWCNTs better or worse free-radical scavengers than MWCNTs?
• Are there dopants capable of significantly increasing the FR-scavenging activity of CNTs?
• What is the effect of the different functionalizations on such activity?†
• Are capped SWCNTs better or worse free-radical scavengers than the open-ended ones?
• Could the free-radical scavenging activity of CNTs be so strong that it might damage some of the natural processes governed by free radicals?
In addition it is known that defects are often present in CNTs.155 Moreover, it has been shown that these defects play a central role in the modification of the physical156–168 and chemical169–178 properties of SWCNTs. In addition to dopants and functionalizations (already discussed), point defects on the sidewalls of SWCNTs have also been described. There are three kinds of them: vacancies, add-atoms, and Stone–Wales defects; and more can be formed by a combination of two or more of them.165 Some examples are shown in Fig. 5.
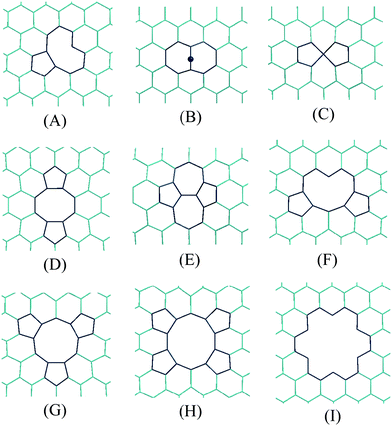 |
| Fig. 5 Some examples of structural defects in the carbon lattice of SWCNTs. | |
Since such defects are expected to modify the chemical properties of the SWCNTs169–178 new questions arise related to the influence of the defects on the free-radical scavenging activity of carbon nanotubes. Some of them are:
• Do the point defects increase or decrease the reactivity of the SWCNTs toward free radicals?
• All kinds of point defects affect the free-radical scavenging activity of carbon nanotubes in the same way?
• Which are the most and the least reactive sites within every kind of defect?‡
There are many more possible questions related with the efficiency of CNTs as free-radical scavengers. What in my opinion is clear is that this is a fascinating and very promising area of research. Hopefully, in the near future there will be abundant papers devoted to it and many of the current questions will be answered.
Conclusions
The potential role of carbon nanotubes as free-radical scavengers is still an emerging area of research. So far encouraging results have been reported indicating that CNTs can be very efficient as free-radical scavengers. Significant progress has been made however in the chemical reactions between CNTs and free radicals for functionalization purposes, which can provide valuable information on the chemistry of the intended processes. There are abundant open questions related to the possible use of CNTs for scavenging free radicals, and consequently for fighting oxidative stress. However the information gathered so far is promising and the important implications of such activity plenty justifies further researches on this subject.
References
- S. Iijima, Nature, 1991, 354, 56–58 CrossRef CAS.
- M. Monthioux and V. L. Kuznetsov, Carbon, 2006, 44, 1621–1623 CrossRef CAS.
-
T. V. Hughes, C. R. Chambers, US Patent 405480, 1889.
- P. Schutzenberger and L. Schutzenberger, Acad. Sci. Paris, 1890, 111, 774–778 Search PubMed.
- C. Pelabon and H. Pelabon, Acad. Sci. Paris, 1903, 137, 706–708 Search PubMed.
- A. Alexiadis and S. Casinos, Chem. Rev., 2008, 108, 5014–5034 CrossRef CAS.
- L. V. Radushkevich and V. M. Lukyanovich, Zurn. Fisic. Chim., 1952, 26, 88–95 Search PubMed.
- M. Hillert and N. Lange, Z Kristallogr., 1958, 111, 24–34 CAS.
- R. T. K. Baker, P. S. Harris, R. B. Thomas and R. J. Waite, J. Catal., 1973, 30, 86–95 CAS.
- S. Iijima and T. Ichihashi, Nature, 1993, 363, 603–605 CrossRef CAS.
- D. S. Bethune, C. H. Kiang, M. S. De Vries, G. Gorman, R. Savoy, J. Vazquez and R. Beyers, Nature, 1993, 363, 605–607 CrossRef CAS.
-
Scopus Citation Index, http://www.scopus.com Search PubMed.
- P. C. P. Watts, P. K. Fearon, W. K. Hsu, N. C. Billingham, H. W. Kroto and D. R. M. Walton, J. Mater. Chem., 2003, 13, 491–495 RSC.
- I. Fenoglio, M. Tomatis, D. Lison, J. Muller, A. Fonseca, J. B. Nagy and B. Fubini, Free Radical Biol. Med., 2006, 40, 1227–1233 CrossRef CAS.
- A. Galano, J. Phys. Chem. C, 2008, 112, 8922–8927 CrossRef CAS.
- R. M. Lucente-Schultz, V. C. Moore, A. D. Leonard, B. K. Price, D. V. Kosynkin, M. Lu, R. Partha, J. L. Conyers and J. M. Tour, J. Am. Chem. Soc., 2009, 131, 3934–3941 CrossRef CAS.
- A. Galano, J. Phys. Chem. C, 2009, 113, 18487–18491 CrossRef CAS.
- P. M. Ajayan, Chem. Rev., 1999, 99, 1787 CrossRef CAS.
- M. F. Yu, B. S. Files, S. Arepalli and R. S. Ruoff, Phys. Rev. Lett., 2000, 84, 5552–5555 CrossRef CAS.
- J. Hone, B. Batlogg, Z. Benes, A. T. Johnson and J. E. Fischer, Science, 2000, 289, 1730–1733 CrossRef CAS.
- M. H. Hong, K. H. Kim, J. Bae and W. Jhe, Appl. Phys. Lett., 2000, 77, 2604–2606 CrossRef CAS.
- P. Kim and C. M. Lieber, Science, 1999, 286, 2148–2150 CrossRef CAS.
- M. Koshino, T. Tanaka, N. Solin, K. Suenaga, H. Isobe and E. Nakamura, Science, 2007, 316, 853 CrossRef CAS.
- W. A. de Heer, A. Chatelain and D. Ugarte, Science, 1995, 270, 1179–1180 CrossRef CAS.
- A. G. Rinzler, J. H. Hafner, P. Nikolaev, P. Nordlander, D. T. Colbert, R. E. Smalley, L. Lou, S. G. Kim and D. Tomanek, Science, 1995, 269, 1550–1553 CrossRef CAS.
- Y. Saito and S. Uemura, Carbon, 2000, 38, 169–182 CrossRef CAS.
- J. Kong, N. R. Franklin, C. Zhou, M. G. Chapline, S. Peng, K. Cho and H. Dai, Science, 2000, 287, 622–625 CrossRef CAS.
- E. S. Snow, F. K. Perkins, E. J. Houser, S. C. Badescu and T. L. Reinecke, Science, 2005, 307, 1942–1945 CrossRef CAS.
- C. Liu, Y. Y. Fan, M. Liu, H. T. Cong, H. M. Cheng and M. S. Dresselhaus, Science, 1999, 286, 1127–1129 CrossRef CAS.
- S. M. Lee, K. H. An, Y. H. Lee, G. Seifert and T. Frauenheim, J. Am. Chem. Soc., 2001, 123, 5059–5063 CrossRef CAS.
- D. Cao, X. Zhang, J. Chen, W. Wang and J. Yun, J. Phys. Chem. B, 2003, 107, 13286–13292 CrossRef CAS.
- B. J. Hinds, N. Chopra, T. Rantell, R. Andrews, V. Gavalas and L. G. Bachas, Science, 2004, 303, 62–65 CrossRef CAS.
- A. Kalra, S. Garde and G. Hummer, Proc. Natl. Acad. Sci. U. S. A., 2003, 100, 10175–10180 CrossRef.
- M. Prato, K. Kostarelos and A. Bianco, Acc. Chem. Res., 2008, 41, 60–68 CrossRef CAS.
- D. Tasis, N. Tagmatarchis, A. Bianco and M. Prato, Chem. Rev., 2006, 106, 1105–1136 CrossRef CAS.
- D. Dubois, K. M. Kadish, S. Flanagan and L. J. Wilson, J. Am. Chem. Soc., 1991, 113, 7773–7774 CrossRef CAS.
- Q. S. Xie, E. Perezcordero and L. Echegoyen, J. Am. Chem. Soc., 1992, 114, 3978–3980 CrossRef.
- D. Pantarotto, C. D. Partidos, R. Graff, J. Hoebeke, J. P. Briand, M. Prato and A. Bianco, J. Am. Chem. Soc., 2003, 125, 6160–6164 CrossRef CAS.
- M. Holzinger, J. Abraham, P. Whelan, R. Graupner, L. Ley, F. Hennrich, M. Kappes and A. Hirsch, J. Am. Chem. Soc., 2003, 125, 8566–8580 CrossRef CAS.
- H. Peng, L. B. Alemany, J. L. Margrave and V. N. Khabashesku, J. Am. Chem. Soc., 2003, 125, 15174–15182 CrossRef CAS.
- Y. Ying, R. K. Saini, F. Liang, A. K. Sadana and W. E. Billups, Org. Lett., 2003, 5, 1471–1473 CrossRef CAS.
- H. Kong, C. Cao and D. Yan, J. Am. Chem. Soc., 2004, 126, 412–413 CrossRef CAS.
- Y. Liu, Z. Yao and A. Adronov, Macromolecules, 2005, 38, 1172–1179 CrossRef CAS.
- J. Chattopadhyay, F. J. Cortez, S. Chakraborty, N. K. H. Slater and W. E. Billups, Chem. Mater., 2006, 18, 5864–5868 CrossRef CAS.
- D. Yang, J. Hu and C. Wang, Carbon, 2006, 44, 3161–3167 CrossRef CAS.
- P. S. Engel, W. E. Billups, D. W. Abmayr, K. Tsvaygboym and R. Wang, J. Phys. Chem. C, 2008, 112, 695–700 CrossRef CAS.
- J. L. Bahr, J. Yang, D. V. Kosynkin, M. J. Bronikowski, R. E. Smalley and J. M. Tour, J. Am. Chem. Soc., 2001, 123, 6536–6542 CrossRef CAS.
- C. A. Dyke, M. P. Stewart, F. Maya and J. M. Tour, Synlett, 2004, 1, 155–160.
- P. R. Marcoux, P. Hapiot, P. Batail and J. Pinson, New J. Chem., 2004, 28, 302–307 RSC.
- J.-x. Zhao and Y.-h. Ding, J. Phys. Chem. C, 2008, 112, 13141–13149 CrossRef CAS.
- H. Peng, P. Reverdy, V. N. Khabashesku and J. L. Margrave, Chem. Commun., 2003, 362–363 RSC.
- P. Umek, J. W. Seo, K. Hernadi, A. Mrzel, P. Pechy, D. D. Mihailovic and L. Forró, Chem. Mater., 2003, 15, 4751–4755 CrossRef CAS.
- F. Liang, J. M. Beach, P. K. Rai, W. Guo, R. H. Hauge, M. Pasquali, R. E. Smalley and W. E. Billups, Chem. Mater., 2006, 18, 1520–1524 CrossRef CAS.
- M. Zhang, M. Yudasaka, Y. Miyauchi, S. Maruyama and S. Ijima, J. Phys. Chem. B, 2006, 110, 8935–8940 CrossRef CAS.
- K. M. Lee, L. Li and L. Dai, J. Am. Chem. Soc., 2005, 127, 4122–4123 CrossRef CAS.
- M. Holzinger, O. Vostrowsky, A. Hirsch, F. Hennrich, M. Kappes, R. Weiss and F. Jellen, Angew. Chem., Int. Ed., 2001, 40, 4002–4005 CrossRef CAS.
- T. Nakamura, M. Ishihara, T. Ohana, A. Tanaka and Y. Koga, Chem. Commun., 2004, 1336–1337 RSC.
- J. Liu, M. Rodriguez i Zubiri, B. Vigolo, M. Dossot, Y. Fort, J.-J. Ehrhardt and E. McRae, Carbon, 2007, 45, 885–891 CrossRef CAS.
- P. A. Denis, Int. J. Quantum Chem., 2009, 109, 772–781 CrossRef CAS.
- B. N. Khare, P. Wilhite, R. C. Quinn, B. Chen, R. H. Schlingler, B. Tran, H. Imanaka, C. R. So, C. W. Bauschlicher and M. Meyyappan, J. Phys. Chem. B, 2004, 108, 8166–8172 CrossRef CAS.
- N. I. Krinsky, Annu. Rev. Nutr., 1993, 13, 561–587 CrossRef CAS.
- R. Edge, D. J. McGarvey and T. G. Truscott, J. Photochem. Photobiol., B, 1997, 41, 189–200 CrossRef CAS.
- J. R. Morton, K. F. Preston, P. J. Krusic, S. A. Hill and E. Wasserman, J. Phys. Chem., 1992, 96, 3576–3578 CrossRef CAS.
- R. Borghi, L. Lunazzi, G. Placucci, P. J. Krusic, D. A. Dixon, N. Matsuzawa and M. Ata, J. Am. Chem. Soc., 1996, 118, 7608–7617 CrossRef CAS.
- L. Gan, S. Huang, X. Zhang, A. Zhang, B. Cheng, H. Cheng, X. Li and G. Shang, J. Am. Chem. Soc., 2002, 124, 13384–13385 CrossRef CAS.
- A. Jelea, F. Marinelli, Y. Ferro, A. Allouche and C. Brosset, Carbon, 2004, 42, 3189–3198 CrossRef CAS.
- P. A. Denis, J. Phys. Chem. C, 2009, 113, 5612–5619 CrossRef CAS.
- M. Valko, M. Izakovic, M. Mazur, C. J. Rhodes and J. Telser, Mol. Cell. Biochem., 2004, 266, 37–56 CrossRef CAS.
- L. A. Pham-Huy, H. He and C. Pham-Huy, Int. J. Biomed. Sci., 2008, 4, 89–96 Search PubMed.
- P. Pacher, J. S. Beckman and L. Liaudet, Physiol. Rev., 2007, 87, 315–424 CrossRef CAS.
- M. Genestra, Cell. Signalling, 2007, 19, 1807–1819 CrossRef CAS.
- M. Valko, D. Leibfritz, J. Moncol, M. T. D. Cronin, M. Mazura and J. Telser, Int. J. Biochem. Cell Biol., 2007, 39, 44–84 CrossRef CAS.
- M. Valko, C. J. Rhodes, J. Moncola, M. Izakovic and M. Mazura, Chem.-Biol. Interact., 2006, 160, 1–40 CrossRef CAS.
- W. Droge, Physiol Rev., 2002, 82, 47–95.
- I. Young and J. Woodside, J. Clin. Pathol., 2001, 54, 176–186 CrossRef CAS.
- B. Halliwell, Biochem. Soc. Trans., 2007, 35, 1147–1150 CrossRef CAS.
- T. P. A. Devasagayam, J. C. Tilak, K. K. Boloor, K. S. Sane, S. S. Ghaskadbi and R. D. Lele, J. Assoc. Physic. Ind, 2004, 52, 794–804 Search PubMed.
- R. Gerschman, D. L. Gilbert, S. W. Nye, P. Dwyer and W. O. Fenn, Science, 1954, 119, 623–626 CAS.
- N. F. Boyd and V. McGuire, Free Radical Biol. Med., 1991, 10, 185–190 CrossRef CAS.
- R. L. Nelson, Free Radical Biol. Med., 1992, 12, 161–168 CrossRef CAS.
- P. Knekt, A. Reunanen, H. Takkunen, A. Aromaa, M. Heliovarara and T. Hakulinen, Int. J. Cancer, 1994, 56, 379–382 CrossRef CAS.
- G. S. Omenn, G. E. Goodman and M. D. Thornquist, N. Engl. J. Med., 1996, 334, 1150–1155 CrossRef CAS.
- J. K. Willcox, S. L. Ash and G. L. Catignani, Crit. Rev. Food Sci. Nutr., 2004, 44, 275–295 CrossRef CAS.
- D. A. Butterfield, K. Hensley, M. Harris, M. Mattson and J. Carney, Biochem. Biophys. Res. Commun., 1994, 200, 710–715 CrossRef CAS.
- K. Hensley, J. M. Carney, M. P. Mattson, M. Aksenova, M. Harris, J. F. Wu, R. A. Floyd and D. A. Butterfield, Proc. Natl. Acad. Sci. U. S. A., 1994, 91, 3270–3274 CAS.
- D. A. Butterfield, L. Martin, J. M. Carney and K. Hensley, Life Sci., 1995, 58, 217–228 CrossRef CAS.
- D. A. Butterfield, Chem. Res. Toxicol., 1997, 10, 495–506 CrossRef CAS.
- M. P. Mattson, Alz. Dis. Rev., 1997, 2, 1–14 Search PubMed.
- Y. Christen, Am. J. Clin. Nutr, 2000, 71, 621S–629S CAS.
- B. Halliwell, Drugs Aging, 2001, 18, 685–716 CrossRef CAS.
- D. A. Butterfield, Free Radical Res., 2002, 36, 1307–1313 CrossRef CAS.
- D. R. Janero, Free Radical Biol. Med., 1991, 11, 129–144 CrossRef CAS.
- D. Steinberg, Circulation, 1991, 84, 1421–1430.
- R. A. Riemersma, D. A. Wood, C. C. A. Macintyre, R. A. Elton, K. F. Gey and M. F. Oliver, Lancet, 1991, 337, 1–5 CrossRef CAS.
- J. T. Salonen, K. Nyyssoner, H. Korpela, J. Tuomilehto, R. Seppanen and R. Salonen, Circulation, 1992, 86, 803–811 CAS.
- D. A. Street, G. Comstock, R. Salkeld and M. Klag, Circulation, 1994, 90, 1154–1161 CAS.
- H. N. Hodis, W. J. Mack, L. LaBree, L. Cashin-Hemphill, A. Sevanian, R. Johnson and S. Azen, JAMA, J. Am. Med. Assoc., 1995, 273, 1849–1854 Search PubMed.
- L. H. Kushi, A. R. Folsom, R. J. Prineas, P. J. Mink, Y. Wu and R. Bostick, N. Engl. J. Med., 1996, 334, 1156–1162 CrossRef CAS.
- N. G. Stephens, A. Parsons, M. J. Brown, P. M. Schofield, F. Kelly, K. Cheesman and M. J. Mitchinson, Lancet, 1996, 347, 781–786 CrossRef CAS.
- O. M. Panasenko, T. V. Nova, O. A. Azizova and Y. A. Vladimirov, Free Radical Biol. Med., 1991, 10, 137–148 CrossRef CAS.
- S. Beatty, H. H. Koh, M. Phil, D. Henson and M. Boulton, Surv. Ophthalmol., 2000, 45, 115–134 CrossRef CAS.
- C. H. Meyer and W. Sekundo, Dev. Ophthalmol., 2005, 103–119 Search PubMed.
- J. Galle, Nephrol., Dial., Transplant., 2001, 16, 2135–2142 CrossRef CAS.
- W. MacNee, Eur. J. Pharmacol., 2001, 429, 195–207 CrossRef CAS.
- G. Caramori and A. Papi, Thorax, 2004, 59, 170–173 CrossRef CAS.
- R. F. Guo and P. A. Ward, Antioxid. Redox Signaling, 2007, 9, 1991–2002 Search PubMed.
- Y. Hoshino and M. Mishima, Antioxid. Redox Signaling, 2008, 10, 701–704 Search PubMed.
- A. Mahajan and V. R. Tandon, J. Indian Rheumatol. Ass., 2004, 12, 139–142 Search PubMed.
- L. Myatt, J. Physiol, 2006, 572, 25–30 CAS.
- K. Braekke, N. K. Harsem and A. C. Staff, Pediatr. Res., 2006, 60, 560–564 CrossRef CAS.
- A. Biri, N. Bozkurt, A. Turp, M. Kavutcu, O. Himmetoglu and I. Durak, Gynecol. Obstet. Invest., 2007, 64, 187–192 Search PubMed.
- Z. Hracsko, H. Orvos, Z. Novak, A. Pal and I. S. Varga, Redox Rep., 2008, 13, 11–16 Search PubMed.
- S. Parthasarathy, N. Santanam, S. Ramachandran and O. Meilhac, J. Lipid. Res., 1999, 40, 2143–2157 CAS.
- D. F. Church and W. A. Pryor, Environ. Health Perspect., 1985, 64, 111–126 CrossRef CAS.
- P. Pasupathi, Y. Y. Rao, J. Farook, G. Saravanan and G. Bakthavathsalam, Res. J. Medic. Med. Sci., 2009, 44, 275–295 Search PubMed.
- M. Valko, H. Morris and M. T. D. Cronin, Curr. Med. Chem., 2005, 4, 151–159.
- D. Stewart and C. T. Imrie, Chem. Commun., 1996, 1383–1384 RSC.
- K. Kirkwood, D. Stewart and C. T. Imrie, J. Polym. Sci., Part A: Polym. Chem., 1997, 35, 3323–3325 CrossRef CAS.
- E. B. Zeinalov and G. Kobmehl, Polym. Degrad. Stab., 2001, 71, 197–202 CrossRef CAS.
- W. K. Hsu, S. Y. Chu, E. Munoz-Picone, J. L. Boldu, S. Firth, P. Franchi, B. P. Roberts, A. Schilder, H. Terrones, N. Grobert, Y. Q. Zhu, M. Terrones, M. E. McHenry, H. W. Kroto and D. R. M. Walton, Chem. Phys. Lett., 2000, 323, 572–579 CrossRef CAS.
- S. N. Song, X. K. Wang, R. P. H. Chang and J. B. Ketterson, Phys. Rev. Lett., 1994, 72, 697–700 CrossRef CAS.
- J. Hone, I. Ellwood, M. Muno, A. Mizel, M. L. Cohen, A. Zettl, A. G. Rinzler and R. E. Smalley, Phys. Rev. Lett., 1998, 80, 1042–1045 CrossRef CAS.
- S. Fiorito, A. Serafino, F. Andreola, A. Togna and G. Togna, J. Nanosci. Nanotechnol., 2006, 6, 591–599 CrossRef CAS.
- E. Flahaut, M. C. Durrieu, M. Remy-Zolghadri, R. Bareille and Ch. Baquey, Carbon, 2006, 44, 1093–1099 CrossRef CAS.
- T. K. Leeuw, R. M. Reith, R. A. Simonette, M. E. Harden, P. Cherukuri, D. A. Tsyboulski, K. M. Beckingham and R. B. Weisman, Nano Lett., 2007, 7, 2650–2654 CrossRef CAS.
- D. B. Warheit, B. R. Laurence, K. L. Reed, D. H. Roach, G. A. M. Reynolds and T. R. Webb, Toxicol. Sci., 2003, 77, 117–125 CrossRef.
- J. Muller, F. Huaux, N. Moreau, P. Misson, J. F. Heilier, M. Delos, M. Arras, A. Fonseca, J. B. Nagy and D. Lison, Toxicol. Appl. Pharmacol., 2005, 207, 221–231 CAS.
- N. A. Monteiro-Riviere, R. J. Nemanich, A. O. Inman, Y. Y. Y. Wang and J. E. Riviere, Toxicol. Lett., 2005, 155, 377–384 CrossRef CAS.
- L. H. Ding, J. Stilwell, T. T. Zhang, O. Elboudwarej, H. J. Jiang, J. P. Selegue, P. A. Cooke, J. W. Gray and F. Q. F. Chen, Nano Lett., 2005, 5, 2448–2464 CrossRef CAS.
- K. F. Soto, A. Carrasco, T. G. Powell, K. M. Garza and L. E. Murr, J. Nanopart. Res., 2005, 7, 145–169 CrossRef CAS.
- G. Jia, H. F. Wang, L. Yan, X. Wang, R. J. Pei, T. Yan, Y. L. Zhao and X. B. Guo, Environ. Sci. Technol., 2005, 39, 1378–1383 CrossRef CAS.
- M. Bottini, S. Bruckner, K. Nika, N. Bottini, S. Bellocci, A. Magrini, A. Bergamaschi and T. Mustelin, Toxicol. Lett., 2006, 160, 121–126 CrossRef CAS.
- A. Magrez, S. Kasas, V. Salicio, N. Pasquier, J. W. Seo, M. Celio, S. Catsicas, B. Schwaller and L. Forro, Nano Lett., 2006, 6, 1121–1125 CrossRef CAS.
- H. Grubek-Jaworska, P. Nejman, K. Czuminska, T. Przybyłlowski, A. Huczko, H. Lange, M. Bystrzejewski, P. Baranowski and R. Chazan, Carbon, 2006, 44, 1057–1063 CrossRef CAS.
- C.-w. Lam, J. T. James, R. McCluskey, S. Arepalli and R. L. Hunter, Crit. Rev. Toxicol., 2006, 36, 189–217 CrossRef CAS.
- P. Wick, P. Manser, L. K. Limbach, U. Dettlaff-Weglikowska, F. Krumeich, S. Roth, W. J. Stark and A. Bruinink, Toxicol. Lett., 2007, 168, 121–131 CrossRef CAS.
- Z. Li, T. Hulderman, R. Salmen, R. Chapman, S. S. Leonard, S. H. Young, A. Shvedova, M. I. Lustre and P. P. Simeonova, Environ. Health Perspect., 2007, 115, 377–382 CAS.
- C. S. Sharma, S. Sarkar, A. Periyakaruppan, J. Barr, K. Wise, R. Thomas, B. L. Wilson and G. T. Ramesh, J. Nanosci. Nanotechnol., 2007, 7, 2466–2472 CrossRef CAS.
- E. Bergamaschi, I. Iavicoli, O. Bussolati, A. Pietroiusti, A. Magrini and A. Bergamaschi, Int. J. Environ. Health., 2009, 3, 249–263 Search PubMed.
- A. A. Shvedova, V. Castranova, E. R. Kisin, D. Schwegler-Berry, A. R. Murray, V. Z. Gandelsman, A. Maynard and P. Baron, J. Toxicol. Environ. Health, Part A, 2003, 66, 1909–1926 Search PubMed.
- S. K. Manna, S. Sarkar, J. Barr, K. Wise, E. V. Barrera, O. Jejelowo, A. C. Rice-Ficht and G. T. Ramesh, Nano Lett., 2005, 5, 1676–1684 CrossRef CAS.
- A. A. Shvedova, E. R. Kisin, R. Mercer, A. R. Murray, V. J. Johnson, A. I. Potapovich, Y. Y. Tyurina, O. Gorelik, S. Arepalli, D. Schwegler-Berry, A. F. Hubbs, J. Antonini, D. E. Evans, B.-K. Ku, D. Ramsey, A. Maynard, V. E. Kagan, V. Castranova and P. Baron, Am. J. Physiol.: Lung Cell. Mol. Phys., 2005, 289, L698–L708 Search PubMed.
- L. Lacerda, A. Bianco, M. Prato and K. Kostarelos, Adv. Drug Delivery Rev., 2006, 58, 1460–1470 CrossRef CAS.
- L. Lacerda, S. Raffa, M. Prato, A. Bianco and K. Kostarelos, Nano Today, 2007, 2, 38–43 CrossRef.
- T. W. Odom, J.-L. Huang, P. Kim and C. M. Lieber, J. Phys. Chem. B, 2000, 104, 2794–2809 CrossRef CAS.
- A. Hirsch, Angew. Chem., Int. Ed., 2002, 41, 1853–1859 CrossRef CAS.
- Q. Liu, W. Ren, Z.-G. Chen, D.-W. Wang, B. Liu, B. Yu, F. Li, H. Cong and H.-M. Cheng, ACS Nano, 2008, 2, 1722–1728 CrossRef CAS.
- M. Zheng and E. D. Semke, J. Am. Chem. Soc., 2007, 129, 6084–6085 CrossRef CAS.
- E. Joselevich, H. Dai, J. Liu, K. Hata and A. H. Windle, Top. Appl. Phys., 2008, 111, 101–164 CAS.
- C. A. Poland, R. Duffin, I. Kinloch, A. Maynard, W. A. H. Wallace, A. Seaton, V. Stone, S. Brown, W. Macnee and K. Donaldson, Nat. Nanotechnol., 2008, 3, 423–428 CrossRef CAS.
- A. Takagi, A. Hirose, T. Nishimura, N. Fukumori, A. Ogata, N. Ohashi, S. Kitajima and J. Kanno, J. Toxicol. Sci., 2008, 33, 105–116 Search PubMed.
- A. B. Kane and R. H. Hurt, Nat. Nanotechnol., 2008, 3, 378–379 CrossRef CAS.
- I. Fenoglio, G. Greco, M. Tomatis, J. Muller, E. Raymundo-Piñero, F. Beguin, A. Fonseca, J. B. Nagy, D. Lison and B. Fubini, Chem. Res. Toxicol., 2008, 21, 1690–1697 CrossRef CAS.
- J. Muller, F. Huaux, A. Fonseca, J. B. Nagy, N. Moreau, M. Delos, E. Raymundo-Piñero and F. Beguin, Chem. Res. Toxicol., 2008, 21, 1698–1705 CrossRef CAS.
- X. Lu and Z. Chen, Chem. Rev., 2005, 105, 3643–3696 CrossRef CAS (and references therein).
- T. W. Ebbesen and T. Takada, Carbon, 1995, 33, 973–978 CrossRef CAS.
- J. C. Charlier, T. W. Ebbesen and Ph. Lambin, Phys. Rev. B: Condens. Matter, 1996, 53, 11108–11113 CrossRef CAS.
- V. H. Crespi and M. L. Cohen, Phys. Rev. Lett., 1997, 79, 2093–2096 CrossRef CAS.
- L. Chico, M. P. L. Sancho and M. C. Muñoz, Phys. Rev. Lett., 1998, 81, 1278–1281 CrossRef CAS.
- D. Orlikowski, M. B. Nardelli, J. Bernholc and C. Roland, Phys. Rev. Lett., 1999, 83, 4132–4135 CrossRef CAS.
- M. Ouyang, J. L. Huang, C. L. Cheung and C. M. Lieber, Science, 2001, 291, 97–100 CrossRef CAS.
- M. Ouyang, J. L. Huang and C. M. Lieber, Acc. Chem. Res., 2002, 35, 1018–1025 CrossRef CAS.
- J. C. Charlier, Acc. Chem. Res., 2002, 35, 1063–1069 CrossRef CAS.
- P. C. P. Watts, W. K. Hsu, H. W. Kroto and D. R. M. Walton, Nano Lett., 2003, 3, 549–553 CrossRef CAS.
- F. Ding, Phys. Rev. B: Condens. Matter Mater. Phys., 2005, 72, 245409 CrossRef.
- J. Kotakoski, A. V. Krasheninnikov and K. Nordlund, Phys. Rev. B: Condens. Matter Mater. Phys., 2006, 74, 245420 CrossRef.
- A. Galano and E. Orgaz, Phys. Rev. B: Condens. Matter Mater. Phys., 2008, 77, 045111 CrossRef.
- B. Xue, X. Shao and W. Cai, J. Chem. Theory Comput., 2009, 5, 1554–1559 CrossRef CAS.
- J. Liu, A. G. Rinzler, H. Dai, J. H. Hafner, R. K. Bradley, P. J. Boul, A. Lu, T. Iverson, K. Shelimov, C. B. Huffman, F. Rodriguez-Macias, Y. S. Shon, T. R. Lee, D. T. Colbert and R. E. Smalley, Science, 1998, 280, 1253–1256 CrossRef CAS.
- D. Bom, R. Andrews, D. Jacques, J. Anthony, B. Chen, M. S. Meier and J. P. Selegue, Nano Lett., 2002, 2, 615–619 CrossRef CAS.
- Y. P. Sun, K. Fu, Y. Lin and W. Huang, Acc. Chem. Res., 2002, 35, 1096–1104 CrossRef CAS.
- Y. Fan, M. Burghard and K. Kern, Adv. Mater., 2002, 14, 130–133 CrossRef CAS.
- M. Grujicic, G. Cao and R. Singh, Appl. Surf. Sci., 2003, 211, 166–183 CrossRef CAS.
- M. Grujicic, G. Cao, A. M. Rao, T. M. Tritt and S. Nayak, Appl. Surf. Sci., 2003, 214, 289–303 CrossRef CAS.
- N. Chakrapani, Y. M. Zhang, S. K. Nayak, J. A. Moore, D. L. Carroll, Y. Y. Choi and P. M. Ajayan, J. Phys. Chem. B, 2003, 107, 9308–9311 CrossRef CAS.
- X. Li, J. Niu, J. Zhang, H. Li and Z. Liu, J. Phys. Chem. B, 2003, 107, 2453–2458 CrossRef CAS.
- A. J. Lu and B. C. Pan, Phys. Rev. Lett., 2004, 92, 105504 CrossRef CAS.
- A. Galano and M. Francisco-Marquez, Chem. Phys., 2008, 345, 87–94 CrossRef CAS.
Footnotes |
† Our group is currently involved in a theoretical investigation on this topic for SWCNTs functionalized with carboxylic groups. |
‡ An ongoing investigation on this topic is being carried out in our group. |
|
This journal is © The Royal Society of Chemistry 2010 |
Click here to see how this site uses Cookies. View our privacy policy here.